DOI:
10.1039/D3QI01828H
(Research Article)
Inorg. Chem. Front., 2024,
11, 215-223
14-electron reduced MoIV6-ε-Keggin polyoxometalates: highly stable and reversible electron/Li+ sponge materials†
Received
10th September 2023
, Accepted 7th November 2023
First published on 7th November 2023
Abstract
First, 14-electron reduced MoIV6-ε-Keggin polyoxometalates (POMs), namely14e-[Sb2MoIV6MoV2MoVI4O32(OH)2py6]2− (1), 14e-[(GeO)2MoIV6MoV2MoVI4O34(OH)2py6]4− (2), and 14e-[Sb2ZnMoIV6MoV2MoVI4O34py7] (3) (py = pyridine), were prepared. X-ray structural analyses of 1–3 revealed two triangularly metal–metal Δ-bonded 6e-[MoIV3O4] incomplete cubane-type units and one 2e-[MoV2O4], which account for the hollow ε-Keggin structures free of central heteroatoms. Long-range π-stacking interactions and super electron-rich Keggin structures of 14e-1 and 14e-2 lead to their 260 times higher electrical conductivity than conventional fully oxidized MoVI-Keggin POMs. The hollow MoIV6-ε-Keggin POM 1 exhibited structural integrity retained during 24-electron charging/discharging processes when being simultaneously monitored by ex situ XPS and IR. A dynamic study of Li-ion migration in 1 revealed its dominant capacitor-like Li+-storage mechanism and effective/fast absorption/desorption of Li+. Therefore, it exhibited a high discharge specific capacity (303 mA h g−1, 50 mA g−1), superior rate and cycling performance, especially at an extremely high current density (121.6 mA h g−1, 100 cycles, 8.0 A g−1), which remarkably enhances the performance of conventional fully oxidized MoVI-Keggin cathode materials, and provides a new option for using super electron-rich, hollow POMs to improve the electrochemical performance of POM electrode materials.
Introduction
The global rush to search for advanced battery materials has led to increased interest in the study of polyoxometalates (POMs) as electrode materials for lithium batteries because of their diverse structures and potentially unique ability to accept/release multiple electrons while retaining structural integrity.1–3 Recently, high-capacity tailored POMs and their derivative electrode materials have been reported.4–8 Of the most essential but challenging issues are the number of redox electrons, structural integrity associated with the multielectron redox processes and electrical conductivity.9–11 The former two are highly dependent on the number of incomplete cuboidal [Mo3(μ3-O)(μ2-O)3] functional units of POMs11,12 since they are able to accept six electrons to form well-established incomplete cubane-type [Mo3(μ3-O)(μ2-O)3] units stabilized by strong triangular d2–d2 metal–metal Δ-bonds.11–15 Hence, the incomplete cuboidal [Mo3O4] units can serve as reversible 6e-redox functional groups via Mo–Mo Δ-bond breakage and re-formation while maintaining their structural integrity. Conventional Keggin-type species [X@M12O40]q− (M = Mo, W) unexceptionally embedded with central atoms X, the earliest discovered and most extensively studied POMs,16 are of special importance in the search for advanced molecular cluster batteries since they are composed of four incomplete cuboidal [M3(μ3-O)(μ2-O)3] units. [PMoVI12O40]3− has been reported as a cathode material for lithium-ion batteries, which exhibits a specific capacity of 260 mA h g−1 at a current density of 1 mA.14 The high specific capacity was ascribed to the reversible [MoVI3O4]4 ↔ 24e-[MoIV3O4]4 interconversion according to in operando Mo K-edge X-ray absorption fine structure measurements.14 However, the [PMoVI12O40]3− cathode material revealed remarkable capacity degradation after 10 cycles.14 Similarly remarkable capacity degradation has also been observed in other Keggin-POM cathode materials.14,17–24 Subsequent first-principles molecular dynamics (FPMD) simulations showed that the highly symmetric 24e-reduced Keggin structure would dissociate when its symmetric constraints were removed, suggesting configurational change.11 One of the important factors leading to this structural instability of the super-reduced [PMoIV12O40]27− has been revealed by recent findings that both 6e- and 12e-reduced MoIV3,6-Keggin POMs12,25–29 are unexceptionally free of a central heteroatom because of the absence of additional coordinating ability of the μ3-O atoms caused by the much weaker trans-MoIV-pyridine dative bonds and hence creation of stronger μ3-O–MoIV3 bonding. However, strong additional μ3-O bonding ability associated with weak MoVI3–μ3-O bonds is important in order to stabilize central heteroatomic groups such as {PO4}. Hence, one effective route to improve and enhance battery performance of POMs is to use empty MoIV3n-Keggin species to maintain structural integrity during multielectron redox processes. Moreover, deeply reduced MoIV3n-POMs with markedly smaller HOMO–LUMO gaps and a large number of metal–metal bonding electrons can effectively increase electrical conductivity compared to conventional fully oxidized POMs.30 However, the synthetic and structural chemistry of MoIV3-POMs has been a long-term uncharted research area, which has led to very limited availability of structural information about the multielectron-reduced states of POM electrode materials. In 2013 and 2018, synthetic strategies for the partial oxidation of [MoIV3O4(Hnta)3]2− (ref. 12) and the [MIV3O2(O2CCH3)6(H2O)3]2+ precursor15 (Hnta = nitrilotriacetic acid) were developed, leading to the rapid development of MoIV3n-POM (n = 1–4) chemistry (Fig. S1†).12,15,25–29,31,32 Deeply reduced MoIV3n-POMs12,14,15,25–29,31,32 featuring strong triangular metal–metal Δ-bonds are of special importance in respect of (i) a varied combination of 6e-MoIV3 subunits can dramatically change the structures of POMs to form novel types of MoIV3n-POMs;12,15,25–29,31,32 (ii) each [MoIV3O4] can act as a reversible 6e-redox functional unit via Δ-bond breakage and re-formation while maintaining structural integrity; (iii) inert terminal MoV,VI
O double bonds are replaced by active MoIV ←: L dative bonds (L = Lewis base ligand), which functionalize POMs with MoIV3 Lewis acidic active sites to form MoIV3⋯OxMy subnano Lewis catalysis fields (LCFs) with extensive Lewis acid–base synergistic effects;25–29,32 (iv) a varied number of Δ-bonded MoIV3 triangles remarkably change electronic structures and decrease HOMO–LUMO gaps to different extents to tune the photoelectric properties and enhance the electrical conductivity of POMs; (v) a large number of surface MoIV3n bonding electrons can act as an “electron sponge/reservoir”14,33 through the breakage and re-formation of Δ-bonds; (vi) peripheral modifiable pyridine ligands allow for the functionalization of MoIV3-POMs via crystal engineering/ligand substitution/π⋯π interactions for varied purposes; (vii) physicochemical properties such as conductivity can be reinforced by efficient MoIV3 → OxMVIy electron transfer and the incorporation of heterometal addenda atoms; and (xiii) X-ray single-crystal structural characterization of mixed-valence MoIV,V,VI-POMs is critical for understanding the MoIV ↔ MoV ↔ MoVI structural evolution during charging/discharging processes of POM electrode materials.11,14,34–36 POM materials have shown excellent electrochemical performance as anode materials and have been widely reported.3,37,38 However, the use of POMs as cathode materials has been less reported and their performance is generally poor14,17,18,20,21,24,39 (Table S8, ESI†) due to the poor electrical conductivity and structural reversibility of POMs in super-electron redox processes at higher voltage ranges (1.5–4.0 V, vs. Li/Li+). In this work, compared to conventional fully oxidized POMs, three empty electron-rich [MoIV3O4]2-incorporated Keggin POMs, namely [Sb2MoIV6MoV2MoVI4O32(OH)2py6]2− (1), [(GeO)2MoIV6MoV2MoVI4O34(OH)2py6]4− (2) and [Sb2ZnMoIV6MoV2MoVI4O32(OH)2py7] (3), exhibit remarkably enhanced cycling life and battery performance, even at an extremely high current density, as cathode materials for lithium batteries.
Results and discussion
Synthesis and structure
The preparation of deeply reduced MoIV-POMs has been a long-term challenging issue because (i) discrete MoIV is unstable and easily oxidized to MoV,VI and (ii) in contrast to WV in POMs,40–43 MoV has a strong tendency to form metal–metal-bonded MoV2 dimer and never disproportionates to MoIV and MoVI. In 2013, the first MoIV-POM [MoIV6MoVI7O36py6]4− (9, Fig. S1†) was prepared.12 In 2018, inspired by the established [MoIV3(μ3-O)2] → [MoIV3O4] skeletal conversion,34–36 the oxidation-controllable bioxo-capped precursor [MoIV3O2(O2CCH3)6(H2O)3]2+ was utilized15 to synthesize a series of MoIV3n-POMs (4–28, Fig. S1†), leading to the rapid development of MoIV-POM chemistry.12,15,25–29,31,32 As shown in Fig. 1, the empty MoIV6-Keggin anions 1(2) were prepared from solvothermal oxidative aggregation of a mixture of [MoIV3O2(O2CCH3)6(H2O)3]ZnCl4 and Sb2O3(GeO2) in py/DMF/H2O/2-pyCO2H at different temperatures for 3 days. The dianionic 1 and tetraanionic 2 were charge balanced by 2{HNH(CH3)2}+ and 2{Hpy}+·2{HNH(CH3)2}+, respectively, wherein dimethylamine commonly came from the solvothermal decomposition of N,N-dimethylformamide (DMF). The electroneutral 3 resulting from the additional electrophilic addition of {Znpy}2+ was obtained from a similar preparative procedure to that of 1 but at an enhanced solvothermal temperature. POMs 1–3 have been characterized by IR (Fig. S11†), TGA (Fig. S7†) and XPS (Fig. S9†). As-prepared and dried samples of 1 show identical IR and XPS spectra (Fig. S11a and S9a–b†), indicative of their retention of structural integrity. The purity of 1–3 has been verified by PXRD (Fig. S8†) and elemental analyses (Experimental procedures, ESI†).
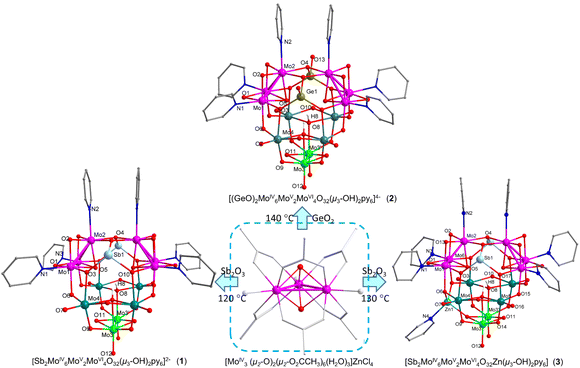 |
| Fig. 1 Solvothermal syntheses and atomically labelled structures of the deeply reduced hollow MoIV6-Keggin POMs 1–3 with hydrogen atoms omitted for clarity. Selected average bond lengths (Å) in 1: MoIV–MoIV (MoV–MoV/MoV⋯MoVI/MoVI⋯MoVI), 2.5073(7) ((2.596/3.352)/3.309); MoIV–N (MoV O/MoVI O), 2.233 (1.689(6)/1.699(4)); MoIV–μ3-O (MoV,VI–μ3-O), 2.036(2.260); MoIV–μ2-O–MoIV (MoIV–μ2-O–MoVI/MoIV–μ3-O–SbIII), 1.939/1.935/2.128(3). SbIII–O, 1.965. Colour code: MoIV, pink; MoV, light green; MoVI, teal; Sb, pale blue; Ge, olive; Zn, turquoise; O, red; N, blue; and C, gray. | |
As shown in Fig. 1, 1 features a Keggin structure with crystallographic C2v-symmetry. The electrophilic addition of two SbIII atoms occurred as commonly encountered for the deeply reduced MoIV3-Keggin adducts 8 and 9
32 (Fig. S1†). Such addition has not been observed for the 6e- or 12e-reduced WVI-incorporated MoIV3-Keggin species 4–7 because of the WVI-induced lower nucleophilicity.32 The existence of two 6e-reduced [MoIV3O4] units has been confirmed by the XPS spectrum (Fig. S9†) and the strong triangular MoIV–MoIV bonds (av. 2.5047(8) Å) (Fig. 1). The markedly shorter MoIV3–μ3-O5 bonds (av. 2.030(4) Å) induced by the weak trans MoIV–N (2.225(3) Å) compared to MoV,VI–μ3-O8 ones (av. 2.256(3) Å) lead to the absence of additional coordinating ability of the former and hence the central atoms. The two much weaker MoVI3–μ3-O bonds resulting from the strong trans MoVI
O double bonds are expectedly stabilized by the bonded protons. As shown in Fig. 1 and Fig. S1,† differing from 6e-α-Keggin 4, 12e-β-Keggin 9 and 12-e-γ-Keggin 5–8, 14e-1 with its unusual further 2e-reduction at Mo(V)3–Mo(V)3′ confirmed by XPS (Fig. S9a†) and its short bond length of 2.5896(8) Å, has an ε-Keggin conformation induced by the Mo(V)3–Mo(V)3′ metal–metal bond (Table S2†). As displayed in Fig. 1, 2 has a similar ε-Keggin structure with two {GeO}2+ adducts instead of SbIII. Differing from ε-Keggin 1 and 2, 3 bears one more electrophilic addition of {Znpy}2+ at three μ2-O of the two bottom MoVMoVI2 triads, responsible for its electroneutrality. Fig. S3† shows edge-to-face π-stacking interactions between coordinating and lattice py rings in 1 and 2. They constitute 1D π⋯π interacted chains with dihedral angles/centroid distance/slipped distance of 74°/4.8/0.94 Å, in contrast to the face-to-face π⋯π interactions (7.5°/3.6 Å) between the two almost parallel coordinating py rings. Differing from 1 and 2, 3 has no lattice py molecules induced by electroneutrality and lacks effective intermolecular π⋯π stacking interactions.
Electronic structures and bonding
As shown in Fig. 2, first-principles density functional theory (DFT) calculations for the deeply reduced Keggin structures 1–3 and the conventional fully oxidized Keggin structures [PMoVI12O40]3− and [SiWVI12O40]4− have been carried out to understand their differences including energy levels and bonding evolution induced by increasing numbers of reduced electrons and heterometals. Detailed DFT calculations are provided in the ESI section.† The HOMO of 1/2 is dominated by d1(MoV)–d1(MoV) and MoV–μ2O bonding orbitals in the MoV2O4 dimer, while that of 3 is mainly contributed by d2(MoIV)–d2(MoIV) and MoIV–μ2,3O bonding interactions in the [MoIV3O4] incomplete cuboidal units caused by the electrophilic addition of {Znpy}2+. The next six frontier MOs (HOMO−1 to HOMO−6) of 1–3 are dominated by MoIV–MoIV and MoIV–μ2,3-O bonding in the two [MoIV3O4] units (Fig. S25–S27†). They have markedly higher energy levels than the fully oxidized MoVI/WVI-Keggin HOMO dominated by μ2-O 2p orbitals (Fig. 2, Table S9 and Fig. S24†) and hence smaller HOMO–LUMO gaps, which, together with the 14 metal–metal bonding electrons, should be beneficial for their electrical conductivity as described later. This has been supported by UV-vis spectrophotometric measurements of 1/2/3 (Fig. S12†) that exhibit much smaller optical band gaps of 1.67/1.72/1.59 eV at 800 nm than those of conventional fully oxidized POMs such as H3[P@WVI12O40], H6[P2WVI18O62] and H3[P@MoVI12O40] (about 2.50 eV)44,45 according to the Tauc plot function. When 14e-1 undergoes a further 10e reduction to produce 24e-1 (S10-1, ESI†), the higher energy level of the HOMO featuring d2–d2 bonding interactions in the [MoIV3O4] units leads to a decreased HOMO–LUMO gap of 1.12 eV (Fig. S22†).
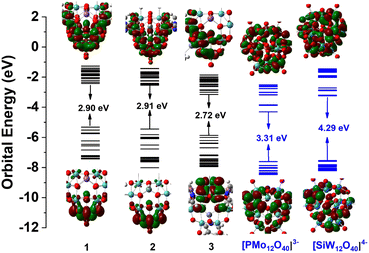 |
| Fig. 2 A comparison of energy levels and HOMO–LUMO gaps of empty MoIV3-POMs 1–3, and fully oxidized Keggin POMs [PMoVI12O40]3− and [SiWVI12O40]4−. | |
Electrical conductivity
Electrical conductivity of electrode materials has a considerable impact on electrochemical performance.46 It largely depends on extended interactions,47,48 HOMO–LUMO gaps and availability of delocalized electrons.30 As detailed above, long-range π-stacking interactions,47,48 narrower HOMO–LUMO gap and metal–metal bonding electrons30 can effectively improve electrical conductivity. Conventional fully oxidized POMs were known to suffer from poor electrical conductivity.46,49–51 As shown in Table S7† and Fig. 3, the electrical conductivity of 14e-1 (4.61 × 10−9 S cm−1) is more than 260 times that of fully oxidized TBA3[P@Mo12O40] (TBA = n-Bu4N+),51 which is largely responsible for the markedly enhanced battery performance described below. 14e-2 with almost the same long-range π⋯π interactions has a similar conductivity (2.72 × 10−9 S cm−1) but 14e-3 lacking long-range π⋯π interactions exhibits significantly smaller conductivity (2.91 × 10−10 S cm−1).
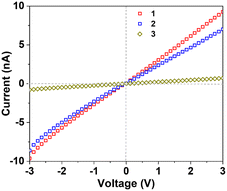 |
| Fig. 3
I–V characteristics of MoIV6-POM 1 (red), MoIV6-POM 2 (blue) and MoIV6-POM 3 (olive) at 30 °C, respectively. | |
Electrochemical properties
The reversible multielectron redox and Li+/electron storage behavior of MoIV6-Keggin cathode materials 1–3 for lithium batteries was investigated. 1 exhibits reversible redox behavior over the voltage range of 1.5–4.0 V (vs. Li/Li+). The cyclic voltammetry (CV) curves for the first five and 10th cycles of the lithium half-cell are illustrated in Fig. 4a. In the first CV curve sweep, a weak cathodic peak at 2.08 V and four anodic peaks at 2.22, 2.97, 3.22 and 3.58 V (vs. Li/Li+) appeared. The irreversible weak cathodic peak at 2.08 V and anodic peak at 3.58 V disappeared in the following cycles, which may be ascribed to the irreversible intercalation and deintercalation of Li+, respectively. In the second sweep, it also showed four cathodic peaks, centered at 2.93, 2.74, 2.18 and 1.76 V (vs. Li/Li+), and four corresponding anodic peaks at 3.22, 2.97, 2.22 and 1.89 V, respectively. The former three pairs of redox peaks are similar to those of [PMoVI12O40]3− (2.85/3.2, 2.68/2.86 and 2.32/2.42 V, vs. Li/Li+). These redox peaks are associated with the reversible MoIV ↔ MoVI conversion according to the ex situ XPS results and related work reported previously.11,14,52 The main anodic peak at 2.97/3.22 V and main cathodic peak at 2.74/2.93 V were obviously enhanced over 5 cycles, in accordance with the increasing charging/discharging specific capacity over several cycles (Fig. 4b). The intensified redox peaks should be associated with the deintercalation/intercalation behavior of Li+ and the “activating” process in the first few cycles.52–54 In those cycles with the crystal → amorphous phase conversion, the deintercalation/intercalation behavior of Li+ is replaced by the absorption/desorption effects.52–55 The CV of the 5th sweep nicely overlaps with those of the following sweeps, indicative of highly reversible redox behavior and the strong structural integrity during the charge–discharge process. MoIV6-POMs 2 and 3 also exhibit similar reversible redox behaviors (Fig. S13†). Additionally, the small polarization between the reduction and oxidation processes forebodes excellent rate performance of the cathode material.
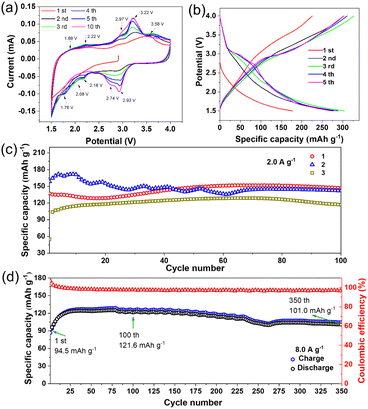 |
| Fig. 4 (a) CV curves of MoIV6-POM 1 at a scan rate of 0.5 mV s−1 and (b) galvanostatic charge/discharge voltage profiles of the 1st to 5th cycles. Cycle performance with coulombic efficiency of MoIV6-POMs 1–3 (c, 2.0 A g−1) and MoIV6-POM 1 (d, 8.0 A g−1) over 100 and 350 cycles, respectively. | |
The galvanostatic charge/discharge profiles of 1 are shown in Fig. 4b. Two plateau-like potential regions, 3.2–2.75 and 1.76–1.5 V (vs. Li/Li+), were observed during the second discharging process, which is in good agreement with the CV results. The voltage gradually decreases from the initial open potential of about 2.89 V to 1.5 V (vs. Li/Li+) and exhibits a discharge capacity of 177 mA h g−1. It presents a high discharge specific capacity of 303 mA h g−1 in the 3rd cycle at a current density of 50 mA g−1 (30 °C). It presents much better rate performance over the voltage range of 1.5–4.0 V (Fig. S14†) compared to not only conventional pure POM electrode materials but also POMs functionalized with nanocarbon materials such as rGO@PANI/PW12 (285 mA h g−1),23 PANI/PMo12 (210 mA h g−1)21 and SiW12/rGO (275 mA h g−1)22 (Table S8†). As shown in Fig. 4c, MoIV6-ε-Keggin cathode materials 1–3 each exhibit a high reversible discharge capacity of about 147, 142 and 117 mA h g−1 after 100 cycles at a high current density of 2.0 A g−1, respectively. In particular, 1 exhibits an amazing long-term cycling performance with reversible capacity of 122 and 101 mA h g−1 after 100 and 350 cycles, respectively, even at a high current density of 8.0 A g−1 (Fig. 3d). Notably, the capacity retention rate after 350 cycles achieved an impressive value of 106.9% compared to the first cycle. 2 and 3 exhibit similar cycling performances at extremely high current density (8.0 A g−1), which present high discharge specific capacity values of 94 and 86 mA h g−1 after 100 cycles (Fig. S15 and S16†), respectively. The better performance of the super electron-rich ε-Keggin POM 1 should be attributed to its higher electrical conductivity and structural stability. As indicated in Table S8,† the deeply reduced empty MoIV6-Keggin cathode materials 1–3 exhibit significantly higher discharge specific capacity and longer cycling life than traditional fully oxidized Keggin POMs and even POMs functionalized with nanocarbon materials (Table S8†). The remarkably enhanced cycling performance, especially at extremely high current density (2 and 8 A g−1), exhibited by deeply reduced empty MoIV6-ε-Keggin POMs compared to conventional fully oxidized POMs is closely related to their unique structural integrity retained during the course of charging/discharging processes, which will be detailed below.
The poor electrochemical performance of fully oxidized POMs might be attributable to the unstable deeply reduced structures when discharged to 1.5 V. Both 6e- and 12e-reduced MoIV3,6-Keggin POMs are unexceptionally free of central heteroatoms (As, Ge, P, Si etc.)12,15,25,26,28,29 because of the absence of additional coordinating ability of the μ3-O atoms caused by the much weaker trans-MoIV-pyridine dative bonds (2.23 Å) and hence the stronger μ3-O–MoIV3 (2.04 Å) bonding, as detailed in Fig. 5. However, the strong additional μ3-O bonding ability associated with the weak MoVI3–μ3-O bonds (2.45 Å) is essential to stabilize central heteroatomic groups such as {PO4} (P
O, 1.52 Å) for fully oxidized Keggin POMs (Fig. 5a). In addition, hollow MoIV3n-Keggin structures with almost intact terminal MoIV,V,VI ← py bonds (and hence similar trans-MoIV,V,VI–μ3-O bonds) benefit in retaining structural stability during super-electron redox processes. Terminal MoIV,V,VI ← py dative bonds were observed to coexist in single MoIV,V,VI-POMs with similar Mo–N distances (2.18–2.32 Å),32 suggesting that the Mo ← py groups of 1 almost remain intact during the charging/discharging process and hence contribute to the structural stability in contrast to fully oxidized Keggin POMs with their remarkable structural change from doubly bonded MoV,VI
O (1.68 Å, Fig. 5) to dative bonded MoIV ← O2− (2.14 Å)14 (and hence markedly different trans-MoIV,V,VI–μ3-O bonds, MoV,VI–μ3-O, 2.45 Å → MoIV–μ3-O, 2.04 Å) during super-electron redox processes. Therefore, the much reduced structural change and the absence of endohedral heteroatoms in the deeply reduced hollow MoIV6-Keggin POMs lead to advantageous electrochemical performance compared to fully oxidized Keggin POMs and even those functionalized by nanocarbon materials (Table S8†) especially at an extremely high current density.
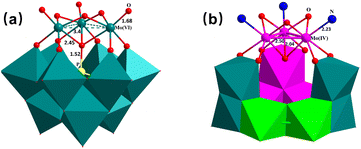 |
| Fig. 5 A structural comparison (Å) of the fully oxidized [MoVI3O4] unit in the conventional endohedral α-Keggin [PMoVI12O40]3− (a) and the 6e-reduced [MoIV3O4] unit in super-reduced hollow ε-Keggin 1 (b, Sb, C and H atoms are omitted for clarity). | |
{MoIV3}4 ⇌ {MoVI3}4 24e-redox monitored by ex situ IR/XPS
The reversible MoIV3 ⇌ MoVI3 6e-redox is characteristic of the interconversion between the terminal MoIV ←: O2− dative bond and the MoVI
O double bonds11,14 and hence can be monitored by both IR and XPS since the MoV/VI
O bond displays a characteristic stretching vibration between 939 and 955 cm−1. Fig. 6 shows the ex situ XPS/IR diagrams measured in the course of discharging → charging → discharging processes at different voltages (Fig. S10 and Table S6†). As-prepared 1 shows a strong MoVI/V
O (939 cm−1) IR band and MoIV/MoV/MoVI (3
:
1
:
2) XPS peaks. When discharged to 1.5 V, all MoVI atoms were reduced to MoIV as demonstrated by the appearance of MoIV XPS peaks only, which is in accordance with the disappearance of the MoVI/V
O peaks at about 946 cm−1. The MoVI/V
O IR bands re-appeared when charged to 3.0 V concomitant with the appearance of the MoVI/MoV/MoIV XPS peaks. Once 1 was further charged to 4.0 V, all MoIV/V atoms were oxidized to MoVI as confirmed by the presence of MoVI XPS peaks only and stronger MoVI
O peaks. The XPS peaks of MoIV and MoV reappeared when discharged to 3.0 V with strong MoVI/V
O IR bands. The re-discharge to 1.5 V led to the disappearance of the MoVI/V
O peaks at 946 cm−1 concomitant with the observation of MoIV-XPS peaks only. The ex situ IR/XPS results and the highly stable cycling performance of MoIV6-Keggin POMs reveal that a highly reversible 24-electron redox reaction occurs during the charging–discharging process (1.5–4.0 V).
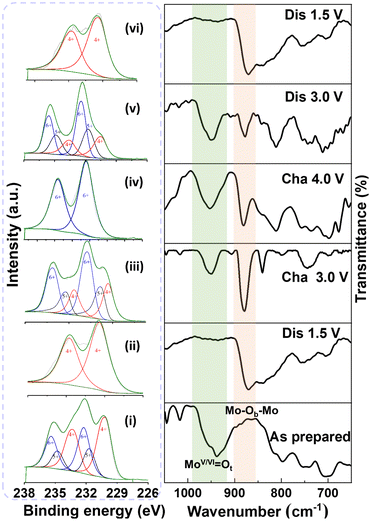 |
| Fig. 6
Ex situ XPS/FT-IR spectra of the cathode materials 1 at different cutoff voltages. | |
Li-ion migration dynamics
The MoIV6-POMs bear six MoIV ←: NC5H5 groups, which, in addition to taking part in long-range π-stacking interactions, also lead to the larger void spaces favoring Li+ migration. The total potential solvent-accessible void volume of MoIV6-Keggin POMs 1–3 was calculated to be 1001.0, 924.6 and 57.2 Å3 (12.1%, 11.0% and 0.9% of the respective unit cell volume) using PLATON.56 This has been supported by the fact that the consistent Li+ diffusion coefficient (DLi) of 1 (10−12 to 10−10 cm2 s−1, Fig. 7a), which is 10–100 times greater than that of classical intercalation compounds LiFePO4/LiCoO2 (10−14 to 10−11 cm2 s−1).57,58 Rate-sweep CV provides deeper insights into the charge storage kinetics on the basis of the following equation:59
where i is the current density (A), a and b are the adjustable parameters, and v is the scan rate (V s−1). The b value is the slope of the plot of log
i vs. log
v. The b values for redox peaks A, B, C and D are 0.74, 0.88, 0.84 and 0.79 for MoIV6-POM 1, respectively (Fig. 5b and c), and log(i) − log(v) also exhibits similar linear relationships for MoIV6-POM 2/3, which suggest that the charge storage of MoIV3-POMs is affected by both capacitor-like and diffusion-controlled behaviors:59
where k1v and k2v1/2 are the current response contributions of surface capacitive effects and diffusion-controlled behaviours, respectively. The contribution of the capacitive fraction is 66.3% at a sweep rate of 2 mV s−1 and increases to 81.7% at 10 mV s−1 for 1 (Fig. 7d and Fig. S17†). MoIV3-POMs 2 and 3 exhibit similar behaviors (Fig. S19 and S21†), indicating that capacitor-like charge storage dominates for MoIV3-POM electrode materials. The charge storage behavior dominated by capacitive effects, fast Li+ diffusion and unique structural stability is believed to account for their excellent cycling life and rate performance at high current density. A comparison of the super electron-rich empty MoIV3-ε-Keggin cathode materials 1–3 and fully oxidized MVI-Keggin species is given in Table S8.† The highly enhanced discharging specific capacity, superior rate capacity and cycling performance of the former can be ascribed to the significantly enhanced electrical conductivity induced by the super electron-rich empty ε-Keggin structure and π⋯π stacking interactions, void spaces induced by coordinating and lattice py groups, and the good structural integrity retained during the super redox process.
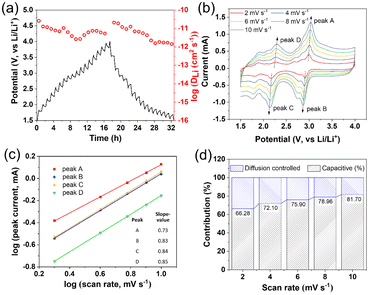 |
| Fig. 7 (a) GITT curve of MoIV6-POM 1. (b) Rate-scan CV curves of MoIV6-POM 1 at various sweep rates. (c) Peak current Ip as a function of the square root of the scan rate v0.5. (d) Normalized contribution ratio of capacitive effects and diffusion-controlled behaviors at different scan rates (2–10 mV s−1) of MoIV6-POM 1. | |
Conclusions
The deeply reduced 14e-MoIV6-Keggin POMs 1–3 prepared exhibit expected hollow Keggin structures as a consequence of the extremely weak additional coordination ability of capping oxygen atoms bonded to the strongly metal–metal-bonded MoIV3 triangles. The ex situ XPS/IR monitoring and highly enhanced cycling performance during the charging/discharging process (1.5–4.0 V) of 1–3 indicate that the deeply reduced hollow ε-Keggin structures have proven essential to maintain the structural integrity in the course of the super 24e-redox process. This, together with the markedly enhanced electrical conductivity resulting from long-range π⋯π stacking interactions, smaller HOMO–LUMO gaps, a super electron-rich structure, and fast Li+ migration kinetics, accounts for their high discharging specific capacity and superior rate/cycling performance especially at high current density. This work reveals the reasons for the poor performance of conventional fully oxidized POM materials as electrode materials and the super electron-rich Keggin POMs providing a new way of thinking to improve POM-based electrode materials.
Author contributions
The experiments and theoretical calculations were mainly performed by JZ, MC, FY and CMR. LX experimentally and theoretically guided and financially supported this work. The work was written by JZ and LX.
Conflicts of interest
There are no conflicts to declare.
Acknowledgements
This work was supported by the National Natural Science Foundation of China (21873102).
References
- Q. Y. Li, L. Zhang, J. L. Dai, H. Tang, Q. Li, H. G. Xue and H. Pang, Polyoxometalate-based materials for advanced electrochemical energy conversion and storage, Chem. Eng. J., 2018, 351, 441–461 CrossRef CAS.
- N. I. Gumerova and A. Rompel, Synthesis, structures and applications of electron-rich polyoxometalates, Nat. Rev. Chem., 2018, 2, 0112 CrossRef CAS.
- J. K. Wang, L. Wang, Y. Yang, H. Xu and X. M. He, Polyoxometalates (POMs) with Ion/Electron–Sponge Properties and Abundant Active Sites as Emerging Electrode Materials for Secondary Batteries: A Review, Batteries Supercaps, 2023, 6, e202200510 CrossRef CAS.
- C. L. Wu, J. L. Hu, J. Tian, F. L. Chu, Z. G. Yao, Y. J. Zheng, D. G. Yin and C.-L. Li, Stacking of Tailored Chalcogenide Nanosheets around MoO2-C Conductive Stakes Modulated by a Hybrid POM⊂MOF Precursor Template: Composite Conversion–Insertion Cathodes for Rechargeable Mg–Li Dual-Salt Batteries, ACS Appl. Mater. Interfaces, 2019, 11, 5966–5977 CrossRef CAS.
- C.-L. Wu, J. L. Hu, Z. G. Yao, D. G. Yin and C.-L. Li, Highly Reversible Conversion Anodes Composed of Ultralarge Monolithic Grains with Seamless Intragranular Binder and Wiring Network, ACS Appl. Mater. Interfaces, 2019, 11, 23280–23290 CrossRef CAS.
- P. Y. Wang, J. Tian, J. L. Hu, X. J. Zhou and C. L. Li, Supernormal Conversion Anode Consisting of High-Density MoS2 Bubbles Wrapped in Thin Carbon Network by Self-Sulfuration of Polyoxometalate Complex, ACS Nano, 2017, 11, 7390–7400 CrossRef CAS PubMed.
- J. J. Xie, Y. Zhang, Y. L. Han and C.-L. Li, High-Capacity Molecular Scale Conversion Anode Enabled by Hybridizing Cluster-Type Framework of High Loading with Amino-Functionalized Graphene, ACS Nano, 2016, 10, 5304–5313 CrossRef CAS PubMed.
- J. W. Meng, M. Lei, C. Z. Lai, Q. P. Wu, Y. Y. Liu and C.-L. Li, Lithium Ion Repulsion-Enrichment Synergism Induced by Core–Shell Ionic Complexes to Enable High-Loading Lithium Metal Batteries, Angew. Chem., Int. Ed., 2021, 60, 23256–23266 CrossRef CAS PubMed.
- Y. C. Ji, L. J. Huang, J. Hu, C. Streb and Y.-F. Song, Polyoxometalate-functionalized nanocarbon materials for energy conversion, energy storage and sensor systems, Energy Environ. Sci., 2015, 8, 776–789 RSC.
- H. Taketa, S. Katsuki, K. Eguchi, T. Seiyama and N. Yamazoe, Electronic structure and redox mechanism of dodecamolybdophosphate, J. Phys. Chem. C, 1986, 90, 2959–2962 CrossRef CAS.
- Y. Nishimoto, D. Yokogawa, H. Yoshikawa, K. Awaga and S. Irle, Super-Reduced Polyoxometalates: Excellent Molecular Cluster Battery Components and Semipermeable Molecular Capacitors, J. Am. Chem. Soc., 2014, 136, 9042–9052 CrossRef CAS PubMed.
- W. P. Chen, R. L. Sang, Y. Wang and L. Xu, An unprecedented [Mo3IVO4]-incorporated polyoxometalate concomitant with MoO2 nucleophilic addition, Chem. Commun., 2013, 49, 5883–5885 RSC.
- A. Bino, F. A. Cotton and Z. Dori, A trinuclear molybdenum(IV) cluster compound having an unusual structure and unusual stability, J. Am. Chem. Soc., 1978, 100, 5252–5253 CrossRef CAS.
- H. Wang, S. Hamanaka, Y. Nishimoto, S. Irle, T. Yokoyama, H. Yoshikawa and K. Awaga, In Operand X-ray Absorption Fine Structure Studies of Polyoxometalate Molecular Cluster Batteries: Polyoxometalates as Electron Sponges, J. Am. Chem. Soc., 2012, 134, 4918–4924 CrossRef CAS PubMed.
- X. Xu, B. L. Luo, L. L. Wang and L. Xu, An unprecedented nanocage-like and heterometallic [Mo3IVO4]-polyoxomolybdate hybrid, Dalton Trans., 2018, 47, 3218–3222 RSC.
- J. F. Keggin, Structure of the Molecule of 12-Phosphotungstic Acid, Nature, 1933, 131, 908–909 CrossRef CAS.
- K. Kume, N. Kawasaki, H. Wang, T. Yamada, H. Yoshikawa and K. Awaga, Enhanced capacitor effects in polyoxometalate/graphene nanohybrid materials: a synergetic approach to high performance energy storage, J. Mater. Chem. A, 2014, 2, 3801–3807 RSC.
- N. Sonoyama, Y. Suganuma, T. Kume and Z. Quan, Lithium intercalation reaction into the Keggin type polyoxomolybdates, J. Power Sources, 2011, 196, 6822–6827 CrossRef CAS.
- H. Wang, N. Kawasaki, T. Yokoyama, H. Yoshikawa and K. Awaga, Molecular cluster batteries of nano-hybrid materials between Keggin POMs and SWNTs, Dalton Trans., 2012, 41, 9863–9866 RSC.
- N. Kawasaki, H. Wang, R. Nakanishi, S. Hamanaka, R. Kitaura, H. Shinohara, T. Yokoyama, H. Yoshikawa and K. Awaga, Nanohybridization of Polyoxometalate Clusters and Single-Wall Carbon Nanotubes: Applications in Molecular Cluster Batteries, Angew. Chem., Int. Ed., 2011, 50, 3471–3474 CrossRef CAS PubMed.
- H. X. Yang, T. Song, L. Liu, A. Devadoss, F. Xia, H. Han, H. Park, W. Sigmund, K. Kwon and U. Paik, Polyaniline/Polyoxometalate Hybrid Nanofibers as Cathode for Lithium Ion Batteries with Improved Lithium Storage Capacity, J. Chem. Phys., 2013, 117, 17376–17381 CAS.
- S. Wang, H. L. Li, S. Li, F. Liu, D. Q. Wu, X. L. Feng and L. X. Wu, Electrochemical-reduction-assisted assembly of a polyoxometalate/graphene nanocomposite and its enhanced lithium-storage performance, Chem. – Eur. J., 2013, 19, 10895–10902 CrossRef CAS PubMed.
- L. B. Ni, G. Yang, C. Y. Sun, G. S. Niu, Z. Wu, C. Chen, X. X. Gong, C. Q. Zhou, G. J. Zhao, J. Gu, W. Ji, X. Huo, M. Chen and G. W. Diao, Self-assembled three-dimensional graphene/polyaniline/polyoxometalate hybrid as cathode for improved rechargeable lithium ion batteries, Mater. Today Energy, 2017, 6, 53–64 CrossRef.
- H. Omachi, T. Inoue, S. Hatao, H. Shinohara, A. Criado, H. Yoshikawa, Z. Syrgiannis and M. Prato, Concise, Single-Step Synthesis of Sulfur-Enriched Graphene: Immobilization of Molecular Clusters and Battery Applications, Angew. Chem., Int. Ed., 2020, 59, 7836–7841 CrossRef CAS PubMed.
- B. L. Luo and L. Xu, POM-FLPs: [Mo3IV]n-polyoxometalate bifunctional catalysis by [Mo3IV]n-Om Lewis pairs frustrated by triangular MoIV-MoIV bonds, Dalton Trans., 2019, 48, 6892–6898 RSC.
- C. M. Ren, Z. H. Lu, B. L. Luo, X. F. Yi, L. F. Lin and L. Xu, Deeply reduced empty Keggin clusters [MoxIVM12-xVIO40−xpyx] (x = 3, 6; M = Mo, W; py = pyridine): synthesis, structures, and Lewis field catalysis, Inorg. Chem. Front., 2021, 8, 5178–5185 RSC.
- F. Yu, B. L. Luo, R. L. Sang and L. Xu, Mo3IV⋯[O8Mo4]3 Lewis acid-base cluster pairs: Highly efficient and stable Lewis catalysis fields frustrated in crystalline nanoclusters, Nanoscale, 2020, 12, 20230–20238 RSC.
- B. L. Luo, R. L. Sang, L. F. Lin and L. Xu, Mo3IV-Polyoxomolybdates with frustrated Lewis pairs for high-performance hydrogenation catalysis, Catal. Sci. Technol., 2019, 9, 65–69 RSC.
- F. Yu and L. Xu, Highly efficient Mo3IV⋯SbIII cluster frustrated Lewis pair hydrogenation, Dalton Trans., 2019, 48, 17445–17450 RSC.
- M. J. Turo, L. F. Chen, C. E. Moore and A. Schimpf, Co2+-linked [NaP5W30O110]14−: A redox-active metal oxide framework with high electron density, J. Am. Chem. Soc., 2019, 141, 4553–4557 CrossRef CAS.
- L. F. Lin, L. L. Wang and L. Xu, Extended M3IV-Polyoxometalates: 1D-[Mo6IVMo4VIGe2ZnO31py9 (H2O)]4− and 2D-[Mo3IVMo9VIZn6P7O56py8]+ (py = Pyridine), Inorg. Chem., 2022, 61, 8558–8569 CrossRef CAS PubMed.
- C. Cheng, X. F. Yi, W. P. Chen, R. L. Sang and L. Xu, Electron-rich Mo3IV-Polyoxomolybdates Resembling the Paratungstic Archetype, Chem. – Eur. J., 2023, e202300043 CrossRef CAS.
- P. Gouzerh and M. Che, From Scheele and Berzelius to Muller - Polyoxometalates (POMs) revisited and the “missing link” between the bottom up and top down approaches, Actual. Chim., 2006, 9–22 CAS.
- L. Xu, Z. H. Li, H. Liu, J. S. Huang and Q. E. Zhang, Syntheses, crystal structures, and properties of novel heterooctametallic clusters Na2M2′[M3O4(O2CEt)8]2 (M’=Fe, Cr, Mo; M3=Mo3, MoW2, W3), Chem. – Eur. J., 1997, 3, 226–231 CrossRef CAS PubMed.
- L. Xu, Z. F. Li, H. Liu and J. S. Huang, Cluster conversion of [MoW2O2(O2CEt)9]− to [MoW2O4(O2CEt)8]4− by chromium hexacarbonyl crystal structures of Na[MoW2O2(O2CMe)9.H2O, [MoW2O2(O2CEt)6(H2O)3. ZnCl4.2H2O, and Na2Cr2 [MoW2O4(O2CEt)8]2, J. Coord. Chem., 1996, 40, 133–144 CrossRef CAS.
- L. Xu, L. Huang, D. C. Yan, J. S. Huang and Q. N. Zhang, Sybthesis, mechanism of formation and characterization of a new class of octanuclear mixed metal clusters Na2M’2[M3O4(O2CET)8]2 (M’=Cr or V, M=Mo or W), J. Chem. Soc., Dalton Trans., 1994, 2099–2107 RSC.
- H. Ilbeygi, I. Y. Kim, M. G. Kim, W. Cha, P. S. M. Kumar, D. H. Park and A. Vinu, Highly Crystalline Mesoporous Phosphotungstic Acid: A High-Performance Electrode Material for Energy-Storage Applications, Angew. Chem., Int. Ed. Engl., 2019, 58, 10849–10854 CrossRef CAS PubMed.
- D. Ma, L. Liang, W. Chen, H. Liu and Y.-F. Song, Covalently Tethered Polyoxometalate-Pyrene Hybrids for Noncovalent Sidewall Functionalization of Single-Walled Carbon Nanotubes as High-Performance Anode Material, Adv. Funct. Mater., 2013, 23, 6100–6105 CrossRef CAS.
- J. L. Liu, Z. Chen, S. Chen, B. W. Zhang, J. Wang, H. H. Wang, B. B. Tian, M. H. Chen, X. F. Fan, Y. Z. Huang, T. C. Sum, J. Y. Lin and Z. X. Shen, “Electron/Ion Sponge”-Like V-Based Polyoxometalate: Toward High-Performance Cathode for Rechargeable Sodium Ion Batteries, ACS Nano, 2017, 11, 6911–6920 CrossRef CAS PubMed.
- Y. Jeannin, J. P. Launay and M. A. S. Sedjadi, Crystal and Molecular Structure of the Six-electron-reduced Form of Metatungstate Rb4H8[H2W12O40]·∼18H2O: Occurrence of a Metal-Metal Bonded Subcluster in a Heteropolyanion Framework., Inorg. Chem., 1980, 19, 2933–2935 CrossRef CAS.
- K. Piepgrass and M. T. Pope, Heteropoly "Browns" as Class I Mixed Valence (W(IV,VI)) Complexes. Tungsten-183NMR of W(IV) Trimers, J. Am. Chem. Soc., 1987, 109, 1586–1587 CrossRef CAS.
- C. Boskovic, M. Sadek, R. T. C. Brownlee, A. M. Bond and A. G. Wedd, Electrosynthesis and solution structure of six-electron reduced forms of metatungstate, [H2W12O40]6−, J. Chem. Soc., Dalton Trans., 2001, 187–196 RSC.
- M. H. Dickman, T. Ozeki, H. T. Evans, C. Y. Rong, G. B. Jameson and M. T. Pope, Polyoxometalates from heteropoly “brown” precursors. A new structural class of mixed valence heteropolytungstates, [(XO4)W3IVW17VIO62Hx]n−, J. Chem. Soc., Dalton Trans., 2000, 149–154 RSC.
- H. F. Shi, G. Yan, Y. Zhang, H. Q. Tan, W. Z. Zhou, Y. Y. Ma, Y. G. Li, W. Chen and E. B. Wang, Ag/AgxH3-xPMo12O40 Nanowires with Enhanced Visible-Light-Driven Photocatalytic Performance, ACS Appl. Mater. Interfaces, 2017, 9, 422–430 CrossRef CAS.
- X. R. Gao, J. F. Wang, Q. H. Xue, Y.-Y. Ma and Y. Z. Gao, AgBr/Polyoxometalate/Graphene Oxide Ternary Composites for Visible Light-Driven Photocatalytic Hydrogen Production, ACS Appl. Nano Mater., 2021, 4, 2126–2135 CrossRef CAS.
- J. M. Tarascon and M. Armand, Issues and challenges facing rechargeable lithium batteries, Nature, 2001, 414, 359–367 CrossRef CAS PubMed.
- A. Facchetti, M.-H. Yoon, C. L. Stern, H. E. Katz and T. J. Marks, Building Blocks for n-Type Organic Electronics: Regiochemically Modulated Inversion of Majority Carrier Sign in Perfluoroarene-Modified Polythiophene Semiconductors, Angew. Chem., 2003, 115, 4030–4033 CrossRef.
- M.-H. Yoon, A. Facchetti, C. E. Stern and T. J. Marks, Fluorocarbon-modified organic semiconductors: molecular architecture, electronic, and crystal structure tuning of arene-versus fluoroarene-thiophene oligomer thin-film properties, J. Am. Chem. Soc., 2006, 128, 5792–5801 CrossRef CAS PubMed.
- M. Genovese and K. Lian, Polyoxometalate modified inorganic-organic nanocomposite materials for energy storage applications: A review, Curr. Opin. Solid State Mater. Sci., 2015, 19, 126–137 CrossRef CAS.
- T. Jin, H. Li, K. Zhu, P. F. Wang, P. Liu and L. Jiao, Polyanion-type cathode materials for sodium-ion batteries, Chem. Soc. Rev., 2020, 49, 2342–2377 RSC.
- R. Tsunashima, Y. Iwamoto, Y. Baba, C. Kato, K. Ichihashi, S. Nishihara, K. Inoue, K. Ishiguro, Y. F. Song and T. Akutagawa, Electrical network of single-crystalline metal oxide nanoclusters wired by pi-molecules, Angew. Chem., Int. Ed., 2014, 53, 11228–11231 CrossRef CAS.
- S. Hartung, N. Bucher, V. S. Nair, C. Y. Ling, Y. X. Wang, H. E. Hoster and M. Srinivasan, Sodium Vanadium Oxide: A New Material for High–Performance Symmetric Sodium–Ion Batteries, ChemPhysChem, 2014, 15, 2121–2128 CrossRef CAS PubMed.
- E. Ni, S. Uematsu and N. Sonoyama, Anderson type polyoxomolybdate as cathode material of lithium battery and its reaction mechanism, J. Power Sources, 2014, 267, 673–681 CrossRef CAS.
- E. Ni, S. Uematsu, Z. Quan and N. Sonoyama, Improved electrochemical property of nanoparticle polyoxovanadate K7NiV13O38 as cathode material for lithium battery, J. Nanopart. Res., 2013, 15, 673–681 Search PubMed.
- X. L. Li, Z. F. Lin, N. Jin, L. Sun, X. J. Yang and Y. Liu, Electrochemically Induced Crystalline-to-Amorphous Transition of Dinuclear Polyoxovanadate for High-Rate Lithium-Ion Batteries, Adv. Funct. Mater., 2023, 33, 2214667 CrossRef CAS.
- G. M. Sheldrick, A short history of SHELX, Acta Crystallogr., Sect. A: Found. Crystallogr., 2008, 64, 112–122 CrossRef CAS PubMed.
- K. Tang, X. Q. Yu, J. P. Sun, H. Li and X. J. Huang, Kinetic analysis on LiFePO4 thin films by CV, GITT, and EIS, Electrochim. Acta, 2011, 56, 4869–4875 CrossRef CAS.
- H. Xia, L. Lu and G. Ceder, Li diffusion in LiCoO2 thin films prepared by pulsed laser deposition, J. Power Sources, 2006, 159, 1422–1427 CrossRef CAS.
- J. Wang, J. Polleux, J. Lim and B. Dunn, Pseudocapacitive contributions to electrochemical energy storage in TiO2 (anatase) nanoparticles, J. Phys. Chem. C, 2007, 111, 14925–14931 CrossRef CAS.
|
This journal is © the Partner Organisations 2024 |
Click here to see how this site uses Cookies. View our privacy policy here.