DOI:
10.1039/D3PM00008G
(Review Article)
RSC Pharm., 2024,
1, 182-203
Advances of cassava starch-based composites in novel and conventional drug delivery systems: a state-of-the-art review
Received
7th October 2023
, Accepted 4th March 2024
First published on 27th March 2024
Abstract
Starch has emerged as a new attractive biopolymer for use in pharmaceutical applications, owing to its distinctive physical, chemical and functional properties. This biopolymer has several potential advantages: it is biocompatible, low cost, non-toxic and easily isolated from plant sources. In the pharmaceutical field, starch is used as a raw material for developing various drug delivery platforms. Generally, cassava starch (tapioca) is obtained from the swollen roots of the perennial shrub Manihot esculenta and it contains a low amount of amylose in contrast to other varieties of starches. Because of this reason, cassava starch exhibits various prime benefits, including a low gelatinization temperature, higher swelling power and a relatively high viscosity paste, making it a preferable excipient for pharmaceutical applications. However, cassava starches in their native form are not effective for many applications because of their inefficiency in handling various processing requirements like high temperature and diverse pH. Their applicability can be enhanced by starch modification. These functional starches have demonstrated outstanding prospects as primary excipients in many pharmaceutical formulations. In this article, we discuss the potential application of cassava starches in the pharmaceutical and biomedical fields, along with the toxicity assessment of modified cassava starches.
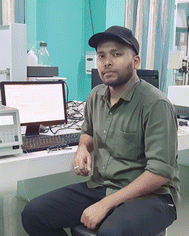 Sanjoy Das | Sanjoy Das is a Ph.D. Research Scholar in the Drug Delivery Research Laboratory (DDRL) of the Department of Pharmaceutical Sciences, Dibrugarh University, Dibrugarh, Assam, India. Mr. Das have been awarded the Senior Research Fellowship by the Indian Council of Medical Research (ICMR), New Delhi, Government of India. His area of expertise is the development of targeted nanomedicine for lung cancer treatment, microparticles, starch modification, biopolymer synthesis and in silico screening for anticancer leads. He has published several research and review articles in peer-reviewed journals and chapters in edited books published by Academic Press, Springer Nature and Bentham Science Publishers. In addition, Mr. Das has served as a peer-reviewer for many academic journals. |
1. Introduction
Biopolymers play a very significant role in the pharmaceutical field because they are used to design a range of carrier systems suitable for the transport of diverse chemical and biological agents, overcoming the limitations of synthetic or conventional polymers.1 The main reason that biopolymers have gained much popularity is that they are plentiful in nature, biodegradable and cheap, easily modifying drugs that have unfavorable pharmacokinetics and instability. They are either derived directly from biological systems or chemically synthesized from biological building blocks.2 Currently, starch has become a new promising biopolymer or excipient in the pharmaceutical field, owing to its thickening, adhesive, film-forming, gelling, and swelling properties, and its biodegradability, biocompatibility and non-toxicity. It is one of the most easily available polymers, and can be obtained from various sources such as rice, potato, corn, sago, banana, wheat, taro, yam, and starchy tubers or root vegetables like cassava (Table 1).3,4
Table 1 Various types of starch and their pharmaceutical and biomedical applications
Sl. no. |
Starch |
Sources |
Applications |
Ref. |
01. |
Potato starch |
Root tubers of potato (Solanum tuberosum) |
Gold nanoparticles for ovarian cancer |
16 and 17 |
02. |
Rice starch |
Endosperm of rice (Oryza sativa L.) |
Thin films for buccal drug delivery |
18 and 19 |
03. |
Wheat starch |
Endosperm of wheat (Triticum aestivum) |
Disintegrant in metronidazole tablet formulations |
20 and 21 |
05. |
Corn or maize starch |
Grains of corn (Zea mays L.) |
Thermoplastic starch films for chlorhexidine delivery |
22 and 23 |
06. |
Yellow nut-grass starch |
Tubers of yellow nut-grass (Cyperus esculentus L.) |
Binder for the formulation of metronidazole tablets |
24 and 25 |
07. |
Sago starch |
Stem of sago palm (Metroxylon spp.) |
Excipient for direct compression tablets |
26 and 27 |
08. |
Banana starch |
Pulp of green banana (Musa paradisiaca) |
Nanoparticles for controlled delivery of curcumin |
27 and 28 |
09. |
Amaranth starch |
Dried seeds of amaranth (Amaranthus cruentus L.) |
Natural nano starch for medical and chemical industries |
29 and 30 |
10. |
Taro starch |
Tubers of taro (Colocasia esculenta (L.) Schott) |
Filler for Thiamine Hydrochloride Tablet |
31 and 32 |
11. |
Yam starch |
Tubers of yam (Dioscorea esculenta) |
Disintegrants for paracetamol tablet formulations |
33 and 34 |
Cassava (Manihot esculenta, belonging to the family Euphorbiaceae), also called manioc or tapioca, is grown annually in tropical and subtropical areas for its edible nature. Its tuberous roots are an excellent source of starch.5 Starch derived from cassava contains a low amount of amylose (0% in waxy cassava starch) or a high amount of amylose (above 30% in self-pollinated progenies of AMYCS-3 and AMYCS-4) as compared with other types of starch. This low amount of amylose provides starch with various prime benefits including low gelatinization temperature, low retrogradation rates, and higher swelling rate, and produces comparatively high-viscosity paste, making it a preferable excipient for pharmaceutical applications.6–9 In addition, starches with a large content of amylose are more exothermic and capable of forming a more stable gel with higher strength. The significant variation of amylose amount in cassava has a profound effect on starch functional properties.10
Nevertheless, there are some limitations in the application of cassava starch due to its poor ability to withstand various processing requirements, such as its swollen nature and thermal resistance, gelatinized granules that cannot retain a granular structure, and the fact that it can collapse instantaneously. The application of cassava starch for industrial purposes is also limited by low shear stress resistance, susceptibility to thermal decomposition, high viscosity even at low concentrations, low process tolerance and strongly hydrophilic nature.11,12 These deficiencies may be improved via various modification techniques or by combining starches with other functional compounds. The techniques for native starch modifications have been generally divided into four categories, i.e., physical, chemical, enzymatic and genetic modifications. These techniques produce various novel starch moieties with improved physicochemical or functional properties, and also offer potential structural attributes for various medicinal, food, industrial or non-food purposes.13 Currently, modified starches, e.g., sodium starch glycolate (chemically modified starch) and pregelatinized starch (physically modified starch), are approved by the United States Food and Drug Administration (FDA). These modified starches are used as an excipient or matrix for drug delivery systems, for example, controlled or sustained-release tablets and capsules, subcutaneous implants, transdermal and ophthalmic systems.14,15 Several modified starches have already been used for the development of various novel microparticulate and nanoparticulate drug carriers for the treatment of diverse forms of ailments. However, systematic studies on their properties, excipient functionalities and proper toxicity assessment are still needed. This article methodically highlights the physicochemical properties, geographical sources, and potential applications of native and modified cassava starches as a material choice in the biomedical or pharmaceutical fields along with the toxicity assessment of modified cassava starches for the first time.
2. Methodology for data extraction
Considering the significance of this study, a thorough literature survey was conducted through online databases such as PubMed, SpringerLink, Science Direct, Scopus, Google Scholar and Research Gate. The title and abstract of articles were searched from the previously mentioned databases by using the corresponding keywords, i.e., starch, cassava, biopolymer, excipient, starch modification, drug delivery platforms and toxicity assessment of modified starch, to understand the recent trends of native and modified cassava starch-based materials with substantial applications in the pharmaceutical and biomedical field.
3. Geographical sources of cassava starch
The good agricultural harvest of cassava starch depends upon several climatic factors like adequate amount of sunlight, rainfall and higher temperatures. These requirements are well fulfilled by the tropics where the mean temperature is always greater than 18 °C. Though cassava is a plant of high economic importance and is considered a staple food by over 800 million people (Food and Agriculture Organization, United Nations), its geographical origins have remained controversial.35–37 Apart from the commonly known Manihot esculenta, which is the widely harvested cassava, there are various other wild variations of it often referred to as Manihot esculenta subspecies (Manihot esculenta subsp. flabellifolia and Manihot esculenta subsp. peruviana). These species are widely grown over the neotropics, viz., Peru, Venezuela, Guyana, Brazil, Bolivia and Surinam.38 Although cassava was predominantly cultivated in parts of South America, later sailors and explorers recognized its potential as a multipurpose plant. Eventually, with the advancement of agricultural technologies and better communication, cassava cultivation spread from the American neotropics to the Asian countries as well. As per the Food and Agriculture Organization Corporate Statistical (FAOSTAT) 2015 reports, world cassava production increased to >263 million tons in 2013, a 27% increase in production during the last 10 years. Of this, Africa contributed 54.8% (144.2 million), Asia 33.5% (88.2 million tons) and the Americas 11.6% (30.5 million tons). Thirty countries, which include 18 African, 4 Latin American and 8 Asian, were the major cassava growers around the globe.39,40 Furthermore, in latest FAOSTAT 2019 report, Nigeria stands to be the biggest grower of cassava, followed by Congo DR, Thailand, Indonesia, Brazil, Ghana, Angola, Cambodia and Vietnam; Thailand, Vietnam and Cambodia stand to be the largest exporters of cassava starch and flour. Furthermore, China, Japan and Indonesia vie for the place of largest importers of cassava flour and starches.41 Cassava production has shown steady growth for the last six decades from 1961 to 2017 (Fig. 1). Advancements in cassava productivity, sustainability and quality could be crucial for ensuring food security in Africa and Nigeria, where the population is predicted to double by 2050, more than in any other country. Hence, a high yield of cassava is very much essential for these regions. The expansion of cassava manufacturing will need to be critically managed, because huge production of cassava crops may not only cause environmental impacts but also contribute to habitat degradation and soil damage. Also, forests and other natural biospheres are destroyed and replaced by cassava farms. The dual aims of raising food production and minimizing environmental conflicts have led to calls for the “ecological intensification” or “sustainable intensification” of food production using “good agricultural practices”.42 To support the use of best agricultural practices, a systematic map of studies about cassava farming is urgently needed.
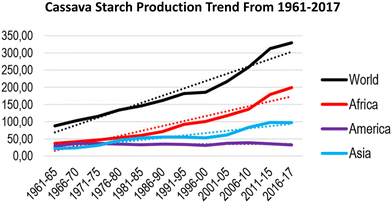 |
| Fig. 1 Graphical representation of worldwide cassava production. The most dramatic production increase in Africa and Asia is seen from 1996 to 2017, while Latin America showed more restrained increases. Adapted from open access article under Creative Commons license: Amelework et al., 2021.43 | |
4. Physicochemical properties of cassava starches as a drug delivery biopolymer
The physicochemical features of cassava starches, including organoleptic, structural, crystalline, swelling, gelatinization, pasting, retrogradation and morphological properties, are crucial factors of starch quality. This can provide a basis for the processing and usage of starch.
4.1. Organoleptic and structural properties
Organoleptic features are important aspects of cassava starch as experienced using parameters including color, odor, taste and surface texture.44,45 These properties are summarized in Table 2.
Table 2 Organoleptic properties of cassava starch
Sl. no. |
Parameters |
Observation |
01. |
Color |
White |
02. |
Odor |
Odorless |
03. |
Taste |
Tasteless |
04. |
Texture |
Homogeneous |
Like other types of starch, cassava starch contains two major molecular components, amylose and amylopectin (Fig. 2). Amylose is essentially linear, formed by units of D-glucose linked in an α-(1 → 4) manner, while amylopectin is highly branched, wherein the D-glycosidic α-(1 → 6) linkages are responsible for branching points.46 The physicochemical characteristics are greatly dependent on these two distinct structural polysaccharide fractions i.e., amylose (17–24%) and amylopectin (76–83%) in content. The interaction between amylose and amylopectin improves the viscosity and textural properties of starch, which include cohesiveness and adhesiveness.47,48 Compared with other starches like corn, rice, potato and wheat starches, there is a significant variation in the content of amylose (0–30.3%) of cassava starches, which provides superior qualities like bland taste, flavor, high paste clarity and slighter tendency to retrograde.49 In the case of amylopectin, the distribution of branch chain diameter gives an indication of swelling power, pasting viscosity and solubility. Thus, it is frequently crucial to measure the concentration of each starch component as well as the overall starch concentration.50
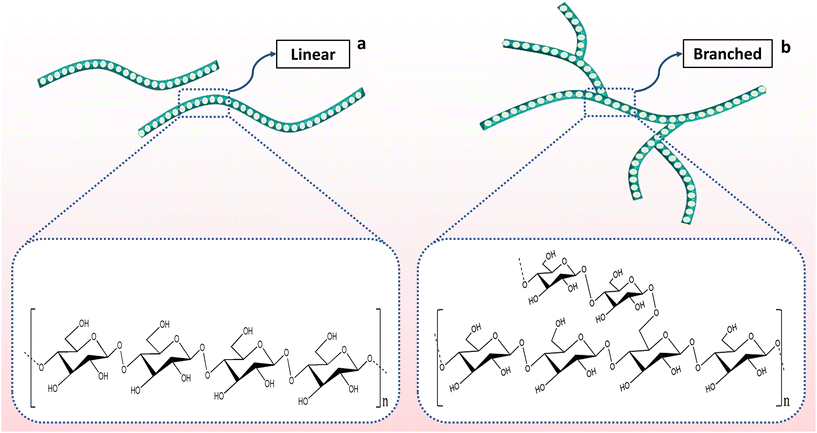 |
| Fig. 2 Chemical structure of the major molecular components of cassava starch. (a) Amylose is predominately made up of long linear chains of α-(1 → 4) glycosidic bonds between two glucose units and has a molecular weight of 105 to 106 Daltons. (b) Amylopectin consists of α-(1 → 4) glycosidic bonds between two glucose units in the straight and α-(1 → 6) glycosidic bonds at the branches, and has a very high molecular weight of 107 to 109 Daltons. | |
4.2. Crystalline properties
Starch is usually biosynthesized as semicrystalline granules whose shape and size are reliant on the botanical sources. The crystallinity is strongly associated with amylopectin molecules, while the amorphous nature is mainly represented by amylose molecules.51,52 The structural crystallinity of starches is identified as type A (Bragg angle 2θ at about 15.3°; 17.1°; 18.2°; and 23.5°), type B (Bragg angle 2θ at about 5.6°; 14.4°; 17.2°; 22.2°; and 24.0°) and type C (Bragg angle 2θ at approximately 5.6°; 15.3°; 17.3°; and 23.5°) using X-ray Diffraction (XRD) analysis.53 Cassava starches showed prominent peaks (2θ) at 15.2°, 23.4°, and a doublet at 17.2° and 18.2°, which corresponds to the A-type crystallinity (Fig. 3). Moreover, relative crystallinity ranged from 36.1 to 41.4%, which is similar to, but slightly higher than the values reported for Thai cassava, which averaged 35.8%. These crystallinity variations within cassava starch may be due to the amount of water or moisture content in the starch samples.54,55
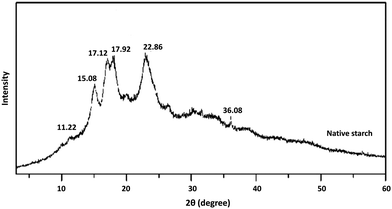 |
| Fig. 3 X-Ray diffraction pattern of native cassava starch. Native cassava starch exhibits three strong diffraction peaks (2θ) at 15.08°, 17.92°, and 22.86°, which indicates a type A crystalline structure. Adapted from open access article under Creative Commons license: Yi et al., 2020.56 | |
4.3. Swelling and solubility properties
The swelling capacity and solubility of starch depend on the ability of the starch molecule to hold water through hydrogen bonding by glucan chains. As the thermal energy rises, the bonds among the glucan chain relax and the granules absorb water and swell.57,58 The earlier works investigated the swelling capacity and solubility of starches from different sources in the temperature range of 60–90 °C. The swelling power significantly increased steadily with temperature, with a twofold change between the temperatures of 60 to 80 °C, in the case of all starches.59 Cassava and potato starches had elevated and lowered swelling capacity and solubility. The elevated swelling capacity and solubility of cassava starch are possibly due to a higher content of amylopectin in comparison with potato flour.60 However, the existence of non-starch constituents (lipids and proteins) in the starch reservoir is one of the most important aspects, harming swelling power and solubility. Since cassava starch granules contain lower amounts of lipids and protein compared with other forms of starch, this may account for their higher swelling and solubility properties.61,62
4.4. Gelatinization and pasting properties
Starch is practically insoluble in cold water; however, upon heating, the amylopectin structure of starch is altered, which causes a decrease in the crystallinity and more of the water is absorbed, leading to the formation of a gel-like mass. The process of gelatinization is mainly influenced by the breakdown of the intermolecular structure of starch fragments.63,64 Gelatinization processes are characterized by the onset temperatures (TO), peak temperatures (TP), conclusion temperature (TC) and enthalpies (ΔHgel) of the phase transitions, which vary between the starches from different sources.65 The earlier investigation reported that potato starches exhibited lower TP (64 °C), while cassava starches exhibited higher TP (71 °C), respectively. However, the TO for the two starches are nearly identical and the range of gelatinization for the cassava starch is 9 °C wider as compared with the potato starch.66 The variations in amylose concentration, length of amylopectin chain, non-starch content and degree of crystallinity may be responsible for the differences in gelatinization between different starches.67–69 Also, high transition temperatures have been observed due to a high degree of crystallinity, which provides structural stability and makes the granule more resistant to gelatinization. This explanation revealed that cassava starches are more stable than other types of starch, such as potato starch.70
Pasting usually occurs after gelatinization, resulting in the formation of amylose-amylopectin paste and a gel-like network. The pasting (rheological) features of any starches are investigated in terms of pasting temperatures and viscosities, which are characterized as peak, minimum or trough, breakdown, final and setback viscosities.71 Peak viscosity provides information on the starch's ability to bind water, and trough viscosity represents the lowest value of viscosity. The final viscosity gives an indication of the capacity of the starch to form a viscous paste or gel after cooking and cooling, while breakdown viscosity provides information regarding the rupturing of starch granules, and finally, setback viscosity is the indicator for the starch retrogradation during storage.72–74 Cassava starches are known to have low pasting temperatures because there are a lot of negatively charged phosphate groups in their structures, hence viscosity development starts at the lowest temperatures. In the case of cereal maize, rice and wheat starches, the pasting temperature is very high, due to the presence of an elevated amount of proteins or lipids and the subsequent formation of lipid–amylose complexes.75,76 Cassava starches with low pasting temperatures easily form a paste, which is an advantage for food or non-food industrial processes. This is also beneficial in the case of energy cost reductions during starch production as well as the minimum temperature required to cook the cassava starch sample. However, several factors affect the cassava starch pasting behavior, including amylose/amylopectin content and the proportion of ingredients in their matrices.77
4.5. Retrogradation and morphological properties
Retrogradation of starches is a phenomenon that occurs in gelatinized starch as it moves from an initial amorphous form to a crystalline state, resulting in the loss of its ability to hold water.78 This process is usually accelerated by a series of physical factors, such as increasing concentration of starch in the paste, amylose content and amylopectin chain length, viscosity of starch paste, degree of crystallinity and finally freeze-storage of starch paste.79,80 During the retrogradation process, the two main components of starch, i.e., amylose and amylopectin, show various functions. The initial hardness of the gel is primarily determined by the re-association of amylose, while retrogradation and long-term gelling capacity are usually influenced by the re-crystallization of amylopectin.81 Moreover, retrograded starch paste displays lower glass transition temperatures (Tg) and enthalpy than the native starch granules. After the modification of native starch, the Tg of the modified cassava starches is found to be 3–6 °C, which is significantly lower than that of the non-modified starch. This trend was ascribed to the weaker crystallinity of retrograded starch.82,83 Gomand et al. evaluated the retrogradation properties of cassava starch pastes and reported that cassava starches showed a much lower enthalpy of retrogradation and almost none compared with potato, amylose-free and high-amylose starches.84 Retrogradation is mainly influenced by low temperature, the presence of non-starch components, and polar substances like lipids, proteins, acids and salts. Cassava starches contain very low amounts of these components and significantly exhibit very low retrogradation, high peak viscosity and produce very stable and transparent gels.85–87
The morphology of starch moieties depends on amyloplast or chloroplast biochemistry, as well as plant physiology. Notably, common starches from different plants (corn, rice, wheat, potato and barley) exhibited distinct morphologies ranging from angular, pentagonal, spherical, lenticular, ovoid, irregular or cuboidal-shaped. The average diameter or shape of the starch granule varies from 1–100 μm when viewed by scanning electron microscopy (SEM).88–90 The morphological features of cassava starches were stated to be ovoid, polygonal and round granules with smooth characteristics.91 SEM analysis revealed that the surfaces of the starch granules from corn, rice, wheat, potato and barley appear to be limited polished than cassava starch granules. The variation in the sizes of cassava starch granules was ascribed to differences in the genotype and botanical origin as well as the variety of the crop.92,93 For instance, Toae et al., characterized the starches from nine Thai non-GM bred waxy cassava varieties developed in Thailand and compared them with native cassava starches. The SEM photographs affirmed that the granular morphologies were fairly similar for waxy and native cassava starches (Fig. 4). For waxy cassava starches, the granule sizes ranged from 3–33 μm with an average size of 13.55–16.91 μm, while the sizes of native cassava starch granules were found to be 13.33–14.67 μm.94
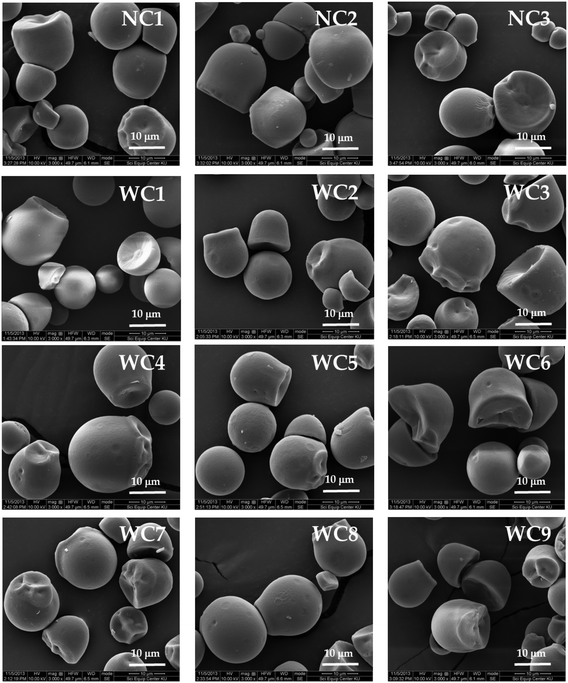 |
| Fig. 4 SEM photographs of Thai non-GM bred waxy cassava starches (WC1–WC9) and wild-type native cassava starches (NC1–NC3). Adapted from open access article under Creative Commons license: Toae et al., 2019.94 | |
5. Current usage of cassava starches in conventional drug delivery systems
Pharmaceutical excipients are compounds or materials that do not possess any health benefit but help in the manufacturing of pharmaceutical formulations. Starch is the safest excipient among the polymers used in pharmaceutical dosage forms. In several conventional formulations, starch is utilized as a binder, disintegrant, lubricant, glidant and diluent due to its nontoxic and nonirritant properties.95,96 Starches used in the pharmaceutical industry are obtained from various botanical sources like corn, potato, rice, wheat and cassava for several benefits. Compared with corn, potato, rice and wheat starch, the investigation of cassava starch as a pharmaceutical biopolymer was not extensively performed, although it appears in many standard books.97,98 Most of the investigations are done in developing countries where cassava is cultivated, mainly in South America, India, Philippines, Indonesia, China, Thailand, Malaysia, Vietnam and Indonesia.99 Conventionally, native cassava starch can be used as excipients or raw materials in tablet and capsule formulations owing to its distinct physicochemical and functional properties.100 The potential applications of cassava starches in conventional drug delivery systems are discussed below (Fig. 5).
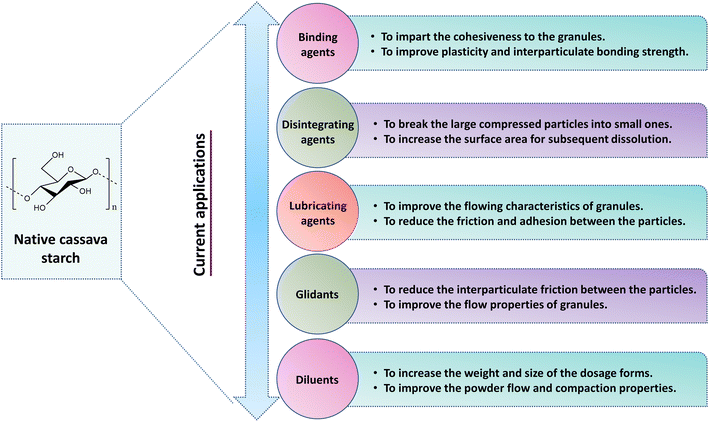 |
| Fig. 5 Current applications of cassava starches in the design of conventional drug delivery systems like tablets and capsules. | |
5.1. Binding agent
Starch is broadly utilized as a binder in the granulation step for massing or screening of materials and components in the fabrication of solid dosage forms like tablets, capsules and so on. As a binder, the starch is converted to a paste, which is generated by heating the starch, which causes the smaller formulation particles to clump together to create larger-sized agglomerates, resulting in reduced cohesiveness and encouraging flow.101,102 The amount of a binder can impart a direct effect on tablet characteristics like crushing strength and friability. As the amount of starch in the formulations is increased, the crushing strength is also raised, indicating that the starch excipients facilitate tablet binding. A study reported that cassava starch had higher crushing strength values than corn starch, and this result was directly correlated with the binding effect.103,104 Moreover, cassava starch has been also discovered to possess stronger binding capability when compared with cocoyam starch and maize starch because of the increased gel strength of its mucilage. This explanation indicates that cassava starch provides excellent binding properties and should be explored for use in pharmaceutical formulations.105
5.2. Disintegrating agents
A disintegrant is an excipient that is added in a pharmaceutical formulation to achieve the breakup of compressed solid dosage forms to small particles when they are in contact with aqueous matter, leading to an increase in surface area for subsequent dissolution.106 Starch is a cheap and convenient disintegrant that is thought to exert this action by swelling its particles in the body fluids, resulting in disruption of confining forces in the dosage form. The usual concentration range of starch as disintegrant in the tablet formulation is 2–10%.107,108 The literature reports that starches isolated from cassava offer superior disintegrant qualities over maize starch BP. This could be ascribed to the tensile and crushing strength of the tablets containing cassava starch, which decreases with an increase in starch concentration (5–10% w/w), leading to easy disintegration in aqueous medium in less than 15 minutes. Hence cassava starch provides new insight as a potential disintegrant and is used as a substitute for pharmaceutical dosage forms.109
5.3. Lubricating agents
Lubricants are agents that are mixed into tablet or capsule formulations in a very small quantity (usually 0.25% to 5.0% w/w) to improve the flowing characteristics, for example reducing the adhesion and friction among the particles and walls of the die cavity during compression.110 In the tablet pelletizing process, starch acts as a lubricant that aids the flow of particles via the pelletizing matrix.111 The role played by cassava starch as a lubricant in tablet formulation is comparable. A study reported that cassava starches showed the lowest flow properties as indicated by the Hausner ratio (HR) as well as Carr's index (IC). Flowing properties of powder or granules are rated based on official HR and IC values. An HR of <1.11 or CI of <10 is considered ‘excellent’ whereas HR >1.60 or CI >38 is considered ‘very poor’ flow. The cassava (tapioca) starch granules have HR value of 1.48 ± 0.03 and an IC value of 28.33 ± 1.53%, thereby granules obtained from cassava starch presented poor flow properties according to the HR and IC values.112,113 In addition, size, shape and uniformity of the particles are significantly involved with their flow properties. Particles of cassava starch presented a smaller diameter than that of potato starches. Because larger particles flow better than smaller ones, smaller particles have a large surface area and more surface energy to attract one another to stick together, resulting in more friction to flow. Thus, cassava starch with the lowest cohesiveness would be the starch of choice when fair flowability is desirable.114
5.4. Glidants
Glidants are inert substances that are combined with tablet formulations to reduce inter-particulate friction. These materials improve the flowing characteristics of granules from the hopper to die cavities during the early stage of compression. They are required at the surface of feed particles and are appropriately incorporated into the mixture. Tropical starches have been widely explored as glidants in many conventional tablet or capsule formulations. As a result, the flow properties of granules at concentrations of 2–10% w/w improved, thus enhancing the fabrication process and outcome.115,116 The literature reports that starches obtained from cassava have shown fair or passable flow properties as indicated by the Hausner ratio (HR) and angle of repose (AoR). The HR and AoR values of cassava starch were found to be 1.44 and 30.82°. The British Pharmacopoeia classifies powder flow as ‘excellent’ (HR: 1.00–1.11 and AoR: 25–30°), and ‘poor’ (HR: 1.35–1.45 and AoR: 46–55°). Generally, AoR values below about 30 are considered to be appropriate for solid dosage forms. Observed AoR values for cassava starch are very similar to the official values suggested in the literature. Hence, these results illustrated that cassava starches may be suitable as an alternative glidant in the field of pharmaceutical formulations.117–119
5.5. Diluents
Diluents are chemically inactive substances or inert materials that act as fillers in the fabrication of solid dosage forms like tablets or capsules.120 The diluent solubility in a formulation has been shown to affect the mechanism and rate of tablet disintegration. The main purpose of diluents in pharmaceutical formulations is that some drugs are used at very low dosages, thus making it very difficult to process them. In such circumstances, inert ingredients that do not have a therapeutic drug effect can be mixed into the formulation to bulk it up to enable the normal formulation processes. Starch is the widely used diluent or filler in tablet production due to its inert, odorless and digestible nature.121,122 Starches obtained from cassava were found to be a promising diluent for pharmaceutical formulation. However, cassava starch in tablet preparation cannot be utilized as a diluent in direct tablet compression due to its poor compressibility and flow properties. Several modification strategies have been shown to improve these functional properties, by adding other components like Avicel PH 101/PH 102 which promotes rapid wetting. As a result, this produces robust granules for fast-disintegrating tablets. Cassava starch co-processed with Avicel PH 101 improves the diluent effectiveness of starches for direct compression tablets with better flowability, friability, disintegration time and tablet uniformity. In this regard, modified cassava starch is offered as a good diluent for producing quick-dissolving tablets with adequate hardness.123,124
6. Limitations of the use of native cassava starches in drug delivery
Native cassava starch has been explored as a special carrier and conventional excipient for the delivery of various active molecules in pharmaceutical dosage forms as classic tablet disintegrants, binders, glidants and diluents.125,126 However, cassava starch in its indigenous form has certain drawbacks like hydrophilic nature, high viscosity and propensity to retrogradation even at minimum concentrations. Other drawbacks like brittleness, thermal instability, poor freeze–thaw stability, gel opacity and low process tolerance prohibit its utilization in various dosage forms.127 The application of native starch as an excipient in the extended or sustained release dosage form is restricted because of its poor compactibility resulting in the production of weak tablets. Sustained-release tablets or capsules comprising native starches are almost completely broken down by the pancreatic enzymes after oral ingestion. This leads to subsequent absorption from the small intestine, and thus fails to release drug over a prolonged period.128,129 Also, native starches exhibited higher swelling behavior (42.6 g g−1) and solubility (25.4 g g−1) over water at 90 °C. This is ascribed to low water-holding capacity, which is very unfavorable for the design of pharmaceutical dosage forms. In contrast to thermal stability and pasting profile, the native cassava starches showed low thermal transition temperature. Furthermore, native starches offer less structural stability and thermal instability, making the granule more liable to gelatinization. Native cassava starch also exhibited noticeable increases in viscosity followed by considerable paste thinning.130 Apart from this, native cassava starches have elevated lubricant sensitivity and poor flowability as well as high cohesiveness, mainly due to small particle size and large surface area, which limit their use in the formulation of direct compaction or compression tablets.131,132 These are all major constraints of native cassava starch which can be overcome by starch modification, expanding the utilization of cassava starches as an excipient in pharmaceutical dosage forms.
7. Modification of cassava starch and its application in novel microparticulate and nanoparticulate drug delivery
Starch modification means the transformation of the physicochemical properties of the native form to improve its functional properties. This modification stabilizes the starch granules during processing. Several methods including chemical, physical, enzymatic and genetic modifications have been implemented to facilitate its utilization for different purposes such as tablet excipients, drug carriers, wound dressing materials, transdermal patches and scaffolds.133,134 The chemical modifications imply the introduction of various functional groups to the structure of starch via esterification, etherification, crosslinking, oxidation and so on. Physical modifications are conferred by physical reinforcement of starch molecules under different hydrothermal conditions, pressure, shear, micronization, irradiation and electric fields without the presence of any chemical or biological reagents.135,136 In contrast, in enzymatic modification the suspension of starch is reacted with diverse enzymes, mainly hydrolyzing enzymes, which directly attack the amorphous regions and produce highly functional derivatives. In genetic modification, enzymes accountable for starch biosynthesis are genetically modified either by introducing new enzymes from other microorganisms or by silencing the plant RNA.137,138 After the modification of native starch, various properties like stability, digestibility, cold-water swellability, film formation, and emulsifying capacity are significantly enhanced. This modification also improves the water binding power and gel characteristics; as a result, its applications in the pharmaceutical field have increased.139 Notably, the most widely used modified starch in the pharmaceutical industry is pregelatinized starch. This modification not only improves the flowability, disintegration and hardness properties, but also provides excellent swelling and wettability in the cold water. Eventually, the amount of pregelatinized starch required is much less than conventional starch for tablet production.140
In the design of drug carriers, some modified starches are used as excipients for controlling the delivery speed of drug molecules to the desired site because of their low cost and good in vivo performance. For example, native starch modified with acetylation was used in the tablet preparation of lamivudine, and it was observed that tablets containing a high concentration of acetylated sago starch which acting as hydrophobic inert matrix former in the formulation of tablets. This modified starch released the drug in a controlled manner by reducing undesired swelling over time in an aqueous environment, and drug release begins when the dissolution media diffuses through the porous matrix.141 In another study, high-amylose content sodium carboxymethyl starch composites have been developed as excipients for the formulation of tablets with sustained release behavior for the oral delivery of Tramadol HCl. The results revealed that tablets containing modified starch sustained the Tramadol HCl release by preventing undesired disintegration of the tablets in the gastrointestinal tract with consequent dose dumping.142 Researchers have also designed two-release rate (2RR) monolithic tablets based on modified calcium carboxymethyl-starch (CaCMS) for controlled delivery of poorly soluble drugs. Their findings suggested that CaCMS-based tablet formulations exhibited an initial fast release of ibuprofen followed by a slow release over a period of 12 hours. This is because the CaCMS complex possesses a high hydration capacity (mainly favored by the swelling of disintegrant crospovidone) leading to a first release. As it is a 2RR tablet, there must be a partial release and even if there is an outer layer that disintegrates, the integrity of the tablets is always maintained and subsequently grants controlled release.143 Likewise, to improve the mucoadhesive features of native starch, its native structure was modified by thiol treatment and evaluated as a potential mucoadhesive excipient for formulations with sustained release behavior. The results indicated that modified starch adhered longest to the goat intestinal mucosa, which might be due to covalent tie-up via disulfide bond construction of the modified starch with the mucus involving thiol exchange reaction and simultaneously sustaining the speed of Irinotecan delivery.144 Furthermore, nanoparticulate carriers were developed by using novel starch composites for topical delivery of flufenamic acid, testosterone and caffeine. Obtained results revealed that hydrophobic flufenamic acid and testosterone were released from nanoparticles in a sustained manner without any outburst effect, while the hydrophilic drug caffeine displayed a much more immediate release owing to its hydrophilic nature. The release pattern is mainly controlled by the hydrophobic interactions among the encapsulated macromolecules (hydrophobic propyl-starch derivatives) and the nanoparticle matrix, which showed a remarkable permeation effect across the barriers of skin.145 A similar controlled-release pattern by polymeric nanoparticles has been formerly investigated by using novel crosslinked reduction-sensitive starch. The results suggested that nanoparticles with disulfide crosslinked starch accelerated the release behavior of 5-aminosalicylic acid in a controlled manner in the presence of reducing agent dithiothreitol due to reductive cleavages of disulfide linkages. Thus, modified starches expand the usefulness of the starches with indigenous form, and have provided some outstanding results as matrix-forming excipients for extended and controlled-release dosage forms.146 As discussed above, both native and modified cassava starches have been investigated as special carriers for delivering water-soluble and poorly water-soluble drugs. This supportive information shows the feasibility of cassava starches for delivering other active pharmaceutical ingredients, and is discussed in below Table 3.
Table 3 Potential of native and modified cassava starch-based formulations for drug delivery
Sl. no. |
Drug name |
Applicability for drug delivery system combined with native and modified cassava starches |
Ref. |
Yes |
No |
01. |
Buprenorphine hydrochloride |
√ (Modified cassava starches had higher water solubility) |
× (Native cassava starches had poor water solubility) |
147
|
02. |
Nilotinib hydrochloride monohydrate |
√ (Modified cassava starches had higher water solubility) |
× (Native cassava starches had poor water solubility) |
148
|
03. |
Fexofenadine hydrochloride |
√ (Modified cassava starches had higher water solubility) |
× (Native cassava starches had poor water solubility) |
149
|
04. |
Hydroxychloroquine sulfate |
√ (Modified cassava starches had higher water solubility) |
× (Native cassava starches had poor water solubility) |
150
|
05. |
Ibrutinib |
√ (Modified cassava starches had higher water solubility) |
× (Native cassava starches had poor water solubility) |
151
|
06. |
Riluzole |
√ (Modified cassava starches had higher water solubility) |
× (Native cassava starches had poor water solubility) |
152
|
07. |
Palbociclib |
√ (Modified cassava starches had higher water solubility) |
× (Native cassava starches had poor water solubility) |
153
|
08. |
Rivaroxaban |
√ (Modified cassava starches had higher water solubility) |
× (Native cassava starches had poor water solubility) |
154
|
09. |
Crizotinib |
√ (Modified cassava starches had higher water solubility) |
× (Native cassava starches had poor water solubility) |
155
|
10. |
Prazosin hydrochloride |
√ (Modified cassava starches had higher water solubility) |
× (Native cassava starches had poor water solubility) |
156
|
11. |
Pazopanib hydrochloride |
√ (Modified cassava starches had higher water solubility) |
× (Native cassava starches had poor water solubility) |
157
|
12. |
Metoclopramide hydrochloride |
√ (Modified cassava starches had higher water solubility) |
× (Native cassava starches had poor water solubility) |
158
|
13. |
Empagliflozin |
√ (Modified cassava starches had higher water solubility) |
× (Native cassava starches had poor water solubility) |
159
|
14. |
Pantoprazole sodium sesquihydrate |
√ (Modified cassava starches had higher water solubility) |
× (Native cassava starches had poor water solubility) |
160
|
15. |
Teriflunomide |
√ (Modified cassava starches had higher water solubility) |
× (Native cassava starches had poor water solubility) |
161
|
16. |
Pholcodine |
√ (Modified cassava starches had higher water solubility) |
× (Native cassava starches had poor water solubility) |
162
|
17. |
Dasatinib monohydrate |
√ (Modified cassava starches had higher water solubility) |
× (Native cassava starches had poor water solubility) |
163
|
18. |
Clemastine fumarate |
√ (Modified cassava starches had higher water solubility) |
× (Native cassava starches had poor water solubility) |
164
|
19. |
Sulfabenzamide |
√ (Modified cassava starches had higher water solubility) |
× (Native cassava starches had poor water solubility) |
165
|
20. |
Quetiapine hemifumarate |
√ (Modified cassava starches had higher water solubility) |
× (Native cassava starches had poor water solubility) |
166
|
21. |
Losartan potassium, Cozaar |
√ (Modified cassava starches had higher water solubility) |
× (Native cassava starches had poor water solubility) |
167
|
22. |
Galantamine |
√ (Modified cassava starches had higher water solubility) |
× (Native cassava starches had poor water solubility) |
168
|
23. |
Ketoconazole |
√ (Modified cassava starches had higher water solubility) |
× (Native cassava starches had poor water solubility) |
169
|
24. |
Amlodipine besylate |
√ (Modified cassava starches had higher water solubility) |
× (Native cassava starches had poor water solubility) |
170
|
25. |
Minoxidil |
√ (Modified cassava starches had higher water solubility) |
× (Native cassava starches had poor water solubility) |
171
|
26. |
Tamsulosin |
√ (Modified cassava starches had higher water solubility) |
× (Native cassava starches had poor water solubility) |
172
|
27. |
Triamterene (2,4,7-Triamino-6-phenylpteridine) |
√ (Modified cassava starches had higher water solubility) |
× (Native cassava starches had poor water solubility) |
173
|
28. |
Sodium valproate |
√ (Modified cassava starches had higher water solubility) |
× (Native cassava starches had poor water solubility) |
174
|
29. |
Lansoprazole |
√ (Modified cassava starches had higher water solubility) |
× (Native cassava starches had poor water solubility) |
175
|
30. |
Azathioprine |
√ (Modified cassava starches had higher water solubility) |
× (Native cassava starches had poor water solubility) |
176
|
31. |
Sorafenib tosylate |
√ (Modified cassava starches had higher water solubility) |
× (Native cassava starches had poor water solubility) |
177
|
32. |
Sunitinib malate |
√ (Modified cassava starches had higher water solubility) |
× (Native cassava starches had poor water solubility) |
178
|
33. |
Esomeprazole |
√ (Modified cassava starches had higher water solubility) |
× (Native cassava starches had poor water solubility) |
179
|
34. |
Repaglinide |
√ (Modified cassava starches had higher water solubility) |
× (Native cassava starches had poor water solubility) |
180
|
35. |
Oxcarbazepine |
√ (Modified cassava starches had higher water solubility) |
× (Native cassava starches had poor water solubility) |
181
|
36. |
Sertraline hydrochloride |
√ (Modified cassava starches had higher water solubility) |
× (Native cassava starches had poor water solubility) |
182
|
37. |
Imatinib mesylate |
√ (Modified cassava starches had higher water solubility) |
× (Native cassava starches had poor water solubility) |
183
|
38. |
Loratadine |
√ (Modified cassava starches had higher water solubility) |
× (Native cassava starches had poor water solubility) |
184
|
39. |
Letrozole |
√ (Modified cassava starches had higher water solubility) |
× (Native cassava starches had poor water solubility) |
185
|
40. |
Ketotifen fumarate |
√ (Modified cassava starches had higher water solubility) |
× (Native cassava starches had poor water solubility) |
186
|
41. |
Amiodarone hydrochloride |
√ (Modified cassava starches had higher water solubility) |
× (Native cassava starches had poor water solubility) |
187
|
42. |
Aprepitant |
√ (Modified cassava starches had higher water solubility) |
× (Native cassava starches had poor water solubility) |
188
|
Apart from the other starch derivatives from diverse sources, modified cassava starches have found great use in the pharmaceutical sectors for the development of various novel and conventional drug delivery vehicles. The modified cassava starches are discussed below and their structural framework is depicted in Fig. 6.
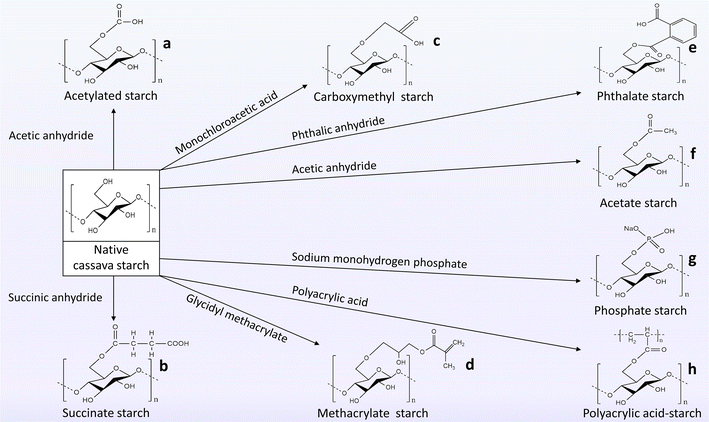 |
| Fig. 6 Modification of native cassava starches to new starch derivatives with special attention to structural illustration. This structural representation of modified cassava starches is provided here according to the information mentioned in Table 4. | |
• Acetylated starch was generated by treatment of cassava starch with acetic anhydride under alkaline conditions (a).
• Succinate cassava starch was produced by esterification with succinic anhydride in base atmospheres (b).
• Carboxymethyl starch was developed by etherification of cassava starch with monochloroacetic acid under basic conditions at the start and neutral conditions at the end of the reaction (c).
• Methacrylate cassava starch was obtained by reacting cassava starch with glycidyl methacrylate in alkaline environments (d).
• Phthalate cassava starch was developed by esterification of cassava starch with phthalic anhydride under semi-dry conditions (e).
• Acetate cassava starch was produced by esterification of cassava starch by acetic anhydride in an alkaline set-up (f).
• Phosphate cassava starch was synthesized by phosphorylation of cassava starch with sodium monohydrogen phosphate under alkaline conditions initially and acidic conditions after 2 hours (g).
• Polyacrylic acid blends starch was prepared from cassava starch and polyacrylic acid by esterification reaction (i).
Table 4 Pharmaceutical applications of newly developed modified cassava starch as an excipient
Sl. no. |
Modified starch |
Drug carriers |
Purpose |
Ref. |
01. |
Pregelatinized cassava starch succinate |
Mucoadhesive microspheres |
pH-dependent controlled delivery of propranolol HCl |
196
|
02. |
Pregelatinized cassava starch |
Floating microspheres |
Gastroretention of metronidazole for peptic ulcer |
197
|
03. |
Cassava starch methacrylate |
Crosslinked microspheres |
Sustained release of curcumin for colonic cancer |
198
|
04. |
Cassava starch acetate |
Crosslinked starch-PEG-gelatin nanocomposites |
Controlled delivery of cisplatin for solid tumor treatment |
199
|
05. |
Acetylated cassava starch |
Silver-starch nanocomposite |
Extended release of Rifampicin for multi-resistant tuberculosis |
200
|
06. |
Cassava starch acetate |
Starch-polyvinyl alcohol nanocomposites |
Controlled and sustained release of paclitaxel for breast cancer treatment |
201
|
07. |
Crosslinked cassava starch acetate |
Starch-polyvinyl alcohol-Closite30b nanocomposites |
Controlled release of curcumin for cancer treatment |
202
|
08. |
Halloysite cassava starch |
Starch-based bio-nanocomposites |
Controlled delivery of silver sulfadiazine for wound infections |
203
|
09. |
Hexadecyl cassava starch-grafted PEG |
Amphiphilic starch-based nanomicelles |
Sustained release of curcumin for cancer treatment |
204
|
10. |
Pregelatinized cassava starch phthalate |
Mucoadhesive buccal films |
Enhanced bioavailability of diltiazem HCl for hypertension |
205
|
11. |
Carboxymethyl cassava starch |
Topical gel formulations |
Controlled delivery of ibuprofen for inflammatory disease |
206
|
12. |
Poly(acrylic acid)-cassava starch graft |
Hydrogels based on starch-nanostructured hybrid systems |
Controlled release of cysteamine for microbial and bacterial disease |
207
|
13. |
Octenyl succinate cassava starch |
Starch-based tablet formulations |
Sustained release matrix for theophylline for respiratory diseases |
208
|
14. |
Pregelatinized cassava starch |
Non-effervescent floating mini tablets |
Controlled release of ranitidine HCl for acid reflux diseases |
209
|
15. |
Oxidized konjac glucomannan-cassava starch |
Starch-based matrix tablet formulations |
Sustained release excipient for bovine serum albumin |
210
|
16. |
Pregelatinized cassava starch phosphate esters |
Starch-based matrix tablet formulations |
Controlled delivery of theophylline for respiratory diseases |
211
|
17. |
Pregelatinized cassava starch |
Starch-based fast disintegrating tablets |
Immediate delivery of famotidine for geriatric and pediatric patients |
212
|
18. |
Microcrystalline tapioca starch |
Starch-based directly compressed tablets |
Delivery of poorly compressible API ascorbic acid and paracetamol |
213
|
Cassava starch deserves particular attention because of its purity and lack of non-starchy compounds like lipids, proteins and ash as distinguished from other origin starches. The modified versions of cassava starch are not only used as a good matrix for drug delivery systems but also can protect the bioactive compounds with a short half-life from degradation and carry the drug molecules to the desired site.189–191 Notably, modified cassava starches allow the incorporation of various specific ligands, particularly flavor compounds, to obtain inclusion complexes. Hydroxyl groups present in their polysaccharide backbone provide protection during processing and storage since the complexes are resistant to elevated temperatures. Free-flavor compounds are very volatile and susceptible to degradation in the presence of moisture, air, light and high temperatures. Hence by using inclusion complexes, flavor compounds can be suitably released in a controlled manner, and are applied to develop a novel carrier for the entrapment of flavor compounds in treating cardiovascular, liver, and other chronic diseases.192–195 The potential utilization of modified cassava starch in the design of novel drug carriers, especially nanomaterials and microparticles, as well as various conventional drug carriers including tablets, buccal films and topical gels, is discussed in Table 4 and illustrated in Fig. 7.
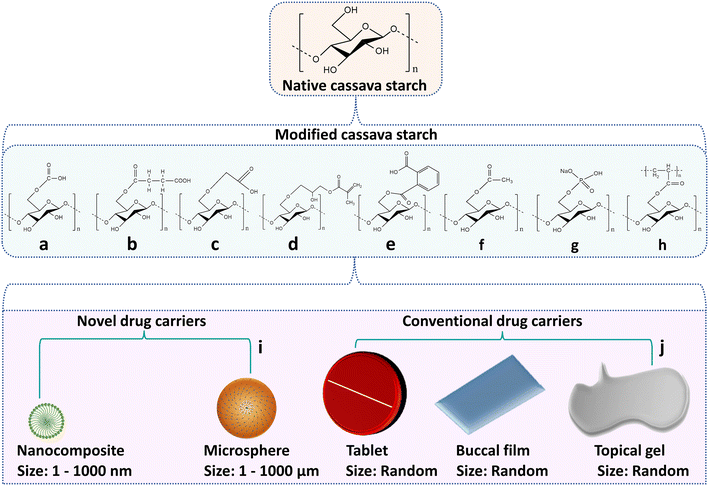 |
| Fig. 7 Potential applications of modified cassava starches. (a) Acetylated starch. (b) Succinate starch. (c) Carboxymethyl starch. (d) Methacrylate starch. (e) Phthalate starch. (f) Acetate starch. (g) Phosphate starch. (h) Polyacrylic acid-starch blends are utilized in the design of: (i) novel drug carriers and (j) conventional drug carriers. | |
Apart from the pharmaceutical applications, some modified cassava starches and their derivatives like crosslinked cassava starch phosphate, hydroxypropyl cassava starch, citrate-esterified cassava starch, dialdehyde cassava starch, cassava ethyl-O-starch, konjac glucomannan-modified cassava starch, crosslinked cassava starch and enzyme-hydrolyzed cassava starch have already been used for other industrial purposes. Application of these modified starches for industrial purposes includes their use in the food, dairy, beverage, textile, paper, dusting powder, bioplastic composites and agrochemical industries.214–221 In the food industry, modified cassava starch is used as a stabilizer, thickening agent, emulsifier, texturizer, packaging material and in ice cream formulations, while in the textile industry, it can be used for sizing, finishing, cloth printing, and coating of fabrics.222–229 Another important purpose for modified cassava starches is that they can be used as coating material, adhesive or binder for paper or non-paper constituents in the paper industry and the adhesive industry. Modified starches are also used as an adsorbent material for the evacuation of dye and heavy metals from water or other materials in chemical and engineering fields.230–233 Moreover, in many industrial applications, there is competition not only among starches from diverse sources but also between starches and other products. As a result, the development of novel materials has continuously grown and allowed the starch industry to continue its expansion. Given all these potentialities, it seems obvious that nowadays practically all industries use starch and its derivatives in single or multiple forms for precise applications. Hence, the growth of the starch industry in the future appears to be very promising, and it can be predicted that new ventures in starch modification and their diverse applications will endure, being of great interest in applied research.234,235
8. Toxicity assessment of modified cassava starch and its derivatives
Over the last decades, starch modification has had a spectacular evolution for providing novel derivatives with a plethora of applications in very diverse fields ranging from biomedical to food or non-food purposes. In parallel to the growth and ever-increasing utilization of modified starches, concerns regarding the safety and toxicity of these starches may have arisen, both during and post-administration. Though at the time of modification, native starches are treated with some chemical or biological agents including acetic anhydride, sodium hypochlorite, sodium trimetaphosphate, ammonium chloride, monochloroacetic acid, hydrochloric acid, chloropropylene glycol, etc., these chemicals are not altogether safe for consumption and may have some harmful effects on human health.236–242 However, the risks of prolonged use of these chemically modified starches are still unknown. The deep investigation of the safety profile of these modified starches is very crucial concerning the toxicological facet. In this regard, the International Toxicological Committee along with the Joint Expert Committee on Food Additives (a combined board of the WHO/FAO) have demanded that thorough studies must be performed on laboratory animals with the intent of protecting consumer safety.243 A few toxicological investigations have been carried out with modified starches, and some data have suggested a higher prevalence of structural changes in the kidneys and intestines of mice after prolonged use. It has been also found in long-term studies in rats that consuming high dietary levels of some substituted or crosslinked modified starches resulted in the increased incidence of mineral deposits in the pelvic region of the kidneys along with caecal enlargement.244,245 Given the interest in these modified cassava starches and their possible use on a large scale, toxicological studies are essential steps in obtaining information on the safe usage of these biopolymers. Various forms of toxicological screening procedure are performed, viz., acute, subchronic, chronic, carcinogenicity and genotoxicity study, as per the Organisation for Economic Co-operation and Development (OECD) guidelines (Fig. 8).
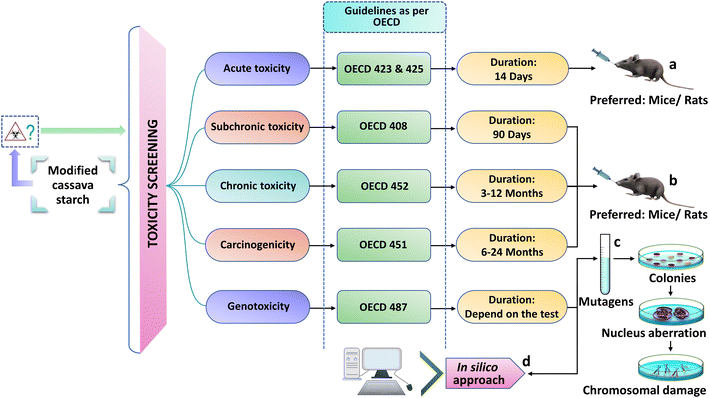 |
| Fig. 8 Toxicity screening methods of modified cassava starch and its derivatives as excipients for pharmaceutical formulations. | |
• Acute toxicity study provides preliminary information on the toxic properties of starch moieties which include possible target organ toxicity, acute adverse reactions and dissimilarities in animal growth behavior (a).
• Subchronic toxicity study provides information on gross pathological changes such as differences in biochemical and cardiovascular parameters, linked to earlier acute toxicity studies (short-term toxicity, 90 days) (b).
• Chronic toxicity study provides information on the potential health hazards regarding the long-term effects of test substances in animals, death and toxicity reversibility linked to earlier subchronic toxicity study (long term, 12 months) (b).
• Carcinogenicity testing provides information on the tumorigenic potential of starch derivatives likely to arise from repeated exposure over a period of 6 to 24 months. These carcinogenic assays are usually performed just before a compound can be marketed or if any compound shows inconclusive results in an earlier toxicity study (b).
• Genotoxicity study provides information regarding the mutagenic potential of modified starch. Initially, modified starch derivatives are mixed with bacterial strains as well as mutagenic material and directly transferred to the media with a histidine-containing Petri plate and incubated. After the incubation period, if the compound is mutagenic, colonies are formed and nuclear aberrations are detected. Subsequently, the presence and extent of chromosomal damage is evaluated. Similarly, one control group is prepared and the test carried out in a similar way without mixing any mutagenic materials, resulting in very few or no colonies being formed. Hence, if the test compounds are not mutagenic then the number of colonies on the test plate will be similar to the number of colonies on the control plate (c).
• In silico computational approaches predicting genotoxicity based on chemical structures, diverse datasets and quantitative structure–activity relationships (QSAR) prediction methodologies are recognized as alternative cost-effective toxicity estimation tools (d).
8.1. Acute toxicity
Acute toxicity studies are carried out to determine the temporary side effects of starch derivatives when given in single or multiple dosages over 24 hours and subsequently daily for a total of 14 days. This study solely provides information regarding LD50 (intermediate lethal dose), therapeutic index, the safety profile of starch derivatives and safe acute doses for humans.246,247 Moreover, the measurement of LD50 has now been utilized as a major criterion in assessing acute toxicity and is the initial procedure for basic screening of pharmacological agents as well as excipients, mainly starch and polymers, for toxicity.248,249 Aside from mortality, other parameters, mainly duration or time of onset, length of recovery of surviving animals, relative organ weight and hematological parameters, are also imperative in the evaluation of acute toxicity. Hence, the results obtained from the acute toxicity study act as a guide in the selection of doses for the investigation of long-term toxicity and also other investigations that involve the usage of animals.250 Notably, different methods (Miller and Tainter method, Karber's method, Lorke's method, Fixed-dose method, Up-/-Down method, Acute Toxic Class method) and guidelines (OECD 423 and OECD 425) have been developed and adopted for the testing of acute toxicity.251,252 In a study, assessment of the acute oral toxicity of modified cassava starch acetate was performed on thirty male and thirty female rats. Animals were separated into 6 different groups and treated with single individual doses of cassava starch acetate (dose range 5, 50, 300, 2000 and finally 5000 mg per kg body weight). After the treatment, all these animals were closely observed for any sign of toxicity for up to 29 days. The results revealed that rats treated with cassava starch acetate did not exhibit any sign of clinically adverse reactions, abnormality, or death. These results may further contribute as a reference safety study which is required for the large-scale commercialization of modified cassava starch.253
8.2. Subchronic toxicity
Subchronic toxicity studies are usually advised for 3 months (90 days), but may be advised to run for up to 12 months in single rodent and non-rodent breeds according to OECD guideline 408. During the study period, all animals are observed for the various pathological conditions that have been linked to behavioral changes and weight variations. Finally, at the end of the experiment, all the animals are killed and the tissues subjected to histopathological analysis to observe the gross pathological changes like differences in biochemical and cardiovascular parameters. Moreover, the results obtained from this study can help to predict the accurate dosages of the starch derivatives for future chronic or long-term toxicity studies.254,255 In a research investigation, 90 days of acute oral toxicity assessments of biodegradable film from acetate cassava starch were investigated in Wister rats (dose range 3, 30, and 300 mg per kg body weight). The obtained results revealed that cassava starch acetate (300 mg per kg body weight) does not cause obvious signs of toxicity or changes in relative organ weight. Histopathological evaluations did not show much difference in comparison with the control group (water), which further indicates the safety of these acetate cassava starch-based biofilms. However, further studies in this area are essential for the exploration of suitable safety efficacy of modified cassava starch.256
8.3. Chronic toxicity
In general, studies on chronic toxicity are performed to describe the profile of starch derivatives in a mammalian breed (especially rodents and non-rodents) for a period of 3 months to 1 year following repeated and prolonged exposure. The results of the chronic toxicity study provide information on the possible health hazards regarding the long-term effects of test substances in animals, toxicity reversibility, death and potential target organ toxicity.257,258 It has also been reported that the chronic toxicity study is crucial for new molecular entities (new drug formulations, polymer derivatives, starch derivatives) and should be started when phase II clinical trials show the efficacy of the same. Simultaneously, these studies could be performed with Phase III clinical trials and should be used to reinforce the safety of long-term clinical trials and marketing approval.259 However, no such information has been reported for the modified version of cassava starches in animal models, hence the execution of a chronic toxicity study is very important for further investigation of the safety profile of these starches.
8.4. Carcinogenicity
The carcinogenicity study measures the tumorigenic role played by various materials such as starch derivatives, plant extracts or small molecule pharmaceuticals, likely to appear from continuous exposure for a period lasting up to the whole lifetime of the test animals used. Carcinogenicity testing should be carried out only if information from other origins indicates a propensity for tumor initiation, and is typically conducted in mouse or rat models. The duration of the study is generally 2 years; however, these studies should be performed if the expected clinical use of the materials would be continuous for at least 6 months or more than that.260,261 Pharmaceuticals provided for a brief period, for example, anesthetics, antibiotics, and radio-tagged imaging materials and diagnostic aids do not require carcinogenicity tests unless there is a reason for concern. Moreover, completion of rodent carcinogenicity testing is not necessary before the commencement of a large clinical study, unless there is a specific consideration for the patient group. For pharmaceuticals that are used to treat fatal or severely disabling disorders, a carcinogenicity test is not required before the market clearance, but tests should be carried out after approval. If such material is designed to be provided to people on a long-term basis, a chronic toxicity test (up to 1 year) may be necessary to detect early tumorigenic effects. New materials or derivatives that show inconclusive results from in vitro analysis and limited in vivo bioassays should be considered for carcinogenicity studies. The carcinogenic potential of modified cassava starches is unexplored because there is no such information available from earlier chronic toxicity study or the animal models.262,263
8.5. Genotoxicity
Genotoxicity testing is intended to identify genetic damage such as gene mutations, DNA damage and chromosomal aberration, which may be reflected in inheritable or tumorigenic mutation potential of the drug or novel molecular compounds. This study is a crucial part of the preclinical safety assessment of new drugs, and is mainly required just before Phase I/II clinical trials.264 Genetic toxicity testing of chemicals is performed using various in vitro approaches which provide information regarding the initial genetic toxicity, and in vivo approaches are suitable for estimating secondary genotoxic reactions like oxidative stress and inflammation. There are several technological preferences in and out of the field of genetic toxicology, including flow cytometric analysis, 3D culture systems, micronucleus tests and high-throughput methods of gene expression assay. This technological approach measures the diverse parameters or effects on the genome leading to cancer as well as mutation, and finally interprets the genotoxicity test outcomes.265,266 However, evaluation of genotoxicity of the modified starch derivatives from other sources (namely oxidized starch, monostarch phosphate, acetylated starch, acetylated distarch phosphate, hydroxypropyl starch, starch sodium octenyl succinate) was evaluated only via in silico study. No genotoxicity studies were available for these starches or for modified cassava starches too. The in silico investigations of modified starch substructures revealed no evidence of genotoxicity, hence, these modified starches do not raise concern for genotoxicity.267 It should be noted that if there is any cause for concern, the genotoxicity study is a crucial step for further safety assessment of modified starches.
9. Recent patents issued in the area of cassava starch research
Technological innovations at the intersections of information technology, engineering, biotechnology, medicine and pharmaceutical sciences are triggering new routes in research & development (R & D) or commercialization. In this regard, intellectual property rights (IPR) play a very important role both in the economic and social development of mankind. Intellectual property not only gives licensed rights to inventors and industries, but also provides authority and protection to transfer their right to use to other people lawfully. One system to guarantee IPR is patenting processes and products.268,269 Patents are one of the most significant inventive parameters attempted to devise the intrinsic value of an original invention and encourage creativity.270 In recent times, patents on starch and starch-based materials have opened a new route for the development of biocompatible or biodegradable pharmaceutical excipients. According to the statistical data from 2000, the global market consisted of around 48.5 million tons of starch including native as well as modified starch from diverse plant sources. It is not only food for humans but also a renewable and biodegradable polymer with a variety of industrial applications. The total annual income from the starch sector is approximated to be €15 billion.271 Additionally, the significant role played by cassava starch used as a biopolymer in pharmaceutical and other fields has gained much attention due to the increasing number of publications and patents issued every year by the Patent and Trademark Office.272 The patents relevant to cassava starch-based inventions applied in drug carriers or as excipients were searched in various databases like Google Patents and Espacenet. After conducting screening processes, patents registered on excipients, drug delivery, nanoparticles, microspheres, tablets and topical gels as well as other industrial purposes are included in the patent analysis and summarized in Table 5.
Table 5 Recent patents published for the cassava starch-based invention (2017–2022)
Sl. no. |
Inventors |
Title |
Patent no. and year |
Ref. |
01. |
Gao Yuanyuan & Co-inventor |
Method for preparing essential oil microcapsules by taking cassava starch alcohol-free esterification mixture as wall material |
CN114190507 (A) & 2022 |
273
|
02. |
Xue Xingyong & Co-inventor |
Preparation method and application of phosphoric acid-based geopolymer/starch composite porous microsphere adsorption material |
CN114950355 (A) & 2022 |
274
|
03. |
Hanchett Douglas & Co-inventor |
Thermally inhibited waxy cassava starch |
AU2022209338 (A1) & 2022 |
275
|
04. |
Yan Kailiang |
Cassava starch residue-liquid separation device |
CN217490061 (U) & 2022 |
276
|
05. |
Lin Rihui & Co-inventor |
Preparation method and application of kaempferol starch nanoparticles |
CN113577307 (A) & 2021 |
277
|
06. |
Huang Lijie & Co-inventor |
Cassava residue nanocellulose-cassava starch film and preparation method thereof |
CN113831563 (A) & 2021 |
278
|
07. |
Lu Yefei & Co-inventors |
Long-term storage and recovering method of cassava crossbred capsules |
CN112514751 (A) & 2021 |
279
|
08. |
Su Shaozhen & Co-inventor |
Preparation method of intercalation modified montmorillonite/cassava starch composite film |
CN113150393 (A) & 2021 |
280
|
09. |
Liao Bo |
Antiseptic process for preparing cassava starch |
CN111205373 (A) & 2020 |
281
|
10. |
Lin Rihui & Co- inventor |
Starch nanoparticles with controllable crystallinity as well as preparation method and application thereof |
CN112321853 (A) & 2020 |
282
|
11. |
Li Yilun & Co-inventor |
Method for improving viscosity of cassava starch |
CN111808205 (A) & 2020 |
283
|
12. |
Ou Wenjun & Co-inventor |
Cassava preservative |
CN111543476 (A) & 2020 |
284
|
13. |
Chen Hui |
Levonorgestrel tablet and preparation method thereof |
CN109276550 (A) & 2019 |
285
|
14. |
Li Heping |
Preparation method for aminated cross-linked AA/MA/EA grafted xanthogenated cassava starch magnetic imprinted microspheres |
CN109280187 (A) & 2019 |
286
|
15. |
Luo Mingchang & Co-inventor |
Preparation method of oxidized hydroxypropyl starch for pharmaceutical capsules |
CN109400726 (A) & 2019 |
287
|
16. |
Shi Xiaodan & Co-inventor |
Method for producing succinic acid-modified cassava starch through ultra-high-pressure microfluidization method |
CN109957035 (A) & 2019 |
288
|
17. |
Li Changying |
Method for preparing cassava starch microspheres |
CN107814880 (A) & 2018 |
289
|
18. |
Ma Xianli & Co-inventor |
Preparation method for cassava oxidized starch-based adhesive |
CN107603513 (A) & 2018 |
290
|
19. |
Li Heping & Co-inventor |
Preparation method for magnetic cross-linked AA/AM graft esterified cyanoethyl tapioca starch microspheres |
CN107722533 (A) & 2018 |
291
|
20. |
Lin Ooi Poh & Co-inventor |
Starch extraction |
WO2017025482 (A1) & 2018 |
292
|
21. |
Swaile Frederick David & Co-inventor |
Aerosol composition comprising particulate tapioca starch |
JP2017061543 (A) & 2017 |
293
|
22. |
Yin Xiulian & Co-inventor |
Method for preparing roxithromycin sustained release tablet through phosphorylated porous cassava starch |
CN106727394 (A) & 2017 |
294
|
23. |
Li Changying |
Preparation method of cassava starch microspheres |
CN106389345 (A) & 2017 |
295
|
24. |
Zhifeng Ren & Co-inventor |
Method to synthesize graphene-based amphiphilic Janus nanosheets |
US20200377675 (A1) & 2017 |
296
|
10. Conclusions and outlook
Starches from cassava have found a wide array of uses in pharmaceutical formulations as a safe excipient. However, some of their intrinsic physicochemical properties make them less efficient as a multifunctional excipient, and need a few technical modifications to qualify as a potential pharmaceutical excipient. A large library of modified cassava starches is currently being developed for the design of drug delivery carriers, as they can control the delivery speed of drug molecules to the desired site with relatively low cost, accessibility and good in vivo performance. Various types of modified cassava starch are well documented in the literature for their efficacy in the formulation of microspheres, nanocomposites, tablet formulations, buccal films and topical gels, etc. Despite the promising benefits offered by these modified starches, their safety and toxicity assessment are the major concerns. A few toxicological investigations have already been conducted on the modified starches obtained from cassava. The obtained results suggested that the modified starch did not show any sign of clinical toxicity, which further supports their safe or biocompatible nature. This versatility of modified cassava starches allows their utility in diverse fields of knowledge and futuristic materials for dosage form design. Finally, systematic studies on cassava starches are suggested to obtain better understanding of their properties, which will be helpful for their timely application as pharmaceutical excipients.
Authors contribution
S. D. conceptualized, writing-original draft, reviewed and edited to all aspects of the article. M. K. D. reviewed and edited the revised version of the article. T. J., B. B., R. M., P. K. Y., N. R. G. B., T. D., D. R., B. S., B. D., I. D., K. P., A. R., A. C., P. S., D. S., D. B., B. K. J., D. T., N. G., B. K. and S. M. discussed and contributed equally throughout the article. S. D. additionally contributed to drawing all the chemical structures by using ChemDraw. S. D., M. K. D. and S. M. substantially contributed to drawing, visualization and drafting all the figures and tables.
Conflicts of interest
The authors declare that they have no known conflicts of interest that could have appeared to influence the work reported in this paper. All the tables are self-made and original. Some figures are self-made and original and some are adapted from open-access articles under the Creative Commons license agreement (citation mentioned throughout the figures caption).
Acknowledgements
The authors are thankful to the Indian Council of Medical Research (ICMR), Government of India for offering Senior Research Fellowship to Mr. Sanjoy Das to conduct this work. The authors further thank the Department of Pharmaceutical Sciences, Dibrugarh University, Dibrugarh, Assam; Department of Natural Products, NIPER, Kolkata, West Bengal; Department of Pharmacy, Tripura University, Suryamaninagar, Tripura; Faculty of Pharmaceutical Science, Assam down town University, Guwahati, Assam; School of Pharmaceutical Sciences, Shoolini University of Biotechnology and Management Sciences, Solan, Himachal Pradesh; Department of Pharmaceutics, ISF College of Pharmacy, Moga, Punjab; Institute of Pharmacy, Silchar Medical College and Hospital, Silchar, Assam; and Department of Biotechnology, NIT, Durgapur, West Bengal for providing the platform to conduct this work.
References
- S. Gopi, A. Amalraj, N. P. Sukumaran, J. T. Haponiuk and S. Thomas, Macromol. Symp., 2018, 380, 1800114 CrossRef.
- E. A. Hassan, J. Bai and D. Dou, Egypt. J. Chem., 2019, 62, 1725–1737 Search PubMed.
- B. J. Bordoloi, B. Kalita and D. Shil, Int. J. Curr. Pharm. Res., 2019, 11, 54–59 CrossRef CAS.
- S. W. Horstmann, K. M. Lynch and E. K. Arendt, Foods, 2017, 6, 29 CrossRef PubMed.
- Y. Yang, X. Luo, W. Wei, Z. Fan, T. Huang and X. Pan, Sci. Rep., 2020, 10, 14197 CrossRef CAS PubMed.
- S. M. Chisenga, T. S. Workneh, G. Bultosa and A. M. Alimi, J. Food Sci. Technol., 2019, 56, 2799–2813 CrossRef CAS PubMed.
- A. Rolland-Sabaté,
et al.
, Food Hydrocolloids, 2012, 27, 161–174 CrossRef.
- A. Rolland-Sabaté,
et al.
, Carbohydr. Polym., 2013, 92, 1451–1462 CrossRef PubMed.
- A. Karlström, F. Calle, S. Salazar, N. Morante, D. Dufour and H. Ceballos, Front. Plant Sci., 2016, 7, 604 Search PubMed.
- I. Cornejo-Ramírez, O. Martínez-Cruz, C. L. Del-Toro-Sánchez, F. J. Wong-Corral, J. Borboa-Flores and F. J. Cinco-Moroyoqui, CyTA – J. Food, 2018, 16, 1003–1017 CrossRef.
- Z. Wang, P. Mhaske, A. Farahnaky, S. Kasapis and M. Majzoobi, Food Hydrocolloids, 2022, 129, 107542 CrossRef CAS.
- L. D. Pérez-Vergara, M. T. Cifuentes, A. P. Franco, C. Pérez-Cervera and R. Andrade-Pizarro, NFS J., 2020, 21, 39–49 CrossRef.
- Ziauddin, H. Xiong and F. Peng, Crit. Rev. Food Sci. Nutr., 2017, 57, 2691–2705 CrossRef CAS PubMed.
- M. A. V. T. Garcia, C. F. Garcia and A. A. G. Faraco, Starch/Staerke, 2020, 72, 1900270 CrossRef CAS.
- N. Charoenthai, T. Sanga-Ngam and S. Puttipipatkhachorn, Pharm. Sci. Asia, 2018, 45, 195–204 CrossRef CAS.
- J. Li,
et al.
, Inorg. Chem. Commun., 2023, 155, 111002 CrossRef CAS.
- H. Gujral,
et al.
, Int. J. Biol. Macromol., 2021, 186, 155–162 CrossRef CAS PubMed.
- S.-Y. Chan, J. Y. Lau, Y. C. Tiew and T. Balakrishnan, Int. J. Pharm., 2019, 562, 203–211 CrossRef CAS PubMed.
- S. Xing,
et al.
, PLoS One, 2016, 11, e0168467 CrossRef PubMed.
- M. A. Odeniyi and J. O. Ayorinde, Polim. Med., 2014, 44, 147–155 Search PubMed.
- K.-H. Kim and J.-Y. Kim, Plants, 2021, 10, 2282 CrossRef CAS PubMed.
- V. M. Queiroz, I. C. S. Kling, A. E. Eltom, B. S. Archanjo, M. D. Prado and R. A. Simão, Mater. Sci. Eng., 2020, 112, 110852 CrossRef CAS PubMed.
- C. P. Palanisamy, B. Cui, Z. Hong-Xia, S. Jayaraman and K. M. Gothandam, Polymers, 2020, 12, 2161 CrossRef CAS PubMed.
- R. V. Manek, P. F. Builders, W. M. Kolling, M. Emeje and O. O. Kunle, AAPS PharmSciTech, 2012, 13, 379–388 CrossRef CAS PubMed.
- R. T. Widodo, A. Hassan, K. B. Liew and L. C. Ming, Polymers, 2022, 14, 3050 CrossRef CAS PubMed.
- C. Du, J. Fan, W. Jiang, W. Ge and S. Du, Int. J. Biol. Macromol., 2020, 164, 1785–1793 CrossRef CAS PubMed.
- L. Acevedo-Guevara, L. Nieto-Suaza, L. T. Sánchez, M. I. Pinzón and C. C. Villa, Int. J. Biol. Macromol., 2018, 111, 498–504 CrossRef CAS PubMed.
- H. Marta, Y. Cahyana, M. Djali and G. Pramafisi, Polymers, 2022, 14, 3092 CrossRef CAS PubMed.
- R. Zuo,
et al.
, Int. J. Biol. Macromol., 2024, 260, 129525 CrossRef CAS PubMed.
- F. Zhu, Crit. Rev. Food Sci. Nutr., 2015, 57, 313–325 CrossRef PubMed.
- P. M. Lestari, A. Widayanti and H. Afifah, Open Access Maced. J. Med. Sci., 2019, 7, 3827–3832 CrossRef PubMed.
- N. Sit, S. C. Deka and S. Misra, J. Food Sci. Technol., 2015, 52, 4324–4332 CrossRef CAS PubMed.
- N. Nattapulwat, N. Purkkao and O. Suwithayapan, AAPS PharmSciTech, 2009, 10, 193–198 CrossRef CAS PubMed.
- O. A. Odeku and B. L. Akinwande, Saudi Pharm. J., 2012, 20, 171–175 CrossRef PubMed.
- A. M. Fermont, P. J. A. Van Asten, P. Tittonell, M. Van Wijk and K. E. Giller, Field Crops Res., 2009, 112, 24–36 CrossRef.
-
K. Kawano, in Hybridization of Crop Plants, ed. W. R. Fehr and H. H. Hadley, ASA, Madison, 1st edn, 1980, ch. 13, pp. 225–233 Search PubMed.
- S. Latif and J. Müller, Trends Food Sci. Technol., 2015, 44, 147–158 CrossRef CAS.
- A. C. Allem, Genet. Resour. Crop Evol., 1994, 41, 133–150 CrossRef.
-
H. Ceballos, E. Okogbenin, J. C. Pérez, L. A. B. López-Valle and D. Debouck, in Root and Tuber Crops: Handbook of Plant Breeding, ed. J. Bradshaw, Springer, New York, 2010, vol. 7, ch. 2, pp. 53–96 Search PubMed.
- P. Chavarriaga-Aguirre, In Vitro Cell. Dev. Biol.: Plant, 2016, 52, 461–478 CrossRef CAS PubMed.
- O. A. Otekunrin and B. Sawicka, Acta Sci. Agric., 2019, 3, 194–202 CrossRef.
- G. E. Shackelford, N. R. Haddaway, H. O. Usieta, P. Pypers, S. O. Petrovan and W. J. Sutherland, Environ. Evid., 2018, 7, 30 CrossRef.
- A. B. Amelework, M. W. Bairu, O. Maema, S. L. Venter and M. Laing, Front. Sustain. Food Syst., 2021, 5, 617783 CrossRef.
- T. A. Dada, L. I. Barber, L. Ngoma and M. Mwanza, Food Sci. Nutr., 2017, 6, 395–399 CrossRef PubMed.
- A. Edhirej, S. M. Sapuan, M. Jawaid and N. I. Zahari, Polym. Compos., 2018, 39, 1704–1715 CrossRef CAS.
- P. Tappiban, D. R. Smith, K. Triwitayakorn and J. Bao, Trends Food Sci. Technol., 2019, 83, 167–180 CrossRef CAS.
- A. L. Charles, Y. H. Chang, W. C. Ko, K. Sriroth and T. Huang, J. Agric. Food Chem., 2005, 53, 2717–2725 CrossRef CAS PubMed.
- D. O. Oni, J. Mwero and C. Kabubo, Open J. Civ. Eng., 2020, 14, 289–301 CrossRef.
- F. Zhu, Carbohydr. Polym., 2015, 122, 456–480 CrossRef CAS PubMed.
- M. Raphael,
et al.
, J. Plant Breed. Crop Sci., 2011, 3, 195–202 CAS.
-
D. Lourdin, J. L. Putaux, G. Potocki-Véronèse, C. Chevigny, A. Rolland-Sabaté and A. Buléon, in Starch: Metabolism and Structure, ed. Y. Nakamura, Springer, Tokyo, 1st edn, 2015, ch. 3, pp. 61–90 Search PubMed.
- R. Bajaj, N. Singh, A. Kaur and N. Inouchi, J. Food Sci. Technol., 2018, 55, 3799–3808 CrossRef CAS PubMed.
- S. M. Chisenga, T. S. Workneh, G. Bultosa and M. Laing, AIMS Agric. Food, 2019, 4, 939–966 Search PubMed.
- N. Morante,
et al.
, Food Hydrocolloids, 2016, 56, 383–395 CrossRef CAS.
- M. K. Mtunguja, Starch-Starke, 2016, 68, 1–14 CrossRef.
- T. Yi,
et al.
, Processes, 2020, 8, 329 CrossRef CAS.
- T. Sasaki and J. Matsuki, Cereal Chem., 1998, 75, 525–529 CrossRef CAS.
- L. M. Nwokocha, N. A. Aviara, C. Senan and P. A. Williams, Carbohydr. Polym., 2009, 76, 362–367 CrossRef CAS.
- E. Nuwamanya, Y. Baguma, E. Wembabazi and P. Rubaihayo, Afr. J. Biotechnol., 2011, 10, 12018–12030 CAS.
- H. Kusumayanti, N. A. Handayani and H. P. Santosa, Procedia Environ. Sci., 2015, 23, 164–167 CrossRef CAS.
- M. R. Debet and M. J. Gidley, Carbohydr. Polym., 2006, 64, 452–465 CrossRef CAS.
- J. A. Montagnac, C. R. Davis and S. A. Tanumihardjo, Compr. Rev. Food Sci. Food Saf., 2009, 8, 181–194 CrossRef CAS PubMed.
- W. S. Ratnayake and D. S. Jackson, Adv. Food Nutr. Res., 2009, 55, 221–268 CAS.
- M. C. Garcia, C. M. L. Franco, M. S. S. Júnior and M. Caliari, J. Therm. Anal. Calorim., 2016, 123, 919–926 CrossRef CAS.
- B. Y. Ritika, B. S. Khatkar and B. S. Yadav, Int. J. Food Prop., 2010, 13, 1339–1354 CrossRef CAS.
- J. Da Cruz Francisco, J. Silverio, A. Eliasson and K. Larsson, Food Hydrocolloids, 1996, 10, 317–322 CrossRef CAS.
- J. Jane,
et al.
, Cereal Chem., 1999, 76, 629–637 CrossRef CAS.
- S. Varavinit, S. Shobsngob, W. Varanyanond, P. Chinachoti and O. Naivikul, Starch/Staerke, 2003, 55, 410–415 CrossRef CAS.
- A. L. Charles, Y. H. Chang, W. C. Ko, K. Sriroth and T. Huang, J. Agric. Food Chem., 2005, 53, 2717–2725 CrossRef CAS PubMed.
- S. C. Alcázar-Alay and M. A. A. Meireles, Food Sci. Technol., 2015, 35, 215–236 CrossRef.
- W. Berski,
et al.
, Carbohydr. Polym., 2011, 83, 665–671 CrossRef CAS.
- Q. Sun, PLoS One, 2014, 9, e95862 CrossRef PubMed.
- K. S. Sandhu and N. Singh, Int. J. Food Prop., 2005, 8, 481–491 CrossRef CAS.
- I. L. Batey and B. M. Curtin, Cereal Chem., 2000, 77, 754–760 CrossRef CAS.
- J. Waterschoot, S. Gomand, E. Fierens and J. A. Delcour, Starch/Staerke, 2015, 67, 14–29 CrossRef CAS.
- O. O. Oladunmoye, O. C. Aworh, B. Maziya-Dixon, O. L. Erukainure and G. N. Elemo, Nutr. Food Sci., 2014, 2, 132–138 CrossRef CAS PubMed.
- T. Thanyasiriwat,
et al.
, Plant Biol., 2014, 16, 197–207 CrossRef CAS PubMed.
- S. Park, Y. Na, J.-W. Kim, S. D. Kang and K.-H. Park, Food Technol. Biotechnol., 2018, 27, 299–312 CAS.
- S. Wang, C. Li, L. Copeland and Q. Niu, Compr. Rev. Food Sci. Food Saf., 2015, 14, 568–585 CrossRef CAS.
- S. Wang, C. Li, X. Zhang, L. Copeland and S. Wang, Sci. Rep., 2016, 6, 20965 CrossRef CAS PubMed.
- M. A. Ottenhof and I. A. Farhat, Biotechnol. Genet. Eng. Rev., 2004, 21, 215–228 CrossRef CAS PubMed.
- T. Tran, K. Piyachomkwan and K. Sriroth, Starch/Staerke, 2007, 59, 46–55 CrossRef CAS.
- X. Lian, K. Cheng, D. Wang, W. Zhu and X. Wang, Int. J. Food Prop., 2017, 20, 3224–3236 CrossRef.
- S. Gomand, L. Lamberts, R. G. F. Visser and J. A. Delcour, Food Hydrocolloids, 2010, 24, 424–433 CrossRef CAS.
- M. Achor, J. Y. Oyeniyi, M. H. Musa and M. S. Gwarzo, J. Appl. Pharm. Sci., 2015, 5, 126–131 CrossRef CAS.
- M. C. Garcia, T. M. Elias, K. De Oliveira Ribeiro, M. S. S. Júnior and M. Caliari, Food Sci. Technol., 2018, 39, 803–809 CrossRef.
- T. P. R. D. Santos, C. M. L. Franco, M. M. Mischan, D. De Souza Fernandes, M. S. DelBem and M. Leonel, Aust. J. Crop Sci., 2019, 13, 1486–1494 Search PubMed.
- S. Pérez and E. Bertoft, Starch-Starke, 2010, 62, 389–420 CrossRef.
- M. Gupta, A. S. Bawa and A. D. Semwal, Int. J. Food Prop., 2009, 12, 587–604 CrossRef.
- H. S. P. Lizarazo, R. Hurtado and C. L. F. Rodríguez, Agron. Colomb., 2015, 33, 244–252 CrossRef.
- W. Wang, C. Hostettler, F. F. Damberger, J. Kossmann, J. R. Lloyd and S. C. Zeeman, Front. Plant Sci., 2018, 9, 1562 CrossRef PubMed.
- S. A. Oyeyinka, A. A. Adeloye, S. Smith, B. O. Adesina and F. F. Akinwande, Agrosearch, 2019, 19, 28–45 CrossRef.
- S. Mishra and T. Rai, Food Hydrocolloids, 2006, 20, 557–566 CrossRef CAS.
- R. Toae,
et al.
, Plants, 2019, 8, 447 CrossRef CAS PubMed.
- Y. Hong, G. Liu and Z. Gu, Drug Delivery, 2016, 23, 12–20 CrossRef CAS PubMed.
- P. Juneja, B. Kaur, O. A. Odeku and I. Singh, J. Drug Delivery, 2014, 2014, 827035 Search PubMed.
- O. A. Odeku, Starch-Starke, 2013, 65, 89–106 CrossRef CAS.
- W. Abotbina,
et al.
, Materials, 2022, 15, 6992 CrossRef CAS PubMed.
- A. I. Malik,
et al.
, Breed. Sci., 2020, 70, 145–166 CrossRef PubMed.
- C. Siriwachirachai and T. Pongjanyakul, Pharmaceutics, 2022, 14, 1245 CrossRef CAS PubMed.
- N. Visavarungroj, J. Herman and J. P. Remon, Int. J. Pharm., 1990, 59, 73–78 CrossRef CAS.
- L. Vandevivere,
et al.
, Int. J. Pharm., 2019, 571, 118760 CrossRef CAS PubMed.
- M. V. Lawal, M. A. Odeniyi and O. A. Itiola, Asian Pac. J. Trop. Biomed., 2015, 5, 585–590 CrossRef.
- J. I. Ordu and J. I. Onyemelukwe, Int. J. Life Sci. Res., 2018, 4, 1834–1843 Search PubMed.
- D. B. Abdallah, N. A. Charoo and A. S. Elgorashi, Pharm. Biol., 2014, 52, 935–943 CrossRef CAS PubMed.
- D. Markl and J. A. Zeitler, Pharm. Res., 2017, 34, 890–917 CrossRef CAS PubMed.
- O. A. Odeku and B. L. Akinwande, Saudi Pharm. J., 2012, 20, 171–175 CrossRef PubMed.
- J. T. Ingram and W. Lowenthal, J. Pharm. Sci., 1968, 57, 393–399 CrossRef CAS PubMed.
- F. K. Adjei, Y. A. Osei, N. Kuntworbe and K. Ofori-Kwakye, J. Pharm., 2017, 2017, 2326912 Search PubMed.
- J. Li and Y. Wu, Lubricants, 2014, 2, 21–43 CrossRef.
- P. G. Surdi de Castro,
et al.
, Sci. Rep., 2021, 11, 900 CrossRef CAS PubMed.
- J. B. M. Fernandes,
et al.
, An. Acad. Bras. Cienc., 2019, 91, e20180094 CrossRef PubMed.
- R. B. Shah, M. A. Tawakkul and M. A. Khan, AAPS PharmSciTech, 2008, 9, 250–258 CrossRef CAS PubMed.
- Md. M. Hasan, S. Rana and S. S. Chowdhury, Bangladesh Pharm. J., 2015, 17, 128–134 CrossRef.
- L. L. Augsburger and R. F. Shangraw, J. Pharm. Sci., 1966, 55, 418–423 CrossRef CAS PubMed.
- B. S. Agboola, T. O. Ajala and O. A. Odeku, Starch/Staerke, 2018, 70, 1700047 CrossRef.
- O. Adeoye and G. Alebiowu, Pharm. Dev. Technol., 2014, 19, 901–910 CrossRef CAS PubMed.
- J. Chitedze, M. Monjerezi, J. D. K. Saka and J. Steenkamp, J. Appl. Pharm. Sci., 2012, 2, 31–37 CrossRef.
- H. P. Goh, P. W. S. Heng and C. V. Liew, Int. J. Pharm., 2018, 547, 133–141 CrossRef CAS PubMed.
- N. Nagpal, P. Kaur, R. Kumar, S. Rahar, R. Dhawan and M. Arora, Bull. Pharm. Res., 2016, 6, 45–49 CrossRef CAS.
- S. G. Late and A. K. Banga, AAPS PharmSciTech, 2010, 11, 1627–1635 CrossRef CAS PubMed.
- P. M. Lestari, A. Widayanti and H. Afifah, Open Access Maced. J. Med. Sci., 2019, 7, 3827–3832 CrossRef PubMed.
- M. Landín, M. González, C. Souto, A. Concheiro, J. L. Gómez-Amoza and R. Martínez-Pacheco, Drug Dev. Ind. Pharm., 1993, 19, 1211–1220 CrossRef.
- Y. Wicaksono and N. Syifa, Indones. J. Pharm., 2008, 19, 165–171 CAS.
- C. E. Bos, G. K. Bolhuis, H. Van Doorne and C. F. Lerk, Pharm. Weekbl., 1987, 9, 274–282 CAS.
- V. C. Orsi, M. M. D. C. Vila, V. M. Hanai-Yoshida, M. V. Chaud, V. M. Balcão and J. M. De Oliveira, Ars Pharm., 2019, 60, 205–211 CrossRef CAS.
- R. S. Ayu, K. Abdan, H. A. Saffian, K. Zaman, M. Jawaid and C. H. Lee, Polymers, 2018, 10, 1187 CrossRef PubMed.
- H. J. Prado, M. C. Matulewicz, P. R. Bonelli and A. L. Cukierman, Carbohydr. Res., 2009, 344, 1325–1331 CrossRef CAS PubMed.
- R. C. Mundargi, N. B. Shelke, A. P. Rokhade, S. A. Patil and T. M. Aminabhavi, Carbohydr. Polym., 2008, 71, 42–53 CrossRef CAS.
- C.-L. Lin, J.-H. Lin, J.-J. Lin and Y. Chang, Molecules, 2020, 25, 5528 CrossRef CAS PubMed.
- Y. Zhang,
et al.
, Starch/Staerke, 2013, 65, 461–468 CrossRef CAS.
- A. Getachew, Z. Yilma and S. Abrha, Adv. Pharmacol. Sci., 2020, 2020, 2708063 CAS.
- A. Golachowski, W. Dróżdż, M. Golachowska, M. Kapelko-Żeberska and B. Raszewski, Foods, 2020, 9, 1311 CrossRef CAS PubMed.
- M. Labelle, P. Ispas-Szabo and M. A. Mateescu, Starch/Staerke, 2020, 72, 2000002 CrossRef CAS.
- Q. Chen,
et al.
, RSC Adv., 2015, 5, 67459–67474 RSC.
- A. O. Ashogbon and E. T. Akintayo, Starch-Starke, 2014, 66, 41–57 CrossRef CAS.
- S. Park, Y. Na, J.-W. Kim, S. D. Kang and K.-H. Park, Food Sci. Biotechnol., 2017, 27, 299–312 Search PubMed.
- K. H. Hebelstrup, D. Sagnelli and A. Blennow, Front. Plant Sci., 2015, 6, 247 CrossRef PubMed.
- K. Bashir and M. Aggarwal, J. Food Sci. Technol., 2019, 56, 513–523 CrossRef PubMed.
- S. Lefnaoui and N. Moulai-Mostefa, Saudi Pharm. J., 2015, 23, 698–711 CrossRef PubMed.
- A. V. Singh and L. K. Nath, Saudi Pharm. J., 2013, 21, 193–200 CrossRef PubMed.
- T. Nabais and G. Leclair, ISRN Pharm., 2014, 2014, 391523 Search PubMed.
- T. C. Le and M. A. Mateescu, Drug Delivery Transl. Res., 2017, 7, 516–528 CrossRef CAS PubMed.
- A. B. Ahmed and I. Bhaduri, Int. J. Pharm. Pharm. Sci., 2017, 9, 132–137 CrossRef.
- M. J. Santander-Ortega,
et al.
, J. Controlled Release, 2010, 141, 85–92 CrossRef CAS PubMed.
- J. Yang, Y. Huang, C. Gao, M. Liu and X. Zhang, Colloids Surf., B, 2014, 115, 368–376 CrossRef CAS PubMed.
- G. Sodeifian, M. A. Nooshabadi, F. Razmimanesh and A. Tabibzadeh, Arabian J. Chem., 2023, 16, 105196 CrossRef CAS.
- H. Nateghi, G. Sodeifian, F. Razmimanesh and J. M. N. Abad, Sci. Rep., 2023, 13, 12906 CrossRef CAS PubMed.
- G. Sodeifian, H. Bagheri, M. A. Nooshabadi, F. Razmimanesh and A. Roshanghias, J. Supercrit. Fluids, 2023, 200, 106000 CrossRef CAS.
- G. Sodeifian, C. Garlapati, M. A. Nooshabadi, F. Razmimanesh and A. Tabibzadeh, Sci. Rep., 2023, 13, 8112 CrossRef CAS PubMed.
- G. Sodeifian, L. Nasri, F. Razmimanesh and M. A. Nooshabadi, J. Chem. Thermodyn., 2023, 182, 107050 CrossRef CAS.
- M. Abadian, G. Sodeifian, F. Razmimanesh and S. Z. Mahmoudabadi, Fluid Phase Equilib., 2023, 567, 113711 CrossRef CAS.
- G. Sodeifian, C.-T. Hsieh, A. Tabibzadeh, H.-C. Wang and M. A. Nooshabadi, Sci. Rep., 2023, 13, 2172 CrossRef CAS PubMed.
- G. Sodeifian, M. M. B. Usefi, F. Razmimanesh and A. Roshanghias, Arabian J. Chem., 2023, 16, 104421 CrossRef CAS.
- G. Sodeifian, C. Garlapati and A. Roshanghias, Sci. Rep., 2022, 12, 17494 CrossRef CAS PubMed.
- G. Sodeifian, R. S. Alwi, F. Razmimanesh and F. Sodeifian, J. Mol. Liq., 2022, 362, 119689 CrossRef CAS.
- G. Sodeifian, R. S. Alwi, F. Razmimanesh and A. Roshanghias, J. Supercrit. Fluids, 2022, 190, 105759 CrossRef CAS.
- G. Sodeifian, C.-T. Hsieh, R. Derakhsheshpour, Y. Chen and F. Razmimanesh, Arabian J. Chem., 2022, 15, 103876 CrossRef CAS.
- G. Sodeifian, C. Garlapati, F. Razmimanesh and H. Nateghi, Sci. Rep., 2022, 12, 9008 CrossRef CAS PubMed.
- G. Sodeifian, C. Garlapati, F. Razmimanesh and H. Nateghi, Sci. Rep., 2022, 12, 7758 CrossRef CAS PubMed.
- G. Sodeifian, L. Nasri, F. Razmimanesh and M. Abadian, J. CO2 Util., 2022, 58, 101931 CrossRef CAS.
- G. Sodeifian, R. S. Alwi and F. Razmimanesh, Fluid Phase Equilib., 2022, 556, 113396 CrossRef CAS.
- G. Sodeifian, R. S. Alwi, F. Razmimanesh and M. Abadian, J. Mol. Liq., 2022, 346, 117899 CrossRef CAS.
- G. Sodeifian, C. Garlapati, F. Razmimanesh and M. Ghanaat-Ghamsari, Sci. Rep., 2021, 11, 24344 CrossRef CAS PubMed.
- G. Sodeifian, C. Garlapati, F. Razmimanesh and F. Sodeifian, J. Mol. Liq., 2021, 335, 116446 CrossRef CAS.
- G. Sodeifian, R. S. Alwi, F. Razmimanesh and K. Tamura, Fluid Phase Equilib., 2021, 537, 113003 CrossRef CAS.
- G. Sodeifian, L. Nasri, F. Razmimanesh and M. Abadian, J. Mol. Liq., 2021, 331, 115745 CrossRef CAS.
- G. Sodeifian, S. M. Hazaveie and F. Sodeifian, J. Mol. Liq., 2021, 330, 115695 CrossRef CAS.
- G. Sodeifian, S. A. Sajadian, F. Razmimanesh and S. M. Hazaveie, Sci. Rep., 2021, 11, 7546 CrossRef CAS PubMed.
- G. Sodeifian, C. Garlapati, F. Razmimanesh and F. Sodeifian, J. Chem. Eng. Data, 2021, 66, 1119–1131 CrossRef CAS.
- G. Sodeifian, N. S. Ardestani, F. Razmimanesh and S. A. Sajadian, Fluid Phase Equilib., 2021, 522, 112745 CrossRef.
- S. M. Hazaveie, G. Sodeifian and S. A. Sajadian, J. Supercrit. Fluids, 2020, 163, 104875 CrossRef CAS.
- G. Sodeifian, C. Garlapati, S. M. Hazaveie and F. Sodeifian, J. Chem. Eng. Data, 2020, 65, 4406–4416 CrossRef CAS.
- G. Sodeifian, N. S. Ardestani, M. R. Golmohammadi and A. Fazlali, J. Chem. Eng. Data, 2020, 65, 1747–1760 CrossRef CAS.
- G. Sodeifian, S. A. Sajadian and R. Derakhsheshpour, Fluid Phase Equilib., 2020, 507, 112422 CrossRef CAS.
- G. Sodeifian, F. Razmimanesh, N. S. Ardestani and S. A. Sajadian, J. Mol. Liq., 2020, 229, 112179 CrossRef.
- G. Sodeifian, F. Razmimanesh, S. A. Sajadian and S. M. Hazaveie, J. Chem. Thermodyn., 2020, 142, 105998 CrossRef CAS.
- G. Sodeifian and F. Razmimanesh, J. Mol. Liq., 2020, 297, 111740 CrossRef CAS.
- G. Sodeifian, R. Detakhsheshpour and S. A. Sajadian, J. Supercrit. Fluids, 2019, 154, 104606 CrossRef CAS.
- G. Sodeifian, S. M. Hazaveie, S. A. Sajadian and N. S. Ardestani, J. Chem. Eng. Data, 2019, 64, 5338–5348 CrossRef CAS.
- G. Sodeifian, S. M. Hazaveie, S. A. Sajadian and F. Razmimanesh, Fluid Phase Equilib., 2019, 493, 160–173 CrossRef CAS.
- G. Sodeifian and S. A. Sajadian, J. Supercrit. Fluids, 2019, 149, 79–87 CrossRef CAS.
- G. Sodeifian and F. Razmimanesh, J. Supercrit. Fluids, 2019, 146, 89–99 CrossRef CAS.
- G. Sodeifian, F. Razmimanesh and H. S. Panah, Fluid Phase Equilib., 2018, 472, 147–159 CrossRef CAS.
- G. Sodeifian, J. Supercrit. Fluids, 2018, 133, 239–252 CrossRef CAS.
- G. Sodeifian, N. S. Ardestani and H. S. Panah, Fluid Phase Equilib., 2018, 458, 102–114 CrossRef CAS.
- G. Sodeifian and F. Razmimanesh, Fluid Phase Equilib., 2017, 450, 149–159 CrossRef CAS.
- G. Sodeifian and N. S. Ardestani, J. Supercrit. Fluids, 2017, 128, 102–111 CrossRef CAS.
- S. T. Tene, J. M. Klang, S. C. N. Houketchang, G. T. Boungo and H. M. Womeni, Food Sci. Nutr., 2019, 7, 1190–1206 CrossRef PubMed.
- A. R. Rajan and T. E. Abraham, Bioprocess Biosyst. Eng., 2006, 29, 65–71 CrossRef CAS PubMed.
-
E. C. Ibezim, in Biopolymers in Drug Delivery: Recent Advances and Challenges, ed. M. U. Adikwu and C. O. Esimone, Bentham Science Publishers, United Arab Emirates, 1st Ed, 2009, ch. 4, pp. 39–56 Search PubMed.
- T. Itthisoponkul, J. R. Mitchell, A. Taylor and I. A. Farhat, Carbohydr. Polym., 2007, 69, 106–115 CrossRef CAS.
- A. Madene, M. Jacquot, J. Scher and S. Desobry, Int. J. Food Sci. Technol., 2006, 41, 1–21 CrossRef CAS.
- Md. K. Saifullah, M. R. I. Shishir, R. Ferdowsi, R. T. Rahman and Q. V. Vuong, Trends Food Sci. Technol., 2019, 86, 230–251 CrossRef CAS.
- C. Zhao, Y. Cao, Y. Zhang and Y. Qiao, Int. J. Mol. Sci., 2019, 20, 752 CrossRef PubMed.
- S. Surini, V. Anggriani and A. Effionora, J. Med. Sci., 2009, 9, 249–256 CrossRef CAS.
- O. A. Odeku, A. A. Aderogba, T. O. Ajala, O. D. Akin-Ajani and A. Okunlola, J. Pharm. Invest., 2017, 47, 445–451 CrossRef CAS.
- A. G. B. Pereira, A. R. Fajardo, S. R. Nocchi, C. V. Nakamura, A. F. Rubira and E. C. Muniz, Carbohydr. Polym., 2013, 98, 711–720 CrossRef CAS PubMed.
- V. Raj and G. Prabha, J. Assoc. Arab Univ. Basic Appl. Sci., 2016, 21, 10–16 Search PubMed.
- C. Isimi and A. D. C. C. Rodrigues, J. Nanomed. Nanotechnol., 2016, 7, 1–8 Search PubMed.
- S. Mohanty, J. Adv. Nanobiotechnol., 2017, 1, 16–24 CrossRef.
- D. P. Mohanty and S. K. B. Al, Int. J. Pharm. Biol. Sci., 2017, 8, 5–11 CAS.
- G. L. P. Da Silva, L. C. De Assunção Morais, J. B. Olivato, J. Marini and P. C. Ferrari, J. Biomater. Appl., 2021, 35, 1096–1108 CrossRef CAS PubMed.
- Z. Kou,
et al.
, Int. J. Biol. Macromol., 2020, 145, 655–662 CrossRef CAS PubMed.
- S. Surini, F. Gotalia and K. S. S. Putri, Int. J. Appl. Pharm., 2018, 10, 225–229 CrossRef CAS.
- T. Gabriel, A. Belete and T. Gebre-Mariam, J. Drug Delivery Ther., 2013, 3, 1–10 Search PubMed.
- M. Palencia, A. L. Tulio and M. C. Enrique, Curr. Chem. Biol., 2017, 11, 28–35 CrossRef CAS.
- G. K. Athira and A. N. Jyothi, J. Pharm. Sci. Res., 2015, 6, 200–211 Search PubMed.
- G. N. A. D. Putra, R. Murwanti, A. Rohman and T. N. S. Sulaiman, Int. J. Appl. Pharm., 2019, 11, 32–40 CrossRef.
- C. Liu, J. Li, K. Li, C. Xie and J. Liu, Int. J. Biol. Macromol., 2020, 156, 1045–1052 CrossRef CAS PubMed.
- E. Anwar, H. Khotimah and A. Yanuar, J. Med. Sci., 2006, 6, 923–929 CrossRef CAS.
- A. Zaki, E. Anwa and S. Surini, Int. J. Appl. Pharm., 2017, 9, 71–73 CrossRef CAS PubMed.
- A. O. Shittu, A. R. Oyi, A. B. Isah, S. Kareem and M. Ibrahim, Int. J. Pharm. Sci. Res., 2012, 3, 2180–2190 CAS.
- E. H. Nabeshima and M. V. E. Grossmann, Carbohydr. Polym., 2001, 45, 347–353 CrossRef CAS.
- L. Z. Jaekel, M. Schmiele, R. Da Silva Rodrigues and Y. K. Chang, J. Food Sci. Technol., 2015, 52, 7305–7312 CrossRef CAS.
- K. Oltramari,
et al.
, Starch/Staerke, 2017, 69, 11–12 Search PubMed.
- R. Wongsagon, S. Shobsngob and S. Varavinit, Starch/Staerke, 2005, 57, 166–172 CrossRef CAS.
- O. Adewoyin, Br. J. Pharm. Res., 2015, 8, 1–7 Search PubMed.
- B. A. Harsojuwono, S. T. Mulyani and I. W. Arnata, J. Appl. Hortic., 2019, 21, 13–19 CrossRef.
- A. N. Jyothi, S. N. Moorthy and K. N. Rajasekharan, Starch/Staerke, 2006, 58, 292–299 CrossRef CAS.
- R. M. Collares,
et al.
, J. Zhejiang Univ., Sci., B, 2012, 13, 579–586 CrossRef CAS PubMed.
- M. Iwe, G. O. Okereke and A. An, Agrotechnol, 2014, 4, 1–6 Search PubMed.
- H. J. J. K.-V. Putten,
et al.
, Transgenic Res., 2012, 21, 39–50 CrossRef PubMed.
- F. Hosseini and S. Ansari, J. Food Sci. Technol., 2019, 56, 5374–5385 CrossRef CAS PubMed.
- P. Mhaske, Z. Wang, A. Farahnaky, S. Kasapis and M. Majzoobi, Crit. Rev. Food Sci. Nutr., 2021, 8, 1–27 Search PubMed.
- D. De Souza Fernandes, M. Leonel, M. S. Del, M. M. Mischan, E. A. Garcia and T. P. R. D. Santos, J. Food Sci. Technol., 2017, 54, 1357–1367 CrossRef PubMed.
- R. Wongsagonsup,
et al.
, Carbohydr. Polym., 2014, 101, 656–665 CrossRef CAS PubMed.
- M. Y. Naz, S. A. Sulaiman, B. Ariwahjoedi and K. Z. K. Shaari, Sci. World J., 2014, 2014, 375206 CAS.
- W. Li, Z. Xu, Z. Wang and J. Xing, Polymers, 2018, 10, 1110 CrossRef PubMed.
- S. Wang, F. Zhang, F. Chen and Z. Pang, Bioresources, 2013, 8, 3574–3589 Search PubMed.
- Z. Wang,
et al.
, Int. J. Biol. Macromol., 2019, 126, 603–611 CrossRef CAS PubMed.
- W. Murdianto, S. Anggrahini, S. Sutardi and Y. Pranoto, Pak. J. Nutr., 2019, 18, 471–478 CrossRef CAS.
- L. Ekebafe, Afr. J. Environ. Sci. Technol., 2012, 6, 275–282 CAS.
- S. Jobling, Curr. Opin. Plant Biol., 2004, 7, 210–218 CrossRef CAS PubMed.
- R. N. Tharanathan, Crit. Rev. Food. Sci. Nutr., 2005, 45, 371–384 CrossRef CAS PubMed.
- V. D. Trela, A. L. Ramallo and O. A. Albani, Braz. Arch. Biol. Technol., 2020, 63, 1–13 Search PubMed.
- L. H. Garrido, E. Schnitzler, M. E. B. Zortéa, T. De Souza Rocha and I. M. Demiate, J. Food Sci. Technol., 2014, 51, 2640–2647 CrossRef CAS PubMed.
- K. Heebthong and K. Ruttarattanamongkol, Starch/Staerke, 2016, 68, 528–540 CrossRef CAS.
- A. G. G. Issola, A. N. Kamlo, A. M. C. Yona and M. K. Ndikontar, J. Renewable Mater., 2018, 6, 642–650 CAS.
- H. Qiu and L. He, Polym. Adv. Technol., 1999, 10, 468–472 CrossRef CAS.
- L. P. Cordoba, Braz. J. Therm. Anal., 2013, 2, 6–11 CrossRef.
- C. S. Schmitz, K. N. De Simas, K. M. O. D. Santos, J. J. João, R. D. De Mello Castanho Amboni and E. R. Amante, Int. J. Food Sci. Technol., 2006, 41, 681–687 CrossRef CAS.
- G. Pifferi and P. Restani, Farmaco, 2003, 58, 541–550 CrossRef CAS PubMed.
- H. P. Til, V. J. Feron, H. R. Immel and W. F. Vogel, Food Chem. Toxicol., 1986, 24, 825–834 CrossRef CAS PubMed.
- O. B. Wurzburg, Nutr. Rev., 1986, 44, 74–79 CrossRef CAS PubMed.
- E. Chinedu, D. Arome and F. S. Ameh, Toxicol. Int., 2013, 20, 224–226 CrossRef CAS PubMed.
- E. Walum, Environ. Health Perspect., 1998, 106, 497–503 CAS.
- J. E. LeBeau, Regul. Toxicol. Pharmacol., 1983, 3, 71–74 CrossRef CAS PubMed.
- P. J. Vit, Arch. Toxicol., 1989, 63, 343–344 CrossRef CAS PubMed.
- M. Porwal, N. A. Khan and K. Maheshwari, Sci. Pharm., 2017, 85, 29 CrossRef PubMed.
- E. O. Erhirhie, C. P. Ihekwereme and E. E. Ilodigwe, Interdiscip. Toxicol., 2018, 11, 5–12 CrossRef PubMed.
- R. L. Lipnick,
et al.
, Food Chem. Toxicol., 1995, 33, 223–231 CrossRef CAS PubMed.
- D. R. Jesus, T. B. L. Prando, D. C. Dragunski, F. M. Gasparotto, E. L. B. Lourenço and A. Gasparotto Jr., Lat. Am. J. Pharm., 2015, 34, 1154–1161 CAS.
- OECD Guideline for the Testing of Chemicals, Repeated dose 90-day oral toxicity study in rodents, 2018, section 4, Test No. 408.
- S. Parasuraman, J. Pharmacol. Pharmacother., 2011, 2, 74–79 CrossRef CAS PubMed.
- D. R. Jesus,
et al.
, J. Evidence-Based Complementary Altern. Med., 2015, 390416 Search PubMed.
- OECD Guideline for the Testing of Chemicals, Chronic toxicity studies, 2018, section 4, Test No. 452.
- R. Zepeda, M. Candiracci, N. Lobos, S. Lux and H. F. Miranda, Mar. Drugs, 2014, 12, 5055–5071 CrossRef CAS PubMed.
-
J. B. Colerangle, in A Comprehensive Guide to Toxicology in Nonclinical Drug Development, ed. A. S. Faqi, Academic Press, London, 2nd edn, 2017, ch. 25, pp. 659–683 Search PubMed.
- R. Corvi,
et al.
, Toxicol. In Vitro, 2017, 45, 278–286 CrossRef CAS PubMed.
- OECD Guideline for the Testing of Chemicals, Carcinogenicity studies., 2018, section 4, Test No. 451.
- G. Jena, C. L. Kaul and P. Ramarao, Indian J. Pharmacol., 2005, 37, 209–222 CrossRef.
-
A. S. Faqi and J. S. Yan, in A Comprehensive Guide to Toxicology in Nonclinical Drug Development, ed. A. S. Faqi, Academic Press, London, 2nd edn, 2017, ch. 30, pp. 813–823 Search PubMed.
- K.-M. Wu, J. Dou, H. Ghantous, S. Chen, A. Bigger and D. Birnkrant, Regul. Toxicol. Pharmacol., 2010, 56, 1–3 CrossRef PubMed.
- H. Türkez, M. Arslan and Ö. Özdemir, Expert Opin. Drug Metab. Toxicol., 2017, 13, 1089–1098 CrossRef PubMed.
- OECD Guideline for the Testing of Chemicals, In vitro mammalian cell micronucleus test., 2016, section 4, Test No. 487.
- A. Mortensen,
et al.
, EFSA J., 2017, 15, e04911 Search PubMed.
- B. Medeiros-Neves, M. C. Nemitz, F. N. S. Fachel and H. F. Teixeira, Recent Pat. Drug Delivery Formulation, 2019, 13, 192–202 CrossRef CAS PubMed.
- H. Jj, D. P. Es, L. Mirjam, M. Margherita, R. H. Michael and H. Michal, J. Clin. Transl. Res., 2017, 3, 250–259 Search PubMed.
- J. L. Tidwell and L. A. Liotta, Methods Mol. Biol., 2012, 823, 391–408 CrossRef CAS PubMed.
- H. Wang, T. Feng, H. Zhuang, Z. Xu, R. Ye and M. Sun, Recent Pat. Food Nutr. Agric., 2018, 9, 23–30 CrossRef CAS PubMed.
- S. Li, Y. Cui, Y. Zhou, Z. Luo, J. Liu and M. Zhao, J. Sci. Food Agric., 2017, 97, 2282–2290 CrossRef CAS PubMed.
-
G. Yuanyuan, et al., Patent China, 2022, CN114190507, A Search PubMed.
-
X. Xingyong, X. Yuanyuan, H. Yaocong, S. Qiaoqiao, L. Songyang and M. Chen, Patent China, 2022, CN114950355, A Search PubMed.
-
H. Douglas, et al., Patent Australia, 2022, AU2022209338A1 Search PubMed.
-
Y. Kailiang, Patent China, 2022, CN217490061U Search PubMed.
-
L. Rihui, S. Yonggui, Y. Xianchao and J. Siyu, Patent China, 2021, CN113577307A Search PubMed.
-
H. Lijie, et al., Patent China, 2021, CN113831563A Search PubMed.
-
L. Yefei, et al., Patent China, 2021, CN112514751A Search PubMed.
-
S. Shaozhen, H. Lijie, H. Xiaoxue, S. Shaoxin and L. Ping, Patent China, 2021, CN113150393A Search PubMed.
-
L. Bo, Patent China, 2020, CN111205373, A Search PubMed.
-
L. Rihui, F. Yanye, Z. Lihong, Z. Yijun and Y. Xianchao, Patent China, 2020, CN112321853A Search PubMed.
-
L. Yilun and L. Xiangdong, Patent China, 2020, CN111808205A Search PubMed.
-
O. Wenjun, et al., Patent China, 2020, CN111543476A Search PubMed.
-
C. Hui, Patent China, 2019, CN109276550A Search PubMed.
-
L. Heping, et al., Patent China, 2019, CN109280187A Search PubMed.
-
L. Mingchang, L. Jingli and Z. Huangqiang, Patent China, 2019, CN109400726A Search PubMed.
-
S. Xiaodan, S. Ancheng and J. Xiachao, Patent China, 2019, CN109957035A Search PubMed.
-
L. Changying, Patent China, 2018, CN107814880A Search PubMed.
-
M. Xianli, L. Fangyao and L. Hanfu, Patent China, 2018, CN107603513A Search PubMed.
-
L. Heping, et al., Patent China, 2018, CN107722533A Search PubMed.
-
P. L. Ooi, V. Malayalam, A. Guha, A. Dasgupta and S. D. Prabhakar, Patent World Intellectual
Property Organization, 2018, WO2017025482A1.
-
S. F. David, R. V. T. Jazmin and T. E. Michael, Patent Japan, 2017, JP2017061543A Search PubMed.
-
Y. Xiulian, Y. Qinghong, W. Miaomiao, Z. Xuejuan and Z. Xinghai, Patent China, 2017, CN106727394A Search PubMed.
-
C. Li, Patent China, 2017, CN106389345A Search PubMed.
-
Z. Ren, D. Luo and F. Wang, Patent United State, 2017, US20200377675A1 Search PubMed.
|
This journal is © The Royal Society of Chemistry 2024 |
Click here to see how this site uses Cookies. View our privacy policy here.