DOI:
10.1039/D3QI01974H
(Research Article)
Inorg. Chem. Front., 2024,
11, 237-245
A cooperative effect of copper-induction and AIE leading to bright luminescence of gold nanoclusters†
Received
27th September 2023
, Accepted 11th November 2023
First published on 13th November 2023
Abstract
Emission enhancement of gold nanoclusters protected with 6-propyl-2-thiouracil (PRT-AuNCs) is achieved through incorporating a suitable amount of Cu2+. Sophisticated mechanisms are involved in the modulation of emission properties of the PRT-AuNCs/Cu2+ system, instead of merely a metal ion induced aggregation. X-ray photoelectron spectroscopy, IR spectroscopy and mass spectroscopy reveal that the Cu2+ is reduced by 6-propyl-2-thiouracil to form a Cu(I)–thiolate complex, which then co-assembles with the AuNCs to form a PRT-Au/Cu composite at an appropriate Cu2+ concentration. Systematic microscopic characterization studies including scanning electron microscopy, X-ray diffraction etc. demonstrate the crucial effect of compact aggregation driven by metallophilic interactions on the emission enhancement of PRT-Au/Cu. Detailed analysis of the photophysical parameters validates that the presence of Cu(I) changes the radiative transition mechanisms of PRT-Au/Cu with respect to primitive PRT-AuNCs. As a result, a cooperative effect of copper-induction and aggregation induced emission (AIE) leads to a strongly emissive composite with bathochromic-shifted emission energy. Moreover, the high molar ratio between Cu and Au in PRT-Au/Cu can effectively reduce the cost of the material in production, and we have developed a WLED to exemplify the potential application of this composite material.
1 Introduction
In pursuit of brightly luminescent materials for various application purposes, gold nanoclusters1 (AuNCs) are perceived as some of the most promising building blocks in multiple research areas including bio-imaging, chemical sensing, phototherapy, lighting etc. AuNCs belong to a class of organic–inorganic complex materials, whose luminescence properties are determined collectively by the metal core and the protecting ligands,2 mostly through a ligand to metal charge transfer (LMCT) mechanism.3 Many studies have been devoted to modulating the structure of the metal core,4 the molecular structure of ligands5 or the structure of Au(I)–ligand motifs on the surface of AuNCs,6 with the aim of rationally improving the emission properties of this class of nanomaterials. Another important factor that influences the emission properties of AuNCs is the aggregation or crystallization state of single cluster building blocks.7 In 2012, Xie's group8 discovered that the dense aggregation of oligomeric Au(I)–thiolate complexes led to a dramatic emission enhancement, and they developed a synthetic procedure to prepare highly emissive AuNCs based on the aggregation induced emission (AIE) of Au(I)–thiolate complexes condensed in the shell. This work enlightened an important strategy for designing AuNC-based luminophores that are bright enough for practical applications.9
Although AuNCs show attractive potential in terms of tunable luminescence properties, biocompatibility etc., their further development is constrained by economic considerations, i.e., the high cost of gold, especially for applications that require mass-production. To tackle this issue, incorporating low-cost metal elements e.g. silver or copper into AuNCs could be an effective solution. However, doping with heterometal atoms will change the electronic structure of the nanocluster system.10 Therefore, how to achieve an alloyed system with luminescence performance comparable or superior to that of the original nanoclusters becomes an important subject. Among the reported studies on alloy nanoclusters, the majority of studies are focused on constructing an atomically precise alloyed structure,11 while those reporting on the improvement of emission properties are still limited.12 Bakr's group13 studied the alloying of Ag25(SR)18 clusters by reacting with a PPh3AuCl complex, and they obtained increased PL intensity by doping a single Au heteroatom into the center of the silver kernel. They found that the emission of the alloyed clusters is highly dependent on the extent of Au doping. Wang's group14 also prepared Au/Ag alloyed nanoclusters via different procedures, and a highly emissive AgxAu25−x (x ≤ 13) cluster was obtained. They ascribed the emission enhancement to the stabilization of charges on the LUMO orbital by the central Ag atom. Yamamoto et al.15 enhanced the emission quantum yield (QY) of a platinum–thiolate complex by 18 times through inclusion of an Ag atom into the Pt core. They demonstrated that the rigid crystallization structure and the reduced nonradiative decay rate are the main reasons that contribute to the emission enhancement of the silver-doped nanoclusters. These prominent studies suggest that by properly manipulating the incorporation of another metal element,13,16 in combination with the emerging assembly behavior of the derived nanoclusters,15 the photoluminescence properties including QY and emission energy can be modulated effectively.
In our previous work, a type of gold nanocluster protected with 6-propyl-2-thiouracil (PRT-AuNCs) was synthesized and applied as a chromophoric building block to fabricate multicolor luminescent hydrogels.17 To further exploit the potential of PRT-AuNCs as bright luminophores for various applications, herein we report another strategy, i.e. induction with copper ions (Cu2+) to improve their luminescence intensity and tunability of emission energy (Scheme 1). Specifically, incorporation of Cu2+ into a PRT-AuNC solution improves their photoluminescence QY from 0.03% to 22.78%, accompanied by the prolongation of the luminescence lifetime from 5.72 μs to 15.59 μs. The intricate mechanism of emission enhancement is systematically demonstrated through structural and spectroscopic characterization. The reduction of Cu2+ in the presence of PRT produces Cu(I)–thiolate complexes which then co-assemble with primitive PRT-AuNCs to form compact aggregates (PRT-Au/Cu composite). The Au(I)⋯Cu(I) metallophilic interaction provides important driving force for aggregation and also facilitates the metal–metal charge transfer process which alters the radiative transition pathway of nanoclusters. The derived compact aggregation and the metal–metal bonding triplet state cooperatively generate strong bathochromic-shifted photoluminescence. Due to the doping with the copper element (molar ratio of Cu/Au ≈ 4), the obtained PRT-Au/Cu composite could be an economical material for production of white light-emitting diodes.
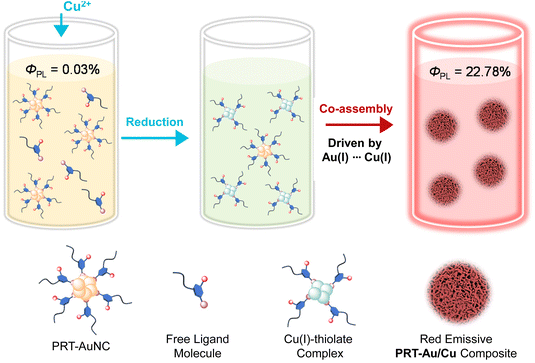 |
| Scheme 1 Schematic illustration for the formation of the strongly emissive PRT-Au/Cu composite, which involves the reduction of Cu2+ to generate Cu(I)–thiolate complexes and subsequent co-assembly to form micrometer-scale spherical aggregates facilitated by Au(I)⋯Cu(I) metallophilic interaction. | |
2 Materials and methods
2.1 Chemicals
All solvents and chemicals were of analytical grade and used as received from commercial suppliers. Chloroauric acid (HAuCl4·3H2O), NaOH and HNO3 were purchased from Sinopharm Chemical Reagent Co. 6-Propyl-2-thiouracil (PRT) and Cu(NO3)2 were purchased from Aladdin Biochemical Technology Co. (Shanghai, China). PDMS elastomer kits (Sylgard 184) were obtained from Merck Ltd. 395 nm emissive GaN chips were purchased from Shenzhen Looking Long Technology Co., Ltd.
2.2 Preparation of PRT-AuNCs and Cu(I)–thiolate complexes
6-Propyl-2-thiouracil (0.0341 g) was dissolved in 4 mL of deionized water in a glass vial, to which an aqueous solution of HAuCl4 (1 mL, 10 mg mL−1) was added. The mixture was stirred for 5 min and then kept standing for 24 h at 20 °C, which yielded a precipitate of PRT-AuNCs. Then NaOH solution (0.2 mL, 2 mol L−1) was added into the system, followed by vigorous stirring for 5 min to dissolve the PRT-AuNCs. Finally, the solution was diluted to 10 mL with deionized water, and the obtained AuNCs were stored at 20 °C prior to use. The ultimate molar concentrations of Au and PRT are 2.9 and 20 mmol L−1, respectively.
Cu(I)–thiolate complexes were prepared by directly mixing Cu(NO3)2 (c = 42.8 mM, 1 mL) with 6-propyl-2-thiouracil (c = 40 mM, 1 mL), followed by addition of NaOH to tune the pH of the solution to 7.5. The sample was stored at 20 °C for 24 h prior to freeze-drying and further SAXS measurement.
2.3 Methods and characterization
Photoluminescence spectral measurements were performed on an F-7000 spectrofluorometer (Hitachi, Japan). The quantum yield (QY) was tested on an FLS1000 fluorescence spectrometer (Edinburgh, England) equipped with an integrating sphere. The sample was diluted to decrease the absorbance (385 nm) to 0.1 (blank solvent: deionized water). Emission spectra of blank and nanoclusters were both scanned from 365 nm to 800 nm. Then quantum yield was calculated (scatter range: 365–405 nm, emission range: 550–800 nm). The photoluminescence lifetime was determined using an FLS 1000 fluorescence spectrometer employing a microsecond flashlamp as a light source. Phospherescence spectra were recored on an FLS1000 fluorescence spectrometer employing the gating method to filter out short-lifetime fluorescence. The delay time was set as 5 μs and the gate width was 20 μs. A microsecond flashlamp was employed as a light source. X-ray photoelectron spectroscopy (XPS) was carried out on an ESCALAB 250 X-ray photoelectron spectrometer with a monochromatized Al Kα X-ray source (1486.71 eV). Fourier transform infrared (FT-IR) spectra were measured on a Nicolet iS5 spectrometer from 4000 to 400 cm−1. Electrospray ionization mass spectrometry (ESI-MS) was performed on a Bruker MicrOTOF-QII mass spectrometer. ICP-MS measurement was performed on a PerkinElmer NexION 2000 inductively coupled plasma–mass spectrometer. Scanning electron microscopy (SEM) was carried out on a Sigma 500 (Zeiss, Germany). High resolution transmission electron microscopy (HR-TEM) was carried out on a JEOL JEM-2100PLUS. Small angle X-ray scattering was measured on a high flux small-angle X-ray scattering instrument (SAXSess, Anton Paar) equipped with a Kratky block-collimation system and a Philips PW3830 sealed-tube X-ray generator (Cu Kα). Confocal laser scanning microscopy (CLSM) images were obtained on a Zeiss LSM/880NLO microscope system. UV/Vis absorption spectra were recorded on a Hitachi UV-vis 4100 spectrophotometer (Hitachi, Japan) with a 0.1 cm path length quartz cuvette. The size distribution of the samples was measured on a Malvern Zetasizer Nano ZS system.
3 Results and discussion
3.1 Preparation of the emission enhanced PRT-Au/Cu composite
The PRT-AuNCs exhibit very weak photoluminescence in solution, which is a common case for most of the reported nanoclusters.18 A luminescence quantum yield (QY) of 0.03% was determined under ambient conditions. In our attempts to seek a method to improve the photoluminescence of PRT-AuNCs, we noticed that the addition of copper ions (Cu2+, in the form of Cu(NO3)2) could dramatically enhance the emission intensity of the system (Fig. 1a), depending on the concentration of added Cu2+ (c[Cu2+]). Based on the photoluminescence spectra, a plot of emission intensity (at λ = 664 nm) versus c[Cu2+] could be obtained (Fig. 1b), which indicates an abrupt increase when c[Cu2+] exceeds 8 mM. The strongest emission intensity is achieved at c[Cu2+] = 12 mM, while a sharp decline is observed in the higher concentration range (c[Cu2+] > 14 mM). The luminescence QY of the PRT-AuNCs/Cu2+ system with the strongest emission (c[Cu2+] = 12 mM) was determined to be 22.78%, which is enhanced by nearly 760 times in comparison with that of the primitive PRT-AuNCs. Moreover, the obtained PRT-AuNCs/Cu2+ system is quite stable over time and temperature. The emission intensity can be well retained even after 6 months of storage, and only shows a slight decrease when heated to 80 °C (Fig. S1†). It is worth noting that the visual appearance of the PRT-AuNC solution experienced a time-dependent drastic change induced by Cu2+ (Fig. 1c). A dark brown precipitate was observed immediately with the addition of Cu2+, after which the precipitate was re-dissolved gradually. Within ca. 90 minutes, a turbid yellow solution was obtained at equilibration. This phenomenon indicated the occurrence of a chemical reaction in the mixing system, instead of merely noncovalent-driven assembly. Thus, intricate mechanisms could be involved in the enhancement of photoluminescence.
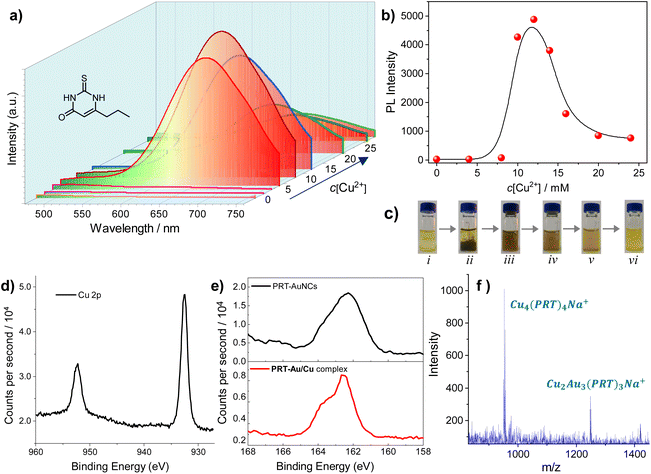 |
| Fig. 1 (a) Photoluminescence spectra of the PRT-AuNCs/Cu2+ system (λEx = 360 nm) with increasing c[Cu2+]; (b) plots of emission intensity (at λ = 664 nm) as a function of c[Cu2+], the curve is a guide to the eye; (c) photographs showing the dynamic change of visual appearance of the PRT-AuNCs/Cu2+ system after addition of 12 mM Cu2+. The photos are taken (i) before addition of Cu2+ and at (ii) 1 min, (iii) 10 min, (iv) 25 min, (v) 45 min, and (vi) 90 min after addition of Cu2+. XPS of (d) Cu 2p for the PRT-Au/Cu composite and (e) S 2p for PRT-AuNCs and the PRT-Au/Cu composite (c[Cu2+] = 12 mM); (f) positive ion mode ESI-MS of the PRT-Au/Cu composite (c[Cu2+] = 12 mM). | |
From XPS results of the PRT-AuNCs/Cu2+ system (Fig. 1d and Fig. S2†), two prominent peaks at 932.5 and 952.3 eV could be assigned to the binding energies of the 2p3/2 and 2p1/2 electrons of Cu(0). However, the 2p3/2 binding energy of Cu(0) is only ca. 0.1 eV away from that of Cu(I) species. Considering that charge transfer between Cu and 6-propyl-2-thiouracil is highly possible, the Cu atoms in the complex are expected to be positive. Thus, it can be deduced that the valence states of the Cu may involve 0 and +1. The absence of a satellite peak at 942.0 eV implies the nonexistence of Cu(II).19 Thus, the Cu2+ is reduced thoroughly by the dissociated 6-propyl-2-thiouracil in the PRT-AuNC system. In addition, the peak between 160 and 166 eV corresponding to S 2p exhibits an obvious change compared to that of the PRT-AuNCs (Fig. 1e), which can be interpreted as the occurrence of Cu(I)⋯S interaction. The nearly identical Au 4f spectra of PRT-AuNCs and PRT-AuNCs/Cu2+ (Fig. S2b†) indicate that the AuNCs remain stable during the reaction process. Moreover, in FTIR spectra (Fig. S3†), the PRT-AuNCs/Cu2+ exhibits an intense stretching vibrational absorption of the C–S bond at 1380 cm−1, which is largely enhanced in comparison with that of the PRT-AuNCs. This spectral change indicates the formation of Cu(I)–thiolate complexes, which is also confirmed by electrospray ionization mass spectrometry (ESI-MS). Two prominent peaks at 952.93 and 1246.88 m/z correspond to the composition of Cu4(PRT)4Na+ and Cu2Au3(PRT)3Na+, respectively (Fig. 1f). Therefore, the PRT-AuNCs/Cu2+ system can be deemed as a complex of AuNCs and CuNCs (denoted as the PRT-Au/Cu composite thereafter). The composition of the PRT-Au/Cu composite was also confirmed by ICP-MS, which indicated a molar ratio of 4.1
:
1 between Cu and Au.
3.2 Structural characterization of the PRT-Au/Cu composite
To understand the interactions occurring inside the PRT-Au/Cu composite, we first characterized the morphological evolution of PRT-AuNCs/Cu2+ mixed systems with increasing c[Cu2+] (Fig. 2). For the samples with c[Cu2+] ≤ 8 mM, no aggregates were observed under electron microscopy. When c[Cu2+] is increased to 10 mM, large size porous aggregates of irregular shape were observed under SEM (Fig. S4a†). At c[Cu2+] = 12 mM, the aggregates became spherical entities with an average diameter of ca. 5 μm, which is in agreement with the images observed in CLSM (Fig. 2a and d). Amplified SEM images showed that these porous spheres were densely fabricated by nanofibers with a width of ca. 20 nm (Fig. 2b and c), which is confirmed by TEM observation (Fig. 2e and Fig. S5†). At c[Cu2+] = 14 mM, the aggregates became an incompact network structure, while further increasing the c[Cu2+] to 16 mM gave a nanocrystalline morphology (Fig. S4b–d†). Therefore, the morphological observation indicates a clear trend that dense aggregation structures lead to stronger luminescence.
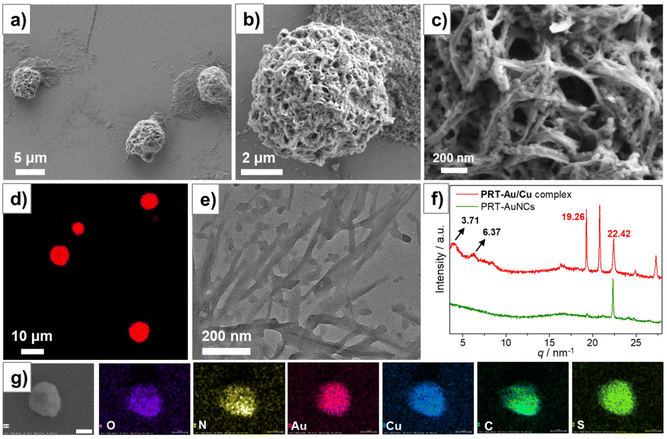 |
| Fig. 2 Morphological characterization of the PRT-Au/Cu composite (c[Cu2+] = 12 mM): (a–c) SEM images at different magnification; (d) CLSM image; (e) HR-TEM image showing the nanofibrous component of aggregates; (f) X-ray diffraction patterns of PRT-AuNCs and the PRT-Au/Cu composite; (g) energy dispersive spectroscopic (EDS) analysis of PRT-Au/Cu spherical aggregates obtained under SEM (scale bar is 2 μm). | |
To verify this conjecture, we modulated the pH of the PRT-AuNCs/Cu2+ system (at a fixed c[Cu2+] of 12 mM). Reducing the pH from 7.5 to 1.0 by the addition of HNO3 did not cause any obvious change of the emission spectra (Fig. S6a†). DLS measurements (Fig. S6b†) showed that the average size of the aggregates remains at ca. 5 μm. In contrast, increasing the pH from 7.5 to 10.0 by addition of NaOH caused obvious emission quenching. The emission band at 664 nm nearly vanished, accompanied by the appearance of an emission band at 508 nm (Fig. S6a†), which corresponded to the radiative transition of the Cu(I)-centered triplet state.20 The emission color of the sample also changed from red to green under irradiation of 365 nm UV light (insets of Fig. S6a†). At higher pH values (≥11.0), the system turned into a clear non-luminescent solution. Meanwhile, DLS results indicated the shrinking of the size of the aggregates with increasing pH. The disintegration of the aggregates is caused by the deprotonation of PRT ligands which increased the electrostatic repulsion between nanoclusters. Thus, it is validated that the aggregation of the nanoclusters has an important impact on the luminescence intensity of the system.
SEM elemental mapping analysis (Fig. 2g) on the spherical aggregates of the PRT-Au/Cu system demonstrated the presence of both Au and Cu as well as the constituent elements of the PRT ligand. Thus, the participation of both AuNCs and Cu(I)–thiolate complexes during the aggregation is confirmed. Small angle X-ray scattering (SAXS) was performed on the powders to investigate the internal structures of PRT-Au/Cu aggregates (Fig. 2f). For PRT-AuNCs, no diffraction was detected in the small angle region. Only a diffraction peak at q = 22.32 nm−1 (d = 0.28 nm) corresponding to the Au(I)⋯Au(I) interaction was observed.21 We also performed SAXS measurement on a freeze-dried sample of Cu(I)–thiolates, which was prepared by directly mixing Cu(NO3)2 with 6-propyl-2-thiouracil (PRT) followed by pH tuning (see section 2.2 for detailed procedures). The pattern of Cu(I)–thiolate complexes displays a prominent peak at 18.18 nm−1 in the wide angle region (Fig. S7†), which could be ascribed to the interlamellar distance of assembled Cu(I)–thiolates driven by Cu(I)–Cu(I) interaction.16 For PRT-Au/Cu, two diffraction peaks at q = 3.71 and 6.37 nm−1 with a ratio of 1
:
√3 could be detected, which correspond to a hexagonal columnar structure with a cell parameter of 1.69 nm. Thus, the assembly structure of nanoclusters is more ordered in the PRT-Au/Cu system. In the wide angle region, the peak for the Au(I)⋯Au(I) interaction (q = 22.32 nm−1) is still present, while the peak for the Cu(I)–Cu(I) interaction vanished. Moreover, the pattern of the PRT-Au/Cu composite displays new diffraction peaks at 19.26 and 20.82 nm−1, which are probably caused by new metallophilic interactions. The peak at q = 19.26 nm−1 corresponds to a distance of 0.33 nm, which is in agreement with the reported Au⋯Cu distance.22 These results confirm the occurrence of Au(I)⋯Cu(I) metallophilic interaction. Therefore, in addition to the AIE effect, charge transfer between Au(I) and Cu(I) could also contribute to the modulation of emission properties of PRT-Au/Cu.
3.3 Emission mechanism of the PRT-Au/Cu composite
To further understand the mechanism accounting for the emission enhancement of the PRT-Au/Cu system, we first measured the luminescence lifetimes of primitive PRT-AuNCs and the PRT-Au/Cu composite (Fig. 3a and Table 1). Bi-exponential fitting of the luminescence decay curves indicated that the PRT-Au/Cu composite has a much longer lifetime (15.59 μs) than primitive PRT-AuNCs (5.72 μs). In combination with their QY results, it can be determined that the emission enhancement is caused by the increase of radiative decay (kr) and decrease of nonradiative decay rate constants (knr) of PRT-Au/Cu compared with PRT-AuNCs (Table 1). A deeper inspection into the composition of the luminescence lifetimes shows that the fractions of short and long lifetime components are approximate for PRT-AuNCs, while for the PRT-Au/Cu composite, the short lifetime component almost diminishes and the long component dominates. This result implies the shift of the radiative transition pathways.6 The phosphorescence spectrum of PRT-Au/Cu was also recorded (Fig. S8†), which confirmed that the emission originated from a triplet state. In UV/Vis absorption spectra (Fig. 3c), the absorption bands of PRT-AuNCs at 260 nm and 305 nm exhibit a gradual decrease with addition of increasing amounts of Cu2+, accompanied by the appearance of a new absorption band at 360 nm. The c[Cu2+]-dependent UV/Vis spectra display a defined isosbestic point at 323 nm. These results indicate the distinct excitation transition between PRT-AuNCs and PRT-Au/Cu.
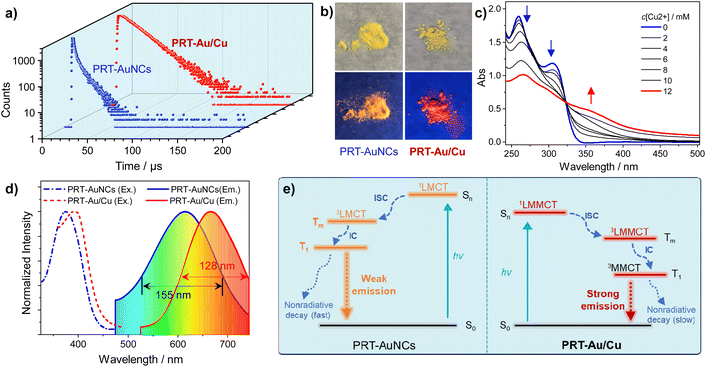 |
| Fig. 3 (a) Time-resolved luminescence decay of PRT-AuNCs and the PRT-Au/Cu composite (λEx = 360 nm); (b) visual appearance of powders under daylight (top) and their emission color under irradiation of UV light (bottom); (c) UV/Vis absorption spectra of the PRT-AuNCs/Cu2+ system with increasing c[Cu2+]; (d) excitation spectra (dashed curves) and emission spectra (filled curves) of powders for PRT-AuNCs and the PRT-Au/Cu composite; the full width at half maximum (FWHM) of the emission bands is indicated with double-headed arrows; (e) schematic diagram showing the photoluminescence mechanisms of PRT-AuNCs and the PRT-Au/Cu composite (IC: internal conversion, ISC: intersystem crossing). | |
Table 1 Photophysical parameters of PRT-AuNCs and the PRT-Au/Cu composite
Species |
λ
Em
/nm |
τ
1
/μs |
τ
2
/μs |
τ c/μs |
Φ d/% |
k
r
/s−1 |
k
nr
/s−1 |
Maximum emission wavelength.
Components of bi-exponential luminescence lifetimes and pre-exponential factors (in parentheses).
Averaged luminescence lifetimes measured at an excitation wavelength of λEx = 365 nm.
Photoluminescence quantum yields.
Radiative decay rate constant: kr = Φ/τ.
Nonradiative decay rate constant: knr = kr × (1/Φ − 1).
|
PRT-AuNCs (in solution) |
620 |
1.56 (0.46) |
9.26 (0.54) |
5.72 |
0.03 |
5.24 × 101 |
1.75 × 105 |
PRT-Au/Cu (in solution) |
664 |
0.14 (0.0015) |
15.62 (0.9985) |
15.59 |
22.78 |
1.46 × 104 |
4.95 × 104 |
The powders also exhibit different emission colors under irradiation of UV light (Fig. 3b). According to the emission spectra of powders, the emission maxima of PRT-AuNCs and PRT-Au/Cu are located at 615 and 666 nm, respectively (Fig. 3d), which should be attributed to the radiative transition from different excited states. The emission energy of PRT-AuNC powder is typical for the relaxation of the Au(I)-centered state resulting from LMCT. In contrast, lower energy emission of PRT-Au/Cu in combination with its wide emission band implies that the luminescence can be ascribed to a metal–metal bonding triplet state.23 Based on the above analysis, a mechanistic diagram can be proposed as in Fig. 3e. For PRT-AuNCs, the emission is attributed to a LMCT triplet state, which stems from the photoexcitation and the subsequent singlet–triplet spin-forbidden transition. In solution, the relaxation of the 3LMCT state occurs mostly through nonradiative decay, due to the unrestricted thermal motion of protecting ligands on dispersed AuNCs.24 Whereas in powder, the PRT-AuNCs exhibit yellow emission (615 nm) due to aggregation. For PRT-Au/Cu, the photoexcitation process absorbs lower energy photons to be excited to the 1LMMCT state, followed by intersystem crossing to a 3LMMCT state. Facilitated by the strong metallophilic interactions, a 3MMCT state is generated through internal conversion,25 which then emits strong red emission (666 nm) because the nonradiative decay is effectively suppressed in compact aggregates. Moreover, the narrower emission spectrum of PRT-Au/Cu implies a more rigid/ordered aggregation packing structure, in agreement with the SEM and XRD results.
3.4 Application of the PRT-Au/Cu composite in light-emitting diodes
Through doping copper, a bright red light-emitting composite PRT-Au/Cu with much lower cost than the primitive AuNCs can be obtained. Moreover, PRT-Au/Cu retains the strong emission when the solvent is evaporated (vide infra, Fig. 3). Thus, this composite is suitable for fabricating white light-emitting diodes (WLED) as a red phosphor. To obtain a WLED, we employed commercially available BaMgAl10O17:Eu2+ and (Ba,Sr)2SiO4:Eu2+ as a blue phosphor (BP) and a green phosphor (GP), respectively, to mix with the red light-emitting PRT-Au/Cu composite. The mixed powder was then suspended in PDMS and coated on a 395 nm GaN UV chip (Fig. 4b), followed by curing at 80 °C for 2 h. Electroluminescence (EL) spectra of the prepared LED at a mass ratio of BP
:
GP
:
PRT-Au/Cu = 1
:
1.5
:
2 are shown in Fig. 4a. Three main emission bands located at 468 nm, 525 nm and 699 nm are distinguishable, which correspond to the luminescence of the three mixed phosphors. The LED emits cool while light (Fig. 4c and e) corresponding to a CIE coordination of (0.2936, 0.3586), with a color rendering index (CRI) of 81.9 and a color temperature of 7433 K. Moreover, the EL spectra of the prepared WLED show continuous enhancement as a function of increasing drive current (I) from 20 mA to 140 mA (Fig. S6†). The CIE coordination is independent of the drive current, and the CRI value remains above 80 (Fig. 4d and Table S2†). These results, in combination with its facile and environmentally friendly preparation method, indicate that the PRT-Au/Cu composite can be a promising candidate as a red phosphor for lighting applications.
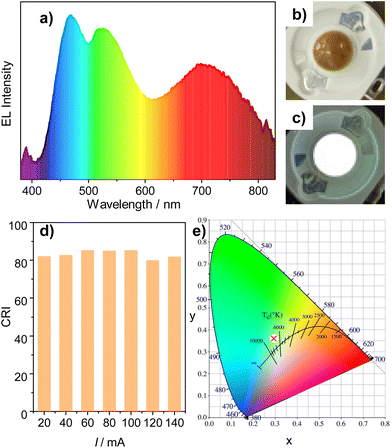 |
| Fig. 4 Performance of the WLED fabricated with the PRT-Au/Cu composite: (a) electroluminescence spectra at I = 140 mA; (b and c) photographs of the prepared LED device by coating the phosphors on a 395 nm UV chip; (d) variation of the color rendering index as a function of drive current; (e) CIE chromaticity diagram of the LED operated at I = 140 mA. | |
4 Conclusion
In summary, a strongly emissive nanocluster assembly was prepared by simply incorporating Cu2+ into an aqueous system of PRT-AuNCs. Instead of merely inducing aggregation through coordination interaction, Cu2+ was actually reduced to form Cu(I)–thiolate complexes. Driven by the strong metallophilic interactions between Au(I) and Cu(I), compact aggregates co-assembled by AuNCs and Cu(I)–thiolate complexes (PRT-Au/Cu composite) were obtained, which are micrometer-scale spherical entities fabricated by nanofibers. The composition of the PRT-Au/Cu composite was validated by multiple spectroscopic measurements. Microscopic characterization revealed the occurrence of an ordered molecular arrangement inside the aggregates, which is advantageous for the emission enhancement through restricting the molecular motion of PRT ligands. As a consequence of compact aggregation, the PRT-Au/Cu composite exhibited intense red emission with a QY of 22.78% and a long lifetime of 15.59 μs. Moreover, the alteration of the charge transfer process as well as the radiative transition pathways facilitated by Au(I)⋯Cu(I) metallophilic interactions led to the bathochromic shift of the emission energy, which was also rationalized by spectroscopic analysis. Therefore, the enhanced emission properties of the PRT-Au/Cu composite are contributed cooperatively by copper-induction and the consequent compact aggregation. Employing the bright red light emissive PRT-Au/Cu, an economical WLED with potential for commercial application was fabricated. This work provides an effective strategy for developing versatile nanocluster-based luminescent materials through simply incorporating the copper element into AuNCs.
Conflicts of interest
The authors declare no conflicts of interest.
Acknowledgements
We gratefully acknowledge the financial support from the National Natural Science Foundation of China (22002069 & 22302078) and the Natural Science Foundation of Shandong Province, China (ZR2020QB062 & ZR2022QB145).
References
-
(a) H. Z. Yu, B. Rao, W. Jiang, S. Yang and M. Z. Zhu, The Photoluminescent Metal Nanoclusters with Atomic Precision, Coord. Chem. Rev., 2019, 378, 595–617 CrossRef CAS;
(b) J. Yang, Y. Peng, S. Li, J. Mu, Z. Huang, J. Ma, Z. Shi and Q. Jia, Metal Nanocluster-Based Hybrid Nanomaterials: Fabrication and Application, Coord. Chem. Rev., 2022, 456, 214391 CrossRef CAS;
(c) F. N. Wu, J. Zhu, G. J. Weng, J. J. Li and J. W. Zhao, Gold Nanocluster Composites: Preparation Strategies, Optical and Catalytic Properties, and Applications, J. Mater. Chem. C, 2022, 10, 14812–14833 RSC;
(d) X. Kang, H. Chong and M. Zhu, Au25(SR)18: the Captain of the Great Nanocluster Ship, Nanoscale, 2018, 10, 10758–10834 RSC.
-
(a) C. M. Aikens, Electronic and Geometric Structure, Optical Properties, and Excited State Behavior in Atomically Precise Thiolate-Stabilized Noble Metal Nanoclusters, Acc. Chem. Res., 2018, 51, 3065–3073 CrossRef CAS PubMed;
(b) J. Kong, W. Zhang, Y. Wu and M. Zhou, Optical Properties of Gold Nanoclusters Constructed from Au13 Units, Aggregate, 2022, 3, e207 CrossRef CAS;
(c) B. Zhang, J. Chen, Y. Cao, O. J. H. Chai and J. Xie, Ligand Design in Ligand-Protected Gold Nanoclusters, Small, 2021, 17, 2004381 CrossRef CAS PubMed.
- X. Kang and M. Zhu, Tailoring the Photoluminescence of Atomically Precise Nanoclusters, Chem. Soc. Rev., 2019, 48, 2422–2457 RSC.
-
(a) R. Jin, C. Zeng, M. Zhou and Y. Chen, Atomically Precise Colloidal Metal Nanoclusters and Nanoparticles: Fundamentals and Opportunities, Chem. Rev., 2016, 116, 10346–10413 CrossRef CAS PubMed;
(b) Y. Yu, Z. Luo, D. M. Chevrier, D. T. Leong, P. Zhang, D. E. Jiang and J. Xie, Identification of a Highly Luminescent Au22(SG)18 Nanocluster, J. Am. Chem. Soc., 2014, 136, 1246–1249 CrossRef CAS PubMed.
-
(a) X. Kang, S. Wang, Y. Song, S. Jin, G. Sun, H. Yu and M. Zhu, Bimetallic Au2 Cu6 Nanoclusters: Strong Luminescence Induced by the Aggregation of Copper(I) Complexes with Gold(0) Species, Angew. Chem., Int. Ed., 2016, 55, 3611–3614 CrossRef CAS PubMed;
(b) Y. Lin, P. Charchar, A. J. Christofferson, M. R. Thomas, N. Todorova, M. M. Mazo, Q. Chen, J. Doutch, R. Richardson, I. Yarovsky and M. M. Stevens, Surface Dynamics and Ligand-Core Interactions of Quantum Sized Photoluminescent Gold Nanoclusters, J. Am. Chem. Soc., 2018, 140, 18217–18226 CrossRef CAS PubMed.
- Z. Wu, Q. Yao, O. J. H. Chai, N. Ding, W. Xu, S. Zang and J. Xie, Unraveling the Impact of Gold(I)-Thiolate Motifs on the Aggregation-Induced Emission of Gold Nanoclusters, Angew. Chem., Int. Ed., 2020, 59, 9934–9939 CrossRef CAS PubMed.
-
(a) M. M. Zhang, K. Li and S. Q. Zang, Progress in Atomically Precise Coinage Metal Clusters with Aggregation–Induced Emission and Circularly Polarized Luminescence, Adv. Opt. Mater., 2020, 8, 1902152 CrossRef CAS;
(b) L. Shi, L. Zhu, J. Guo, L. Zhang, Y. Shi, Y. Zhang, K. Hou, Y. Zheng, Y. Zhu, J. Lv, S. Liu and Z. Tang, Self-Assembly of Chiral Gold Clusters into Crystalline Nanocubes of Exceptional Optical Activity, Angew. Chem., Int. Ed., 2017, 56, 15397–15401 CrossRef CAS PubMed.
- Z. Luo, X. Yuan, Y. Yu, Q. Zhang, D. T. Leong, J. Y. Lee and J. Xie, From Aggregation-Induced Emission of Au(I)-thiolate Complexes
to Ultrabright Au(0)@Au(I)-thiolate Core-shell Nanoclusters, J. Am. Chem. Soc., 2012, 134, 16662–16670 CrossRef CAS PubMed.
- T. Li, H. Zhu and Z. Wu, Viewing Aggregation-Induced Emission of Metal Nanoclusters from Design Strategies to Applications, Nanomaterials, 2023, 13, 470 CrossRef CAS PubMed.
- T. Chen, S. Yang, J. Chai, Y. Song, J. Fan, B. Rao, H. Sheng, H. Yu and M. Zhu, Crystallization-Induced Emission Enhancement: A Novel Fluorescent Au-Ag Bimetallic Nanocluster with Precise Atomic Structure, Sci. Adv., 2017, 3, e1700956 CrossRef PubMed.
-
(a) S. Hossain, Y. Niihori, L. V. Nair, B. Kumar, W. Kurashige and Y. Negishi, Alloy Clusters: Precise Synthesis and Mixing Effects, Acc. Chem. Res., 2018, 51, 3114–3124 CrossRef CAS PubMed;
(b) L. Fang, W. Fan, G. Bian, R. Wang, Q. You, W. Gu, N. Xia, L. Liao, J. Li, H. Deng, N. Yan and Z. Wu, Sandwich-Kernelled AgCu Nanoclusters with Golden Ratio Geometry and Promising Photothermal Efficiency, Angew. Chem., Int. Ed., 2023, 62, e202305604 CrossRef CAS PubMed.
-
(a) M. Jash, A. Jana, A. K. Poonia, E. Khatun, P. Chakraborty, A. Nagar, T. Ahuja, K. V. Adarsh and T. Pradeep, Phosphine-Protected Atomically Precise Silver–Gold Alloy Nanoclusters and Their Luminescent Superstructures, Chem. Mater., 2023, 35, 313–326 CrossRef CAS;
(b) X. Kang, Y. Li, M. Zhu and R. Jin, Atomically Precise Alloy Nanoclusters: Syntheses, Structures, and Properties, Chem. Soc. Rev., 2020, 49, 6443–6514 RSC.
- M. S. Bootharaju, C. P. Joshi, M. R. Parida, O. F. Mohammed and O. M. Bakr, Templated Atom-Precise Galvanic Synthesis and Structure Elucidation of a [Ag24Au(SR)18](-) Nanocluster, Angew. Chem., Int. Ed., 2016, 55, 922–926 CrossRef CAS PubMed.
- J. H. Jia and Q. M. Wang, Intensely Luminescent Gold(I)-Silver(I) Cluster with Hypercoordinated Carbon, J. Am. Chem. Soc., 2009, 131, 16634–16635 CrossRef CAS PubMed.
- Y. Akanuma, T. Imaoka, H. Sato and K. Yamamoto, Silver in the Center Enhances Room-Temperature Phosphorescence of a Platinum Sub-nanocluster by 18 Times, Angew. Chem., Int. Ed., 2021, 60, 4551–4554 CrossRef CAS PubMed.
- J. Liu, Z. Wu, Y. Tian, Y. Li, L. Ai, T. Li, H. Zou, Y. Liu, X. Zhang, H. Zhang and B. Yang, Engineering the Self-Assembly Induced Emission of Cu Nanoclusters by Au(I) Doping, ACS Appl. Mater. Interfaces, 2017, 9, 24899–24907 CrossRef CAS PubMed.
- Y. Yu, X. H. Zhang, M. G. Wang, J. Y. Luan, X. M. Liu, J. L. Shen and W. Qi, Au Nanocluster-Based Smart Multicolor Luminescent Hydrogels for Encryption Applications, ACS Appl. Nano Mater., 2022, 5, 10047–10054 CrossRef CAS.
- J. Wang, X. Lin, T. Shu, L. Su, F. Liang and X. Zhang, Self-Assembly of Metal Nanoclusters for Aggregation-Induced Emission, Int. J. Mol. Sci., 2019, 20, 1891 CrossRef CAS PubMed.
-
(a) Z. Wang, Y. Xiong, S. V. Kershaw, B. Chen, X. Yang, N. Goswami, W.-F. Lai, J. Xie and A. L. Rogach, In Situ Fabrication of Flexible, Thermally Stable, Large-Area, Strongly Luminescent Copper Nanocluster/Polymer Composite Films, Chem. Mater., 2017, 29, 10206–10211 CrossRef CAS;
(b) L. Kong, X. Chu, C. Wang, H. Zhou, Y. Wu and W. Liu, D-Penicillamine-Coated Cu/Ag Alloy Nanocluster Superstructures: Aggregation-Induced Emission and Tunable Photoluminescence from Red to Orange, Nanoscale, 2018, 10, 1631–1640 RSC;
(c) W. Wei, Y. Lu, W. Chen and S. Chen, One-Pot Synthesis, Photoluminescence, and Electrocatalytic Properties of Subnanometer-Sized Copper Clusters, J. Am. Chem. Soc., 2011, 13, 2060–2063 CrossRef PubMed.
-
(a) Z. Wu, H. Liu, T. Li, J. Liu, J. Yin, O. F. Mohammed, O. M. Bakr, Y. Liu, B. Yang and H. Zhang, Contribution of Metal Defects in the Assembly Induced Emission of Cu Nanoclusters, J. Am. Chem. Soc., 2017, 139, 4318–4321 CrossRef CAS PubMed;
(b) P. P. Sun, B. L. Han, H. G. Li, C. K. Zhang, X. Xin, J. M. Dou, Z. Y. Gao and D. Sun, Real-Time Fluorescent Monitoring of Kinetically Controlled Supramolecular Self-Assembly of Atom-Precise Cu8 Nanocluster, Angew. Chem., Int. Ed., 2022, 61, e202200180 CrossRef CAS PubMed;
(c) Y. J. Kong, Z. P. Yan, S. Li, H. F. Su, K. Li, Y. X. Zheng and S. Q. Zang, Photoresponsive Propeller-like Chiral AIE Copper(I) Clusters, Angew. Chem., Int. Ed., 2020, 59, 5336–5340 CrossRef CAS PubMed;
(d) Z. Wu, J. Liu, Y. Gao, H. Liu, T. Li, H. Zou, Z. Wang, K. Zhang, Y. Wang, H. Zhang and B. Yang, Assembly-Induced Enhancement of Cu Nanoclusters Luminescence with Mechanochromic Property, J. Am. Chem. Soc., 2015, 137, 12906–12913 CrossRef CAS PubMed.
- Z. Wu, Y. Du, J. Liu, Q. Yao, T. Chen, Y. Cao, H. Zhang and J. Xie, Aurophilic Interactions in the Self-Assembly of Gold Nanoclusters into Nanoribbons with Enhanced Luminescence, Angew. Chem., Int. Ed., 2019, 58, 8139–8144 CrossRef CAS PubMed.
- Y. J. Wang, X. Y. Shi, P. Xing and S. Q. Zang, Metallophilic Interactions Drive Supramolecular Chirality Evolution and Amplify Circularly Polarized Luminescence, JACS Au, 2023, 3, 565–574 CrossRef CAS PubMed.
- J. Zheng, Z. Lu, K. Wu, G. H. Ning and D. Li, Coinage-Metal-Based Cyclic Trinuclear Complexes with Metal-Metal Interactions: Theories to Experiments and Structures to Functions, Chem. Rev., 2020, 120, 9675–9742 CrossRef CAS PubMed.
-
(a) N. Goswami, F. Lin, Y. Liu, D. T. Leong and J. Xie, Highly Luminescent Thiolated Gold Nanoclusters Impregnated in Nanogel, Chem. Mater., 2016, 28, 4009–4016 CrossRef CAS;
(b) N. Goswami, Q. Yao, Z. Luo, J. Li, T. Chen and J. Xie, Luminescent Metal Nanoclusters with Aggregation-Induced Emission, J. Phys. Chem. Lett., 2016, 7, 962–975 CrossRef CAS PubMed.
-
(a) R. Galassi, M. M. Ghimire, B. M. Otten, S. Ricci, R. N. McDougald Jr., R. M. Almotawa, D. Alhmoud, J. F. Ivy, A. M. Rawashdeh, V. N. Nesterov, E. W. Reinheimer, L. M. Daniels, A. Burini and M. A. Omary, Cupriphication of Gold to Sensitize d10−d10 Metal–Metal Bonds and Near-Unity Phosphorescence Quantum Yields, Proc. Natl. Acad. Sci. U. S. A., 2017, 114, E5042–E5051 CrossRef CAS PubMed;
(b) W. X. Ni, Y. M. Qiu, M. Li, J. Zheng, R. W. Sun, S. Z. Zhan, S. W. Ng and D. Li, Metallophilicity-Driven Dynamic Aggregation of a Phosphorescent Gold(I)-Silver(I) Cluster Prepared by Solution-Based and Mechanochemical Approaches, J. Am. Chem. Soc., 2014, 136, 9532–9535 CrossRef CAS PubMed.
Footnotes |
† Electronic supplementary information (ESI) available: X-ray photoelectron spectra, FT-IR spectra, morphological characterization, pH-dependent photoluminescence spectra, dynamic light scattering, and electroluminescence spectra of the WLED. See DOI: https://doi.org/10.1039/d3qi01974h |
‡ These authors contributed equally to this work. |
|
This journal is © the Partner Organisations 2024 |