DOI:
10.1039/D3NR04097F
(Paper)
Nanoscale, 2024,
16, 343-359
Nanobody-loaded nanobubbles targeting the G250 antigen with ultrasound/photoacoustic/fluorescence multimodal imaging capabilities for specifically enhanced imaging of RCC†
Received
15th August 2023
, Accepted 18th November 2023
First published on 20th November 2023
Abstract
Clinicians have attempted to discover a noninvasive, easy-to-perform, and accurate method to distinguish benign and malignant renal masses. The targeted nanobubbles (NBs) we constructed that target the specific membrane antigen of renal cell carcinoma (RCC), G250, and contain indocyanine green (ICG) provide multimodal enhanced imaging capability in ultrasound/photoacoustic/fluorescence for RCC which may possibly solve this problem. In this study, we encapsulated ICG in the lipid shell of the NBs by mechanical oscillation, then anti-G250 nanobodies (AGN) were coupled to the surfaces by the biotin–streptavidin bridge method, and the nanobubble named AGN/ICG-NB was completely constructed. The average particle diameter of the prepared AGN/ICG-NBs was (427.2 ± 4.50) nm, and the zeta potential was (−13.33 ± 1.01) mV. Immunofluorescence and flow cytometry confirmed the specific binding capability of AGN/ICG-NBs to G250-positive cells. In vitro imaging experiments confirmed the multimodal imaging capability of AGN/ICG-NBs, and the in vivo imaging experiments demonstrated the specifically enhanced ability of AGN/ICG-NBs for ultrasound/photoacoustic/fluorescence imaging of human-derived RCC tumors. The biosafety of AGN/ICG-NB was verified by CCK-8 assay, organ H&E staining and blood biochemical indices. In conclusion, the targeted nanobubbles we prepared with ultrasound/photoacoustic/fluorescence multimodal imaging capabilities provide a potentially feasible approach to address the need for early diagnosis and differential diagnosis of renal masses.
1. Introduction
RCC is a common disease of the urinary system and accounts for approximately 85% of renal masses (RMs). With the improvement of detection methods and the strengthening of public awareness of regular physical examination, 60% of patients are detected without symptoms through noninvasive imaging methods.1,2 Early diagnosis and treatment can greatly improve the prognosis of patients. Currently, the conventional imaging methods used to detect RMs include computed tomography (CT), ultrasound imaging (US) and magnetic resonance imaging (MRI). However, these conventional imaging modalities sometimes cannot clearly distinguish tumors from benign lesions and adjacent normal tissues surrounding renal tumors.3,4 Therefore, it is necessary to explore a new imaging diagnostic technique for the differential diagnosis of renal tumors.
As a noninvasive technology for assessing the molecular and cellular phenotypes of diseases in human or animal models, molecular imaging (MI) has the advantage of visualizing molecular motion and continuously tracking molecular transport without interfering with the metabolic function of the organism. At present, MI mainly includes radionuclide imaging (RI), MRI, US, optical imaging (OI), photoacoustic imaging (PAI), etc., and the combination of two or more of the above imaging methods is called multimodal imaging.5 In previous studies, we successfully developed a type of targeted nanobubble (NB), a nanoscale ultrasound contrast agent (UCA), targeting the G250 antigen of RCC. Its ability to specifically aggregate in renal cancer tissues achieves satisfactory imaging in contrast-enhanced ultrasound (CEUS), which has been demonstrated by experiments on nude mice.6 In addition, due to the advantages of particle size (446 nm) compared with the microscale UCA now used in clinical practice, NBs possess the capacity to penetrate tumor blood vessels and enter the tissue space, which can not only prolong the retention time of UCA in the target area, but also provide potential for the material to be used as a targeting carrier of chemotherapy drugs. Although NBs recognise RCC in US, the images are not intuitive enough, and quantitative software analysis is needed to clarify the nature of the mass. Therefore, exploring other imaging methods on the basis of CEUS will have greater value for the diagnosis and differential diagnosis of RCC.
Indocyanine green (ICG), a water-soluble cyanine dye with few side effects in humans, was approved by the U.S. Food and Drug Administration as an optical developer for clinical use in 1995. In the diagnosis and characterization of cancer, ICG is capable of photoacoustic/near-infrared fluorescence dual-mode imaging.7,8 PAI is a hybrid noninvasive imaging technique that receives the thermoelastic expansion caused by photons in tissues as well as pressure waves, and uses the low scattering of tissues to achieve high-resolution anatomical and functional imaging.9,10 PAI is relatively intuitive, which can help distinguish whether there is abnormal tissue with the naked eye. When combined with US, PAI provides a complementary contrast mechanism to provide more comprehensive tissue information, which is quite helpful for the diagnosis and differential diagnosis of tumors, and it is a valuable research work to carry out. Compared with fluorescence imaging, PAI has a higher optical imaging contrast and the ability to penetrate deep tissues. When ICG is injected intravenously, it combines with proteins in the vessels to form an ICG–protein complex, which can be excited by near-infrared (NIR) light with a wavelength of 780 nm and emit NIR light with a wavelength of 840 nm, which makes ICG applicable to in vivo NIR fluorescence imaging.7 However, free ICG has defects such as fluorescence quenching, fast metabolism and poor tumor targeting in vivo. The shortcomings of direct intravenous ICG injection can be overcome by introducing nanoparticles as ICG carriers.
Patient-derived tumor xenografts (PDXs) were established by transplanting tumor tissue from patients into immunodeficient mice.11 The PDX model can accurately represent the biological characteristics and heterogeneity of cancers, and its ability to simulate the tumor microenvironment is satisfactory. Conventional cell line tumor models have limitations in scientific research such as clinical drug development. Studies have confirmed that long-term in vitro cell culture shows altered tumor characteristics caused by epigenetic and genetic changes, thus affecting its predictive value as an experimental model. For these reasons, the PDX model is gaining increasing acceptance in the field of cancer research.12 The PDX model retains most histological and genetic features of its donor tumors and remains stable during passage. It has been demonstrated to have the capacity to predict clinical outcomes or as a research strategy for preclinical drug evaluation, biomarker identification, biological studies, and personalized medicine.13,14 We obtained clear cell renal carcinoma (ccRCC) tissue samples from the metastasized lesion on the left kidney of a patient in our hospital. The tumor tissue was surgically implanted under the renal capsule of NOD/SCID/IL2λ-receptor null (NPG) mice and an orthotopic PDX model was established.
The G250 antigen is a transmembrane protein that is induced by hypoxia. It belongs to the alpha carbonic anhydrase (CA) family of zinc metalloenzymes, and is also known as CA IX.15 In normal tissues, G250 is only expressed in the gastrointestinal tract. However, abnormal expression has been found in a number of tumors, including kidney, lung, head and neck, cervical, ovarian, esophageal, and breast cancers.16,17 Overexpression of G250 is related to the signaling pathways that are activated during tumorigenesis. It is involved in reversible catalysis of the hydration of carbon dioxide, which results in the accumulation of extracellular protons and, helps in building the acidic microenvironment of tumor cells. In addition, G250 mediates a variety of adaptations of tumor cells, including angiogenesis, metabolic alterations, tumor heterogeneity, and drug resistance, as well as the regulation of cancer-specific chemokines.18,19 G250 is highly expressed in RCC, but is unexpressed in normal renal tissue or in benign renal masses, which makes it a potential target site for specific diagnosis/treatment of RCC.15,20 In previous studies, we constructed a camel variable domain of heavy chain (VHH) immune library and screened the anti-G250 nanobodies (AGNs) that could specifically bind to the extracellular region of the G250 protein. Then, we connected AGNs to NBs as targeted groups to construct a UCA that could specifically enhance imaging. Compared with the UCA connected with the G250 monoclonal antibodies, the UCA connected with AGNs has a smaller molecular weight and stronger tissue-penetration ability, and its enhancement effect on the US of RCC has been confirmed in previous studies.6
Based on our previous research, we intended to prepare a nanobubble, AGN/ICG-NB, with ultrasound/photoacoustic/fluorescence multimodal imaging function by encapsulating ICG into the lipid shell of NBs and connecting AGNs to its surface. Its physicochemical properties, biotoxicity, biological characteristics and ultrasound/photoacoustic/fluorescence imaging ability for PDX renal carcinoma were studied in vitro and in vivo (Scheme 1). This novel multimodal contrast agent may provide a new method for the differential diagnosis of RM in clinical practice.
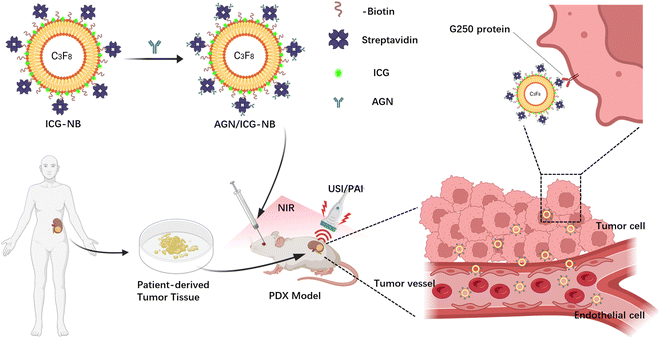 |
| Scheme 1 Schematic illustration of the preparation of targeted multi-imaging nanobubbles (AGN/ICG-NBs) specifically bound to G250(+) RCC cells and enhancing the ultrasound/photoacoustic/fluorescence imaging of PDX-RCC. Abbreviations: C3F8, perfluoropropane; ICG, indocyanine green; AGN, anti-G250 nanobody; USI, ultrasound imaging; PAI, photoacoustic imaging; NIR, near infrared ray; PDX, patient-derived xenograft. | |
2. Materials and methods
2.1 Cell culture and construction of orthotopic PDX models
The human renal clear cell carcinoma cell line 786-O (G250+) (iCell Bioscience Inc., Shanghai) and human renal adenocarcinoma cell lines ACHN (G250−) (Procell Life Sci & Tech Co., Wuhan) were cultured in RPMI-1640 (Vivacell, Shanghai) containing 10% fetal bovine serum (FBS) (Gibco Co., USA), in a 5% CO2 incubator at 37 °C. Cells were dissociated in the logarithmic growth phase with trypsin EDTA solution C (Vivacell, Shanghai) for cell passage or cell experiments.
All procedures involving human participants were in accordance with the ethical standards of the institutional research committee and the 1964 Helsinki Declaration and its later amendments, or comparable ethical standards. Written informed consent was obtained from each patient. All samples were collected with approval from the Research Ethics Committee of Daping Hospital, Army Medical University. The animal experiments were conducted according to the NC3Rs ARRIVE guidelines and were approved by the Institutional Animal Care and Use Committee of the Army Medical University. All of the animals were raised according to the animal welfare guidelines of the Army Medical University.
NOD.Cg-Prkdcscid Il2rgtm1Vst/Vst (NPG) mice, the immunodeficient mice, were used to construct PDX models. After anesthetization with high-flow isoflurane, 4–5-week-old mice were transferred to a sterile operating table for fixation under low-flow maintenance gas anesthesia. Under sterile conditions, the skin was opened by a 5–7 mm flank incision along the dorsal midline. After the subcutaneous and muscle tissue were dissected, a small incision of the body wall was then made to push out the left kidney and secured by applying pressure. Ophthalmic forceps and ophthalmic scissors were used to tear the kidney capsule. The RCC tissue was cut into samples 1 mm3 in size, and the tissue fragments were inserted into the renal capsule and fixed on the surface of the kidney to obtain blood supply. After the kidney was gently returned into the peritoneal space, the body wall incision was closed using a 5/0 absorbable suture, and the mice were warmed to wake up. Xenograft growth was observed weekly by ultrasound. After approximately 4 weeks, the tumors grew to an experimental size. The mice were sacrificed, and the tumors were removed, embedded in wax and cut into slices. H&E staining and immunohistochemistry of renal carcinoma markers were performed to identify the tumor nature. The same operation was repeated to continue the transplantation of the xenografts and immunohistochemistry tests were performed regularly to ensure the stability of the obtained xenografts.
2.2 Preparation of AGN/ICG-NBs
1,2-Dipalmitoyl-sn-glycero-3-phosphoglycerol (DPPG), 1,2-dipalmitoyl-sn-glycero-3-phosphatidyletha-nolamine (DPPE), 1,2-dipalmitoyl-sn-glycero-3-phos-phocholine (DPPC), 1,2-dipalmitoyl-glycero-3-phosphate (DPPA) (Corden Pharma, Switzerland), 1,2-distearoyl-sn-glycero-3-phosphoethanol-amine-PEG-2000-biotin (DSPE-PEG2000-Biotin) (NANOCS, USA) and indocyanine green (MedChemExpress, USA) were dissolved at 3
:
3
:
3
:
1
:
1
:
0.5 mass ratio in a mixed solution containing phosphate-buffered saline (PBS) and glycerol (9
:
1). Air in the vial was replaced with perfluoropropane (C3F8, Tianjin Nuclear Industry Institute of Physics and Chemistry, Tianjin, China). Thereafter, the vial was placed in a HL-YMY 10 tissue grinder (Zhongrun Medical Instrument Co. Ltd, Hangzhou) and horizontally mechanically shaken (3600 rpm, 90 s). The obtained liquid was placed at 4 °C for 1.5 h to separate the undissolved lipid and microbubbles from NBs. Then, the middle layer of a light green milky suspension in the vial was obtained, which was the ICG-NBs. Finally, AGNs were connected to ICG-NBs by the biotin–streptavidin bridging method (streptavidin purchased from Solarbio Biotechnology Co., Ltd, Beijing). Targeted nanobubbles named AGN/ICG-NBs were obtained.
2.3 Characterization of AGN/ICG-NBs
The AGN/ICG-NBs were diluted to a proper concentration with PBS and added dropwise onto a hemocytometer, which was then placed under an inverted light microscope (Dmi8, Leica, Germany) to observe their morphology and calculate the concentration using ImageJ software. The particle sizes, size distributions, and zeta potentials of the NBs were measured using a Zetasizer Nano ZS90 particle size analyzer (Malvern Instruments Inc., UK). The NBs were observed and measured daily over a period of one week to evaluate changes in the concentration and particle diameter with time. The morphology of AGN/ICG-NBs was also observed under a transmission electron microscope (JEOL, Japan), and the core particle size of NBs was measured on the TEM images using ImageJ software.
ICG methanol solutions with different concentrations of ICG were prepared and placed in a multifunctional spectrometer (Molecular Devices, SpectraMax iD3, Shanghai) to determine the absorption spectrum at an excitation wavelength of 600–900 nm. The absorbance values of each concentration at the optimal wavelength were collected to make an ICG concentration–absorbance standard curve. A microplate reader was used to measure the absorbance at 780 nm of unencapsulated ICG in the supernatant. The mass of the entrapped ICG was equal to the mass of the total ICG minus the non-entrapped mass. The encapsulation efficiency (EE) and drug loading efficiency (LE) of ICG were calculated using the following formulas: EE = (amount of ICG entrapped in NBs/total amount of added ICG) × 100%; LE = (amount of ICG entrapped in NBs/total lipid used to prepare NBs) × 100%. This process was repeated three times to obtain the average values. Pure ICG, ICG-NB, and AGN/ICG-NB methanol solutions with the same concentration of ICG were prepared and placed on a multifunctional spectrometer to obtain the absorption spectra of the three groups of solutions and to determine whether the encapsulation of ICG in the lipid layer of NBs changed the optical properties of ICG.
2.4 Cytotoxicity of AGN/ICG-NBs on RCC cells
786-O and ACHN cells at the logarithmic growth stage were implanted in 96-well plates at a concentration of 5 × 103 cells per well. The prepared AGN/ICG-NBs were sterilized using a bacterial filter with a pore size of 0.22 μl and then diluted to concentrations of 1.0 × 107 mL−1, 5.0 × 107 mL−1, 1.0 × 108 mL−1, 5.0 × 108 mL−1, and 1.0 × 109 mL−1. Different concentrations of solutions were added to the plates and cultured with cells for 48 h (4 repeated wells for each concentration). Three washes with PBS, 100 μl of fresh medium without FBS and 10 μl of CCK-8 reagent (MedChemExpress, USA) were added to each well. After the reaction at 37 °C for 50 min, the 96-well plate was placed in a multifunctional spectrometer to determine the OD values at 450 nm. The cell survival rate at each concentration was calculated by the formula: cell viability(%) = [(experimental group OD − blank group OD)/(control group − blank group OD)] × 100%.
2.5 Identification of connection between AGN and ICG-NBs
A fluorescence experiment was used to identify whether AGNs were connected to the surface of the ICG-NBs. 1′-Octadecyl-3,3,3′,3′-tetramethylphthalocyanine perchlorate (Dil) (Beyotime Biotechnology, Shanghai), a dye, was used to label the lipid shells of ICG-NBs. Then the FITC-labeled AGN was connected to ICG-NBs by the biotin–streptavidin bridging method. The obtained suspension was diluted to a proper concentration with PBS and added dropwise on a slice. Under an inverted fluorescence microscope (Leica DMi8, Leica, Germany), the NBs were observed.
2.6
In vitro cell assay to detect the targeted binding ability of AGN/ICG-NBs
Fluorescence.
786-O and ACHN cells at the logarithmic growth stage were implanted in 6-well plates at a concentration of 1 × 106 cells per well. A cell slide piece was placed in each well. Both types of cells were divided into 3 groups: AGN/ICG-NBs, ICG-NBs and AGN/ICG-NBs + AGN blocked. The cells were fixed with 4% paraformaldehyde and blocked with 5% bovine serum albumin (BSA) (GEN-VIEW Scientific Inc., USA). Meanwhile, 200 μl of diluted AGN solution (2 mg ml−1) was added to the AGN/ICG-NB + AGN blocked group. DiO (Beyotime Biotechnology, Shanghai), a membrane dye, was added to stain for 30 min before each well was washed three times with PBS. AGN/ICG-NBs and ICG-NBs at a concentration of 1 × 107 ml−1 were added to the experimental groups, respectively. After the reaction for 2 h and staining with DAPI (Beyotime Biotechnology, Shanghai), the cell slides were taken out and observed under a confocal laser scanning microscope (CLSM, LSM 880, ZEISS, Germany).
Flow cytometry.
786-O and ACHN cells at the logarithmic growth stage were implanted in 6-well plates at a concentration of 1 × 106 cells per well. Both types of cells were divided into 3 groups: AGN/ICG-NBs, ICG-NBs, and PBS. One milliliter of fresh medium without FBS was added to the wells, and then 200 μl of AGN/ICG-NBs or ICG-NBs at a concentration of 1.0 × 107 ml−1, or PBS, was added to the wells, respectively. After the reaction at 37 °C for 1 h and 3 washes with PBS, the cells were dissociated and then resuspended with PBS. Finally, the affinity of NBs to the cells was measured by using a FACSCalibur flow cytometer (BD Biosciences, USA).
2.7
In vitro ultrasound/photoacoustic/fluorescence imaging of AGN/ICG-NBs
1% agarose solution was prepared and heated in a microwave oven for 3 min. EP tubes (1.5 ml) were inserted to form grooves before the liquid solidified. After gel solidification, the cavity model for in vitro imaging was prepared.
Ultrasound imaging.
AGN/ICG-NBs diluted at concentrations of 5.0 × 106 ml−1, 1.0 × 107 ml−1, 5.0 × 107 ml−1, 1.0 × 108 ml−1, 5.0 × 108 ml−1 and 1.0 × 109 ml−1 with PBS were transferred into the agarose cavity model. A Vevo 2100 small animal ultrasound imaging system (VisualSonics Inc., Canada) was used to acquire B-mode and contrast-enhanced mode images (frequency 18 MHz, gain 40 dB). The gray value of the images was calculated using ImageJ.
Photoacoustic imaging.
AGN/ICG-NBs diluted at concentrations of 5.0 × 106 ml−1, 1.0 × 107 ml−1, 5.0 × 107 ml−1, 1.0 × 108 ml−1, 5.0 × 108 ml−1 and 1.0 × 109 ml−1 with PBS were transferred into the agarose cavity model. A Vevo LAZR photoacoustic imaging system (Visual Sonics Inc., Toronto, Canada) was used to acquire B-mode and PA images. Firstly, AGN/ICG-NBs at a concentration of 1.0 × 109 ml−1 were placed in the 1% gel well model and the optimum photoacoustic excitation wavelength was determined by full wavelength scanning with the photoacoustic transducer. Then, PA images of AGN/ICG-NBs at each concentration gradient were acquired by imaging at the optimal excitation wavelength (wavelength 770 nm, frequency 21 MHz, gain 47 dB). The quantified PA signal intensity within the region of interest (ROI) of each image was then analyzed using Vevo LAZR software.
Fluorescence imaging.
ICG, ICG-NBs, and AGN/ICG-NBs solutions with equal ICG content, as well as ddH2O, were prepared and placed into the IVIS spectrum small animal in vivo imaging system (PerkinElmer, USA). The images were acquired under irradiation conditions of excitation wavelength of 780 nm and emission wavelength of 840 nm, and fluorescence intensities were quantified using IVIS software. AGN/ICG-NBs diluted at concentrations of 5.0 × 106 ml−1, 1.0 × 107 ml−1, 5.0 × 107 ml−1, 1.0 × 108 ml−1, 5.0 × 108 ml−1 and 1.0 × 109 ml−1 with PBS were placed into the IVIS spectrum small animal in vivo imaging system (PerkinElmer, USA). The images were acquired under irradiation conditions of exactly the same excitation/emission wavelengths, and fluorescence intensities were quantified using IVIS software.
2.8
In vivo ultrasound/photoacoustic/fluorescence imaging of AGN/ICG-NBs
Ultrasound imaging.
After hair removal, tumor-bearing mice were randomly collected and were anesthetized by isoflurane and fixed on the operating table in a prone position. The coupling agent was applied to the tumor-bearing kidney area. An MS250 high-frequency probe was used to observe the size of the tumor, and then the Doppler mode was adjusted to observe the blood supply in the tumor to evaluate the tumor imaging effect. Xenograft tumors with an appropriate growth size and abundant blood supply were selected to perform ultrasound imaging experiments. One hundred microliters of ICG-NBs diluted at a concentration of 1.0 × 109 ml−1 was injected intravenously into the mice through the venae canthus posterior. An MS250 high-frequency probe with contrast-enhanced mode (frequency 18 MHz, gain 40 dB) was used to dynamically observe the xenograft tumor and the kidney. After approximately 30 min, the enhanced signal completely dissipated in the ROI, and the mice were injected with AGN/ICG-NBs at the same concentration. The images were acquired by using a Vevo 2100 small animal ultrasound imaging system. A total of three mice performed the above steps. The intensity (dB value) of the collected images was analyzed by using Vevo 2100 onboard software, and a time–intensity curve was drawn to calculate the area under the curve (AUC).
Photoacoustic imaging.
After hair removal, tumor-bearing mice were randomly collected and were anesthetized by isoflurane and fixed on the operating table in a prone position. The coupling agent was applied to the tumor-bearing kidney area. An MS250 high-frequency probe was used to observe the size of the tumor, and then the Doppler mode was adjusted to observe the blood supply in the tumor to evaluate the tumor imaging effect. Xenograft tumors with an appropriate growth size and abundant blood supply were selected to perform photoacoustic imaging experiments. One hundred microliters of ICG-NBs diluted at a concentration of 1.0 × 109 ml−1 was injected intravenously into the mice through the venae canthus posterior. The probe (wavelength 770 nm, frequency 21 MHz, gain 47 dB) was used to dynamically observe the xenograft tumor and the kidney. After the PA signal completely dissipated in the ROI, the mice were injected with AGN/ICG-NBs at the same concentration. The images were acquired by using a Vevo LAZR photoacoustic imaging system. A total of three mice performed the above steps. The PA intensity was analyzed by using Vevo LAZR software, and a time–intensity curve was drawn to calculate the area under the curve (AUC).
Fluorescence imaging.
After hair removal, tumor-bearing mice were anesthetized by isoflurane and fixed on the operating table in a prone position. A total of six mice were divided into two groups, with three mice per group. One hundred microliters of ICG-NBs or AGN/ICG-NBs diluted at a concentration of 1.0 × 109 ml−1 was injected intravenously into the mice through the venae canthus posterior. Images were collected at different time periods by using the IVIS spectrum small animal in vivo imaging system. Two hours after injection, the two groups of mice were sacrificed, and their kidneys were removed and placed in the imaging system for image collection. The enrichment of the two types of NBs in tumors and healthy kidneys was compared between the two groups. All images were acquired under irradiation conditions of excitation wavelength of 780 nm and emission wavelength of 840 nm, and the fluorescence intensities of all acquired images were quantitively analyzed using IVIS software.
2.9 Distribution of AGN/ICG-NBs in tumor tissues
Dil labeled AGN/ICG-NBs and ICG-NBs diluted to a concentration of 1.0 × 109 ml−1 were injected intravenously into the two groups of mice, which were sacrificed 2 h after injection. The xenograft tumors and thigh muscle were fixed and embedded in paraffin sections. Then, the tissue blocks were cut to 2 mm thickness and adhered to slides. After heating at 65 °C for 2 h, the slices were deparaffinized and rehydrated in xylene and ethanol solutions with different concentration gradients. Heat-induced antigen retrieval was performed with EDTA solution for 15 min and then the sections were cooled to room temperature. After permeabilized with 0.2% Triton X-100 (Biosharp Life Science, Hefei) solution for 30 min and blocked with 5% BSA for 1 h, 50 ml of rabbit anti-mouse CD31 monoclonal antibody solution (1
:
2000) (Abcam, UK) was added, and the slices were incubated at 4 °C overnight. After 3 rinses, 50 μl of FITC-labeled goat anti-rabbit secondary antibody solution (1
:
200) (Beyotime Biotechnology, Shanghai) was added and the slices were incubated in the dark at 37 °C for 2 h. Finally, mounting medium with DAPI was added to stain the cell nuclei, and the slices were placed under CLSM to observe the distribution of the two types of NBs in the xenograft tumor and muscle.
2.10 Detection of biosafety
H&E staining of majority organs.
One hundred microliters of AGN/ICG-NBs diluted at a concentration of 1.0 × 109 ml−1 was injected intravenously into mice through the venae canthus posterior. The mice were sacrificed 2 weeks after injection, and the livers, hearts, kidneys, lungs, spleens and thigh muscle were fixed and embedded in paraffin sections, which were then cut into 2 mm thick slices and were H&E stained. The stained slices were observed and images were collected using an optical microscope (Olympus, Japan).
Determination of blood biochemical and blood routine indices.
Nine mice were divided into 3 groups and injected with 100 μl of AGN/ICG-NBs diluted to a concentration of 1.0 × 109 ml−1 or PBS. In the AGN/ICG-NB groups, blood extractions of eyeballs were performed 1 week and 2 weeks after the injection, while in the PBS group, the operation was performed 2 weeks after the injection. The blood samples were measured and analyzed by a hematology analyzer.
2.11 Statistical analysis
One-way ANOVA and paired t tests were performed by using the R programming language, and data were expressed as mean ± standard deviation. P < 0.05 was considered to be significant for the statistical analysis, and lines and histograms were drawn using GraphPad Prism 8.0.2.
3. Results
3.1 Verification of the PDX model
The tumor sample used to construct the PDX models was taken from a male patient on March 25, 2021. The patient was first diagnosed with right RCC in November 2014, and underwent radical resection of right kidney carcinoma (Furman grade I., clinical grade cT2aN0MO). Subsequently he received 2.5 years of targeted therapy from July 2018 to December 2020. In January 2021, resection of the left lung metastasis was carried out (pathological results: RCC metastasis, WHO/ISUP grade 4). On March 25, 2021, he was treated with nephron-sparing left kidney metastatic carcinoma resection (pathological result: RCC with focal necrosis, WHO/ISUP grade 4). The tumor tissue in which we established the PDX model was taken from this left kidney metastasis.
The appearance of PDX was observed (Fig. 1A and B). The transplanted tumor protrusively grew out of the kidney. The tumor's color was pale yellow, with an outer envelope and a soft jelly like tumor tissue inside with occasional clastic necrotic tissue. The tumor was wax-embedded and then cut into slices. The results of H&E staining (Fig. 1C) showed that tumor cells were dominated by clear cytoplasm, arranged in a mixture of solid, trabecular and acinar patterns, with a fragile network of thin-walled blood vessels interspersed within the tissue, which is consistent with the morphological characteristics of RCC. The results of immunohistochemical staining of biomarkers of RCC (Fig. 1D–F): G250(+), CD10(+), and Pax8(+).
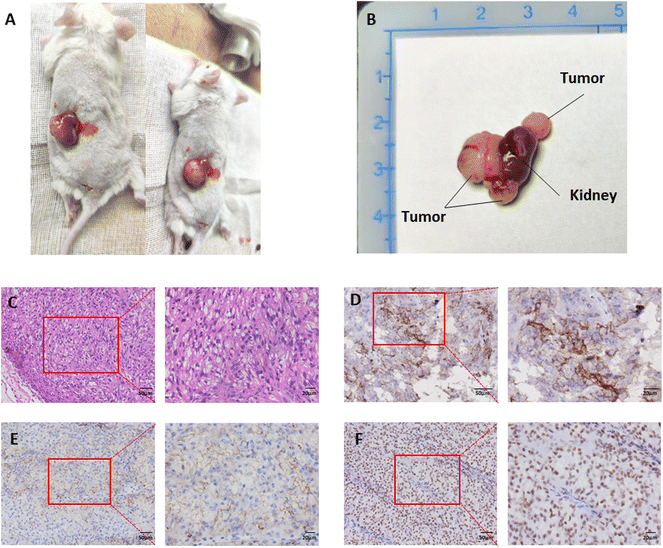 |
| Fig. 1 Macroscopic and histological identification of PDX tumors. (A) Orthotopic PDX tumors grew on the kidneys of NPG mice. (B) The tumor-bearing kidney. (C) H&E staining image of PDX tumors. (D–F) IHC staining images of G250, CD10 and Pax 8. | |
3.2 Preparation and characterization of AGN/ICG-NBs
The appearance of the prepared blank-NBs, ICG-NBs, and AGN/ICG-NBs was observed (Fig. 2A). Blank-NBs were milky white, opaque emulsions while ICG-NBs and AGN/ICG-NBs were both light green, opaque emulsions with no obvious difference in appearance. Under an optical microscope (eyepiece ×10, objective ×63, oil lens), AGN/ICG-NBs exhibited uniform size and a regular shape with good dispersion (Fig. 2B). Under a transmission electron microscope, the above conclusions are verified again (Fig. 2C). The concentration of AGN/ICG-NBs was approximately (8.57 ± 0.97) × 109 ml−1 (Fig. 2D). Stored at 4 °C, the concentration of the AGN/ICG-NB solution showed a statistically significant decline on day 4 compared with day 0 (P < 0.05), indicating that AGN/ICG-NB solution had a certain stability. The particle sizes of AGN/ICG-NBs and ICG-NBs were measured and found to be 411.5 ± 5.97 nm and 427.2 ± 4.50 nm, and the core particle sizes of the two types of NBs were measured and found to be 407.6 ± 3.26 nm and 399.1 ± 5.25 nm. The zeta potentials of the two types of NBs were measured and found to be −14.30 ± 1.25 mV and −13.33 ± 1.01 mV, with polydispersity indices (PDIs) of 0.21 ± 0.01 and 0.23 ± 0.01, respectively (Table 1). The particle size dispersion of the two types of NBs is shown in Fig. 2E. The particle sizes of the AGN/ICG-NBs and ICG-NBs were dynamically observed for one week (Fig. 2F). The results showed that the particle sizes of the two types of NBs gradually decreased with time, and a statistically significant decline appeared on day 4 compared with day 0 (P < 0.05). The time consists of the point at which the concentration changes. The absorption spectra of ICG solutions with different concentrations were measured. The results showed that the absorption peak of each concentration was at the excitation wavelength of 780 nm (Fig. S1A†), indicating that the optimal excitation wavelength of ICG did not shift due to the change in concentration. The fitted curve was solved with the absorption value of each concentration at 780 nm, which turns out to be y = 0.2703x − 0.0515, R2 = 0.9899 (Fig. S1B†). Absorbance increases with the ICG concentration. In addition, via calculations, the encapsulation efficiency of ICG in AGN/ICG-NBs was found to be 81.5 ± 0.94%, and the drug loading efficiency 5.0 ± 0.31 μg mg−1. The absorption spectra of pure ICG, ICG-NBs and AGN/ICG-NBs solutions with equal amounts of ICG (0.01 mg ml−1) were measured, and the results showed that the optimal excitation wavelengths of the three groups of solutions are the same at 780 nm (Fig. 2G). The results indicated that ICG encapsulated with nanoparticles did not change its excitation wavelength. In addition, the peak absorption value of ICG-NBs or AGN/ICG-NBs was significantly higher than that of pure ICG solution. What we believed was related to the fact that ICG encapsulated with NBs was more stable than the free ICG dissolved in solution, which decreased the occurrence of ICG self-quenching.
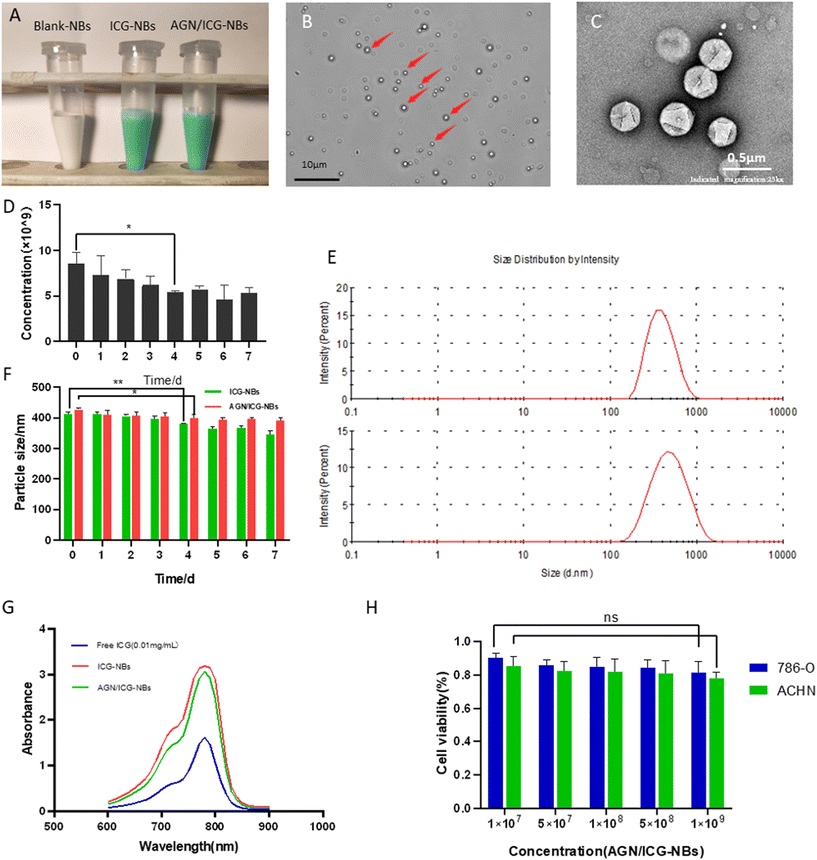 |
| Fig. 2 Basic characteristics of the AGN/ICG-NBs. (A) The appearance of blank-NBs, ICG-NBs and AGN/ICG-NBs. (B) Morphology of the AGN/ICG-NBs (red arrows) as viewed by optical microscopy. (C) Morphology of the AGN/ICG-NBs as viewed by transmission electron microscopy. (D) Histogram showing the time dependence of changes in the concentration of the AGN/ICG-NBs. © Particle size distribution of ICG-NBs and AGN/ICG-NBs. (F) Histogram showing the time dependence of changes in the particle sizes of AGN/ICG-NBs and ICG-NBs. (G) Absorption spectra of free ICG solution, ICG-NBs and AGN/ICG-NBs. (H) Cytotoxicity of ACP/ICG-NBs on 786-O and ACHN cells detected by CCK-8 assay. | |
Table 1 Particle sizes and zeta potentials of the two types of nanobubbles
|
AGN/ICG-NB |
ICG-NB |
Hydrodynamic size |
(427.2 ± 4.50) nm |
(411.5 ± 5.97) nm |
Core size |
(403.2 ± 3.26) nm |
(399.1 ± 6.25) nm |
Zeta potential |
(−13.33 ± 1.01) mV |
(−14.30 ± 1.25) mV |
The cytotoxicity of AGN/ICG-NBs to 786-O and ACHN cells was evaluated with a CCK-8 assay, and a histogram of cell viability was plotted (Fig. 2H). The results showed that there was no statistically significant difference (P > 0.05) between the two groups when the minimum concentration of AGN/ICG-NBs was 1 × 107 ml−1 and the maximum concentration was 1 × 109 ml−1. The cell viabilities of 786-O were 90.3% ± 2.45% and 81.5% ± 5.77%, respectively, and the cell viabilities of ACHN were 85.3% ± 4.81% and 78.0% ± 3.40%, respectively. The results indicated that AGN/ICG-NBs showed low toxicity to cells.
Verification of the coupling of AGN to NBs was achieved by immunofluorescence. Under optical microscopy, the dispersion of AGN/ICG-NBs can be seen under the light field (Fig. 3A). The lipid membranes of NBs were labeled with Dil, which showed red fluorescence (Fig. 3B), and the AGNs labeled with FITC showed green fluorescence (Fig. 3C). The perfect overlap of the red and green fluorescence indicates that the AGNs were coupled to the surfaces of the NBs (Fig. 3D).
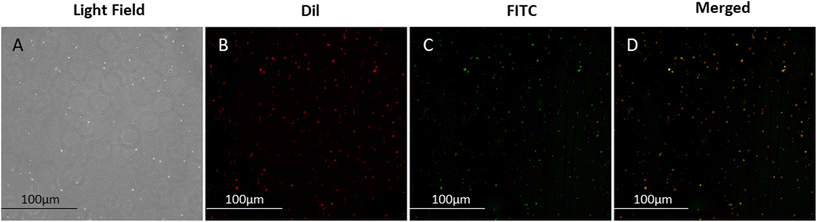 |
| Fig. 3 Verification of the coupling of nanobodies to NBs by immunofluorescence. Under optical microscopy, (A) the dispersion of AGN/ICG-NBs can be seen under the light field, (B) Dil labeled lipid membranes showed red fluorescence, (C) FITC labeled AGN showed green fluorescence, and (D) the overlap of the two types of fluorescence indicates that the AGNs were ligated to the surfaces of the ICG-NBs. | |
3.3 Specific targeting and binding ability of AGN/ICG NBs to RCC cells
A cellular immunofluorescence experiment was performed and the aggregation of AGN/ICG-NBs around tumor cells was observed to detect their specific targeting ability. The red fluorescence intensity of NBs around 786-O cells of the AGN/ICG-NB group was significantly higher than that of the ICG-NB or AGN/ICG-NBs + AGN blocked (Fig. 4A) groups, while the red fluorescence intensity around ACHN cells had no significant difference between the AGN/ICG-NB and ICG-NB (Fig. 4B) groups. The red fluorescence intensity of each group was quantitatively analyzed, and the results were consistent with the above results (Fig. S2†). The results of fluorescence experiments verified the specific targeting and binding ability of AGN/ICG-NBs to G250(+) cells.
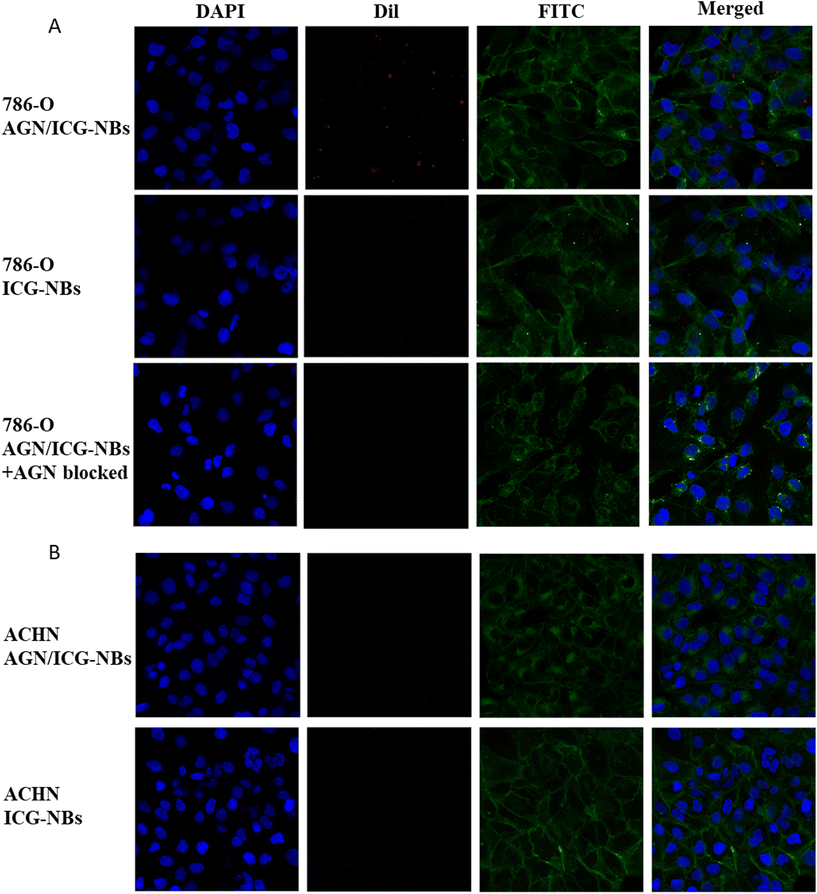 |
| Fig. 4 Binding capacity of AGN/ICG-NBs and ICG-NBs to 786-O (A) and ACHN (B) tumor cells. Under CLSM, the nuclei of the cells appeared blue, the NBs appeared red, and the cell membrane appeared green. In 786-O cells, aggregation of AGN/ICG-NBs was observed around the cells, while no obvious aggregation of ICG-NBs was found. In addition, no obvious aggregation of AGN/ICG-NBs could be found around the cells that were pretreated with AGN. In ACHN cells, no aggregation of AGN/ICG-NBs or ICG-NBs was observed around the cells. | |
Flow cytometry was performed to verify the targeting and binding ability of AGN/ICG-NBs. The results showed that the binding rate of 786-O cells with AGN/ICG-NBs (43.73% ± 1.96%) was significantly higher than that with ICG-NBs (17.83% ± 0.54%) or with PBS (18.03% ± 1.61%), while no significant difference was found in the three groups of data conducted on ACHN cells. The data were 14.77% ± 1.06%, 13.5% ± 0.78% and 12.63% ± 1.01%, respectively (Fig. 5A and B).
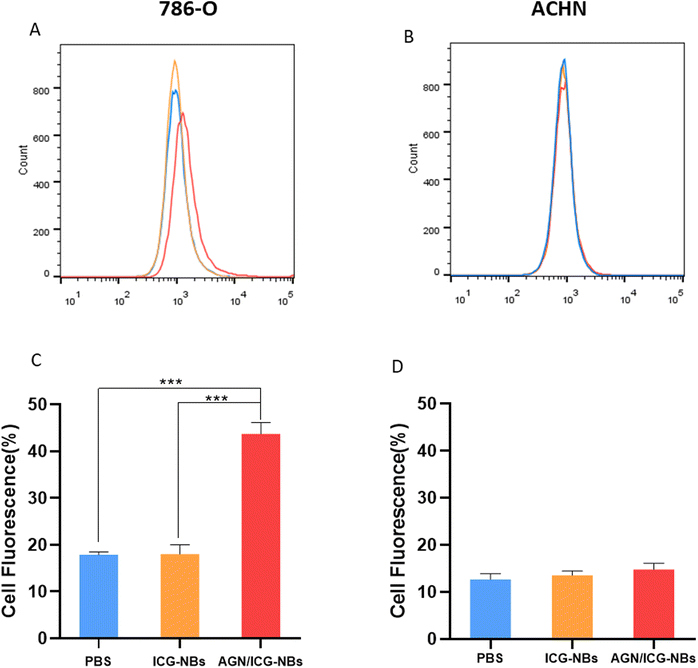 |
| Fig. 5 Flow cytometry identifies the binding capacity of AGN/ICG-NBs and ICG-NBs to 2 kinds of kidney cancer cells. (A) 786-O cells bound AGN/ICG-NBs significantly more than ICG-NBs. (B) ACHN cells bound a few AGN/ICG-NBs or ICG-NBs, and no significant difference was observed. (C and D) Quantification of the affinities of the two types of NBs to the two types of tumor cells (*** indicates P < 0.001). | |
3.4
In vitro ultrasound/photoacoustic/fluorescence imaging of AGN/ICG-NBs
In vitro images of AGN/ICG-NB solutions with different concentrations were acquired and collected by using a Vevo 2100 small animal ultrasound imaging system, Vevo LAZR photoacoustic imaging system and IVIS spectrum small animal imaging system, respectively. The intensities of the images were analyzed by using the corresponding software (Fig. 6A). In the set concentration gradient range, the average gray value of the CEUS image increased with increasing concentrations of AGN/ICG-NBs, and the highest value approached 194.0 ± 3.10 dB (Fig. 6B). In addition, we found that photoacoustic and fluorescence imaging did not follow the above rule. Both imaging methods produced the highest intensity values at concentrations of 1.0 × 108 ml−1, which were 0.53 ± 0.03 a.u and 6.19 ± 0.37 p s−1 cm−2 sr−1/μW cm−2, respectively (Fig. 6C and D). The results indicate that ICG has its optimal concentration in photoacoustic imaging and fluorescence imaging.
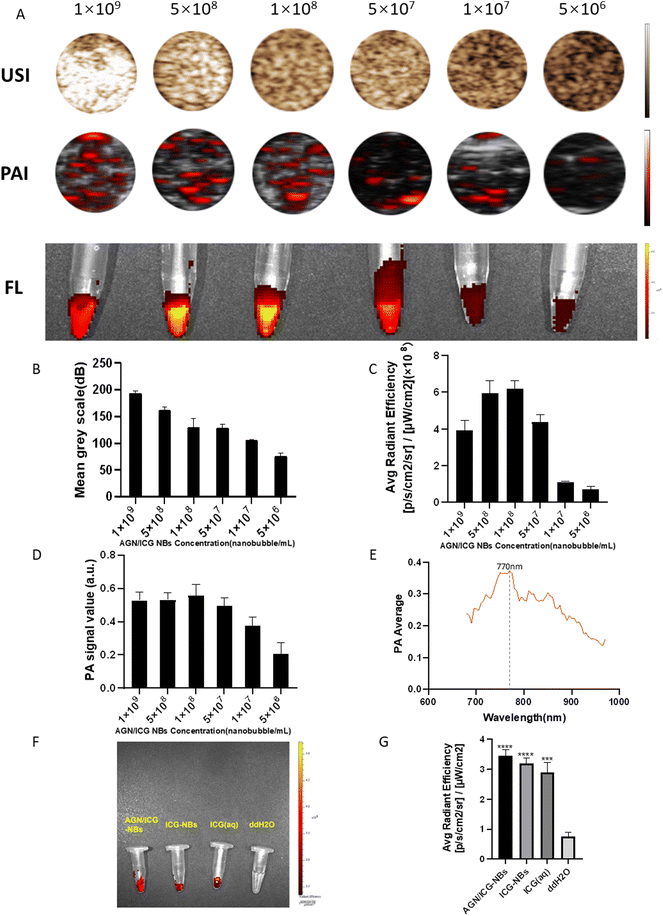 |
| Fig. 6 (A) In vitro ultrasound, photoacoustic and fluorescence imaging of AGN/ICG-NBs at different concentrations. (B–D) Quantification of USI, FL and PAI signal intensities at different AGN/ICG NB concentrations. (E) Full wavelength excited AGN/ICG-NBs, and the optimal excitation was found (770 nm). (F) Fluorescence imaging of AGN/ICG-NBs, ICG-NBs, ICG solution and ddH2O in vitro. (G) Quantification of the fluorescence signal intensity of AGN/ICG-NBs, ICG-NBs, ICG solution and ddH2O in vitro (*** indicates P < 0.001). | |
AGN/ICG-NBs were scanned by a full wavelength by using a laser photoacoustic producer, and the optimal excitation wavelength was shown to be 770 nm (Fig. 6E), which was used for subsequent photoacoustic imaging. Fluorescence imaging of AGN/ICG-NBs, ICG-NBs, ICG solution with an equal ICG content and ddH2O as the blank control was performed (Fig. 6F). The results showed that there was no significant difference in the imaging effect and the fluorescence intensity between the AGN/ICG-NBs, ICG-NBs or ICG solution, indicating that ICG combined with NBs would not affect the fluorescence imaging effect.
3.5
In vivo ultrasound/photoacoustic/fluorescence imaging of AGN/ICG-NBs
Photoacoustic imaging.
B-mode images of PDX tumors and tumor-bearing kidneys before injection of NBs were collected, and continuous dynamic PA images of PDX tumors and the tumor-bearing kidney were acquired within 20 min after injection of AGN/ICG-NBs or ICG-NBs. Both the xenograft tumor and the kidney area are indicated by dotted lines (Fig. 7D). Images showed that the PA signal in the ROI appeared at 5 min after injection, and then reached its peak at approximately 15 min after injection. Thereafter, the signal began to weaken. The intensity of the PA signal produced by AGN/ICG-NBs in tumors was obviously stronger than that produced by ICG-NBs, which can be demonstrated intuitively by plotting the time–intensity curves and calculating the AUC (Fig. 7E and F) (P < 0.05). The results above indicated that AGN/ICG-NBs could significantly enhance the PA imaging of RCC compared with ICG-NBs.
Fluorescence imaging.
Fluorescence imaging was performed on both groups of tumor-bearing mice before injection: there was hardly any fluorescence signal on the mice. After injections of AGN/ICG-NBs and ICG-NBs, continuous dynamic fluorescence images were acquired within 90 min (Fig. 8A). As shown in the images, the contrast agent was relatively evenly distributed throughout the body of the mice at the beginning of the injection. At 15 min after injection, the fluorescence signals gathered in the blood-enriched organs, such as the liver and heart (yellow rectangle marked). From 30 min after injection, the contrast agent began to accumulate in the kidney area. The normal kidney areas of both groups showed strong fluorescence signals, while the signals at the tumor-bearing region (the red marked) showed a significant difference: the AGN/ICG-NB group could show a fluorescence signal in the marked region, while ICG-NBs could not. We stopped the dynamic observation at 90 min after injection, the mice were executed and both normal kidneys and tumor-bearing kidneys were taken and observed in the imaging instrument. The results showed that the fluorescence signal of the AGN/ICG-NB tumors was significantly stronger than that of ICG-NB tumors, while there was no significant difference in the fluorescence intensity of normal kidneys between the two groups. Quantitative analysis of fluorescence intensity confirmed the above conclusions (Fig. 8B and C) (P < 0.05).
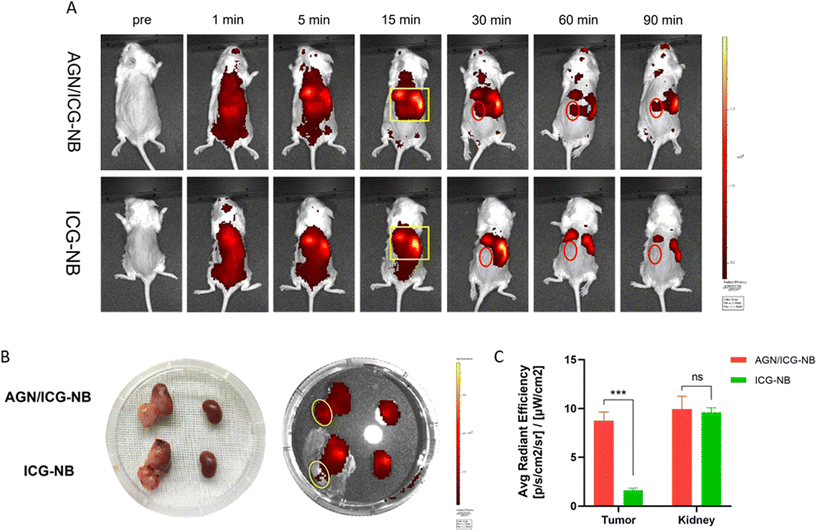 |
| Fig. 8
In vivo fluorescence imaging of AGN/ICG-NBs and ICG-NBs. (A) Fluorescence imaging of PDX tumors (n = 3) before and after injection of the two types of NBs (the yellow rectangle indicates the range of the liver and heart; the red circles indicate the region of the tumor-bearing kidney). (B) Fluorescence imaging of tumor-bearing kidneys and normal kidneys 1.5 h after injection of the two types of NBs (the yellow circles indicate tumors). (C) Quantification of fluorescence signal intensity of tumors and normal kidneys after injection for 1.5 h (n = 3) (*** indicates P < 0.001; ns indicates no significant difference). | |
3.6 Distribution of AGN/ICG-NBs in tumors
Immunofluorescence was performed to observe the distribution of NBs in tumors and other tissues. The NBs appeared red, the blood vessels of the tumor/muscle appeared green, and the nuclei appeared blue. A large amount of red fluorescence could be observed both inside and outside the vascular lumen in the xenograft tumor injected with AGN/ICG-NBs (Fig. 9A–D), while only a little red fluorescence could be seen in the vascular lumen in the tumor injected with ICG-NBs (Fig. 9E–H). The results indicated that both types of NBs penetrated the tumor vascular barrier by their own particle size, but the AGN/ICG-NBs aggregated by binding to the surface of cells more than the ICG-NBs, thus achieving a higher concentration and longer duration of enrichment. On the other hand, no red fluorescence was observed in normal muscle tissue (Fig. 9I–L), indicating that the NBs had some difficulty penetrating normal blood vessel walls, which provided their specificity for tumor imaging.
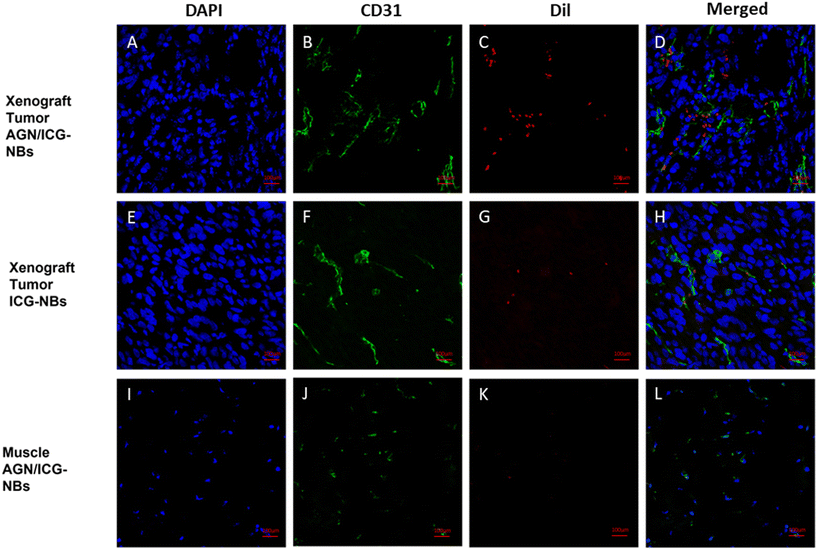 |
| Fig. 9 Localization of AGN/ICG-NBs and ICG-NBs in PDX tumor tissues by immunohistofluorescence. The nuclei of the tumor cells appeared blue (DAPI), the blood vessels of the tumor appeared green (CD31-FITC), and the NBs appeared red (Dil). (A–D) A large number of AGN/ICG-NBs were observed in the intercellular space of PDX tumors. (E–H) Few ICG-NB was observed in the intercellular space. (I–L) Few AGN/ICG-NB was observed in the tissue except PDX tumors, such as muscle. | |
3.7 Biosafety evaluation
An ideal contrast agent should be nontoxic or have low toxicity. We verified by CCK-8 assay that AGN/ICG-NB has no obvious toxicity to cells at a certain concentration. Therefore, the effects of AGN/ICG-NBs on major organs and liver and kidney functions of mice were verified by H&E staining and blood biochemical indices, to study whether there was potential toxicity. H&E staining images of major organs in the AGN/ICG-NB group showed no obvious injury or inflammation compared with the PBS control group (Fig. S3A†). Therefore, the blood biochemical indices (ALT/AST/UREA/CREA), as well as the major indices of blood routine (RBC/WBC/PLT/HGB), showed no significant difference within groups 1 week and 2 weeks after injection of AGN/ICG-NBs and the PBS control group (Fig. S3B and C†). The lower WBC counts were due to the absence of T/B cells and the loss of NK cell activity in NPG mice as immunodeficient mice. Other indicators were normal. These results indicated that AGN/ICG-NBs had certain biosafety and could be used as a relatively safe contrast agent.
4. Discussion
Approximately 400
000 cases of RCC are diagnosed worldwide each year, resulting in 175
000 deaths. Approximately 35% of patients initially develop advanced or metastatic RCC (mRCC), and 30% relapse even after treatment.21 For diseases with a high metastasis rate, which seriously endanger patients’ lives, searching for a diagnostic method that can specifically identify renal malignant tumors at an early stage has become one of the current research focus areas. The ICG-encapsulated targeting NB in this study is a meaningful and valuable exploration for the differential diagnosis and early diagnosis of RCC.
Ultrasonic molecular imaging technology relies on contrast agents with specific targeting ligands, selectively enriched at the target location, to play a role in strengthening the imaging effect for certain diseases. Compared with CT/MRI, ultrasonic molecular imaging has the advantages of being real-time, noninvasive and easy to operate.22 In our previous works, we constructed UCAs from NBs conjugated with monoclonal antibodies, ligands and nanobodies to achieve targeted imaging, and the results showed that NBs with targeting function showed advantages such as imaging intensity, action duration time and AUC compared with those without targeting function,6,23,24 which proves that the strategy works.25 However, single ultrasonic molecular imaging has some shortcomings: single imaging effect, poor differentiation of benign/malignant tumors, and the need for quantitative software to analyze the images. While the information provided by a single imaging method is limited, multimodal imaging can provide more comprehensive information and support for definite diagnosis.26,27 PAI is based on NIR pulsed laser irradiation, and the structure absorbs photons and feedback sound waves to form an image. While the image formation and the factors that affect the spatial resolution of PAI and US are essentially the same, the contrast of the resulting images is fundamentally different: the US image describes the morphological characteristics of the tissue, while the PA image represents the initial pressure distribution generated by the absorption of the laser pulse.28 In practice, PAI and US are usually integrated as a whole, which provides the feasibility of “single platform multimodal imaging”.29 As a safe and reliable bio dye with NIR fluorescence/PAI functions, ICG has been clinically applied in tumor localization and characterization.8,30,31 In this study, we used ICG as a marker group to pair with previously developed NBs with G250 targeted binding ability, and constructed a multimodal molecular imaging contrast agent for RCC enrichment imaging. Through complementary imaging of US/PAI, we can compensate for the defect that single US is not intuitive in judging benign/malignant RM. At the same time, the metabolism of ICG in vivo was studied by using the fluorescence tracer ICG and whole-body imaging of experimental animals. Many studies have confirmed that the construction of ICG nanoparticles is a good approach to make up for some of its shortcomings. These shortcomings include aggregation in solution, quenching and clearance in circulation, limited stability, and poor specificity against tumors.32,33 Following this generally accepted idea, we prepared AGN/ICG-NBs.
In this study, antigen G250 was selected as the target protein because of its good specificity for renal cancer: more than 85% of primary and metastatic renal cancers express G250 antigen, while almost all clear cell renal carcinomas, which account for more than 90% of renal cancers, express the G250 antigen. On the other hand, normal renal tissues do not.34 A multicenter clinical study by Wang et al. suggested that G250 expression was significantly associated with the TNM stage of primary RCC, which means that G250 was more likely to be highly expressed in early-stage tumors than in later-stage tumors.35 This means that the detection of G250 expression is more valuable for the diagnosis of small, early RCC. In other words, AGN/ICG-NBs can enhance the imaging effect of early RCC and contribute to reliable early diagnosis.
In addition, the in vivo tumor models in this study were all patient-derived xenografts. Compared with conventional cell line tumor models, PDX has more realistic environmental simulation, more stable feature retention, and more accurate clinical prediction,13,36 which provide us with more accurate and reliable data for the development of new contrast agents.
The AGN/ICG-NBs prepared in this study are stable and biosafe. Under confocal microscopy, the red fluorescence of the NB lipid membrane and the green fluorescence of AGN were overlapped well, indicating that the targeted NBs were successfully constructed. By comparing the absorption spectra with that of ICG pure solution, it was found that the optimal excitation wavelength of the ICG group in the AGN/ICG-NBs did not change, indicating that encapsulation in the lipid shell would not affect the normal function of ICG.
In this study, the feasibility of AGN/ICG-NBs as a multimode developer was preliminarily verified by in vitro imaging experiments. In vivo imaging experiments were performed on immunodeficient mice implanted with human G250 (+) RCC. In in vivo ultrasound/photoacoustic/fluorescence imaging, AGN/ICG-NBs showed superior imaging effects compared to ICG-NBs. According to quantitative analysis of the ultrasound imaging results, AGN/ICG-NBs achieved a longer duration time, stronger intensity and larger AUC imaging than ICG-NBs. The reason is related to the specific binding of AGN to tumor cells, which mediates the larger number and longer retention of AGN/ICG-NBs in tissues. In photoacoustic imaging, AGN/ICG-NBs had a much stronger imaging effect than ICG-NBs, and the imaging effect of the two types of NBs was basically consistent in the time span, reaching the highest intensity within 15–17.5 min after injection. In addition, compared with the quantitative calculation required for ultrasound imaging, the difference between AGN/ICG-NBs and ICG-NBs is more intuitive and helpful for the differential diagnosis of benign and malignant RMs in the clinic. Fluorescence imaging not only showed the distribution of NBs over time, but also again verified the stronger enrichment ability of AGN/ICG-NBs in xenograft tumors compared with ICG-NBs. The multimodal imaging formed by the combination of ultrasound/photoacoustic/fluorescence imaging can obtain the tumor tissue structure and cell molecular information more comprehensively, which is conducive to the accurate early diagnosis and localization of tumors, as well as the visual evaluation of tumor areas after treatment.
The unique affinity of AGN/ICG-NBs to tumor cells was confirmed by immunofluorescence. AGN/ICG-NBs and ICG-NBs have little difference in particle size (429.2 nm and 411.5 nm). Compared with the gap size of tumor neovascular endothelial cells, which is approximately 380–780 nm,37 both NBs enter the tissue space through tumor blood vessels and directly interact with tumor cells. However, the specific binding properties of AGN/ICG-NB enable it to remain in tumor tissue for a long time after injection. This property also provides a way for us to develop a targeted drug carrier for RCC based on targeted nanobubbles.
5. Conclusion
In this study, an advanced type of nanobubble, AGN/ICG-NBs, with the multimodal imaging function of ultrasound/photoacoustic/fluorescence was prepared by encapsulating ICG in the lipid shell of the previous single-mode targeted UCA nanobubbles. The experimental results verified its ability to enhance ultrasound/photoacoustic/fluorescence imaging of RCC-PDX. This integrated multimodal imaging agent provides a new method for early diagnosis of small RCC and differential diagnosis of RMs, and provides a possibility for further development of an integrated platform of diagnosis and targeted therapy.
Author contributions
Jiajiu Chen: investigation, formal analysis, and writing – original draft; Jingyi Li and Chengjie Zhong: methodology and investigation; Deng Liu, Yi Ling and Xin Li: visualization; Jing Xu: resources and project administration; Qiuli Liu: supervision; Yanli Guo and Luofu Wang: conceptualization, writing – review and editing and supervision.
Conflicts of interest
There are no conflicts in this work to declare within the author reports.
Acknowledgements
Many thanks to Professor Pan Li and his team from Chongqing Institute of Ultrasound Imaging for their support of the photoacoustic part of this study.
This study was supported by the Training Plan of Talent Innovation Ability of the Army Medical Center (no. 2019CXLCB009).
References
- W. Meredith, S. David and P. Gyan, Semin. Nephrol., 2020, 40, 42–48 CrossRef.
- C. de la Encarnacion, D. Jimenez de Aberasturi and L. M. Liz-Marzan, Adv. Drug Delivery Rev., 2022, 189, 114484 CrossRef CAS.
- L. Börje, A. Laurence, A. Yasmin, B. Jens, C. Umberto and D. Saeed, Eur. Urol., 2022, 82, 399–410 CrossRef PubMed.
- N. Carlos and N. A, Medicina, 2021, 57 Search PubMed.
- E. Brown and J. R. Lindner, Curr. Cardiol. Rep., 2019, 21, 30 CrossRef.
- Z. Yu, M. Hu, Z. Li, X. Dan, L. Zhu, Y. Guo, Q. Liu, W. Lan, J. Jiang and L. Wang, Nanotechnology, 2020, 31, 205101 CrossRef CAS PubMed.
- A. H. Chao, S. A. Schulz and S. P. Povoski, Expert Rev. Med. Devices, 2021, 18, 367–374 CrossRef CAS.
- C. Egloff-Juras, L. Bezdetnaya, G. Dolivet and H. P. Lassalle, Int. J. Nanomed., 2019, 14, 7823–7838 CrossRef PubMed.
- M. Capozza, F. Blasi, G. Valbusa, P. Oliva, C. Cabella, F. Buonsanti, A. Cordaro, L. Pizzuto, A. Maiocchi and L. Poggi, Photoacoustics, 2018, 11, 36–45 CrossRef PubMed.
- S. Zackrisson, S. van de Ven and S. S. Gambhir, Cancer Res., 2014, 74, 979–1004 CrossRef CAS.
- G. J. Yoshida, J. Hematol. Oncol., 2020, 13, 4 CrossRef PubMed.
- T. Inoue, N. Terada, T. Kobayashi and O. Ogawa, Nat. Rev. Urol., 2017, 14, 267–283 CrossRef.
- M. Hidalgo, F. Amant, A. V. Biankin, E. Budinská, A. T. Byrne, C. Caldas, R. B. Clarke, S. de Jong, J. Jonkers, G. M. Mælandsmo, S. Roman-Roman, J. Seoane, L. Trusolino and A. Villanueva, Cancer Discovery, 2014, 4, 998–1013 CrossRef CAS.
- S. Abdolahi, Z. Ghazvinian, S. Muhammadnejad, M. Saleh, H. Asadzadeh Aghdaei and K. Baghaei, J. Transl. Med., 2022, 20, 206 CrossRef.
- J. Courcier, A. de la Taille, M. Nourieh, I. Leguerney, N. Lassau and A. Ingels, Int. J. Mol. Sci., 2020, 21 Search PubMed.
- T. S. Keshava Prasad, R. Goel, K. Kandasamy, S. Keerthikumar, S. Kumar, S. Mathivanan, D. Telikicherla, R. Raju, B. Shafreen, A. Venugopal, L. Balakrishnan, A. Marimuthu, S. Banerjee, D. S. Somanathan, A. Sebastian, S. Rani, S. Ray, C. J. Harrys Kishore, S. Kanth, M. Ahmed, M. K. Kashyap, R. Mohmood, Y. L. Ramachandra, V. Krishna, B. A. Rahiman, S. Mohan, P. Ranganathan, S. Ramabadran, R. Chaerkady and A. Pandey, Nucleic Acids Res., 2009, 37, D767–D772 CrossRef CAS.
- A. Queen, H. N. Bhutto, M. Yousuf, M. A. Syed and M. I. Hassan, Semin. Cancer Biol., 2022, 86, 899–913 CrossRef CAS.
- S. Pastorekova and R. J. Gillies, Cancer Metastasis Rev., 2019, 38, 65–77 CrossRef CAS.
- Z. Li, L. Jiang, S. H. Chew, T. Hirayama, Y. Sekido and S. Toyokuni, Redox Biol., 2019, 26, 101297 CrossRef CAS.
- P. Raina, S. K. Singh, A. K. Goswami, M. K. Kashyap, M. Khullar, S. K. Sharma and K. C. Barwal, Mol. Cell. Biochem., 2022, 477, 333–343 CrossRef CAS PubMed.
- F. Quhal, K. Mori, A. Bruchbacher, I. Resch, H. Mostafaei, B. Pradere, V. M. Schuettfort, E. Laukhtina, S. Egawa, H. Fajkovic, M. Remzi, S. F. Shariat and M. Schmidinger, Eur. Urol. Oncol., 2021, 4, 755–765 CrossRef PubMed.
- G. Zhang, H. R. Ye, Y. Sun and Z. Z. Guo, ACS Sens., 2022, 7, 2857–2864 CrossRef CAS PubMed.
- L. Zhu, Y. Guo, L. Wang, X. Fan, X. Xiong, K. Fang and D. Xu, J. Nanobiotechnol., 2017, 15, 63 CrossRef.
- Y. Wang, M. Lan, D. Shen, K. Fang, L. Zhu, Y. Liu, L. Hao and P. Li, Int. J. Nanomed., 2020, 15, 4289–4309 CrossRef CAS.
- J. Qi, H. L. Ou, Q. Liu and D. Ding, Aggregate, 2021, 2, 95–113 CrossRef CAS.
- B. P. Burke, C. Cawthorne and S. J. Archibald, Philos. Trans. R. Soc., A, 2017, 375 Search PubMed.
- C. de la Encarnación, D. Jimenez de Aberasturi and L. M. Liz-Marzán, Adv. Drug Delivery Rev., 2022, 189, 114484 CrossRef.
- M. Maturi, E. Locatelli, I. Monaco and M. Comes Franchini, Biomater. Sci., 2019, 7, 1746–1775 RSC.
- Y. W. Wang, N. Niu, Y. Huang, S. L. Song, H. Tan, L. Wang, D. Wang and B. Z. Tang, Small Methods, 2022, 6, 2200393 CrossRef CAS PubMed.
- S. H. Lim, H. T. A. Tan and V. G. Shelat, Surg. Endosc., 2021, 35, 1511–1520 CrossRef PubMed.
- H. Gao, X. Qi, J. Zhang, N. Wang, J. Xin, D. Jiao, K. Liu, J. Qi, Y. Guan and D. Ding, Small Methods, 2023, 7, 2201582 CrossRef CAS.
- Z. Chen, P. Zhao, Z. Luo, M. Zheng, H. Tian, P. Gong, G. Gao, H. Pan, L. Liu, A. Ma, H. Cui, Y. Ma and L. Cai, ACS Nano, 2016, 10, 10049–10057 CrossRef CAS.
- X. Jin, X. Lu, Z. Zhang and H. Lv, Int. J. Nanomed., 2020, 15, 3193–3206 CrossRef CAS PubMed.
- A. S. Lo, C. Xu, A. Murakami and W. A. Marasco, Mol. Ther. – Oncolytics, 2014, 1, 14003 CrossRef CAS.
- Y. Wang, L. Yin, Y. Cui, L. Wang, J. Wu, J. Wang, H. Zhao, C. Liu, Y. Cui, Y. Zhang, X. Li, Z. Zhu and L. Yang, Cancer Biother. Radiopharm., 2022, 37, 494–502 CrossRef CAS.
- A. T. Tracey, K. S. Murray, J. A. Coleman and K. Kim, Cancers, 2020, 12 Search PubMed.
- J. Ma, C. S. Xu, F. Gao, M. Chen, F. Li and L. F. Du, Mol. Med. Rep., 2015, 12, 4022–4028 CrossRef CAS PubMed.
|
This journal is © The Royal Society of Chemistry 2024 |