DOI:
10.1039/D3NA01063E
(Review Article)
Nanoscale Adv., 2024,
6, 1331-1360
Nanocrystals as performance-boosting materials for solar cells
Received
29th November 2023
, Accepted 31st January 2024
First published on 1st February 2024
Abstract
Nanocrystals (NCs) have been widely studied owing to their distinctive properties and promising application in new-generation photoelectric devices. In photovoltaic devices, semiconductor NCs can act as efficient light harvesters for high-performance solar cells. Besides light absorption, NCs have shown great significance as functional layers for charge (hole and electron) transport and interface modification to improve the power conversion efficiency and stability of solar cells. NC-based functional layers can boost hole/electron transport ability, adjust energy level alignment between a light absorbing layer and charge transport layer, broaden the absorption range of an active layer, enhance intrinsic stability, and reduce fabrication cost. In this review, recent advances in NCs as a hole transport layer, electron transport layer, and interfacial layer are discussed. Additionally, NC additives to improve the performance of solar cells are demonstrated. Finally, a summary and future prospects of NC-based functional materials in solar cells are presented, addressing their limitations and suggesting potential solutions.
1. Introduction
Nanocrystals (NCs), sometimes termed as quantum dots (QDs) or nanoparticles (NPs), with a size less than 100 nm, are popular worldwide and have been paid extensive attention owing to their outstanding photoelectric properties. In the energy field, photovoltaic devices based on semiconductor NCs are among the most potential systems with high power conversion efficiency (PCE), good stability, low cost, facile fabrication, and other advantages.1–16 In recent years, NCs have been widely used as light harvesting layers in high-performance solar cells. Semiconducting NCs such as PbX (X = S, Se, and Te), CdSe, and CuInS2 have been suggested as excellent light harvesters in third-generation solar cells.11,14,15,17–22 Additionally, perovskite NCs are also good alternatives for light harvesting in solar cells.12–14,16,23–28 Besides active layers (light harvesting layers), functional layers based on NCs, mainly including hole transport layers (HTLs),29–99 electron transport layers (ETLs)100–173 and interfacial functional layers (IFLs),174–221 are of great importance in solar cells. Notably, NC additives also boost the performance of solar cells through defect passivation, plasmonic effect, solar concentration, light up-conversion and down-conversion/shifting, light scattering and reflection, and heat sinks.222–258
To efficiently transport charge in solar cells, HTLs and ETLs must have suitable energy levels matching the active layers such as dyes, Si, Pb-based light harvesting layers, and perovskite films. For example, to fabricate high-performance perovskite solar cells (PSCs), the work function of HTLs and ETLs should have hole and electron transport layers aligned with the valence band edge and conduction band edge of a perovskite. As is well known, the energy levels of NCs can be easily controlled and adjusted by changing their sizes, ligands, and dopants during their synthesis process, which is favourable to satisfy the energy alignment. Meanwhile, the easily-accomplished modifications in NCs mentioned above also favour high mobility to allow carrier transport and form a more effective current circulation path. Furthermore, the transmittance of HTL or ETL based on NCs can be improved during synthesis processes, which leads to less light loss and thus, higher performance of solar cells. Consequently, NCs are a good choice as HTLs and ETLs for efficient solar cells and have gained much attention.
Some researchers found that inserting different IFLs in solar cells could promote the power conversion efficiency (PCE) and stability of the devices. Good IFL can modulate the formation of adjacent layers (especially the perovskite layer in PSCs), optimize energy alignment, and impede charge recombination. As multi-functional IFL, NCs have been extensively investigated due to their compatible properties, such as appropriate morphology for compact, smooth films, gradual energy level to transfer carriers, and self-stability to protect the devices.
Besides acting as a separate functional layer in efficient solar cells, NCs have also been used as additives to improve active layer quality, accelerate carrier transfer, convert infrared or ultraviolet light to visible light, scatter and reflect light, sink heat, and other functions.222–258 The addition of NCs could enlarge the grain size of perovskite film, reduce the recombination, enhance charge extraction, improve the charge mobility, broaden the spectral response range, and so on.
In this review, functional materials based on NCs for high-performance solar cells are summarized. NCs as HTL, ETL, IFL, and additives for NC-light-harvestor solar cells (NC-LHSCs), PSCs, organic solar cells (OSCs), Si solar cells, and dye-sensitized solar cells (DSSCs) are successively analyzed. In Section 2, NCs as charge transport layers are analyzed in detail. This can provide a deep understanding of the extensive application of NCs in solar cells. As an important functional layer, IFL based on NCs is summarized in the next section. Section 4 describes NCs as efficient additives in different functional layers due to their small sizes and excellent photoelectric properties. Finally, we discuss the existing challenges of NCs for boosting the performance of solar cells and provide some feasible suggestions on these issues, expecting to improve the performance of solar cells based on NCs.
2. Nanocrystals as charge transport layers
In this section, we focus on NCs-based charge transport layers for solar cells, mainly including top/bottom HTL and ETL in n-i-p and p-i-n solar cells.
2.1 Nanocrystals as HTL
As known to us, solar cells work as follows: absorption of sun light, generation and separation of hole–electron pairs, transport of holes through HTL and electrons through ETL, and current produced by the flow of electrons through external circuits. Solar cells using NCs as HTL are not exceptional, and the typical structures of solar cells based on NC HTL are shown in Fig. 1. According to the position of HTL, we call HTL between the ITO/FTO and active layer as bottom HTL while the HTL between metal electrode and active layer as top HTL.
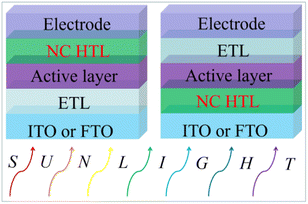 |
| Fig. 1 Typical structure of solar cells based on top (left) and bottom (right) NC HTLs. | |
2.1.1 Recent NC HTL.
Semiconductor NCs have shown great potential in photoelectronic devices due to their excellent properties. The advances of NC HTLs for solar cells are listed in Table 1, including the size of NCs, device structure, PCE, and stability of solar cells.
Table 1 Recent advances in selected NC HTLs for solar cells (EDT = 1,2-ethanedithiol, relative humidity = RH)
|
NC |
Active layer |
PCE (%) |
Long-term stability |
Ref. |
Top HTL |
PbS-EDT |
CH3NH3PbI3 |
7.88 |
8% decay after 2 days |
29
|
PbS |
11.6 |
Null |
30
|
10.4 |
100%, after 103 days in the dark under ambient conditions |
32
|
9.44 |
∼100%, after 4 months in ambient air |
33
|
13.2 |
Null |
37
|
10.4 |
①96%, after baking on a hotplate in air for 120 min; ②97%, after 60 min oxygen plasma treatment |
38
|
13.3 |
Null |
39
|
10.5 |
Null |
40
|
13.0 |
Null |
41
|
PbS–PbI2 |
11.2 ± 0.22 |
Nearly no drop of PCE after 180 h under continuous heating at 85 °C in ambient air |
44
|
11.29 |
>90, after 600 h under ambient condition |
45
|
PbS:F |
12.7 |
Null |
46
|
PbX2–PbS |
8.16 |
Null |
47
|
10.35 |
Null |
48
|
10.6 |
Null |
49
|
PbS–PbX2–KI3 |
12.1 |
94%, after 20 h continuous operation in air |
50
|
PbSe–PbS |
1.24 (infrared PCE) |
∼95%, after 25 days in air |
51
|
PbSe–PbI2 |
10.4 |
90%, after 30 days in ambient condition |
51
|
CsMAFA–PbS |
11.3 |
96%, after 1200 h shelf storage |
53
|
MAPbI3–PbS |
9.5 |
95%, after 2 months in ambient environment |
54
|
Sb2Se3 |
6.5 (certified) |
Null |
55
|
KPbS |
12.6 |
83%, after 300 h under continuous operation at MPP in ambient air |
56
|
CuInS2/ZnS core/shell |
MAPbI3 |
8.38 |
Null |
57
|
CdZnSe@ZnSe |
CdZnSe@ZnSe |
8.65 |
Null |
58
|
Cu2ZnSnSe4 |
MAPbI3 |
9.72 |
Null |
59
|
Cu2ZnSnS4 |
10.72 |
SnS |
(CsPbI3)0.05(FAPbI3)0.79(MAPbI3)0.16(PbI3)0.03 |
13.7 |
①99%, after 1000 h storage in air; ②75%, after 500 h under continuous 1 sun illumination in a N2 atmosphere at 25 °C |
60
|
CuInSe2 |
MAFAPbClBrI |
12.8 |
78%, after 96 h in air |
61
|
Ag–In–Ga–S |
CsPbBr3 |
8.46 |
96.1%, after 240 h in air |
62
|
Cu12Sb4S13 |
CsPbI3 |
10.02 |
94%, after storage in ambient air for 360 h |
63
|
Cu2SnS3 |
Cs0.05(MA0.17–FA0.83)0.95Pb(I0.83Br0.17)3 |
13.01 |
90%, after 1200 h in an ambient atmosphere |
64
|
CuGaS |
(FAPBI3)1−x(MAPbBr3)x |
17.56 |
81%, aging for 30 days |
65
|
CuInS2 |
(FAPBI3)1−x(MAPbBr3)x |
18.81 |
91%, aging for 30 days |
66
|
NiO |
MAPbI3 |
6.2 |
Null |
67
|
MAPbI3NCs |
10.89 |
Null |
68
|
Ti-doped MoO2 |
MAPbI3 |
15.8 |
∼95%, after 15 days with 50–70% RH |
70
|
Co3O4 |
MAPbI3 |
13.27 |
Up to 2500 hours under ambient conditions |
71
|
Cu2O |
Cs0.05FA0.81MA0.14PbI2.55Br0.45 |
18.9 |
80%, after 30 days in air with ∼30% RH |
72
|
CuCrO2 |
Cs0.05(MA0.15FA0.85)0.95Pb(I0.85Br0.15)3 |
16.68 |
88%, after 500 h at the MPP under one sun and a N2 atmosphere |
73
|
CuGaO2 |
MAPbI3 |
18.51 |
>85%, after 30 days at 25 °C with 30–55% humidity without encapsulation |
74
|
CsPbI3 |
MAPbI3 |
17.0 |
Null |
75
|
Bottom HTL |
PbS |
MAPbI3 |
7.5 |
Null |
76
|
Cu2ZnSnS4 |
MAPbI3 |
15.4 |
Null |
77
|
6.02 |
87%, after 43 days in N2 atmosphere |
78
|
Ni–NiO core–shell |
P3HT |
0.86 |
Null |
79
|
NiOx |
Cs0.08(MA0.17FA0.83)0.92Pb(I0.83Br0.17)3 |
12.8 |
Null |
81
|
MAPbI3 |
16.1 |
90%, after 60 days in air at RT |
84
|
Cs0.05(MA0.17FA0.83)0.95Pb(I0.9Br0.1)3 |
18.6 |
>80%, after 1000 h |
85
|
MAPbI3 |
17.60 |
93%, after 30 days |
86
|
1.02 cm2: 18.49 (rigid) and 15.89 (flexible) |
90%, after 500 h in the thermal aging test (85 °C, 85% RH) |
87
|
Cu:NiOx |
15.01 (flexible, >1 cm2) |
86%, after 1 month in an ambient environment at 25 °C with about 40% humidity |
88
|
18.3 |
Null |
89
|
(Li,Cu):NiOx |
MAPbI3−xClx |
20.80 |
95%, after 60 days of storage |
90
|
NiOx |
CsPbIxBr3−x |
16.1 |
85%, after 350 h light soaking |
91
|
CuO |
MAPbI3 |
15.3 |
Null |
92
|
CuCrO2 |
19.0 |
∼90, after 30 days in an Ar-filled dry glove box and continuously irradiated by a UV optical fiber with 5 mW cm−2 |
94
|
CuGaO2 |
15.6 |
Null |
95
|
NiCo2O4 |
MAPbI3−xClx |
18.23 |
∼90%, after 500 h illumination at AM 1.5G |
96
|
ZnCo2O4 |
PTB7-Th:PC71BM |
9.37 |
>60%, after 60 h in ambient environment with 50% RH without capsulation |
98
|
MAPbI3−xClx |
18.14 |
>60%, after 110 h in ambient environment with capsulation and under continuous 1 sun illumination soaking |
In:CuCrO2 |
Cs0.05(MA0.15FA0.85)0.95Pb(I0.85Br0.15)3 |
20.54 |
∼90%, after 800 h of continuous radiation in glovebox |
99
|
Lead chalcogenide NCs, especially PbS, have been massively used as efficient top HTL in NC-LHSCs.29–56 Additionally, other metal chalcogenide NCs are also excellent choices as top HTL for high-performance perovskite solar cells (PSCs), mainly containing CuInS2/ZnS (core/shell),57 CdZnSe@ZnSe,58 Cu2ZnSnS4/Se4,59 SnS,60 CuInSe2,61 Ag–In–Ga–S,62 Cu12Sb4S13,63 Cu2SnS3,64 CuGaS,65 and CuInS2.66 Metal oxide NCs of NiO have shown potential for hole transport on top of the light harvesting layer in PSCs and Si solar cells.67–69 Besides NiO, some other metal oxide NCs such as MoO2,70 Co3O4,71 Cu2O,72 CuCrO2,73 CuGaO2,74 and CsPbI3 (ref. 75) have been used as efficient top HTL for PSCs due to their excellent properties. It can be noticed that when PbS NCs are used as top HTL in solar cells, the light harvesting layers are mostly based on the PbS/Se family. There are not many perovskite used as light harvest materials when PbS/Se is used as top HTL. Only MAPbI3 was applied in 2015, and 7.88% PCE was gained.29 We suppose this is because the PbS NCs with long carbon chains or 1,2-ethanedithiol are not good at charge transport. If high-temperature annealing is adopted to enhance the conductivity of PbS, the perovskite underneath will be destroyed. This encourages us to regulate the synthesis or modification of NCs with less or even no charge transfer inhibition capture and improve their electronic properties.
Similar to top HTL, NCs can also be applied as bottom HTL for high-performance solar cells. NCs of PbS,76 Cu2ZnSnS4,77,78 NiOx and its doped derivatives,79–91 CuO,92 and ternary oxides of CuCrO2,93,94 CuGaO2,95 NiCo2O4,96,97 ZnCoO4,98 and doped ternary oxide In:CuCrO2 (ref. 99) have offered excellent hole transport ability in PSCs and PTB7-Th-based organic solar cells (OSCs).
Ever since demonstrated as efficient HTL by Luther et al. in 2008,248 PbS NCs with a 1,2-ethanethiol (EDT) ligand (named as PbS-EDT or EDT-PbS) have been massively applied for hole transport in solar cells. From the established NC HTLs listed in Table 1, we can find some regular patterns: (i) as top HTL, EDT-linked sulphides were mainly used in NC-LHSCs but were not popular in PSCs. This is because EDT will strongly attack the perovskite materials in the n-i-p device and decrease the device performance.61 (ii) As bottom HTL, oxides are widely used and sulphides are rare. We speculate that it is (iii) NiOx with high hole transport quality mostly used to transport holes in PSCs.
The previous light harvesting NC layers are mainly based on the Pb-based chalcogenide family or their mixture. NC-LHSCs using NCs as HTL have gained high PCE above 13%,45,50 but this is significantly lower than that of the theoretical value.11 Some strategies were also adopted to modify the light-harvesting NCs, which is not the keynote in this review. The current relatively low PCE of NC-LHSCs may be on account of non-radiative recombination resulting from the high density of surface traps due to some intrinsic properties of NCs, such as high surface-to-volume ratios. Using NC top HTL, the PCE value can be improved. Some oxides, for instance, NiO, Ti-doped MoO2, Co3O4, Cu2O, CuCrO2, and CuGaO2, have acted as excellent top HTL in high-performance PSCs. As shown in Fig. 2a, SnS NCs prepared by the one-pot hot-injection method were utilized as top HTL in efficient and stable (CsPbI3)0.05(FAPbI3)0.79(MAPbI3)0.16 PSCs. The high PCE mainly resulted from good surface coverage and an excellent hole extraction ability demonstrated by Nyquist plots. Additionally, SnS-based PSC presented better air stability than the 2,2′,7,7′-tetrakis[N,N-di(4-methoxyphenyl)amino]-9,9′-spirobifluorene (spiro-OMeTAD)-based device. Surface-modified Cu2O NCs boosted the efficiency of PSC to 18.9% with distinctly better stability than the reference device based on spiro-OMeTAD.61 One important reason is the difference in hydrophobicities illustrated by water contact angles in Fig. 2b. Ternary oxide CuGaO2NCs with promising photoelectronic properties boosted the n-i-p PSCs with higher PCE and stability than spiro-OMeTAD (Fig. 2c).75
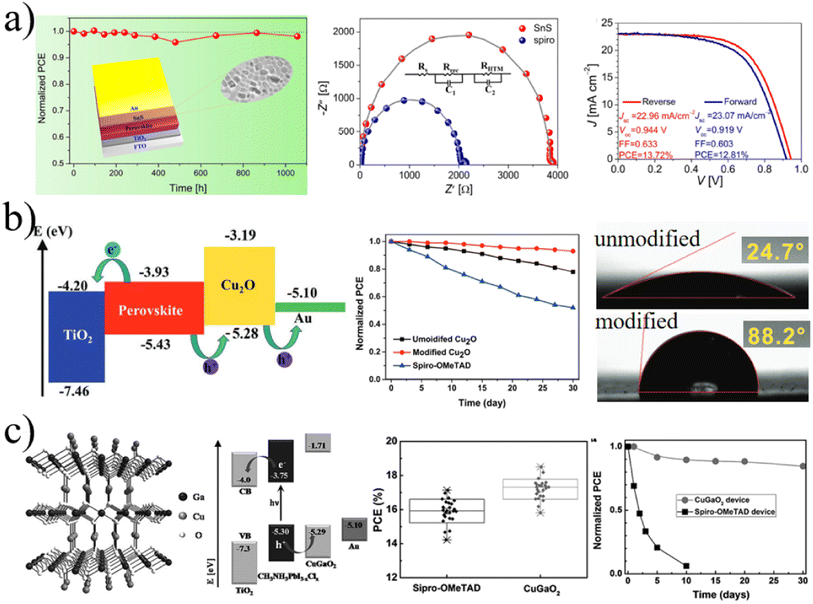 |
| Fig. 2 (a) Evolution of PCE over time of the unencapsulated SnS-based PSCs in the ambient air of ∼30–50% humidity, Nyquist plots at 0.9 V forward bias measured in the dark, forward, and reverse J–V curves of champion PSCs based on SnS. (b) Schematic view of PSC configuration, device performance durability of PSCs based on different HTLs in ambient air for 30 days, and water contact angles of unmodified and modified Cu2O. (c) Schematic illustration of the crystal structure of CuGaO2, device architecture of a regular PSC based on CuGaO2, standard deviations of PCEs to evaluate reproducibility by statistics of 50 devices based on CuGaO2 and spiro-OMeTAD. (a) Adapted with permission.61 Copyright 2019, American Chemical Society. (b) Adapted with permission.73 Copyright 2019, Wiley-VCH. (c) Adapted with permission.75 Copyright 2017, Wiley-VCH. | |
In n-i-p type solar cells, NiOX and its doped family oxide NCs are the most popular NC HTLs because of their facile synthesis and outstanding photoelectronic properties.86,101 Meanwhile, sulphides of PbS and Cu2ZnSnS4 together with multi basic oxides of CuCrO2, CuGaO2, NiCo2O4, ZnCo2O4, and In:CuCrO2 are valuable substitutes for NiOX family. Ligand-free NiOx NCs in ethanol (E-NiOx) are spin-coated onto a substrate to form a smooth and compact NiOx film that has good hole extraction capability. As seen in Fig. 3a, this E-NiOx bottom HTL can be used both in rigid and flexible PSC, producing high PCE and stability. Similar to top HTL, ternary oxide NCs like CuCrO2 were also utilized as bottom HTL for high-performance solar cells. The low-temperature solution-processed CuCrO2 NCs provide suitable electronic structure, charge carrier transport properties, and greater UV light-harvesting, demonstrating its potential as an efficient HTL for highly efficient and photostable n-i-p PSCs (Fig. 3b).
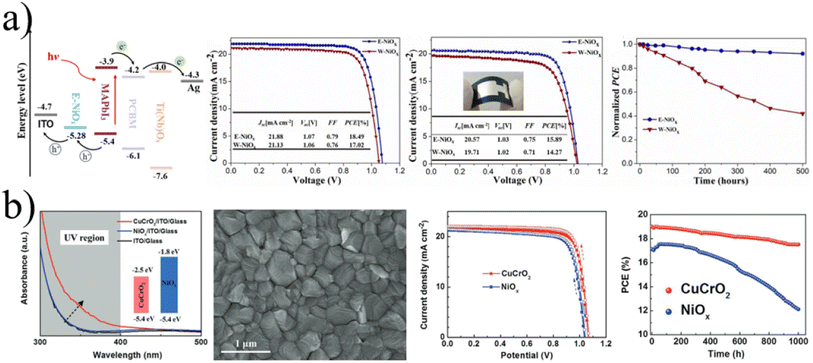 |
| Fig. 3 (a) Energy level diagram of the materials used in the device, J–V curves of rigid and flexible PSCs, normalized PCE. (b) UV-vis spectra of the CuCrO2 and NiOx layer with optimum thickness with energy level in the inset. Adapted with permission.87,94 Copyright 2018, Wiley-VCH. | |
2.1.2 Advantages of NC HTL.
One important inherent advantage of semiconductor NCs is their bandgap variation along with modification. The conduction band, valence band, and Fermi level of PbS NCs can be modified by changing surface ligands (Fig. 4), thus enhancing the performance of solar cells.252Fig. 4 shows that the energy level of PbS NCs is easily changed in a large range by different ligands, facilitating energy matching with the light-harvesting layer and other functional layers. Compared with mostly used organic HTLs of spiro-OMeTAD, poly(3,4-ethylenedioxythiophene):poly(styrene-sulfonate) (PEDOT:PSS) and poly[bis(4-phenyl)(2,4,6-trimethylphenyl)amine] (PTAA), inorganic NCs show higher stability with good hole transport ability, suggesting great potential for boosting solar cell performance. Equally important, facile synthesis and low cost would be helpful for the commercialization of new-generation solar cells.
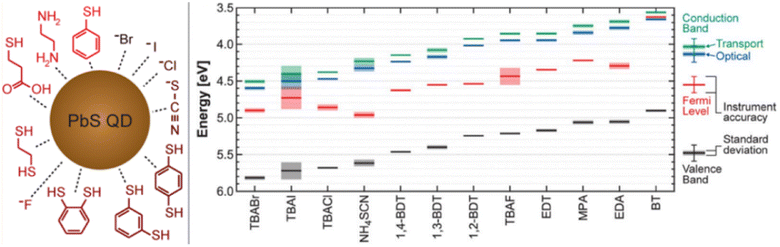 |
| Fig. 4 Energy band position of PbS NC films for different surface ligands. Adapted with permission.249 Copyright 2014, American Chemical Society. | |
2.1.3 Potential NC HTL.
To further develop more strategies for fabricating high-performance solar cells and boost the device PCE and stability, we should find more potential NCs for efficient hole transport in solar cells. According to the previous reports and our understanding, excellent NC HTLs should satisfy the following requirements: (i) matched energy level alignments with other functional layers; (ii) high hole mobility and conductivity; (iii) enhancing the quality of adjacent layers; (iv) intrinsic resistance to heat, light and water; (v) convenient fabrication with low cost; and (vi) high transmittance for bottom HTL and reflectivity for top HTL. NCs have many good properties such as easily-controlled energy level, excellent spreading and filling ability due to their small sizes, stable intrinsic structure, skilled synthesis process, and variant surface ligand. Considering the above rules and the advantages of NCs, besides the existing NC HTLs, some other p-type sulphide and oxide NCs have huge potential for efficient and stable solar cells. Additionally, semiconductor NCs with enhanced hole-transporting ability by p-type doping are also good alternatives.
2.2 Nanocrystals as ETL
2.2.1 Recent advances of NC ETL.
As important as HTL, ETL is also of great significance for high-performance solar cells to transport electrons and block holes, and it acts as well as a trap passivating layer and water/oxygen preventing layer. The typical structure of solar cells based on NC ETL is shown in Fig. 5.
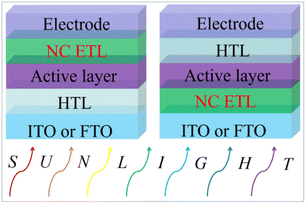 |
| Fig. 5 Structure of solar cells based on top (left) and bottom (right) NC ETLs. | |
ZnO is good at electron transport in photoelectrical devices due to excellent properties such as bandgap (3.3 eV), low cost, high electron mobility (∼10−5–102 cm2 V−1 s−1), and matching energy levels.100,101 ZnO NCs with different sizes have been used as efficient top ETL in NC-LHSCs, OSCs, and PSCs.43,84,85,91,101–108 TiO2 and SnO2NCs are as popular as ZnO for electron transport due to their outstanding photoelectronic properties of high mobility and conductivity.250 Certainly, these oxide NCs can be modified by doping, ligand changing, and other strategies. Tetrabutylammonium hydroxide (TBAOH)-capped metal oxide NCs for SnO2 also extend to TiO2, ITO and CeO2NCs as top ETL for PSCs.108 TiO2NCs have always been as the top ETL for PSCs.109–111 CeOx,112 In2O3 and its Sn doped derivative formed bilayer ETL,113 and CdSe114 were also used for high-performance n-i-p PSCs. As efficient bottom ETL, modified TiO2 by Sn, Al, Co, Cu, and N doping and N, F and S co-doped graphene NCs were widely used in dye-sensitized solar cells (DSSCs) based on N719 and N3 ({cis-Ru(H2dcbpy)2(NCS)2, H2dcbpy = 4,4′-dicarboxy-2,2′-bipyridyl }).115–120 Additionally, TiO2, CdS NCs-modified TiO2, and Nb-doped TiO2NCs were applied for electron transport in PSCs with high PCE and stability.121–123 Meanwhile, TiO2NCs have been widely applied in n-i-p solar cells using chalcogenide NC as light harvesters, such as CdSe, CdS, and PbS.124–130 Similarly, ZnO NCs were used as ETL for n-i-p PbS-based NC-LHSCs,30,32,35,39,40,44,45,58,131–145 PSCs,146 and OSCs.147–149 SnO NCs as ETL for DSSCs,150–156 for OSCs,157–159 and for PSCs,27,160–168 have been demonstrated. Additionally, a bilayer of SnO2/TiO2 (ref. 169) and SnO2/InP–ZnS170 were used for PSCs and DSSCs. Ni-doped Co3S4 and Co4S3 and ternary sulphide NCs of NiCo2S4 and Zn2SnO4 have been used for DSSCs,171,172 while doped SrSnO3 NCs for PSCs.173 NCs have been used as IFL for NC-LHSCs,49,141,174–179 OSCs,180–185 DSSCs,186–195 PSCs,196–212 and Si solar cells.213–220 FAPbBr3 perovskite NCs have been used as a multifunctional luminescent-downshifting passivation layer for GaAs solar cells.221
From the reported literature listed in Table 2, selenide NCs of CdSe, and doped sulfide NCs of Ni:Co2S4, Ni:Co4S3, and Ni:Co2S4 have shown their potential, suggesting more substitutes for traditional NC ETL. The advantages of NC ETL and some potential NCs suitable as ETLs are discussed in the following sections.
Table 2 Recent advances in NC ETLs (bathocuproine = BCP)
|
NC |
Active layer |
PCE (%) |
Long-term stability |
Ref. |
Top ETL |
ZnO |
PbS–PbX2 |
10.35 |
Null |
43
|
PCDTBT:PC61BM |
2.66 |
Null |
100
|
CsPbI3NCs |
13.1 |
80%, after 40 h under 25 °C with 30–40% RH |
103
|
MAPbI3 |
17.2 |
66%, after 120 days stored in air |
105
|
MAPbI3 |
16.1 |
90% after 60 days in air at RT |
84
|
Cs0.05(MA0.17FA0.83)0.95Pb(I0.9Br0.1)3 |
18.6 |
>80% after 1000 h |
85
|
CsPbIxBr3−x |
16.1 |
85%, after 350 h light soaking |
91
|
Ag modified BCP:ZnO |
MAPbI3 |
15.5 |
Null |
106
|
In:ZnO |
MAPbI3 |
16.2 |
85% after 460 h of light soaking |
107
|
TBAOH-SnO2 |
MAPbI3 |
18.77 |
90% after aging at 45 °C for 240 h, followed by 65 °C for 240 h and 85 °C for 240 h |
108
|
TiO2 |
Cs0.05(MA0.15FA0.85)0.95Pb(I0.85Br0.15)3 |
20.5 |
∼90% after 350 h MPP tracking test |
109
|
Carbide-TiO2 |
CsPbI2Br |
14.8 |
①>94%, after 1000 h at 85 °C in dark under N2; ②>90%, after 1000 h at 60 °C under continuous illumination |
110
|
CeOx |
MAPbI3 |
16.7 |
100%, after 200 h in air with 30% RH |
112
|
Sn:In2O3/In2O3 (bilayer) |
Cs0.05(MA0.15FA0.85)0.95Pb(I0.85Br0.15)3 |
20.65 |
①91.9% after 69 days under 85 °C;②91.8% after 2000 h, 12 h continuous 1 sun illumination and then 12 h interval in the dark |
113
|
CdSe |
MAPbI3 |
15.1 |
Null |
114
|
Bottom ETL |
Sn:TiO2 |
N719 |
6.24 |
Null |
115
|
Al:TiO2 |
4.27 |
Null |
116
|
Co:TiO2 |
4.85 |
Null |
117
|
Ti0.94Cu0.06O2 |
N3/electrolyte |
6.51 |
Null |
118
|
TiO2 modified by N, F and S, co-doped graphene NCs |
N719 |
11.7 |
∼85.5, after one month |
120
|
TiO2 |
Cs–FA–MA mixed cation perovskite |
19.03 |
84%, after 30 days |
121
|
CdS NCs-modified TiO2 |
MAPbI3 |
8.16 |
Null |
122
|
Nb-doped TiO2 |
Cs0.05(MA0.15FA0.85)0.95Pb(I0.85Br0.15)3 |
18.97 |
Null |
123
|
TiO2 |
CdS |
4.59 |
Null |
125
|
Cu2O doped TiO2 |
CdSe/CdS/ZnS/electrolyte |
3.01 |
Null |
126
|
TiO2 |
Mn–CdSe |
3.55 |
Null |
128
|
TiO2 |
CdSe/ZnS/SiO2/Cu2S |
4.00 |
Null |
129
|
Cl@ZnO |
PbS |
11.6 |
Null |
30
|
ZnO |
PbX2–PbS |
9.5 |
95%, after 2 months in ambient environment |
33
|
MgZnO |
PbS |
10.4 |
100%, after 103 days in the dark under ambient conditions |
32
|
ZnO |
Lead halide-PbS |
12.7 |
Null |
46
|
ZnO |
PbS |
13.1 ± 0.1 |
Null |
37
|
ZnO |
PbS NC |
12.44 |
Null |
140
|
Cs-ZnO |
PbS |
10.43 |
97%, after 3 months under 20 °C and 30% RH without any encapsulation |
144
|
ZnO |
CsPbBr3–CsPb2Br5 |
6.81 |
No detectable decay after 100 days under 25 °C and 45% RH |
146
|
ZnO |
PTB7-Th:PC71BM |
12.02 |
Null |
147
|
Na–ZnO |
p-DTS(FBTTh2)2:PC70BM |
9.2 |
90%, after 28 h |
149
|
SnO2 |
N719 |
3.2 |
Null |
151
|
Ni:SnO2 |
3.6 |
Zn:SnO2 |
4.2 |
SnO2 |
Eosin-Y |
3.89 |
Null |
155
|
Sn0.92O2:Sb0.08 |
4.15 |
Null |
156
|
SnO2 |
PBDR-T & ITIC-4 |
12.023 |
①>90%, up to 75 h after exposure to an ambient atmosphere with continuous illumination; ②>95%, after storage in N2 for 200 h with continuous illumination |
157
|
PM6:Y6 |
14.9 |
81%, after 15 days stored in the air at RM without encapsulation |
158
|
PTB7-Th:PC71BM |
10.30 |
Null |
159
|
PM7:ITC6-4F |
13.93 |
Null |
PM6:Y6 |
15.38 |
94.3%, after 100 h light aging |
Cl@SnO2 |
CsPbI3NCs |
14.5 |
80%, without encapsulation under 1-sun light soaking and 50% relative humidity for 8 h |
27
|
Ga:SnO2 |
Cs0.05(MA0.17FA0.83)0.95Pb(I0.83Br0.17)3 |
18.18 |
Null |
161
|
SnO2 |
CsPbI3/FAPbI3 |
16.07 |
96%, after 1000 h in ambient storage |
162
|
Csx(MA0.17FA0.83)(100−x)Pb(I0.83Br0.17)3 |
17.92 |
89%, after 2500 h stored under 20 ± 5% RH |
163
|
MAPbI3 |
13.90 (flexible device) |
Null |
164
|
(CsPbI3)0.04(FAPbI3)0.82(MAPbBr3)0.14 |
20.34 ± 0.5 |
90%, after 720 h storage |
165
|
Cs0.05(MA0.17FA0.83)0.95Pb(I0.83Br0.17)3 |
20.79 |
Null |
167
|
FAPbI3 |
25.39 (0.0803 cm2) |
①80% after 1000 h in ambient air at 25% RH and 25 °C without sealing; ②70.5% after 700 h light soaking; ③95% (encapsulated cell) after 100 h MPP tracking and 2 h dark recovery under ambient conditions |
168
|
23.3 (1 cm2) |
Null |
21.7 (20 cm2) |
Null |
20.6 (64 cm2) |
Null |
SnO2/TiO2 (bilayer) |
CsPbI2Br |
15.86 |
①∼95%, after 1 month storage in N2 glovebox without encapsulation; ②>80%, after 1 month stored under RT and 20–30% RH without encapsulation |
169
|
SnO2/InP–ZnS (bilayer) |
PM6:Y6 |
15.22 |
>80%, after 500 h in N2 without encapsulation |
170
|
Ni:Co3S4 |
N719 |
6.01 |
Null |
171
|
Ni:Co4S3 |
6.82 |
NiCo2S4 |
7.43 |
Zn2SnO4 |
4.9 ± 0.2 |
172
|
Y:SrSnO3 |
FA0.85MA0.15Pb(I0.85Br0.15)3 |
19.0 |
91%, after 1000 h stored in N2 |
173
|
2.2.2 Advantages of NC ETL.
Compared with common organic ETLs such as fullerenes (C60/C70) and phenyl-C61-butyric acid methyl ester (PCBM), semiconductor NCs can easily overcome the shortcomings of poor stability, high cost, and unsuitable energy levels. In detail, PCBM film degrades at 85 °C, indicating its thermal instability.110 The cost of synthesis and purification for organic electron transport materials is higher than most semiconductor NCs.252 Moreover, the semiconductor NCs can offer adjustable energy levels by easily controlling the size. The above advantages strongly demonstrate that semiconductor NCs are a very good choice as efficient ETL in high-performance solar cells.
2.2.3 Potential NC ETL.
Thanks to their unique application advantages in solar cells, NC ETLs have been widely used, boosting the devices to higher PCE and stability. More oxide and chalcogenide NCs as well as their doped congeners are anticipated alternatives. As we know, TiO2NCs always act as splendid ETL in solar cells. The properties of TiO2NCs can also be improved by doping, enhancing the device performance. As shown in Fig. 6, many elements were successfully doped in TiO2 as ETL for PSCs. Meanwhile, more elements are potential dopants for high-quality TiO2 and this suggests that there is much room for doped-TiO2NC ETL. Additionally, we reasonably think this strategy is also applicable to other oxide NCs like SnO2 due to its comparable nature with TiO2.
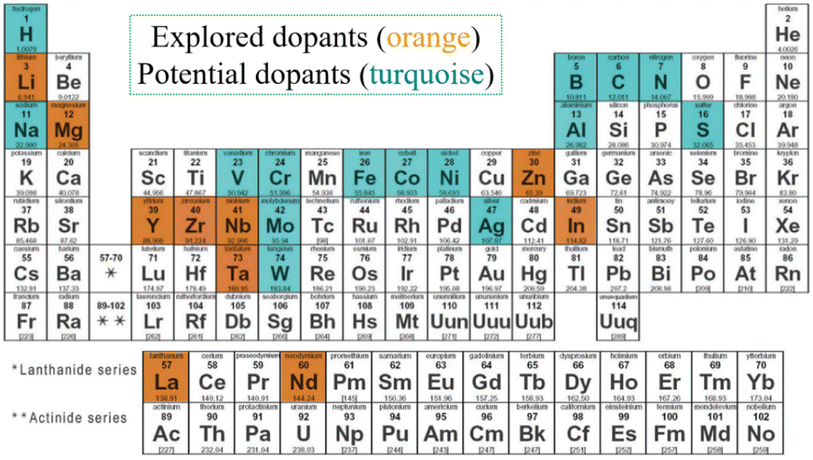 |
| Fig. 6 Summary of the explored and potential elements as dopants in TiO2-based electron transporters for PSCs in the periodic table, as in 2017.253 Copyright 2017, Wiley-VCH. | |
3. Nanocrystals as IFL
Besides HTL and ETL, another essential layer is the interfacial functional layer (IFL). Interfaces play a non-ignorable role in improving both PCE and stability through many paths. The position of a typical IFL is shown in Fig. 7. The IFL can be located at different positions in the solar cells to make different contributions. NCs IFL can enhance light absorption, decrease carrier recombination, improve charge transport ability, reduce impedance, convert ultraviolet (UV) or near-infrared (NIR) light to visible light, enhance optical transmission, decrease trap states of the active layer, and so on. Table 3 shows the recent advances of NC IFLs, and the detailed discussion is as follows.
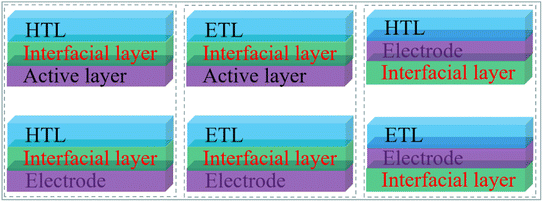 |
| Fig. 7 Typical position sketch of interfacial layers in solar cells. | |
Table 3 Recent advances in selected NCIFLs
|
IFL and its adjacent layers |
PCE (%) |
Functions |
Ref. |
PbCdS |
TiO2/PbCdS/CdS |
3.35 |
Enhance light absorption; suppress interfacial recombination |
174
|
CdS + amine/ZnS |
TiO2:Al3+/CdS + amine/ZnS/electrolyte/CuS |
2.4 |
Decrease electron–hole recombination |
175
|
CuxS |
CIZS + mp-TiO2/electrolyte/CuxS/electrode |
1.13 |
Null |
176
|
CdSe |
ZnO/CdSe/PbS |
7.9 |
Suppress interface recombination; contribute additional photogenerated carriers |
141
|
NiO |
PbS-EDT/NiO/Au |
10.4 |
Improve hole extraction efficiency; suppress the penetration of moisture and oxygen; good band alignment |
49
|
PbS-EDT |
PbS-TBAI/PbS-EDT/P3HT |
8.7 |
Adjust the valence band; improve charge transfer |
177
|
CsPbBr3 |
PbSe-MPA/CsPbBr3/Au |
7.22 |
Suppress carrier recombination |
178
|
ZnO |
Mg-doped ZnO/ZnO/PbS |
7.06 |
Decrease the interface recombination |
179
|
CdSe |
ZnO/CdSe/P3HT:PCBM |
2.25 |
Increase conductivity; electron transport and hole block |
180
|
CuInS2–ZnS |
TiO2/CuInS2–ZnS/PCDTBT:PC71BM |
7.01 |
Increase the electron extraction; reduce impedance |
181
|
CdSe |
P3HT:PCBM/CdSe/Al |
3.08 |
Down-conversion, reduce charge recombination |
182
|
ZnCdS |
TiO2/ZnCdS/PTB7:PC71BM |
7.75 |
Suppress the recombination; reduce the series resistance |
183
|
SnO2 |
ITO/SnO2/ZnO |
7.16 |
Enhance optical transmission; reduce energy barrier; suppress carrier recombination |
184
|
Ag2Se |
TiO2/Ag2Se/N719 |
5.89 |
Null |
186
|
CdS |
TiO2/CdS/N719 |
7.54 |
Suppress the charge recombination; increase the optical absorption |
187
|
CeO2:Eu3+ |
N719/CeO2:Eu3+/electrolyte |
8.36 |
Down-convert UV light to visible light, light scattering |
190
|
NaGdF4:Eu3+ |
FTO/TiO2/N719/electrolyte/Pt/FTO/NaGdF4:Eu3+ |
9.34 |
Act as luminescent down-conversion centers and light scatterers in the ultraviolet and visible domains |
191
|
CaCe2(MoO4)4:Er3+/Yb3+ |
Electrolyte/CaCe2(MoO4)4:Er3+/Yb3+/Pt |
7.78 |
Convert the NIR and UV radiation to visible emissions |
192
|
NaYF4:20%Yb, 2%Er@NaYF4:7%Eu |
N719/NaYF4:20%Yb,2%Er@NaYF4:7%Eu/Pt |
7.664 |
Convert NIR and UV lights to visible lights |
193
|
SrF2:Pr3+–Yb3+ |
FTO/SrF2:Pr3+–Yb3+/TiO2-N719 |
9.07 |
Absorb blue light and emit green and red light |
194
|
BaWO4:Pr3+ |
TiO2/BaWO4:Pr3+/N719 |
8.08 |
Absorb UV light and emit blue, green, and red light |
195
|
MAPbBr0.9I2.1 |
TiO2/MAPbI3/MAPbBr0.9I2.1 |
13.32 |
Facilitate hole transfer from MAPbI3 to HTL |
196
|
CuInS |
MAPbI3/CuInS/spiro-MeOTAD |
13.8 |
Enhance charge transfer; suppress charge recombination pathways |
198
|
MgO |
FTO/MgO/SnO2 |
18.23 |
Smoother surface; less FTO surface defects; suppressed electron–hole recombination |
199
|
CdS |
TiO2/CdS/MAPbI3 |
10.52 |
Longer electron lifetime; lower charge carrier recombination rate |
200
|
SnO2 |
PC61BM/SnO2/Al |
19.7 |
Block holes; enhance the conductivity; reduce the recombination |
201
|
Cl–SnO2 |
FTO/Cl–SnO2/CsMAFAPbI3Br3−x |
17.3 |
Fill the pinholes; passivate the trapping defects |
202
|
FAPbX3 |
MAPbI3/FAPbX3/C60 |
7.59 |
Enhance absorption |
203
|
PbS |
MAPbI3/PbS/spiro-OMeTAD |
19.24 |
Enhance hole extraction; retard interfacial recombination; improve perovskite film morphology |
204
|
CuOx |
NiOx/CuOx/MAPbI3 |
19.91 |
Lead higher transfer efficiency and lower carrier recombination |
205
|
MAPbBr0.9I2.1 |
SnO2/MAPbBr3/MAPbBr0.9I2.1 |
20.21 |
Optimize the energy level; improve hole extraction |
206
|
Co-CuGaO2 |
Cs0.05(MA0.15FA0.85)0.95Pb(I0.85Br0.15)3/Co-CuGaO2/spiro-OMeTAD |
20.39 |
Reduce the energy gap; prevent direct contact between PVK and oxygen and moisture |
207
|
MoS2:RGO |
MAPbI3/MoS2:RGO/spiro-OMeTAD |
20.12 |
Extract holes and block electrons |
208
|
CsCu5S3 |
MAFAPbI3/CsCu5S3/spiro-OMeTAD |
22.29 |
Favor for energy levels; reduce carrier recombination |
209
|
Cu2−xS@SiO2@Er2O3 |
TiO2/Cu2−xS@SiO2@Er2O3/MAPbI3 |
17.8 |
Upconvert infrared light to visible light |
210
|
NaYF4:Yb3+,Er3+/@NaYF4:Yb3+,Nd3+ |
IR-783 + NaYF4:Yb3+,Er3+/@NaYF4:Yb3+,Nd3+ + Au/CsMAFAPbBrI |
20.5 |
Convert IR to visible light, scatter light |
212
|
MoS2 |
SiOx/MoS2/MoOx |
22.8 |
Provide electron-blocking and hole-extraction properties |
213
|
PbS |
PbS/c-Si solar cell |
12.6 |
Luminescent solar concentrator |
214
|
CdS |
Si solar cell/CdS |
9.37 |
Reduce the reflectance spectral ranging from 250 to 1100 nm, passivation, and down-conversion |
216
|
ZnxCd1−xS–ZnS:Mn |
Si solar cell/ZnxCd1−xS–ZnS:Mn |
14.271 ± 0.268 |
Down-conversion |
217
|
Cd0.5Zn0.5S–ZnS:Mn |
Si solar cell/Cd0.5Zn0.5S–ZnS:Mn |
17.90 |
Down-convert UV light of 250–450 nm to yellow-orange light at 583 nm |
218
|
NaGdF4:Ce@NaGdF4:Nd/Yb@NaYF4 |
c-Si solar cell/NaGdF4:Ce@NaGdF4:Nd/Yb@NaYF4 |
0.8 (under 254 nm illumination) |
Expand the spectrum of quantum cutting in the NIR |
219
|
CsPbCl1.5Br1.5:Yb3+,Ce3+ |
c-Si solar cell/CsPbCl1.5Br1.5:Yb3+,Ce3+ |
21.5 |
Larger absorption cross-section, weaker electron-phonon coupling and higher inner luminescent quantum yield |
220
|
3.1 Suppressing recombination
As shown in Fig. 7, IFL is a separate layer between the charge transport layer/active layer or charge transport layer/electrode. So, it can convincingly act as a functional layer to prevent hole–electron recombination. Ternary semiconductor NCs of PbCdS were deposited on TiO2 as an IFL to impede the direct contact between ETL and the active layer of CdS, thus suppressing recombination efficiently and boosting the device PCE significantly (Fig. 8a). As the same, oxide NCs of MgO and SnO2 film inserted between ETLs and electrodes blocked holes and reduced recombination (Fig. 8b and c).199,201
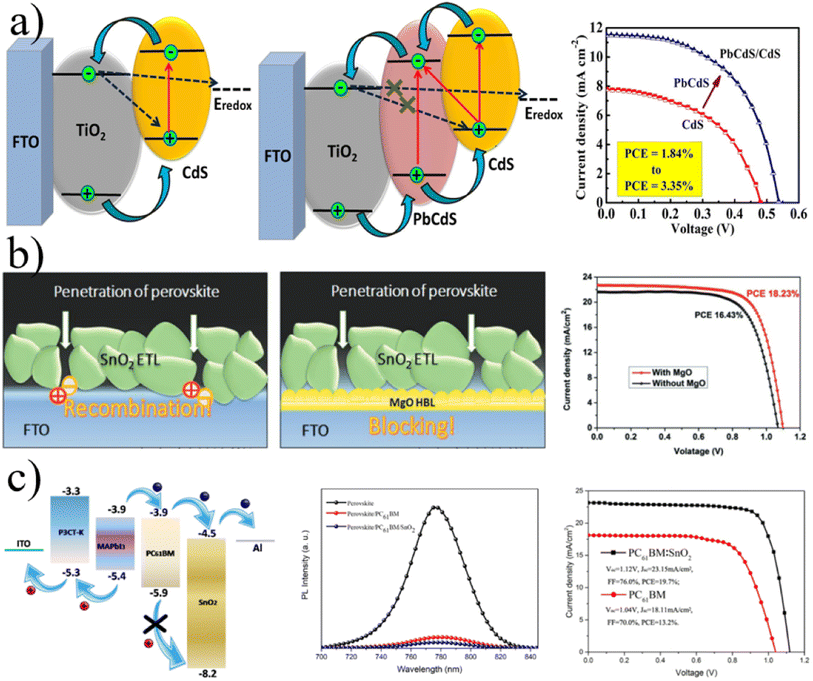 |
| Fig. 8 (a) Schematic illustration of the possible electron transfer process in bare CdS and PbCdS/CdS based solar cells and J–V curves of CdS and PbCdS/CdS based solar cells. (b) The perovskite can directly contact the FTO surface along a shunt pathway in the absence of SnO2 ETLs; the MgO2 layer can inhibit the penetration of perovskite reaching the FTO surface, and the best performance of the PSCs with and without MgO. (c) Corresponding energy level diagram of PSCs, steady-state PL spectra of the perovskite film deposited on the PC61BM layer and PC61BM:SnO2 bilayer, J–V characteristics in the illumination for the devices based on the PC61BM layer and PC61BM:SnO2 bilayer. (a) Adapted with permission.174 Copyright 2017, Elsevier. (b) Adapted with permission.199 Copyright 2017, Wiley-VCH. (c) Adapted with permission.201 Copyright 2018, American Chemical Society. | |
3.2 Charge transport improvement
As we know, charge transport ability inevitably affects the performance of solar cells. As shown in Fig. 9a, MAPbBr0.9I2.1NCs with good energy alignment enhance the hole transport ability and the PCE of the PSCs. Co-CuGaO2 NCs with ∼20 nm size were synthesized by hydrothermal method and used for surface passivation at the interface of perovskite and spiro-OMeTAD. Furthermore, the larger bandgap and lower valence band energy of Co-CuGaO2 reduced the energy gap between Co-CuGaO2 and perovskite. Considering that the reduced energy gap improved hole conduction and electron blocking, the PCE of PSCs was enhanced from 18.60% to 20.39%.207 MoS2NCs were also used to improve hole transport and the device PCE and stability (Fig. 9b).
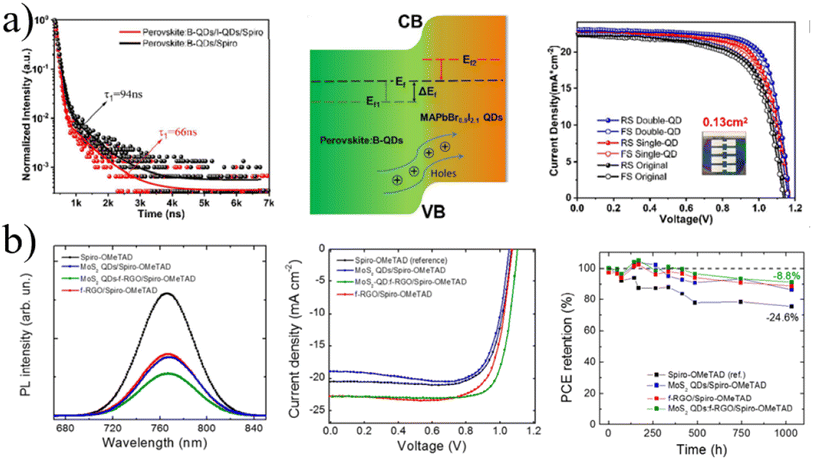 |
| Fig. 9 (a) TRPL spectra of the perovskite film with and without MAPbBrI2 NCs, schematic diagram of band bending in the heterojunction structure formed by the perovskite and MAPbBrI2 NCs. (b) Steady-state PL measurements of MAPbI3 after the deposition of spiro-OMeTAD and different IFL/spiro-OMeTAD, I–V characteristics of tested PSCs using different IFLs, normalized PCE trends vs. time extracted by I–V characteristics under 1 sun illumination, periodically acquired during the shelf life test for the PSCs. (a) Adapted with permission.206 Copyright 2020, American Chemical Society. (b) Adapted with permission.208 Copyright 2018, American Chemical Society. | |
3.3 Light conversion and harvest enhancement
It is known that the active layer of recent solar cells cannot respond to the whole solar spectrum, resulting in energy loss and relatively low efficiency. Broadening the absorption of the active layer is an efficacious strategy to reduce energy loss and enhance the performance of solar cells. CdS NCs with general Stocks shifts were used in Si solar cells for spectral range enhancement.216 In Fig. 10a, we can see that Mn-doped NCs expand the spectral range response of solar cells by absorbing short-wave lights and emitting the characteristic light around 580 nm, suggesting a larger range of light harvesting by active layer and PCE improvement. ZnxCd1−xS/ZnS:Mn2+ NCs were also used to broaden the light response range of Si solar cells.217 Furthermore, Mn-doped semiconductor NCs have a large Stocks shift, avoiding self-absorption and thus reducing energy loss. Sr2CeO4 NCs with down-shifting properties could improve the stability of organic P3HT:PCBM solar cells without significant loss of short-circuit current.185 Certainly, by using NCs with a Stocks shift for expanded light harvesting, the photoluminescence yield and other photoelectronic properties should keep the rules of high-performance solar cells.
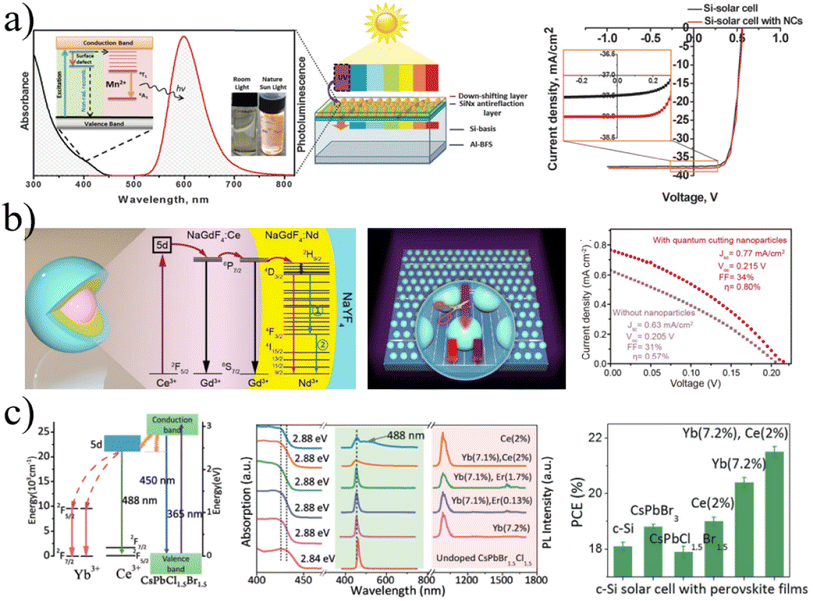 |
| Fig. 10 (a) Scheme of the down-shifting mechanism of the Zn0.5Cd0.5S:Mn (5%)/ZnS NC converter material and the design of proof-of-concept solar cell; J–V curves of the corresponding solar cells. (b) Schematic and proposed energy transfer for Ce3+-sensitized quantum cutting in Nd3+ ions; schematic design for boosting the energy-harvesting efficiency of c-Si solar cells with quantum cutting nanocrystals; comparison of current density–voltage characteristic for c-Si solar cells with and without a nanocrystal coating layer (the solar cells were illuminated with a 254 nm UV lamp at a power density of 7 mW cm−2). (c) Schematic diagram of energy transfer mechanism in the Yb3+, Ce3+ codoped CsPbCl1.5Br1.5NCs, absorption; visible and near-infrared emission spectra of CsPbCl1.5Br1.5 perovskite NCs codoping with different rare earth ions; PCE of Si solar cells with different perovskite NCs. (a) Adapted with permission.217 Copyright 2016, the Royal Society of Chemistry. (b) Adapted with permission.219 Copyright 2017, American Chemical Society. (c) Adapted with permission.220 Copyright 2017, Wiley-VCH. | |
Besides the above NCs, rare elements are good at light conversion due to their special energy levels. Undoped and doped NCs based on rare elements were massively applied to broaden the light response in solar cells and efficiently boost the device performance. As seen in Fig. 10b and c, Yb3+, Ce3+, and Nd3+ based NC layers efficiently convert light and enhance the device performance. Additionally, Eu3+, Er3+, and Pr3+ were also used for efficient solar cells.
3.4 Energy level optimization
To ensure propitious charge transfer among the functional layers in solar cells, un-matched energy levels are a tricky issue that we need to address. One origin of the open-circuit voltage (Voc) loss of NC-LHSC, Si solar cells, PSCs, and OSCs is mainly analyzed quantitatively via the energy difference between bandgap and the Schokley–Queisser limit voltage.15 For n-i-p solar cells, band alignment between the active layer and ETL directly limits the splitting of the quasi-Fermi level. For p-i-n solar cells, the band alignment between HTL and active layer has a great impact on the Voc. The conduct band of recent HTL is relatively deep, and the barrier for electron transport is not sufficient, resulting in electron leakage and reducing the device performance. Therefore, energy level is of great importance to improve the performance of solar cells. Shown in Fig. 11a and b, Co-doped CuGaO2 and SnO2NCs film were used for energy level optimization for high-performance solar cells, illuminating the large potential of NCs as ITL for reducing Voc loss of solar cells.
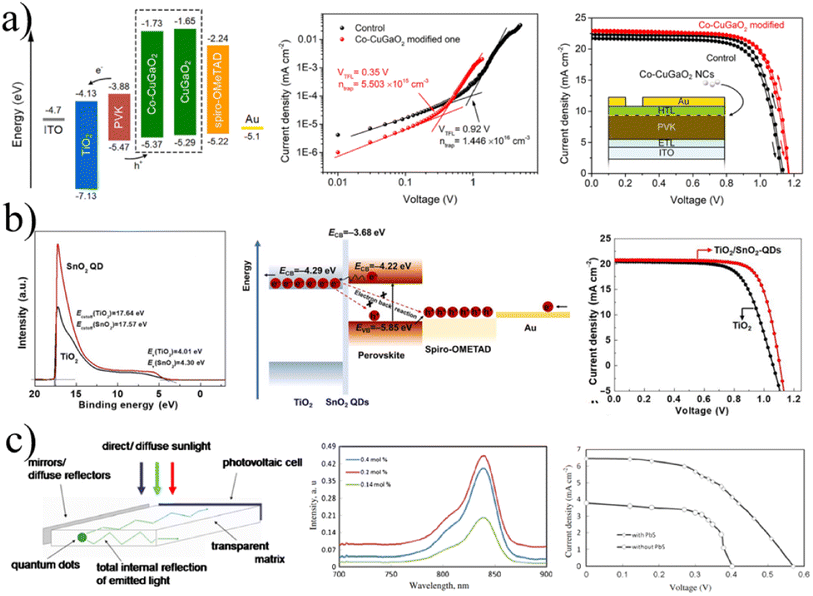 |
| Fig. 11 (a) Energy level diagram of PSCs with the ITO/TiO2/PVK/Co-CuGaO2/spiro-OMeTAD/Au structure, dark I–V curves of devices based on spiro-OMeTAD and Co-CuGaO2/spiro-OMeTAD, forward and reverse scan J–V curves based on spiro-OMeTAD and Co-CuGaO2/spiro-OMeTAD. (b) Band edge alignment and photocarrier dynamics in the resultant device. (c) Schematic illustration of an NC-luminescent solar concentrator, PL spectra of PbS NC-luminescent solar concentrator with different concentrations of PbS, and J–V curves for solar cells with and without PbS NC-luminescent solar concentrator. (a) Adapted with permission.207 Copyright 2022, Elsevier. (b) Adapted with permission.202 Copyright 2019, Elsevier. (c) Adapted with permission.215 Copyright 2015, Springer. | |
3.5 Concentrating luminescent solar radiation
Solar radiation is geographically extensive, but the energy density is not high. So, concentrating solar light for higher density is a feasible strategy to improve solar cell performance. PbS NC luminescent solar concentrator (LSC) was found to show potential advantages over silicon solar cell panels (Fig. 11c). They can reduce the size of solar cells and offer great flexibility in design, which results in cost reduction with any desired shape. One of the attractive LSCs is based on NCS.
3.6 Prevention
The main reason for unstable solar cells is the invasion of oxygen and water. IFL between the active layer and HTL, ETL, or electrode can arrest this invasion to a great degree and increase the stability of solar cells. The above-mentioned IFL of Co-CuGaO2 NCs in Fig. 11a not only acts as a hole transport accelerator but also prevents the direct contact of perovskite with oxygen and moisture, boosting the stability of the PSCs.207
4. Nanocrystals as efficient additives
Besides an independent layer to improve the performance of solar cells, NCs have also been used as additives for boosting active layer quality, carrier transfer acceleration, spectral response broadening due to plasmonic effect, light conversion, light scattering/reflection, heat sinking, and some other functions. Table 4 lists the recent progress of NCs as efficient additives for high-performance solar cells.
Table 4 Recent advances in NC additives for high-performance solar cells
NC |
Added layer |
NC additives and the mixture |
PCE (%) |
Functions |
Ref. |
SnO2–Sb2O3 |
Bottom ETL |
SnO2–Sb2O3 + TiO2 |
7.7 |
①Improve the electron mobility and light harvesting; ②avoid the recombination |
222
|
PbS |
PbS + m-TiO2 |
5.04 |
①Enlarge the grain size of CsPbBr3 perovskite film; ②suppress the activation of intrinsic trap sites of m-TiO2 |
223
|
PbS + TiO2 |
14.95 |
Downshift the conduction band of TiO2, promote the driving force of an electron injection |
227
|
Cu–Zn–In–S–Se (CZISSe) |
CZISSe + mp-TiO2 + CsPbBr3 |
5.37 |
①Enhance charge extraction; ②reduce charge recombination |
225
|
Ho3+–Yb3+–F− tri-doped TiO2 |
Ho3+–Yb3+–F− tri-doped TiO2 + TiO2 |
9.91 ± 0.3 |
Convert NIR light to green light |
232
|
Gd1.54Er0.46(MoO4)3 (GMO:Er) |
GMO:Er + TiO2 |
3.41 |
Convert NIR light to the visible region (near 550 nm) |
233
|
β-NaYF4:Yb3+/Er3+/Sc3+@NaYF4 |
β-NaYF4:Yb3+/Er3+/Sc3+@NaYF4 + TiO2 |
20.19 |
Convert NIR to red and green light |
243
|
SrAl2O4:Eu3+ |
SrAl2O4:Eu3+ + TiO2 |
4.64 |
Elevation of the Fermi energy level of TiO2, improves light harvesting |
245
|
ZnSTe |
Bottom HTL |
ZnSTe + PEDOT:PSS |
2.31 |
Reduce series resistance, increase shunt resistance, improve mobility |
230
|
NaCsWO3@NaYF4@NaYF4:Yb,Er |
Top HTL |
NaCsWO3@NaYF4@NaYF4:Yb,Er + spiro |
18.28 ± 0.34 |
①Widen the perovskite spectral response range; ②increase the light reflection; ③ prolong the light path, and light absorption |
231
|
NaLuF4:Yb,Er@NaLuF4 |
NaLuF4:Yb,Er@NaLuF4 + PTAA |
15.86 |
Convert NIR light to visible light, scatter light |
237
|
Li(Gd,Y)F4:Yb,Er |
Li(Gd,Y)F4:Yb,Er + spiro-MeOTAD |
18.34 |
Convert NIR to visible light |
242
|
SnO2 |
Active layer |
SnO2 + P3HT-PCBM |
3.39 |
Reduce the recombination |
224
|
Fe-doped SnO2 |
Fe-doped SnO2 + P3HT |
3.04 |
Extension of photogenerated exciton lifetime, overcome the burn-in regime faster |
247
|
NaYF4:Yb,Er/NaYF4 |
NaYF4:Yb,Er/NaYF4 + N719 |
9.15 |
Convert NIR light to visible light (450–700 nm) |
235
|
TiO2:Sm3+ |
TiO2:Sm3+ + P25 + N719 |
5.31 |
Convert UV to visible light |
244
|
SnS |
SnS + MAPbI3 |
14.26 |
①Provide more nucleation sites for the growth of perovskite grains; ② accelerate carrier transfer and reduce the recombination |
226
|
NaYF4:Yb/Er |
NaYF4:Yb,Er + MAPbI3 |
17.8 |
Broaden the solar spectral use to NIR light, minimize the non-absorption energy loss |
236
|
IR-806-β-NaYF4:Yb,Er |
IR-806-β-NaYF4:Yb,Er + MAPbI3Er3+–Yb3+ doped Zn |
17.49 |
Convert NIR light (800–1000 nm) to visible emissions |
238
|
Ho3+–Yb3+–Li+-doped TiO2 |
Ho3+–Yb3+–Li+-doped TiO2 + FAMAPbBrI3 |
16.88 ± 0.5 |
Convert NIR to visible light, improve electron injection efficiency, and decrease recombination |
240
|
β-NaYF4:Yb3+,Tm3+@TiO2 |
β-NaYF4:Yb3+,Tm3+@TiO2 + MAPbI3 |
16.27 |
Convert NIR to visible light, serve as the light scatter centers |
241
|
4.1 Active layer quality improvement and carrier transfer accelerator
The performance of solar cells largely depends on the quality of the active layer, such as the purity of Si for Si solar cells and the composition of perovskite for PSCs. For example, defects unavoidably exist at the surface of perovskite thin film during the low-temperature fabrication process, and reducing defects is a very useful way to improve the performance of PSC.254 This subsection discusses NCs as outstanding additives in the active layer for boosting its quality and carrier transfer acceleration.
SnO2–Sb2O3 NCs were used to modify TiO2 nanorod arrays for electron mobility improvement and electron transport resistance reduction. This NCs modified ETL enhanced the PCE of CH3NH3PbI3−xClx PSC from 6.5% to 7.7%.222 PbS NCs suppress the activation of the intrinsic trap sites, provide nucleation sites to enlarge the grain size, and suppress the charge combination in CsPbBr3 PSCs (Fig. 12).223 Adding SnO2 NCs of size around 5 nm into the active layer of P3HT:PC61BM made electrons more easily pass through the active layer and accelerate the electron transfer, improving the PCE of OSC from 2.67% to 3.39%.224 S2O32−-capped Cu–Zn–In–S–Se NCs with ∼5 nm size was introduced in the perovskite precursor of PbBr2 solution to boost 22.6% enhancement of the PCE of inorganic Cs-based PSCs, which was due to promoted crystallization of CsPbBr3 and hole extraction.225 SnS NCs with an average size of 6.9 nm were implanted into the active layer of carbon-based HTL-free mesoporous PSCs, and the device gained a high PCE of 14.26% with a 12.42% improvement. This improvement was demonstrated by more nucleation sites for the growth of perovskite grains and the accelerated carrier transfer.226 PbS NCs doped TiO2 nanotubes (TNTs) modified the electronic and optical properties by downshifting the conduction band of TiO2 ETL from −4.22 to −4.58 eV and promoting the driving force of an electron injection to the conductive electrode.227
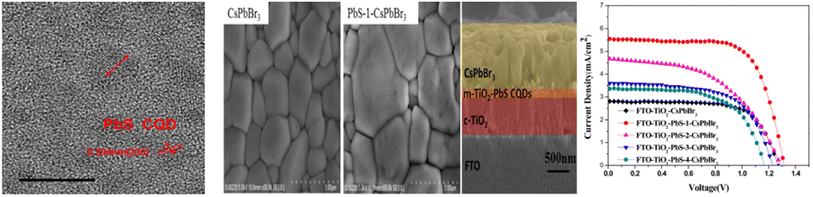 |
| Fig. 12 Top-view and cross-section images of CsPbBr3 film decorated with PbS NCs, and J–V curves of all-inorganic PSCs based on different TiO/PbS photoanodes. Adapted with permission.243 Copyright 2019, American Chemical Society. | |
4.2 Plasmonic Effect
Semiconductor NCs have exhibited localized surface plasmon resonances (LSPR), and this plasmonic effect has been used in many fields, such as solar photovoltaics, in-door energy comfort, water splitting, and so on (Fig. 13). Compared with traditional LSPR materials (noble metals), the semiconductor LSPR NCs allow a wide range of wavelength tunability from visible towards near-infrared (NIR) and further to mid-IR, leading to larger absorption of solar light. Higher absorption of the active layer in solar cells increases the current intensity and thus, the device performance. DSSCs based on plasmonic effect by ZnO or SnO2 and TiO2/SnO2 have been investigated.254 From Fig. 13, it can be seen that different oxides, sulfides, and selenides such as MoO3−x, CsxWO3, TiO2−x, CdO1−x, doped In2O3, doped ZnO, Cu2−xS, CuxInyS2, Cu2−xSe, etc. broaden the response spectra from 500 nm to nearly 3800 nm. Effective light harvesting due to the plasmonic effect shows great potential in solar cell application. Given the limited self-absorption bands of solar cells, the above oxide and sulfide NCs can be applied as additives to widen the light harvesting range. Clearly, this will reduce energy loss and raise the device performance in a considerable way.
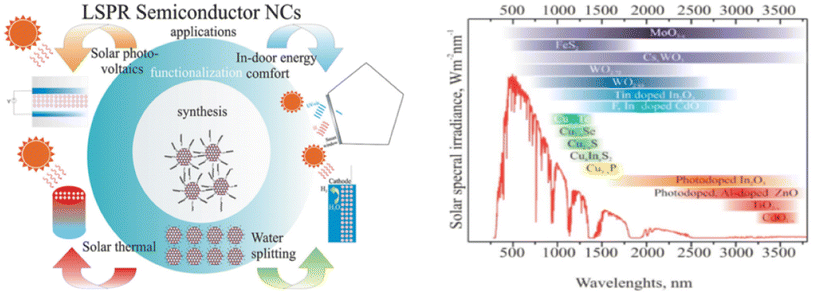 |
| Fig. 13 Schematic of the applications of LSPR semiconductor NCs; the solar spectrum and its relevancy for energy-targeted applications of LSPR semiconductor NCs via their tunable plasmon absorbance. Adapted with permission.255 Copyright 2021, Elsevier. | |
4.3 Light conversion
As discussed in Section 3, light conversion is an efficient way to boost the PCE and stability of solar cells. Based on this point, NC light-converting layers were investigated in the previous part. It is also true that light conversion can be realized by NC additives, which will be summarized in this part.
4.3.1 Up-conversion.
Rare-earth (RE) elements are famous for light up-conversion and further application in solar cells. RE element-doped semiconductor NCs have been added as additives in active layers and charge transport layers for high-performance DSSCs and PSCs. NIR lights were up-converted to visible lights and thus elevated the device PCE due to wider solar radiation absorption (Fig. 14). The RE element-based NCs, which can be used in up-converting materials as additives in solar cells, mainly contain Yb, Er, Ho, and Sc doped materials like Ho–Yb–F doped TiO2, Er–Yb doped ZnO2, Ho–Yb doped Gd2O3, Yb–Er doped NaYF4 and Yb–Er doped Li(Gd,Y)F4. As shown in Fig. 14, the schematic energy diagram for Yb3+ and Er3+ are suitable for up-conversion and the related solar cells gain high efficiency. The up-conversion NCs can be added in the active layer, ETL, and HTL in different solar cells like OSC, DSSC, and PSC. The original active layers cannot absorb the whole range of sunlight and thus result in energy loss. After assembling NCs with up-conversion ability, the non-responsive long-wavelength range of sunlight will be converted to shorter wavelengths and absorbed by active layers to re-generate hole–electron pairs. This strategy can enhance the utilization of the infrared range of sunlight and the performance of solar cells.
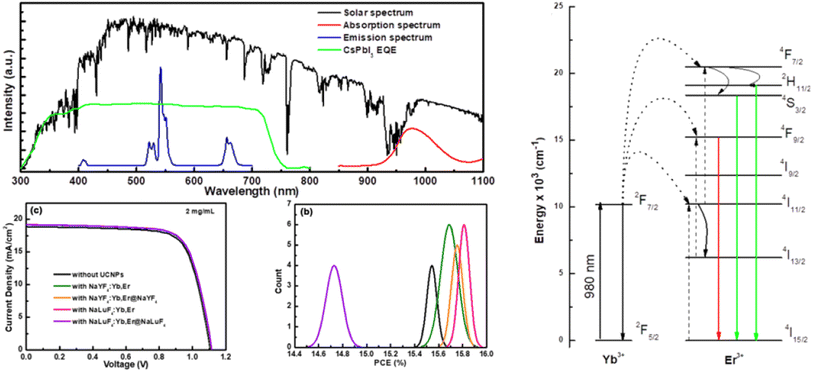 |
| Fig. 14 Solar spectrum, absorption and emission spectrum of up-conversion nanocrystals and EQE curve of γ-CsPbI3 PSC, J–V curves of PSCs based on different nanocrystals, and statistical PCE distribution histograms of 30 devices, schematic energy diagram for Yb3+ and Er3+. Adapted with permission.237 Copyright 2019, American Chemical Society. | |
4.3.2 Down-conversion and down-shifting.
Down-conversion and down-shifting NCs are advantageous for high-performance solar cells due to the efficient utilization of UV lights. Typical Sm3+-based TiO2NCs were used in DSSCs and obvious improvement of PCE was gained (Fig. 15) through converting ultraviolet to visible light. Better performance of solar cells was obtained by means of down-converting NCs such as ZnS:Er in Si-based devices and CeO2:Gd in OSC.249,250 The shortest wavelength response by perovskite is about 400 nm.256 The energy of sunlight with wavelengths shorter than 400 nm will be wasted. Furthermore, the UV lights can damage the perovskite or organic active layer and reduce the device stability. So, the PCE and light stability of solar cells can be increased by using down-conversion or down-shifting NCs.
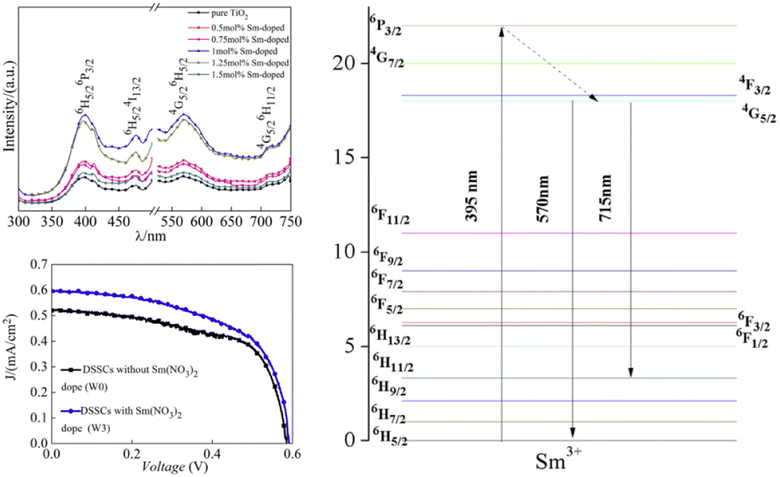 |
| Fig. 15 Excitation spectrum (λem = 567 nm) and emission spectrum (λex = 395 nm) of TiO2:Sm3+ NCs with different Sm3+ doping concentrations, and schematic energy-level diagrams to show the details of down-conversion mechanisms via excitation using 395 nm radiation for TiO2:Sm3+ NCs. Adapted with permission.244 Copyright 2016, Elsevier. | |
4.4 Light scattering and reflection
Light scattering and reflection are well-known for boosting the optical absorption of different solar cells. TiO2:Zn NCs can scatter light and promote the performance of conventional DSSCs.257 Due to the ultralow (<1%) photoluminescence quantum yield, NaLuF4:Yb,Er@NaLuF4NCs acted as scattering centers and extended the sunlight optical path by combining scattering and reflecting sunlight.237 NaYF4:Yb3+,Tm3+ NCs serve as scatter centers to enhance light harvesting for PSCs.241 We can conclude that NCs are good at light absorption enhancement due to light scattering and reflecting, and this is an available approach for improving the device performance.
4.5 Heat sinks
Except for optical management, heat control is also important for solar cell operation because elevated temperatures may increase energy loss and destroy the devices. In the traditional photovoltaic/thermal (PV/T) system, the temperature of thermal energy is always limited by the operation temperature of PV cells. The oleylamine solution of Cu9S5NCs was adopted in the spectral splitting filter to harvest the moderate-temperature heat. After successful thermal energy collection, the maximum overall efficiency of the present PV/T collector is 34.2%, with a 17.9% improvement.258 In a concentrator photovoltaic nanocrystal-phase change material (PCM) hybrid system, Al2O3, CuO, and SiO2 were used to save energy and offer safe operating conditions. Compared with pure PCM (0 wt%), Al2O3-PCM at 5 wt% increased the thermal conductivity, and the melting rate reduced the solar cell temperature. The electrical efficiency was improved from 6.36% to 8% and gained temperature uniformity from 20 °C to 12 °C. This strategy would be recommended for residential and industrial applications in solar cells.259
4.6 Other functions
ZnSTe NCs with an average of 2.96 nm in the active layer demonstrated increased photo-generation and improved efficiency by reduced series resistance and improved mobility.230 Fe-doped SnO2 NCs incorporated into the active layer of P3HT:PCBM improved the Jsc of OSC due to the extension of photogenerated exciton lifetime as a result of the magnetic field. Meanwhile, these NC-reinforced devices showed the tendency to overcome the burn-in regime faster and indicated the diluted magnetic semiconductor NCs had the potential to increase the stability of the devices.247
5. Summary and outlook
In recent years, NCs as functional layers and additives have been widely used in solar cells, significantly enhancing their performance. Here, we summarize NCs-based HTL, ETL, IFL, and additives for solar cells. NCs can boost the device performance in many ways, such as increasing the charge transport ability, suppressing charge recombination, broadening light harvest, and so on.
Based on previous investigations, we propose some promising strategies to enhance the performance of solar cells by using NCs.
(I) Optical management. Full spectrum absorption under low-cost conditions: both up-converting and down-shifting materials. For down-shifting, doped NCs with large Stokes shift, such as Mn or Cu doped NCs, have great potential due to no self-absorption, facile synthesis, and low cost. Cu+, Ag+ doped n-type metal oxide NCs,260 Fe1−xS2 NCs,261 and In doped CuxS NCs,262 with great potential for spectra broadening are also suggested for high-performance solar cells. CaMoO4:Er3+,Yb3+ NCs would offer great potential for conserving energy in Si solar cells.263 Certainly, the photoluminescence efficiency of NCs is very important when they are used to convert light in solar cells. Excellent optical management of solar cells can utilize more sunlight and improve the device performance.
(II) Electronic optimization. The charge transfer ability is mainly determined by the electronic properties of charge transport materials. The performance of solar cells can be improved by electronic optimization. One approach is a component change of materials such as n doping for n-type NCs and p doping for p-type NCs. Finding more suitable dopants for NCs will further boost the PCE and stability of solar cells. Meanwhile, the size and ligand control of NCs are also considered to optimize their electronic properties and fabricate better-performance solar cells.
(III) Interface engineering. Interfacial layers between different functional layers show different functions in solar cells. In further developments, more NCs IFL will be used to improve device performance by preventing direct contact between the active layer and charge transport layer, impeding the entry of water and oxygen, and protecting and destroying the active layer with UV lights.264 So, NC IFL, with good photoelectronic properties, can adjust energy alignment, accelerate charge transport, enhance light harvest, and protect the active layer.
(IV) Cross utilization. The metal–organic framework (MOF) materials can improve the efficiency and stability of solar cells due to their unique properties.265 NCs with small sizes can be considered to mix with MOF and enhance the performance of devices. In addition, NCs can be utilized as light harvesters, HTL, ETL, and IFL, so we suggest their application in all-NC solar cells.
Conflicts of interest
The authors declare no conflict of interest.
Acknowledgements
This work was supported by the Natural Science Foundation of the Guizhou Province (No. 2071). The authors thank Prof. Ma Wanli, Liu Zeke, and Dr Lu Huiyuan at Soochow University for their helpful discussion and suggestions for improving the manuscript.
References
- I. J. Kramer and E. H. Sargent, The architecture of colloidal quantum dot solar cells: Materials to devices, Chem. Rev., 2014, 114, 863 CrossRef CAS PubMed.
- G. H. Carey, A. L. Abdelhady, Z. Ning, S. M. Thon, O. M. Bakr and E. H. Sargent, Colloidal quantum dot solar cells, Chem. Rev., 2015, 115, 12732 CrossRef CAS PubMed.
- K. R. Ryan, M. P. Down, N. J. Hurst, E. M. Keefe and C. E. Banks, Additive manufacturing (3D printing) of electrically conductive polymers and polymer nanocomposites and their applications, eScience, 2022, 2, 365–381 CrossRef.
- M. J. Yuan, M. X. Liu and E. H. Sargent, Colloidal quantum dot solids for solution-processed solar cells, Nat. Energy, 2016, 1, 16016 CrossRef CAS.
- J. H. Jing, Y. J. Dou, S. H. Chen, K. Zhang and F. Huang, Solution sequential deposited organic photovoltaics: From morphology control to large-area modules, eScience, 2023, 3, 100142 CrossRef.
- Z. K. Liu, J. Y. Yuan, S. A. Hawks, G. Z. Shi, S.-T. Lee and W. L. Ma, Photovoltaic devices based on colloidal PbX quantum dots: Progress and prospects, Sol. RRL, 2017, 1, 1600021 CrossRef.
- Z. X. Pan, H. S. Rao, I. Mora-Seró, J. Bisquert and X. H. Zhong, Quantum dot-sensitized solar cells, Chem. Soc. Rev., 2018, 47, 7659–7702 RSC.
- S. Aftab, M. Z. Iqbal, S. Hussain, F. Kabir, A. A. Al-Kahtani and H. H. Hegazy, Quantum junction solar cells: Development and prospects, Adv. Funct. Mater., 2023, 33, 2303449 CrossRef CAS.
- A. Shrestha, M. Batmukh, A. Tricoli, S. Qiao and S. Dai, Near-infrared active lead chalcogenide quantum dots: Preparation, post-synthesis ligand exchange, and applications in solar cells, Angew. Chem., Int. Ed., 2019, 58, 5202–5224 CrossRef CAS PubMed.
- A. O. El-Ballouli, O. M. Bakr and O. F. Mohammed, Compositional, processing, and interfacial engineering of nanocrystal-and quantum-dot-based perovskite solar cells, Chem. Mater., 2019, 31, 6387–6411 CrossRef CAS.
- G. S. Selopal, H. Zhao, Z. M. Wang and F. Rosei, Core/shell quantum dots solar cells, Adv. Funct. Mater., 2020, 30, 1908762 CrossRef CAS.
- J. Y. Yuan, A. Hazarika, Q. Zhao, X. F. Ling, T. Moot, W. L. Ma and J. M. Luther, Metal halide perovskite in quantum dot solar cells: Progress and prospect, Joule, 2020, 4, 1160–1185 CrossRef CAS.
- F. G. Zhou, Z. Z. Li, H. Y. Chen, Q. Wang, L. M. Ding and Z. W. Jin, Application of perovskite nanocrystals (NCs)/quantum dots (QDs) in solar cells, Nano Energy, 2020, 73, 104757 CrossRef CAS.
- M. Albaladejo-Siguan, E. C. Baird, D. Becker-Koch, Y. X. Li, A. L. Rogach and Y. Vaynzof, Stability of quantum dots solar cells: A matter of (life) time, Adv. Energy Mater., 2021, 11, 2003457 CrossRef CAS.
- J. W. Liu, K. H. Xian, L. Ye and Z. H. Zhou, Open-circuit voltage loss in lead chalcogenide quantum dot solar cell, Adv. Mater., 2021, 33, 2008115 CrossRef CAS PubMed.
- S. Liang, M. Y. Zhang, M. Biesold, W. Choi, Y. J. He, Z. L. Li, D. F. Shen and Z. Q. Lin, Recent advances in synthesis, properties, and applications of metal halide perovskite nanocrystals/polymer nanocomposites, Adv. Mater., 2021, 33, 2005888 CrossRef CAS PubMed.
- T. Kim, S. Lim, S. Yun, S. Jeong, T. Park and J. Choi, Design strategy of quantum dot thin-film solar cells, Small, 2020, 16, 2002460 CrossRef CAS PubMed.
- T. Blachowicz and A. Ehrmann, Recent development of solar cells from PbS colloidal quantum dots, Appl. Sci., 2020, 10, 1743 CrossRef CAS.
- Y. Liu, G. Z. Shi, Z. K. Liu and W. L. Ma, Toward printable solar cells based on PbX colloidal quantum dot inks, Nanoscale Horiz., 2021, 6, 8–23 RSC.
- Y. Liu, F. Li, G. Z. Shi, Z. K. Liu, X. F. Lin, Y. Shi, Y. F. Chen, X. Meng, Y. Lv, W. Deng, X. Q. Pan and W. L. Ma, PbSe quantum dot solar cells based on directly synthesized semiconductive inks, ACS Energy Lett., 2020, 5, 3797–3803 CrossRef CAS.
- Y. Li, F. Yang, Y. J. Wang, G. Z. Shi, Y. M. Maung, J. Y. Yuan, S. J. Huang and W. L. Ma, Magnetron sputtered SnO2 constituting double electron transport layers for efficient PbS quantum dot solar cells, Sol. RRL, 2020, 4, 2000218 CrossRef CAS.
- Y. N. Zhang, Y. Y. Kan, K. Gao, M. F. Gu, Y. Shi, X. L. Zhang, Y. Xue, X. N. Zhang, Z. K. Liu, Y. Zhang, J. Y. Yuan, W. L. Ma and A. K.-Y. Jen, Hybrid quantum dot/organic heterojunction: A route to improve open-circuit voltage in PbS colloidal quantum dot solar cells, ACS Energy Lett., 2020, 5, 2335–2342 CrossRef CAS.
- J. Khan, X. L. Zhang, J. Y. Yuan, Y. Wang, G. Z. Shi, R. Patterson, J. W. Shi, X. F. Ling, L. Hu, T. Wu, S. Y. Dai and W. L. Ma, Tuning the surface-passivating ligand anchoring position enables phase robustness in CsPbI3 perovskite quantum dot solar cells, ACS Energy Lett., 2020, 5, 3322–3329 CrossRef CAS.
- X. F. Ling, J. Y. Yuan, X. L. Zhang, Y. L. Qian, S. M. Zakeeruddin, B. W. Larson, Q. Zhao, J. W. Shi, J. C. Yang, K. Ji, Y. N. Zhang, Y. J. Wang, C. Y. Zhang, S. Duhm, J. M. Luther, M. Grätzel and W. L. Ma, Guanidinium-assisted surface matrix engineering for highly efficient perovskite quantum dot photovoltaics, Adv. Mater., 2020, 32, 2001906 CrossRef CAS.
- K. Ji, J. B. Yuan, F. C. Li, Y. Shi, X. F. Ling, X. L. Zhang, Y. N. Zhang, H. Y. Lu, J. Y. Yuan and W. L. Ma, High-efficiency perovskite quantum dot solar cells benefiting from a conjugated polymer-quantum dot bulk heterojunction connecting layer, J. Mater. Chem. A, 2020, 8, 8104–8112 RSC.
- X. L. Zhang, Y. L. Qian, X. F. Ling, Y. Wang, Y. N. Zhang, J. W. Shi, Y. Shi, J. Y. Yuan and W. L. Ma, α-CsPbBr3 perovskite quantum dots for application in semitransparent photovoltaics, ACS Appl. Mater. Interfaces, 2020, 12, 27307–27315 CrossRef CAS PubMed.
- S. Lim, J. Kim, J. Y. Park, J. Min, S. Yun, T. Park, Y. Kim and J. Choi, Suppressed degradation and enhanced performance of CsPbI3 perovskite quantum dot solar cells via engineering of electron transport layers, ACS Appl. Mater. Interfaces, 2021, 13, 6119–6129 CrossRef CAS PubMed.
- S. Manzhos, C. C. Chueh, G. Giorgi, T. Kubo, G. Saianand, J. Lüder and M. Ihara, Materials design and optimization for next-generation solar cell and light-emitting technologies, J. Phys. Chem. Lett., 2021, 12, 4638–4657 CrossRef CAS PubMed.
- Y. Li, J. Zhu, Y. Huang, J. F. Wei, F. Liu, Z. P. Shao, L. H. Hu, S. H. Chen, S. F. Yang, J. W. Tang, J. X. Yao and S. Y. Dai, Efficient inorganic solid solar cells composed of perovskite and PbS quantum dots, Nanoscale, 2015, 7, 9902–9907 RSC.
- J. Choi, Y. Kim, J. W. Jo, J. Kim, B. Sun, G. Walters, F. P. García de Arquer, R. Quintero-Bermudez, Y. Li, C. S. Tan, L. N. Quan, A. P. T. Kam, S. Hoogland, Z. H. Lu, O. Voznyy and E. H. Sargent, Chloride passivation of ZnO electrodes improves charge extraction in colloidal quantum dot photovoltaics, Adv. Mater., 2017, 29, 1702350 CrossRef PubMed.
- J. C. An, X. C. Yang, W. H. Wang, J. J. Li, H. X. Wang, Z. Yu, C. H. Gong, X. N. Wang and L. C. Sun, Stable and efficient PbS colloidal quantum dot solar cells incorporating low-temperature processed carbon paste counter electrodes, Sol. Energy, 2017, 158, 28–33 CrossRef CAS.
- X. L. Zhang, P. K. Santra, L. Tian, M. B. Johansson, H. Rensmo and E. M. J. Johansson, Highly efficient flexible quantum dot solar cells with improved electron extraction using MgZnO nanocrystals, ACS Nano, 2017, 11, 8478–8487 CrossRef CAS PubMed.
- J. Yang, J. Lee, J. Lee and W. Yi, Oxygen annealing of the ZnO nanoparticle layer for the high-performance PbS colloidal quantum-dot photovoltaics, J. Power Sources, 2019, 421, 124–131 CrossRef CAS.
- C. Mahajan, A. Sharma and A. K. Rath, Solution-phase hybrid passivation for efficient infrared-band gap quantum dot solar cells, ACS Appl. Mater. Interfaces, 2020, 12, 49840–49848 CrossRef CAS PubMed.
- Y. Y. Wei, M. B. Xing, D. D. Wang and R. X. Wang, Current density improvement of colloidal PbS quantum dot solar cells by adding NaCl in ZnO electron transport layer, Energy Rep., 2020, 6, 2370–2375 CrossRef.
- M. B. Xing, Y. Y. Wei, D. D. Wang, Q. Shen and R. X. Wang, Mg-doped ZnO layer to enhance electron transporting for PbS quantum dot solar cells, Curr. Appl Phys., 2021, 21, 14–19 CrossRef.
- M. Biondi, M.-J. Choi, S. Lee, K. Bertens, M. Y. Wei, A. R. Kirmani, G. Lee, H. T. Kung, L. J. Richter, S. Hoogland, Z.-H. Lu, F. P. García de Arquer and E. H. Sargent, Control over ligand exchange reactivity in hole transport layer enables high-efficiency colloidal quantum dot solar cells, ACS Energy Lett., 2021, 6, 468–476 CrossRef CAS.
- S. Q. Liu, L. Hu, S. J. Huang, W. Q. Zhang, J. J. Ma, J. C. Wang, X. W. Guan, C.-H. Lin, J. Kim, T. Wan, Q. Lei, D. W. Chu and T. Wu, Enhancing the efficiency and stability of PbS quantum dot solar cells through engineering an ultrathin NiO nanocrystalline interlayer, ACS Appl. Mater. Interfaces, 2020, 12, 46239–46246 CrossRef CAS PubMed.
- M.-J. Choi, F. P. García de Arquer, A. H. Proppe, A. Seifitokaldani, J. Choi, J. Kim, S.-W. Baek, M. X. Liu, B. Sun, M. Biondi, B. Scheffel, G. Walters, D.-H. Nam, J. W. Jo, O. Ouellette, O. Voznyy, S. Hoogland, S. O. Kelley, Y. S. Jung and E. H. Sargent, Cascade surface modification of colloidal quantum dot inks enables efficient bulk homojunction photovoltaics, Nat. Commun., 2020, 11, 103 CrossRef CAS PubMed.
- A. Sharma, N. V. Dambhare, J. Bera, S. Sahu and A. K. Rath, Crack-free conjugated PbS quantum dot-hole transport layers for solar cells, ACS Appl. Nano Mater., 2021, 4, 4016–4025 CrossRef CAS.
- M. Biondi, M.-J. Choi, O. Ouellette, S.-W. Baek, P. Todorović, B. Sun, S. Lee, M. Y. Wei, P. C. Li, A. R. Kirmani, L. K. Sagar, L. J. Richter, S. Hoogland, Z.-H. Lu, F. P. García de Arquer and E. H. Sargent, A chemically orthogonal hole transport layer for efficient colloidal quantum dot solar cells, Adv. Mater., 2020, 32, 1906199 CrossRef CAS PubMed.
- Z. L. Teh, L. Hu, Z. L. Zhang, A. R. Gentle, Z. H. Chen, Y. J. Gao, L. Yuan, Y. C. Hu, T. Wu, R. J. Patterson and S. J. Huang, Enhanced power conversion efficiency via hybrid ligand exchange treatment of p-type PbS quantum dots, ACS Appl. Mater. Interfaces, 2020, 12, 22751–22759 CrossRef CAS.
- H. B. Wang, M. Desbordes, Y. Xiao, T. Kubo, T. Bessho, J. Nakazaki and H. Segawa, Highly stable interdigitated PbS quantum dot and ZnO nanowire solar cells with an automatically embedded electron-blocking layer, ACS Appl. Energy Mater., 2021, 4, 5918–5926 CrossRef CAS.
- G. Z. Shi, H. B. Wang, Y. H. Zhang, C. Cheng, T. S. Zhai, B. T. Chen, X. Y. Liu, R. Jono, X. N. Mao, Y. Liu, X. L. Zhang, X. F. Ling, Y. N. Zhang, X. Meng, Y. F. Chen, S. Duhm, L. Zhang, T. Li, L. Wang, S. Y. Xiong, T. Sagawa, T. Kubo, H. Segawa, Q. Shen, Z. K. Liu and W. L. Ma, The effect of water on colloidal quantum solar cells, Nat. Commun., 2021, 12, 4381 CrossRef CAS PubMed.
- X. K. Yang, J. Yang, M. I. Ullah, Y. Xia, G. J. Liang, S. Wang, J. B. Zhang, H.-Y. Su, H. S. Song and J. Tang, Enhanced passivation and carrier collection in ink-processed PbS quantum dot solar cells via a supplementary ligand strategy, ACS Appl. Mater. Interfaces, 2020, 12, 42217–42225 CrossRef CAS PubMed.
- K. Bertens, J. Z. Fan, M. Biondi, A. S. Rasouli, S. Leee, P. C. Li, B. Sun, S. Hoogland, F. P. García de Arquer, Z.-H. Lu and E. H. Sargent, Colloidal quantum dot solar cell band alignment using two-step ionic doping, ACS Mater. Lett., 2020, 2, 1583–1589 CrossRef CAS.
- D. Becker-Koch, M. Albaladejo-Siguan, Y. J. Hofstetter, O. Solomeshch, D. Pohl, B. Rellinghaus, N. Tessler and Y. Vaynzof, Doped organic hole extraction layers in efficient PbS and AgBiS2 quantum dot solar cells, ACS Appl. Mater. Interfaces, 2021, 12, 18750–18757 CrossRef PubMed.
- A. Chiu, E. Rong, C. Bambini, Y. D. Lin, C. C. F. Lu and S. M. Thon, Sulfur-infused hole transport materials to overcome performance-limiting transport in colloidal quantum dot solar cells, ACS Energy Lett., 2020, 5, 2897–2904 CrossRef CAS.
- O. Ouellette, A. Lesage-Landry, B. Scheffel, S. Hoogland, F. P. García de Arquer and E. H. Sargent, Spatial collection in colloidal quantum dot solar cell, Adv. Funct. Mater., 2020, 30, 1908200 CrossRef CAS.
- L. Hu, Q. Lei, X. W. Guan, R. Patterson, J. Y. Yuan, C.-H. Lin, J. Kim, X. Geng, A. Younis, X. X. Wu, X. F. Liu, T. Wan, D. W. Chu, T. Wu and S. J. Huang, Optimizing surface chemistry of PbS colloidal quantum dot for highly efficient and stable solar cells via chemical binding, Adv. Sci., 2021, 8, 2003138 CrossRef CAS PubMed.
- L. Hu, X. Geng, S. Singh, J. J. Shi, Y. C. Hu, S. Y. Li, X. W. Guan, T. Y. He, X. N. Li, Z. X. Cheng, R. Patterson, S. J. Huang and T. Wu, Synergistic effect of electron transport layer and colloidal quantum dot solid enable PbSe quantum dot solar cell achieving over 10% efficiency, Nano Energy, 2019, 64, 103922 CrossRef CAS.
- M. H. Zhu, X. X. Liu, S. S. Liu, C. Chen, J. G. He, W. W. Liu, J. Yang, L. Gao, G. D. Niu, J. Tang and J. B. Zhang, Efficient PbSe colloidal quantum dot solar cells using SnO2 as a buffer layer, ACS Appl. Mater. Interfaces, 2019, 12, 2566–2571 CrossRef PubMed.
- M. Albaladejo-Siguan, D. Becker-Koch, A. D. Taylor, Q. Sun, V. Lami, P. G. Oppennheimer, F. Paulus and Y. Vaynzof, Efficient and stable PbS quantum dot solar cells by triple-cation perovskite passivation, ACS Nano, 2020, 14, 384–393 CrossRef CAS PubMed.
- A. R. Kirmani, F. García de Arquer, J. Z. Fan, J. I. Khan, G. Walters, S. Hoogland, N. Wehbe, M. M. Said, S. Barlow, F. Laquai, S. R. Marder, E. H. Sargent and A. Amassian, Molecular doping of the hole-transporting layer for efficient, single-step-deposited colloidal quantum dot photovoltaics, ACS Energy Lett., 2017, 2, 1952–1959 CrossRef CAS.
- C. Chen, L. Wang, L. Gao, D. Nam, D. B. Li, K. H. Li, Y. Zhao, C. Ge, H. Cheong, H. Liu, H. S. Song and J. Tang, 6.5% certified efficiency Sb2Se3 solar cells using PbS colloidal quantum dot film as hole-transporting layer, ACS Energy Lett., 2017, 2, 2125–2132 CrossRef CAS.
- J. Choi, M.-J. Choi, J. Kim, F. Dinic, P. Todorovic, B. Sun, M. Y. Wei, S. Hoogland, F. P. García de Arquer, O. Voznyy and E. H. Sargent, Stabilizing surface passivation enables stable operation of colloidal quantum dot photovoltaic devices at maximum power point in an air ambient, Adv. Mater., 2020, 32, 1906497 CrossRef CAS.
- M. Lv, J. Zhu, Y. Huang, Y. Li, Z. P. Shao, Y. F. Xu and S. Y. Dai, Colloidal CuInS2 quantum dot as inorganic hole-transporting material in perovskite solar cells, ACS Appl. Mater. Interfaces, 2015, 7, 17482–17488 CrossRef CAS PubMed.
- Q. H. Li, J. K. Bai, T. T. Zhang, C. Nie, J. L. Duan and Q. W. Tang, CdZnSe@ZnSe colloidal alloy quantum dots for high-efficiency all-inorganic perovskite solar cells, Chem. Commun., 2018, 54, 9575–9578 RSC.
- M. Yuan, X. M. Zhang, J. Kong, W. H. Zhou, Z. J. Zhou, Q. W. Tian, Y. N. Meng, S. X. Wu and D. X. Kou, Controlling the band gap to improve open-circuit voltage in metal chalcogenide based perovskite solar cells, Electrochim. Acta, 2016, 215, 374–379 CrossRef CAS.
- Y. Li, Z. W. Wang, D. Ren, Y. H. Liu, A. B. Zheng, S. M. Zakeeruddin, X. D. Dong, A. Hagfeldt, M. Grätzel and P. Wang, SnS quantum dots as hole transporter of perovskite solar cells, ACS Appl. Energy Mater., 2019, 2, 3822–3829 CrossRef CAS.
- Y. Zhang, Z. L. Zhang, Y. Y. Liu, Y. F. Liu, H. P. Gao and Y. L. Mao, An inorganic hole-transport material of CuInSe2 for stable and efficient perovskite solar cells, Org. Electron., 2019, 67, 168–174 CrossRef CAS.
- F. Li, J. H. Wei, G. Q. Liao, C. Y. Guo, Y. Huang, Q. Zhang, X. Jin, S. Q. Jiang, Q. W. Tang and Q. H. Li, Quaternary quantum dots with gradient valence band for all-inorganic perovskite solar cells, J. Colloid Interface Sci., 2019, 549, 33–41 CrossRef CAS PubMed.
- Y. L. Liu, X. Z. Zhao, Z. F. Yang, Q. T. Li, W. Wei, B. Hu and W. Chen, Cu12Sb4S13 quantum dots with ligand exchanges as hole transport materials in all-inorganic perovskite CsPbI3 quantum dot solar cells, ACS Appl. Energy Mater., 2020, 3, 3521–3529 CrossRef CAS.
- M. Hedariramsheh, M. Mirhosseini, K. Abdizadeh, S. M. Mahdavi and N. Taghavinia, Evaluating Cu2SnS3 nanoparticle layers as hole-transporting materials in perovskite solar cells, ACS Appl. Energy Mater., 2021, 4, 5560–5573 CrossRef.
- W. B. Ma, Z. L. Zhang, M. G. Ma, Y. F. Liu, G. C. Pan, H. P. Gao and Y. L. Mao, CuGaS2 quantum dots with controlled surface defects as an hole-transport material for high-efficient and stable perovskite solar cells, Sol. Energy, 2020, 211, 55–61 CrossRef CAS.
- Y. Y. Liu, Z. L. Zhang, H. P. Gao, H. F. Zhang and Y. L. Mao, A novel inorganic hole-transporting material of CuInS2 for perovskite solar cells with high efficiency and improved stability, Org. Electron., 2019, 75, 105430 CrossRef CAS.
- A. Kotta and H. K. Seo, Nickel oxide monodispersed quantum dots as hole transport layer in n-i-p hybrid perovskite solar cells, J. Nanoelectron. Optoelectron., 2019, 14, 895–899 CrossRef CAS.
- K. C. Icli and M. Ozenbas, Fully metal oxide charge selective layers for n-i-p perovskite solar cells employing nickel oxide nanoparticles, Electrochim. Acta, 2018, 263, 338–345 CrossRef CAS.
- S. Chakrabarti, D. Carolan, B. Alessi, P. Maguire, V. Svrcek and D. Mariotti, Microplasma-synthesized ultra-small NiO nanocrystals, a ubiquitous hole transport material, Nanoscale Adv., 2019, 1, 4915–4925 RSC.
- K. Im, J. H. Heo, S. H. Im and J. Kim, Scalable synthesis of Ti-doped MoO2 nanoparticle-hole-transporting-material with high moisture stability for CH3NH3PbI3 perovskite solar cells, Chem. Eng. J., 2017, 330, 698–705 CrossRef CAS.
- A. Bashir, S. Shukla, J. H. Lew, S. Shukl, A. Bruno, D. Gupta, T. Baikie, R. Patidar, Z. Akhter, A. Priyadarshi, N. Mathews and S. G. Mhaisalkar, Spinel Co3O4 nanomaterials for efficient an stable large area cabon-based printed perovskite solar cells, Nanoscale, 2018, 10, 2341–2350 RSC.
- C. Liu, X. Y. Zhou, S. M. Chen, X. Z. Zhao, S. Y. Dai and B. M. Xu, Hydrophobic Cu2O quantum dots enabled by surfactant modification as top hole-transport materials for efficient perovskite solar cells, Adv. Sci., 2019, 6, 1801169 CrossRef PubMed.
- S. Akin, Y. Liu, M. I. Dar, S. M. Zakeeruddin, M. Grätzel, S. Turan and S. Sonmezoglu, Hydrothermally processed CuCrO2 nanoparticles as an inorganic hole transporting material for low-cost perovskite solar cells with superior stability, J. Mater. Chem. A, 2018, 6, 20327–20337 RSC.
- H. Zhang, H. Wang, W. Chen and A. K.-Y. Jen, CuGaO2: A promising inorganic hole-transporting material for highly efficient and stable perovskite solar cells, Adv. Mater., 2017, 29, 1604984 CrossRef PubMed.
- T.-C. Liang, H.-Y. Su, S.-A. Chen, Y.-J. Chen, C.-Y. Chiang, C.-H. Chiang, T.-T. Kao, L.-C. Chen and C.-C. Lin, Planar perovskite solar cells using perovskite CsPbI3 quantum dots as efficient hole transporting layers, Materials, 2022, 24, 8902 CrossRef PubMed.
- L. Hu, W. W. Wang, H. Liu, J. Peng, H. F. Cao, G. Shao, Z. Xia, W. L. Ma and J. Tang, PbS colloidal quantum dots as an effective hole transporter for planar heterojunction perovskite solar cells, J. Mater. Chem. A, 2015, 3, 515–518 RSC.
- L. S. Khanzada, I. Levchuk, Y. Hou, H. Azimi, A. Osvet, R. Ahmad, M. Brandl, P. Herre, M. Distaso, R. Hock, W. Peukert, M. Batentschuk and C. J. Brabec, Effective ligand engineering of the Cu2ZnSnS4 nanocrystal surface for increasing hole transport efficiency in perovskite solar cells, Adv. Funct. Mater., 2016, 26, 8300–8306 CrossRef CAS.
- G. Y. Ashebir, C. Dong, Z. Y. Wan, J. J. Qi, J. W. Chen, Q. Y. Zhao, W. W. Chen and M. T. Wang, Solution-processed Cu2ZnSnS4 nanoparticle film as efficient hole transporting layer for stable perovskite solar cells, J. Phys. Chem. Solids, 2019, 129, 204–208 CrossRef CAS.
- W. Lee, I. Kim, H. Choi and K. Kim, Synthesis of Ni/NiO core-shell nanoparticles for wet-coated hole transport layer of the organic solar cell, Surf. Coat. Technol., 2013, 231, 93–97 CrossRef CAS.
- S. S. Chen, S. W. Yang, H. Sun, L. Zhang, J. J. Peng, Z. Q. Liang and Z.-S. Wang, Enhanced interfacial electron transfer of inverted perovskite solar cells by introduction of CoSe into the electron-transporting-layer, J. Power Sources, 2017, 353, 123–130 CrossRef CAS.
- S. Weber, T. Rath, J. Mangalam, B. Kunert, A. M. Coclite, M. Bauch, T. Dimopoulos and G. Trimmel, Investigation of NiOx-hole transport layers in triple cation perovskite solar cells, J. Mater. Sci., 2018, 29, 1847–1855 CAS.
- D. Saranin, T. Komaricheva, L. Luchnikov, D. S. Muratov, T. S. Le, Y. Karpov, P. Gostishchev, S. Yurchuk, D. Kuznetsov, S. Didenko and A. D. Carlo, Hysteresis-free perovskite solr cells with compact and nanoparticle NiO for indoor application, Sol. Energy Mater. Sol. Cells, 2021, 227, 111095 CrossRef CAS.
- G. Luo, Y. X. Zhang, Q. L. Zhu, Z. Q. An, P. Lv, J. H. Chen, Y. Q. Zhu, M. Hu, W. N. Li, K. Cao, Z. L. Ku, W. C. Huang, Y.-B. Cheng and J. F. Lu, Ruthenium complex optimized contact interfaces of NiOX nanocrystals for efficient and stable perovskite solar cells, Sol. RRL, 2023, 2300890 CrossRef.
- J. B. You, L. Meng, T.-B. Song, T.-F. Guo, Y. Yang, W.-H. Chang, Z. R. Hong, H. J. Chen, H. P. Zhou, Q. Chen, Y. S. Liu, N. D. Marco and Y. Yang, Improved air stability of perovskite solar cells via solution-processed metal oxide transport layers, Nat. Nanotechnol., 2016, 11, 75–81 CrossRef CAS PubMed.
- M. Najafi, F. D. Giacomo, D. Zhang, S. Shanmugam, A. Senes, W. Verhees, A. Hadipour, Y. Galagan, T. Aernouts, S. Veenstra and R. Andriessen, Highly efficient and stable flexible perovskite solar cells with metal oxides nanoparticle charge extraction layers, Small, 2018, 14, 1702775 CrossRef PubMed.
- H. Zhang, J. Q. Cheng, F. Lin, H. X. He, J. Mao, K. S. Wong, A. K.-Y. Jen and W. C. H. Choy, Pinhole-free and surface-nanostructured NiOx film by room-temperature solution process for high-performance flexible perovskite solar cells with good stability and reproducibility, ACS Nano, 2016, 10, 1503–1511 CrossRef CAS PubMed.
- J. J. He, E. B. Bi, W. T. Tang, Y. B. Wang, Z. M. Zhou, X. D. Yang, H. Chen and L. Y. Han, Ligand-free, highly dispersed NiOx nanocrystal for efficient, stable, low-temperature processable perovskite solar cells, Sol. RRL, 2018, 2, 1800004 CrossRef.
- Q. Q. He, K. Yao, X. F. Wang, X. F. Xia, S. F. Leng and F. Li, Room-temperature and solution-processable Cu-doped nickel oxide nanoparticles for efficient hole-transport layers of flexible large-area perovskite solar cells, ACS Appl. Mater. Interfaces, 2017, 9, 41887–41897 CrossRef CAS PubMed.
- J. J. He, Y. R. Xiang, F. Zhang, J. R. Lian, R. Hu, P. J. Zeng, J. Song and J. L. Qu, Improvement of red light harvesting ability and open circuit voltage of Cu:NiOx based p-i-n planar perovskite solar cells boosted by cysteine enhanced interface contact, Nano Energy, 2018, 45, 471 CrossRef CAS.
- D. Ouyang, J. W. Zheng, Z. F. Huang, L. Zhu and W. C. H. Choy, An efficacious multifunction codoping strategy on a room-temperature solution-processed hole transport layer for realizing high-performance perovskite solar cells, J. Mater. Chem. A, 2021, 9, 371–379 RSC.
- J. Wang, J. Zhang, Y. Z. Zhou, H. B. Liu, Q. F. Xue, X. S. Li, C.-C. Chueh, H.-L. Yip, Z. L. Zhu and A. K.-Y. Jen, Highly efficient all-inorganic perovskite solar cells with suppressed non-radiative recombination by a Lewis base, Nat. Commun., 2020, 11, 177 CrossRef CAS PubMed.
- A. Savva, I. T. Papadas, D. Tsikritzis, G. S. Armatas, S. Kennou and S. A. Choulis, Room temperature nanoparticulate interfacial layers for perovskite solar cells via solvothermal synthesis, J. Mater. Chem. A, 2017, 5, 2038120389 RSC.
- W. A. Dunlap-Shohl, T. B. Daunis, X. M. Wang, J. Wang, B. Y. Zhang, D. Barrera, Y. F. Yan, J. W. P. Hsu and D. B. Mitzi, Room-temperature fabrication of a delafossite CuCrO2 hole transport layer for perovskite solar cells, J. Mater. Chem. A, 2018, 6, 469–477 RSC.
- H. Zhang, H. Wang, H. M. Zhu, C.-C. Chueh, W. Chen, S. H. Yang and A. K.-Y. Jen, Low-temperature solution-pocessed CuCrO2 hole-transporting layer for efficient and photostable perovskite solar cells, Adv. Energy Mater., 2018, 8, 1702762 CrossRef.
- I. T. Papadas, A. Savva, A. Loakeimidis, P. Eleftheriou, G. S. Armatas and S. A. Choulis, Employing surfactant-assisted hydrothermal synthesis to control CuGaO2 nanoparticle formation and improved carrier selectivity of perovskite solar cells, Mater. Today Energy, 2018, 8, 57–64 CrossRef.
- D. Ouyang, J. Y. Xiao, F. Ye, Z. F. Huang, H. Zhang, L. Zhu, J. Q. Cheng and W. C. H. Choy, Strategic synthesis of ultrasmall NiCo2O4 NPs as hole transport layer for highly efficient perovskite solar cells, Adv. Energy Mater., 2018, 16, 1702722 CrossRef.
- I. T. Papadas, A. Ioakeimidis, G. S. Armatas and S. A. Choulis, Low-temperature combustion synthesis of a spinel NiCo2O4 hole transport layer for perovskite photovoltaics, Adv. Sci., 2018, 5, 1701029 CrossRef PubMed.
- Z. F. Huang, D. Ouyang, R. M. Ma, W. Wu, V. A. L. Roy and W. C. H. Choy, A general method: Designing a hypocrystalline hydroxide intermediate to achieve ultrasmall and well-dispersed ternary metal oxide for efficient photovoltaic devices, Adv. Funct. Mater., 2019, 29, 1904684 CrossRef CAS.
- B. P. Yang, D. Ouyang, Z. F. Huang, X. G. Ren, H. Zhang and W. C. H. Choy, Multifunctional synthesis approach of In:CuCrO2 nanopaticles for hole transport layer in high-performance perovskite solar cells, Adv. Funct. Mater., 2019, 29, 1902600 CrossRef.
- A. Kumar, D. K. Jarwal, A. K. Mishra, S. Ratan, C. Kumar, R. K. Upadhyay, B. Mukherjee and S. Jit, Effects of HTL and ETL thicknesses on the performance of PQT-12/PCDTBT:PC61BM/ZnO QDs solar cells, IEEE Photonics Technol. Lett., 2020, 32, 677–680 CAS.
- B. P. Yang, S. M. Peng and W. C. H. Choy, Inorganic top electron transport layer for high performance inverted perovskite solar cells, EcoMat, 2021, 3, e12127 CrossRef CAS.
- A. Wibowo, M. A. Marsudi, M. I. Amal, M. B. Ananda, R. Stephanie, H. Ardy and L. J. Diguna, ZnO nanostructured materials for emerging solar cell applications, RSC Adv., 2020, 10, 42838–42859 RSC.
- S. B. Shivarudraiah, M. Ng, C.-H. A. Li and J. E. Halpert, All-inorganic, solution-processed, inverted CsPbI3 quantum dot solar cells with a PCE of 13.1% achieved via a layer-by-layer FAI treatment, ACS Appl. Energy Mater., 2020, 3, 5620–5627 CrossRef CAS.
- L. J. Meng, Q. W. Xu, U. K. Thakur, L. Gong, H. B. Zeng, K. Shankar and X. H. Wang, Unusual surface ligand doping-induced p-type quantum dot slides and their application in solar cells, ACS Appl. Mater. Interfaces, 2020, 12, 53942–53949 CrossRef CAS PubMed.
- T. T. Jiang and W. F. Fu, Improved performance and stability of perovskite solar cells with bilayer electron-transporting layers, RSC Adv., 2018, 8, 5897–5901 RSC.
- C.-J. Chen, A. Chandel, D. Thakur, J.-R. Wu, S.-E. Chiang, G.-S. Zeng, J.-L. Shen, S.-H. Chen and S. H. Chang, Ag modified bathocuproine:ZnO nanoparticles electron buffer layer based bifacial inverted-type perovskite solar cells, Org. Electron., 2021, 92, 106110 CrossRef CAS.
- Y.-H. Chiang, C.-K. Shih, A.-S. Sie, M.-H. Li, C.-C. Peng, P.-S. Shen, Y.-P. Wang, T.-F. Guo and P. Chen, Highly stable perovskite solar cells with all-inorganic selective contacts from microwave-synthesized oxide nanoparticles, J. Mater. Chem. A, 2017, 5, 25485–25493 RSC.
- P.-H. Lee, T.-T. Wu, K.-Y. Tian, C.-F. Li, C.-H. Hou, J.-J. Shyue, C.-F. Lu, Y.-C. Huang and W.-F. Su, Work-function-tunable electron transport layer of molecule-capped metal oxide for a high-efficiency and stable p-i-n perovskite solar cells, ACS Appl. Mater. Interfaces, 2020, 12, 45936–45949 CrossRef CAS PubMed.
- Y. Zhao, H. Zhang, X. G. Ren, H. L. Zhu, Z. F. Huang, F. Ye, D. Ouyang, K. W. Cheah, A. K.-Y. Jen and W. C. H. Choy, Thick TiO2-based top electron transport layer on perovskite for highly efficient and stable solar cells, ACS Energy Lett., 2018, 3, 2891–2898 CrossRef CAS.
- S. S. Zhang, W. T. Chen, S. H. Wu, R. Chen, Z. H. Liu, Y. Q. Huang, Z. C. Yang, H. M. Zhu, J. Y. Li, L. Y. Han and W. Chen, Hybrid inorganic electron-transporting layer coupled with a halogen-resistant electrode in CsPbI2Br-based perovskite solar cells to achieve robust long-term stability, ACS Appl. Mater. Interfaces, 2019, 11, 43303–43311 CrossRef CAS PubMed.
- J. Lim, W. Jang, M. S. Kim, M. Ji, Y.-I. Lee and D. H. Wang, Hydrogenerated black titanium dioxide-embedded conducting polymer for boosting electron flow in perovskite devices, J. Alloys Compd., 2020, 846, 156329 CrossRef CAS.
- R. Fang, S. H. Wu, W. T. Chen, Z. H. Liu, S. S. Zhang, R. Chen, Y. F. Yue, L. L. Deng, Y.-B. Cheng, L. Y. Han and W. Chen, [6,6]-Phenyl-C61-butyric acid methyl ester/cerium oxide bilayer structure as efficient and stable electron transport layer for inverted perovskite solar cells, ACS Nano, 2018, 12, 2403–2414 CrossRef CAS PubMed.
- B. P. Yang, R. M. Ma, Z. S. Wang, D. Ouyang, Z. F. Huang, J. L. Lu, X. H. Duan, L. Yue, N. Xu and W. C. H. Choy, Efficient gradient potential top electron transport structures achieved by combining an oxide family for inverted perovskite solar cells with high efficiency and stability, ACS Appl. Mater. Interfaces, 2021, 13, 27179–27187 CrossRef CAS PubMed.
- F. R. Tan, W. Z. Xu, X. D. Hu, P. Yu and W. F. Zhang, Highly efficient inverted perovskite solar cells with CdSe QDs/LiF electron transporting layer, Nanoscale Res. Lett., 2017, 12, 614 CrossRef PubMed.
- R. T. Ako, P. Ekanayake, D. J. Young, J. Hobley, V. Chellappan, A. Tan, S. Gorelik, G. S. Subramanian and C. M. Lim, Evaluation of surface energy state distribution and bulk defect concentration in DSSC photoanodes based on Sn, Fe, and Cu doped TiO2, Appl. Surf. Sci., 2015, 351, 950–961 CrossRef CAS.
- M. Neetu, I. C. Maurya, A. K. Gupta, P. Srivastava and L. Bahadur, Extensive enhancement in power conversion efficiency of dye-sensitized solar cell by using Al-doped TiO2 photoanode, J. Solid State Electrochem., 2017, 21, 1229–1241 CrossRef.
- B. Ünlü, S. Çakar and M. Özacar, The effects of metal doped TiO2 and dithizone-metal complexes on DSSCs performance, Sol. Energy, 2018, 166, 441–449 CrossRef.
- J. Manju and S. J. Jawhar, Facile synthesis and characterization of Ti(1-x)CuxO2 nanoparticles for high efficiency dye sensitized solar cell applications, Opt. Mater., 2017, 69, 119–127 CrossRef CAS.
- M.-J. Lee, J.-Y. Park, C.-S. Kim, K. Okuyama, S.-E. Lee and T.-O. Kim, Improvement of light scattering capacity in dye-sensitized solar cells by doping with SiO2 nanoparticles, J. Power Sources, 2016, 327, 96–103 CrossRef CAS.
- S. Kundu, P. Sarojinijeeva, R. Karthick, G. Anantharaj, G. Saritha, R. Bera, S. Anandan, A. Patra, P. Ragupathy, M. Selvaraj, D. Jeyakumar and K. Pillai, Enhancing the efficiency of DSSCs by the modification of TiO2 photoanodes using N, F, and S, co-doped graphene quantum dots, Electrochim. Acta, 2017, 242, 337–343 CrossRef CAS.
- X. Z. Zhang, T. Y. Wu, X. X. Xu, L. Zhang, J. Tang, X. He, J. H. Wu and Z. Lan, Ligand-exchange TiO2 nanocrystals induced formation of high-quality electron transporting layers at low temperature for efficient planar perovskite solar cells, Sol. Energy Mater. Sol. Cells, 2018, 178, 65–73 CrossRef CAS.
- K. Sanglee, S. Chuangcote, T. Krajangsang, J. Sritharathikhun, K. Sriprapha and T. Sagawa, Quantum dot-modified titanium dioxide nanoparticles as an energy-band tunable electron-transporting layer for open air-fabricated planar perovskite solar cells, Nanomater. Nanotechnol., 2020, 10, 1847980420961638 CAS.
- R. Singh, I. Ryu, H. Yadav, J. Park, J. W. Jo, S. Yim and J.-J. Lee, Non-hydrolytic sol-gel route to synthesize TiO2 nanoparticles under ambient condition for highly efficient and stable perovskite solar cells, Sol. Energy, 2019, 185, 307–314 CrossRef CAS.
- A. Badawi, N. Al-Hosiny and S. Abdallah, The photovoltaic performance of CdS quantum dots sensitized solar cell using graphene/TiO2 working electrode, Superlattices Microstruct., 2015, 81, 88–96 CrossRef CAS.
- P. N. Kumar, R. Narayanan, S. Laha, M. Deepa and A. K. Srivastava, Phoroelectrochromic cell with a CdS quantum dots/graphitic-nanoparticles sensitized anode and a molybdenum oxide cathode, Sol. Energy Mater. Sol. Cells, 2016, 153, 138–147 CrossRef CAS.
- Z. Zolfaghari-Isavandi and Z. Shariatinia, Enhanced efficiency of quantum dot sensitized solar cells using Cu2O/TiO2 nanocomposite photoanodes, J. Alloys Compd., 2018, 737, 99–112 CrossRef CAS.
- S. Zhang, Z. Lan, J. H. Wu, X. Chen and C. Y. Zhang, Preparation of novel TiO@ quantum dot blocking layers at conductive glass/TiO2 interfaces for efficient CdS quantum dot sensitized solar cells, J. Alloys
Compd., 2016, 656, 253–258 CrossRef CAS.
- M. Marandi, P. Talebi and S. Bayat, Optimization of the doping process and light scattering in CdS:Mn quantum dots sensitized solar cells for the efficiency enhancement, J. Mater. Sci.: Mater. Electron., 2019, 30, 3820–3832 CrossRef CAS.
- Y. B. Lu, L. Li, S. C. Su, Y. J. Chen, Y. L. Song and S. J. Jiao, A novel TiO2 nanostructure as photoanode for highly efficient CdSe quantum dot-sensitized solar cells, RSC Adv., 2017, 7, 9795–9802 RSC.
- J. P. Deng, M. Q. Wang, J. F. Fang, X. H. Song, Z. Yang and Z. L. Yuan, Synthesis of Zn-doped TiO2 nano-particles using metal Ti and Zn as raw materials and application in quantum dot sensitized solar cells, J. Alloys Compd., 2019, 791, 371–379 CrossRef CAS.
- S. M. Thon, A. H. Ip, O. Voznyy, L. Levina, K. W. Kemp, G. H. Carey, S. Masala and E. H. Sargent, Role of bond adaptability in the passivation of colloidal quantum dot solids, ACS Nano, 2013, 7, 7680–7688 CrossRef CAS PubMed.
- A. E. Tom, A. Thomas and V. V. Ison, Novel post-synthesis purification strategies and the ligand exchange processes in simplifying the fabrication of PbS quantum dot solar cells, RSC Adv., 2020, 10, 30707–30715 RSC.
- N. L. Zhang, D. C. J. Neo, Y. Tazawa, X. T. Li, H. E. Assender, R. G. Compton and A. A. R. Watt, Narrow band gap lead sulfide hole transport layers for quantum dot photovoltaics, ACS Appl. Mater. Interfaces, 2016, 8, 21417–21422 CrossRef CAS PubMed.
- D. C. J. Neo, N. L. Zhang, Y. Tazawa, H. B. Jiang, G. M. Hughes, C. R. M. Grovenor, H. E. Assender and A. A. R. Watt, Poly(3-hexylthiophene-2,5-diyl) as a hole transport layer for colloidal quantum dot solar cells, ACS Appl. Mater. Interfaces, 2016, 8, 12101 CrossRef CAS PubMed.
- A. Mnoyan, K. Kim, J. Y. Kim and D. Y. Jeon, Colloidal nanocomposite of reduced grapheme oxide and quantum dots for enhanced surface passivation in optoelectronic applications, Sol. Energy Mater. Sol. Cells, 2016, 144, 181–186 CrossRef CAS.
- S. P. Zang, Y. L. Wang, M. Y. Li, W. Su, H. C. Zhu, X. T. Zhang and Y. C. Liu, Fabrication of efficient PbS colloidal quantum dot solar cell with low temperature sputter-deposited ZnO electron transport layer, Sol. Energy Mater. Sol. Cells, 2017, 169, 264–269 CrossRef CAS.
- J. Choi, J. W. Jo, F. P. García de Arquer, Y.-B. Zhao, B. Sun, J. Kim, M.-J. Choi, S.-W. Baek, A. H. Proppe, A. Seifitokaldani, D.-H. Nam, P. Li, O. Ouellette, Y. Kim, O. Voznyy, S. Hoogland, S. O. Kelley, Z.-H. Lu and E. H. Sargent, Activated electron-transport layers for infrared quantum dot optoelectronics, Adv. Mater., 2018, 30, 1801720 CrossRef PubMed.
- Y. Xue, F. Yang, J. Y. Yuan, Y. N. Zhang, M. F. Gu, Y. L. Xu, X. F. Ling, Y. Wang, F. C. Li, T. S. Zhai, J. N. Li, C. H. Cui, Y. W. Chen and W. L. Ma, Toward scalable PbS quantum dot solar cells using a tailored polymeric hole conductor, ACS Energy Lett., 2019, 4, 2850–2858 CrossRef CAS.
- E. G. Durmusoglu, G. S. Selopal, M. Mohammadnezhad, H. Zhang, P. Dagtepe, D. Barba, S. H. Sun, H. G. Zhao, H. Y. Acar, Z. M. Wang and F. Rosei, Low-cost, air-processed quantum dot solar cells via diffusion-controlled synthesis, ACS Appl. Mater. Interfaces, 2020, 12, 36301–36310 CrossRef CAS PubMed.
- C. Ding, F. Liu, Y. H. Zhang, S. Hayase, T. Masuda, R. X. Wang, Y. Zhou, Y. F. Yao, Z. G. Zou and Q. Shen, Passivation strategy of reducing both electron and hole states for achieving high-efficiency PbS quantum-dot solar cells with power conversion efficiency over 12%, ACS Energy Lett., 2020, 5, 3224–3236 CrossRef CAS.
- T. S. Zhao, E. D. Goodwin, J. C. Guo, H. Wang, B. T. Diroll, C. B. Murray and C. R. Kagan, Advanced architecture for colloidal PbS quantum dot solar cells exploiting a CdSe quantum dot buffer layer, ACS Nano, 2016, 10, 9267–9273 CrossRef CAS PubMed.
- S. Kumar, R. Upadhyay and B. Pradhan, Performance enhancement of heterojunction ZnO/PbS quantum dot solar cells by interface engineering, Sol. Energy, 2020, 211, 283–290 CrossRef CAS.
- J. P. Deng, M. Q. Wang, W. Ye, J. F. Fang, P. C. Zhang, Y. P. Yang and Z. Yang, CdS/CdSe-sensitized solar cell based on Al-doped ZnO nanoparticles prepared by the decomposition of zinc acetate solid solution, Solid-State Electron., 2017, 127, 38–44 CrossRef CAS.
- F. Yang, Y. L. Xu, M. F. Gu, S. J. Zhou, Y. J. Wang, K. Y. Lu, Z. K. Liu, X. F. Ling, Z. J. Zhu, J. M. Chen, Z. Y. Wu, Y. N. Zhang, Y. Xue, F. C. Li, J. Y. Yuan and W. L. Ma, Synthesis of cesium-doped ZnO nanoparticles as an electron extraction layer for efficient PbS colloidal quantum dot solar cells, J. Mater. Chem. A, 2018, 6, 17688–17697 RSC.
- N. Sukharevska, D. Bederak, V. M. Goossens, J. Momand, H. Duim, D. N. Dirin, M. V. Kovalenko, B. J. Kooi and M. A. Loi, Scalable PbS quantum dot solar cell production by blade coating from stable inks, ACS Appl. Mater. Interfaces, 2021, 13, 5195–5207 CrossRef CAS PubMed.
- X. S. Zhang, Z. W. Jin, J. R. Zhang, D. L. Bai, H. Bian, K. Wang, J. Sun, Q. Wang and S. Z. F. Liu, All-inorganic processed binary CsPbBr3-CsPb2Br5 perovskites with synergistic enhancement for high-efficiency Cs-Pb-Br-based solar cells, ACS Appl. Mater. Interfaces, 2018, 10, 7145–7154 CrossRef CAS PubMed.
- Y. N. Zhang, Y. Y. Kan, K. Gao, M. F. Gu, Y. Shi, X. L. Zhang, Y. Xue, X. N. Zhang, Z. K. Liu, Y. Zhang, J. Y. Yuan, W. L. Ma and A. K.-Y. Jen, Hybrid quantum dot/organic heterojunction: A route to improve open-circuit voltage in PbS colloidal quantum dot solar cells, ACS Energy Lett., 2020, 5, 2335–2342 CrossRef CAS.
- P. D. Li, T. G. Jiu, G. Tang, G. J. Wang, J. Li, X. F. Li and J. F. Fang, Solvents induced ZnO nanoparticles aggregation associated with their interfacial effect on organic solar cells, ACS Appl. Mater. Interfaces, 2014, 6, 18172–18179 CrossRef CAS PubMed.
- S. Bishnoi, V. Gupta, C. Sharma, D. Haranath, M. Kumar and S. Chand, Luminescent cathode buffer layer for enhanced power conversion efficiency and stability of bulk-heterojunction solar cells, Org. Electron., 2016, 38, 193–199 CrossRef CAS.
- M. Abrari, M. Ghanaatshoar, H. R. Moazami and S. S. H. Davarani, Synthesis of SnO2 nanoparticles by electrooxidation method and their application in dye-sensitized solar cells: The influence of the counterion, J. Electron. Mater., 2019, 48, 445–453 CrossRef CAS.
- A. E. Shalan, M. Rasly, I. Osama, M. M. Rashad and I. A. Ibrahim, Photpcurrrent enhancement by Ni2+ and Zn2+ ion doped in SnO2 nanoparticles in highly porous dye-sensitized solar cells, Ceram. Int., 2014, 40, 11619–11626 CrossRef CAS.
- Y. Wang, M. L. Feng, H. R. Chen, M. Ren, H. F. Wang, Y. F. Miao, Y. T. Chen and Y. X. Zhao, Highly crystalized Cl-doped SnO2 nanocrystals for stable aqueous dispersion toward high-performance perovskite photovoltaics, Adv. Mater., 2023, 23058498 Search PubMed.
- M. Saeidi, M. Abrari and M. Ahmadi, Fabrication of dye-sensitized solar cell based on mixed tin and zinc oxide nanoparticles, Appl. Phys. A, 2019, 125, 409 CrossRef.
- M. Asemi and M. Ghanaatshoar, Studying the effect of the controlled off-stoichiometry on the properties of Zn2SnO4 nanoparticles for DSSC applications, J. Mater. Sci., 2018, 29, 6730–6740 CAS.
- A. P. S. Souza, F. G. S. Oliveira, V. F. Nunes, F. M. Lima, A. F. L. Almeida, I. M. M. de Carvalho and M. P. F. Graca, High performance SnO2 pure photoelectrode in dye-sensitized solar cells achieved via electrophoretic technique, Sol. Energy, 2020, 211, 312–323 CrossRef CAS.
- K. Balasubramanian and G. Venkatachari, Synthesis and characterization of Sb doped SnO2 for the photovoltaic applications: different route, Mater. Res. Express, 2019, 6, 1250k6 CrossRef.
- S. J. In, M. Park and J. W. Jung, Reduced interface energy loss in non-fullerene organic solar cells using room temperature-synthesis SnO2 quantum dots, J. Mater. Sci. Technol., 2020, 52, 12–19 CrossRef.
- T. Y. Kong, R. Wang, D. Zheng and J. S. Yu, Modification of the SnO2 electron transporting layer by using perylene diimide derivative for efficient organic solar cells, Front. Chem., 2021, 9, 703561 CrossRef CAS PubMed.
- S. Guang, J. Yu, H. Wang, X. Liu, S. Qu, R. Zhu and W. Tang, J. Energy Chem., 2021, 56, 496 CrossRef CAS.
- S. N. Vijayaraghavan, J. Wall, L. Li, G. Xing, Q. Zhang and F. Yan, Low-temperature processed highly efficient hole transport layer free carbon-based planar perovskite solar cells with SnO2 quantum dot electron transport layer, Mater. Today Phys., 2020, 13, 100204 CrossRef.
- Z. Ma, W. Y. Zhou, Z. Xiao, H. Zhang, Z. Y. Li, J. Zhuang, C. T. Peng and Y. L. Huang, Negligible hysteresis planar perovskite solar cells using Ga-doped SnO2 nanocrystal as electron transport layers, Org. Electron., 2019, 71, 98–105 CrossRef CAS.
- S. Y. Park and H. C. Shim, Highly efficient and air-stable heterostructured perovskite quantum dot solar cells sing a solid-state cation-exchange reaction, ACS Appl. Mater. Interfaces, 2020, 12, 57124–57133 CrossRef CAS PubMed.
- M. Singh, A. Ng, Z. Ren, H. L. Hu, H.-C. Lin, C.-W. Chu and G. Li, Facile synthesis of composite tin oxide nanostructures for high-performance planar perovskite solar cells, Nano Energy, 2019, 60, 275–284 CrossRef CAS.
- H. X. Xie, X. T. Yin, P. Chen, J. Liu, C. H. Yang, W. X. Que and G. F. Wang, Solvothermal synthesis of highly crystalline SnO2 nanoparticles for flexible perovskite solar cells application, Mater. Lett., 2019, 234, 311–314 CrossRef CAS.
- Z. C. Wang, T. Wu, L. Xiao, P. L. Qin, X. L. Yu, L. Ma, L. Xiong, H. X. Li, X. B. Chen, Z. Wang, T. Wu, L. Xiao, P. Qin, X. Yu, L. Ma, L. Xiong, H. Li and X. Chen, Multifunctional potassium hexafluorophosphate passivates interface defects for high efficiency perovskite solar cells, J. Power Sources, 2021, 488, 229451 CrossRef CAS.
- H. R. Liu, Z. L. Chen, H. B. Wang, F. H. Ye, J. J. Ma, X. L. Zheng, P. B. Gui, L. B. Xiong, J. Wen and G. J. Fang, A facial room temperature solution synthesis of SnO2 quantum dots for perovskite solar cells, J. Mater. Chem. A, 2019, 7, 10636–10643 RSC.
- G. Yang, C. Chen, F. Yao, Z. L. Chen, Q. Zhang, X. L. Zheng, J. J. Ma, H. W. Lei, P. L. Qin, L. B. Xiong, W. J. Ke, G. Li, Y. F. Yan and G. J. Fang, Effective carrier-concentration tuning of SnO2 quantum dot electron-selective layers for high-performance planar perovskite solar cells, Adv. Mater., 2018, 30, 1706023 CrossRef PubMed.
- M. Kim, J. Jeong, H. Lu, T. K. Lee, F. T. Eickemeyer, Y. Liu, I. W. Choi, S. J. Choi, Y. Jo, H.-B. Kim, S.-I. Mo, Y.-K. Kim, H. Lee, N. G. An, S. Cho, W. R. Tress, S. M. Zakeeruddin, A. Hagfeldt, J. Y. Kim, M. Grätzel and D. S. Kim, Conformal quantum dot-SnO2 layers as electron transporters for efficient perovskite solar cells, Science, 2022, 375, 302–306 CrossRef CAS PubMed.
- Y. Wang, C. H. Duan, X. L. Zhang, N. Rujisamphan, Y. Liu, Y. Y. Li, J. Y. Yuan and W. L. Ma, Dual interfacial engineering enables efficient and reproducible CsPbI2Br all-inorganic perovskite solar cells, ACS Appl. Mater. Interfaces, 2020, 12, 31659–31666 CrossRef CAS PubMed.
- R. X. Peng, T. T. Yan, J. W. Chen, S. F. Yang, Z. Y. Ge and M. T. Wang, Passivating surface defects of n-SnO2 electron transporting layer by InP/ZnS quantum dots: Toward efficient and stable organic solar cells, Adv. Electron. Mater., 2020, 6, 1901245 CrossRef CAS.
- N. Huang, G. W. Li, H. Huang, P. P. Sun, T. L. Xiong, Z. F. Xia, F. Zheng, J. Xu and X. H. Sun, One-step solvothermal tailoring the compositions and phases of nickel cobalt sulfides on conducting oxide substrates as counter electrodes for efficient dye-sensitized solar cells, Appl. Surf. Sci., 2016, 390, 847–855 CrossRef CAS.
- K. Al-Attafi, F. H. Jawdat, H. Qutaish, P. Hayes, A. Al-Keisy, K. Shim, Y. Yamauchi, S. X. Dou, A. Nattestad and J. H. Kim, Cubic aggregates of Zn2SnO4 nanoperticles and their application in dye-sensitized solar cells, Nano Energy, 2019, 57, 202–213 CrossRef CAS.
- Q. Jiang, L. Zhang, H. Wang, X. Yang, J. Meng, H. Liu, Z. Yin, J. Wu, X. Zhang and J. You, Nat. Energy, 2017, 2, 16177 CrossRef CAS.
- H.-J. Kim, G.-C. Xu, C. V. V. M. Gopi, H. Seo, M. Venkata-Haritha and M. Shiratani, Enhanced light harvesting and charge recombination control with TiO2/PbCdS/CdS based quantum dot-sensitized solar cells, J. Electroanal. Chem., 2017, 788, 131–136 CrossRef CAS.
- E. Jalali-Moghadam and Z. Shariatinia, Al3+ doping into TiO2 photoanodes improved the performance of amine anchored CdS quantum dot sensitized solar cells, Mater. Res. Bull., 2018, 98, 121–132 CrossRef CAS.
- S. Muthu, G. Zaiats, M. B. Sridharan and P. V. Kamat, influence of plasmonic CuxS interfacing layer on photovoltaic performance of CIZS quantum dot sensitized solar cells, J. Electrochem. Soc., 2019, 166, H3133–H3137 CrossRef CAS.
- H. T. Dastjerdi, P. F. Qi, Z. Y. Fan and M. M. Tavakoli, Cost-effective and semi-transparent PbS quantum dot solar cells using copper electrodes, ACS Appl. Mater. Interfaces, 2020, 12, 818–825 CrossRef PubMed.
- Z. L. Zhang, Z. H. Chen, J. B. Zhang, W. J. Chen, J. F. Yang, X. M. Wen, B. Wang, N. Kobamoto, L. Yuan, J. A. Stride, G. J. Conibeer, R. J. Patterson and S. J. Huang, Significant improvement in the performance of PbSe quantum dot solar cell by introducing CsPbBr3 perovskite colloidal nanocrystal back layer, Adv. Energy Mater., 2017, 7, 1601773 CrossRef.
- H. Ren, A. Xu, Y. Y. Pan, D. H. Qin, L. T. Hou and D. Wang, Efficient PbS quantum dot solar cells with both Mg-doped ZnO window layer and ZnO nanocrystal interface passivation layer, Nanomaterials, 2021, 11, 219 CrossRef CAS PubMed.
- K. M. Kim, J. H. Jeon, Y. Y. Kim, H. K. Lee, O. O. Park and D. H. Wang, Effects of ligand exchange CdSe quantum dot interlayer for inverted organic solar cells, Org. Electron., 2015, 25, 44–49 CrossRef CAS.
- Z. Q. Li, S. J. Li, Z. H. Zhang, X. Y. Zhang, J. F. Li, C. Y. Liu, L. Shen, W. B. Guo and S. P. Ruan, Enhanced electron extraction capability of polymer solar cells via modifying the cathode buffer layer with inorganic quantum dots, Phys. Chem. Chem. Phys., 2016, 18, 11435–11442 RSC.
- J. H. Jeon, D. H. Wang, H. Park, J. H. Park and O. O. Park, Stamping transfer of a quantum dot interlayer for organic photovoltaic cells, Langmuir, 2012, 28, 9893–9898 CrossRef CAS PubMed.
- C. H. Duan, W. N. Luo, T. G. Jiu, J. S. Li, Y. Wang and F. S. Lu, Facile preparation and characterization of ZnCdS nanocrystals for interfacial applications in photovoltaic devices, J. Colloid Interface Sci., 2018, 512, 353–360 CrossRef CAS PubMed.
- S. Huang, Y. T. Tang, A. C. Yu, Y. H. Wang, S. Shen, B. N. Kang, S. R. P. Silva and G. Y. Lu, Solution-processed SnO2 nanoparticle interfacial layers for efficient electron transport in ZnO-based polymer solar cells, Org. Electron., 2018, 62, 373–381 CrossRef CAS.
- M. Dusza, M. Stefanski, M. Wozniak, D. Hreniak, Y. Gerasymchuk, L. Marciniak, F. Granek and W. Strek, Luminescent Sr2CeO4 nanocrystals for applications in organic solar cells with conjugated polymers, J. Lumin., 2016, 169, 857–861 CrossRef CAS.
- Z. Zhang, Y. Yang, J. Gao, S. Xiao, C. H. Zhou, D. Q. Pan, G. Liu and X. Y. Guo, Highly efficient Ag2Se quantum dots blocking layer for solid-state dye-sensitized solar cells: Size effects on device performance, Mater, Mater. Today Energy, 2018, 7, 27–36 CrossRef.
- S. S. Hosseini, M. Ebadi and K. Motevalli, Facial microwave approach for synthesis of CdS quantum dots as barrier layer for increasing dye-sensitized solar cells efficiency, J. Mater. Sci.: Mater. Electron., 2016, 27, 12240–12246 CrossRef CAS.
- W. Zhang, D. Wang, Q. M. Wang and H. Q. Sun, The high performance of quantum dot sensitized solar cells co-sensitized with mixed-joint CdS and SnS quantum dots, ECS J. Solid State Sci. Technol., 2019, 8, Q96–Q100 CrossRef.
- M. Zahedifar, Z. Chamanzadeh and S. M. M. Hosseinpoor, Synthesis of LaVO4:Dy3+ luminescent nanostructured and optimization of its performance as down-converter in dye-sensitized solar cells, J. Lumin., 2013, 135, 66–73 CrossRef CAS.
- J. Roh, S. H. Hwang and J. Jang, Dual-functional CeO2:Eu3+ nanocrystals for performance-enhanced dye-sensitized solar cells, ACS Appl. Mater. Interfaces, 2014, 22, 19825–19832 CrossRef PubMed.
- J. Shen, Z. Q. Li, R. Cheng, Q. Luo, Y. D. Luo, Y. W. Chen, X. H. Chen, Z. Sun and S. M. Huang, Eu3+-doped NaGdF4 nanocrystal down-converting layer for efficient dye-sensitized solar cells, ACS Appl. Mater. Interfaces, 2014, 6, 17454–17462 CrossRef CAS PubMed.
- M. S. Morassaei, A. Salehabadi, A. Akbari, S. H. Tavassoli and M. Salavati-Niasari, Anhanced dye sensitized solar cells efficiency by utilization of an external layer of CaCe2(MoO4)4:Er3+/Yb3+ nanoparticles, J. Alloys Compd., 2018, 769, 732–739 CrossRef CAS.
- T. Chen, Y. F. Shang, S. W. Hao, L. Tian, Y. D. Hou and C. H. Yang, Enhancement of dye sensitized solar cell efficiency through introducing concurrent upconversion/downconversion core/shell nanoparticles as spectral converters, Electrochim. Acta, 2018, 282, 743–749 CrossRef CAS.
- A. K. Kaliamurthy, H. C. Kang, F. K. Asiam, K. Yoo and J.-J. Lee, Trap-assisted transition energy levels of SrF2:Pr3+-Yb3+ nanophosphor in TiO2 photoanode for luminescence tuning in dye-sensitized photovoltaic cells, Sol. RRL, 2021, 10, 2100411 CrossRef.
- R. Kamal and H. Hafez, Novel down-converting single-phased white light Pr3+ doped BaWO4 nanophosphors material for DSSC applications, Opt. Mater., 2021, 121, 111646 CrossRef CAS.
- M. Y. Cha, P. M. Da, J. Wang, W. Y. Wang, Z. H. Chen, F. X. Xiu, G. F. Zheng and Z.-S. Wang, Enhancing perovskite solar cell performance by interface engineering using CH3NH3PbBr0.9I2.1 quantum dots, J. Am. Chem. Soc., 2016, 138, 8581–8587 CrossRef CAS PubMed.
- P. Liu, Y. Sun, S. F. Wang, H. J. Zhang, Y. N. Gong, F. J. Li, Y. F. Shi, Y. X. Du, X. F. Li, S.-S. Guo, Q. D. Tai, C. L. Wang and X.-Z. Zhao, Two dimensional graphitic carbon quantum dots modified perovskite solar cells and photodetectors with high performances, J. Power Sources, 2020, 451, 227825 CrossRef CAS.
- Y. Z. Ma, P. Vashishtha, S. B. Shivarudraiah, K. Chen, Y. Liu, J. M. Hodgkiss and J. E. Halpert, A hybrid perovskite solar cell modified with copper indium sulfide nanocrystals to enhance hole transport and moisture stability, Sol. RRL, 2017, 1, 1700078 CrossRef.
- J. J. Ma, G. Yang, M. C. Qin, X. L. Zheng, H. W. Lei, C. Chen, Z. L. Chen, Y. X. Guo, H. W. Han, X. Z. Zhao and G. J. Fang, MgO nanoparticles modified anode for highly efficient SnO2-based planar perovskite solar cells, Adv. Sci., 2017, 4, 1700031 CrossRef PubMed.
- S. M. Ali, S. M. Ramay, M. H. Aziz, N. Ur-Rehman, M. S. AlGarawi, S. S. AlGhamd, A. Mahmood, T. S. Alkhuraiji and S. Atiq, Efficiency enhancement of perovskite solar cells by incorporation of CdS quantum dot through fast electron injection, Org. Electron., 2018, 62, 21–25 CrossRef CAS.
- Y. Wang, C. H. Duan, J. S. Li, W. Han, M. Zhao, L. L. Yao, Y. Y. Wang, C. Yan and T. G. Jiu, Performance enhancement of inverted perovskite solar cells based on smooth and compact PC61BM:SnO2 electron transport layers, ACS Appl. Mater. Interfaces, 2018, 10, 20128–20135 CrossRef CAS PubMed.
- T. T. Wu, C. Zhen, J. B. Wu, C. X. Jia, M. Haider, L. Z. Wang, G. Liu and H.-M. Cheng, Chlorine capped SnO2 quantum-dots modified TiO2 electron selective layer to enhance the performance of planar perovskite solar cells, Sci. Bull., 2019, 64, 547–552 CrossRef CAS PubMed.
- L.-C. Chen, C.-H. Tien, Z.-L. Tseng and J.-H. Ruan, Enhanced efficiency of MAPbI3 perovskite solar cells with FAPbX3 perovskite quantum dots, NanoMaterials, 2019, 9, 121 CrossRef CAS PubMed.
- X. Y. Zhu, B. Cheng, X. H. Li, J. J. Zhang and L. Y. Zhang, Enhanced efficiency of perovskite solar cells by PbS quantum dot modification, Appl. Surf. Sci., 2019, 487, 32–40 CrossRef CAS.
- Y. H. Ma, Y. W. Zhang, H. Y. Zhang, H. Lv, R. Y. Hu, W. Liu, S. L. Wang, M. Jiang, L. Chug, J. Zhang, X. A. Li, R. D. Xia and W. Huang, Effective carrier transport tuning of CuOx quantum dots hole interfacial layer for high-performance inverted perovskite solar cell, Appl. Surf. Sci., 2021, 547, 149117 CrossRef CAS.
- J. Y. Yin, Y. J. Yuan, J. Ni, J. Y. Guan, X. J. Zhou, Y. Liu, Y. Ding, H. K. Cai and J. J. Zhang, CH3NH3PbBr3-xIx quantum dots enhance bulk crystallization and interface charge transfer for efficient and stable perovskite solar cells, ACS Appl. Mater. Interfaces, 2020, 12, 48861–48873 CrossRef CAS PubMed.
- R. S. Li, Y. F. Dou, Y. S. Liao, D. Wang, G. D. Li, J. H. Wu and Z. Lan, Enhancing efficiency of perovskite solar cells from surface passivation of Co2+ doped CuGaO2 nanocrystals, J. Colloid Interface Sci., 2022, 607, 1280–1286 CrossRef CAS PubMed.
- L. Najafi, B. Taheri, B. Martín-García, S. Bellani, D. D. Girolamo, A. Agresti, R. Oropesa-Nuñez, S. Pescetelli, L. Vesce, E. Calabrò, M. Prato, A. E. D. R. Castillo, A. D. Carlo and F. Bonaccorso, MoS2 quantum dot/graphene hybrids for advanced interface engineering of a CH3NH3PbI3 perovskite solar cell with an efficiency of over 20%, ACS Nano, 2018, 12, 10736–10754 CrossRef CAS PubMed.
- C. Yang, Z. J. Wang, Y. H. Lv, R. H. Yuan, Y. H. Wu and W.-H. Zhang, Colloidal CsCu5S3 nanocrystals as an interlayer in high-performance perovskite solar cells with an efficiency of 22.29, Chem. Eng. J., 2021, 406, 126855 CrossRef CAS.
- D. L. Zhou, D. L. Liu, J. J. Jin, X. Chen, W. Xu, Z. Yin, G. C. Pan, D. Y. Li and H. W. Song, Semiconductor plasmon-sensitized broadband upconversion and its enhancement effect on the power conversion efficiency of perovskite solar cells, J. Mater. Chem. A, 2017, 5, 16559–16567 RSC.
- J. B. Jia, J. M. Dong, J. M. Lin, Z. Lan, L. Q. Fan and J. H. Wu, Improved photovoltaic performance of perovskite solar cells by utilizing down-conversion NaYF4:Eu3+ nanophosphors, J. Mater. Chem. C, 2019, 7, 937–942 RSC.
- W. B. Bi, Y. J. Wu, C. Chen, D. L. Zhou, Z. L. Song, D. Y. Li, G. Y. Chen, Q. L. Dai, Y. S. Zhu and H. W. Song, Dye sensitization and local surface plasmon resonance-enhanced upconversion luminescence for efficient perovskite solar cells, ACS Appl. Mater. Interfaces, 2020, 12, 24737–24746 CrossRef CAS PubMed.
- J. Ma, Y. J. Yuan and P. Sun, Approaching 23% silicon heterojunction solar cells with dual-functional SiOx/MoS2 quantum dots interface layers, Sol. Energy Mater. Sol. Cells, 2021, 227, 111110 CrossRef CAS.
- A. Thomas, R. Vinayakan and V. V. Ison, An inverted ZnO/P3HT:PbS bulk-heterojunction hybrid solar cell with a CdSe quantum dot interface buffer layer, RSC Adv., 2020, 10, 16693–16699 RSC.
- S. M. Reda, Enhanced efficiency of solar cell using luminescence PbS quantum dots concentrators, J. Fluoresc., 2015, 3, 631–639 CrossRef PubMed.
- W. L. Feng, Y. W. Wang, J. Liu and X. B. Yu, Polycrystalline Si nanocorals/CdS quantum dots composited solar cell with efficient light harvesting and surface passivation, Chem. Phys. Lett., 2014, 608, 314–318 CrossRef CAS.
- I. Levchuk, C. Wuerth, F. Krause, A. Osvet, M. Batentschuk, U. Resch-Genger, C. Kolbeck, P. Herre, H. P. Steinruck, W. Peukert and C. J. Brabec, Industrially scalable and cost-effective Mn2+ doped ZnxCd1-xS/ZnS nanocrystals with 70% photoluminescence quantum yield, as efficient down-shifting materials in photovoltaics, Energy Environ. Sci., 2016, 9, 1083–1094 RSC.
- M. Jalalah, Y.-H. Ko, F. A. Harraz, M. S. Al-Assiri and J.-G. Park, Enhanced efficiency and current
density of solar cells via energy-down-shift having energy-tuning-effect of highly UV-light-harvesting Mn2+-doped quantum dots, Nano Energy, 2017, 33, 257–265 CrossRef CAS.
- T. Y. Sun, X. Chen, L. M. Jin, H.-W. Li, B. Chen, B. Fan, B. Moine, X. S. Qiao, X. P. Fan, S.-W. Tsang, S. F. Yu and F. Wang, Broadband Ce(III)-sensitized quantum cutting in core-shell nanoparticles: Mechanistic investigation and photovoltaic application, J. Phys. Chem. Lett., 2017, 20, 5099–5104 CrossRef PubMed.
- D. L. Zhou, D. L. Liu, G. C. Pan, X. Chen, D. Y. Li, W. Xu, X. Bai and H. W. Song, Cerium and ytterbium codoped halide perovskite quantum dots: A novel and efficient downconverter for improving the performance of silicon solar cells, Adv. Mater., 2017, 42, 1704149 CrossRef PubMed.
- M. Rwaimi, C. G. Bailey, P. J. Shwa, T. M. Mercier, C. Krishnan, T. Rahman, P. G. Lagoudakis, R.-H. Horng, S. A. Boden and M. D. B. Charlton, FAPbBr3 perovskite quantum dos as a multifunctional luminescent-downshifting passivation layer for GaAs solar cells, Sol. Energy Mater. Sol. Cells, 2022, 234, 111406 CrossRef CAS.
- Y. M. Li, Q. S. Zhang, L. Y. Niu, J. Liu and X. F. Zhou, TiO2 nanorod arrays modified with SnO2-Sb2O3 nanoparticles and application in perovskite solar cell, Thin Solid Films, 2017, 621, 6–11 CrossRef CAS.
- M. X. Huang, Z. Z. Lu, L. L. Wen, X. S. Zhang, T. J. Huang, Y. B. Meng, J. J. Tang and L. Y. Zhou, Core/shell-structured TiO2/PbS phoanodes for high-performance CsPbBr3 perovskite solar cells, Mater. Lett., 2019, 256, 126619 CrossRef CAS.
- Z. H. Wu, Y. Z. Wang, Y. H. Zhang, W. J. Zhang, Q. R. Liu, Q. M. Liu, Q. Chen, Y. L. Li, J. S. Li and D. Y. He, Enhanced performance of polymer solar cells by adding SnO2 nanoparticles in the photoactive layer, Org. Electron., 2019, 73, 7–12 CrossRef CAS.
- E. J. Lee, D.-H. Kim and D.-K. Hwang, Effect of embedded chalcogenide quantum dots in PbBr2 film on CsPbBr3 inorganic perovskite solar cells, J. Ind. Eng. Chem., 2020, 90, 281–286 CrossRef CAS.
- X. H. Luo, Z. Y. He, R. W. Meng, C. Zhang, M. W. Chen, H. F. Lu and Y. P. Yang, SnS quantum dots with different sizes in active layer for enhancing the performance of perovskite solar cells, Appl. Phys. A, 2021, 127, 317 CrossRef CAS.
- P. Kumnorkaew, N. Rattanawichai, C. Ratanatawanate, S. Yoriya, K. Lohawet, Y. X. Zhao and P. Vas-Umuay, Influence of PbS quantum dots-doped TiO2 nanotubes in TiO2 film as an electron transport layer for enhanced perovskite solar cell, IEEE J. Photovoltaics, 2020, 10, 287–295 Search PubMed.
- A. Kirkeminde, R. Scott and S. Q. Ren, All inorganic iron pyrite nano-heterojunction solar cells, Nanoscale, 2012, 4, 7649–7654 RSC.
- J. S. Niezgoda, E. Yap, J. D. Keene, J. R. McBride and S. J. Rosenthal, Plasmonic CuxInyS2 quantum dots make better photovoltaics than their nonplasmonic counterparts, Nano Lett., 2014, 14, 3262–3269 CrossRef CAS PubMed.
- M. A. Najeeb, S. M. Abdullah, F. Aziz, Z. Ahmad, R. A. Shakoor, A. M. A. Mohamed, U. Khalil, W. Swelm, A. A. Al-Ghamdi and K. Sulaiman, A comparative study on the performance of hybrid solar cells containing ZnSTe QDs in hole transporting layer and photoactive layer, J. Nanopart. Res., 2016, 18, 384 CrossRef.
- F. Xu, Y. Sun, H. P. Gao, S. Y. Jin, Z. L. Zhang, H. F. Zhang, G. C. Pan, M. Kang, X. Q. Ma and Y. L. Mao, High-performance perovskite solar cells based on NaCsWO3@NaYF4@NaYF4:Yb,Er upconversion nanoparticles, ACS Appl. Mater. Interfaces, 2021, 13, 2674–2684 CrossRef CAS PubMed.
- J. Yu, Y. L. Yang, R. Q. Fan, D. Q. Liu, L. G. Wei, S. Chen, L. Li, B. Yang and W. W. Cao, Enhanced near-infrared to visible upconversion nanoparticles of Ho3+-Yb3+-F− tri-doped TiO2 and its application in dye-sensitized solar cells with 37% improvement in power conversion efficiency, Inorg. Chem., 2014, 53, 8045–8053 CrossRef CAS PubMed.
- D. Y. Li, W. F. Sun, L. X. Shao, S. Y. Wu, Z. Huang, X. Jin, Q. Zhang and Q. H. Li, Tailoring solar energy spectrum for efficient organic/inorganic hybrid solar cells by up-conversion luminescence nanophosphors, Electrochim. Acta, 2015, 182, 416–423 CrossRef CAS.
- N. N. Yao, J. Z. Huang, K. Fu, X. L. Deng, M. Ding, M. H. Shao and X. J. Xu, Enhanced light harvesting of dye-sensitized solar cells with up/down conversion materials, Electrochim. Acta, 2015, 154, 273–277 CrossRef CAS.
- N. Chander, A. F. Khan, V. K. Komarala, S. Chawla and V. Dutta, Enhancement of dye sensitized solar cell efficiency via incorporaton of upconverting phosphor nanoparticles as a spectral converters, Prog. Photovoltaics, 2016, 24, 692–703 CAS.
- M. He, X. C. Pang, X. Q. Liu, B. B. Jiang, Y. J. He, H. Snaith and Z. Q. Lin, Monodisperse dual-functional upconversion nanoparticles enabled near-infrared organolead halide perovskite solar cells, Angew. Chem., Int. Ed., 2016, 55, 4280–4284 CrossRef CAS PubMed.
- L. Liang, M. Liu, Z. W. Jin, Q. Wang, H. R. Wang, H. Bian, F. Shi and S. Z. Liu, Optical management with nanoparticles for a light conversion efficiency enhancement in inorganic γ–CsPbI3 solar cells, Nano Lett., 2019, 19, 1796–1804 CrossRef CAS PubMed.
- X. S. Lai, X. T. Li, X. D. Lv, Y.-Z. Zheng, F. L. Meng and X. Tao, Broadband dye-sensitized upconversion nanocrystals enable near-infrared planar perovskite solar cells, J. Power Sources, 2017, 372, 125–133 CrossRef CAS.
- V. Kumar, A. Pandey, S. K. Swami, O. M. Ntwaeaborwa, H. C. Swart and V. Dutta, Synthesis and characterization of Er3+-Yb3+ doped ZnO upconversion nanoparticles for solar cell application, J. Alloys Compd., 2018, 766, 429–435 CrossRef CAS.
- W. J. Shi, Z. L. Zhang, J. Q. Qin, Y. Zhang, Y. Y. Liu, Y. F. Liu, H. P. Gao and Y. L. Mao, Interface modification by up-conversion material of Ho3+-Yb3+-Li+ tri-doped TiO2 to improve the performance of perovskite solar cells, J. Alloys Compd., 2018, 754, 124–130 CrossRef CAS.
- J. W. Liang, H. P. Gao, M. J. Yi, W. J. Shi, Y. F. Liu, Z. L. Zhang and Y. L. Mao, β-NaYF4:Yb3+,Tm3+@TiO2 core/shell nanoparticles incorporated into the mesoporous layer for high efficiency perovskite solar cells, Electrochim. Acta, 2018, 261, 14–22 CrossRef CAS.
- X. S. Deng, C. X. Zhang, J. F. Zheng, X. Zhou, M. D. Yu, X. H. Chen and S. M. Huang, Highly bright Li(Gd,Y)F4:Yb,Er upconverting nanocrystals incorporated hole transport layer for efficient perovskite solar cells, Appl. Surf. Sci., 2019, 485, 332–341 CrossRef CAS.
- Q. Y. Guo, J. H. Wu, Y. Q. Yang, X. P. Liu, J. B. Jia, J. Dong, Z. Lan, J. M. Lin, M. L. Huang, Y. L. Wei and Y. F. Huang, High performance perovskite solar cells based on β-NaYF4:Yb3+/Er3+/Sc3+@NaYF4 core/shell upconversion nanoparticles, J. Power Sources, 2019, 426, 178–187 CrossRef CAS.
- Y. Y. Qin, Z. Q. Hu, B. H. Lim, W. S. Chang, K. K. Chong, P. T. Zhang and H. T. Zhang, So-hydrothermal synthesis of TiO2:Sm3+ nanoparticles and their enhanced photovoltaic properties, J. Alloy, Comp, 2016, 686, 803–809 CAS.
- L. J. Wang, W. H. Guo, H. S. Hao, Q. Su, S. S. Jin, H. Li, X. F. Hu, L. Qin, W. Y. Gao and G. S. Liu, Enhancing photovoltaic performance of dye-sensitized solar cells by rare-earth doped oxide of SrAl2O4:Eu3+, Mater. Res. Bull., 2016, 76, 459–465 CrossRef CAS.
- A. Pattnaik, S. Jha, M. Tomar, V. Gupta, B. Prasad and S. Mondal, Improving the quantum efficiency of the monocrystalline silicon solar cell using erbium-doped zinc sulphide nanophosphor in downshift layer, Mater. Res. Express, 2018, 5, 095014 CrossRef.
- M. S. Pereira, F. A. S. Lima, T. S. Ribeiro, M. R. da Silva, R. Q. Almeida, E. B. Barros and I. F. Vasconcelos, Application of Fe-doped SnO2 nanoparticles in organic solar cells with enhanced stability, Opt. Mater., 2017, 64, 548–556 CrossRef CAS.
- J. M. Luther, M. Law, Q. Song, C. L. Perkins, M. C. Beard and A. J. Nozik, Structural, optical, and electrical properties of self-assembled films of PbSe nanocrystals treated with 1,2-ethanedithiol, ACS Nano, 2008, 2, 271–280 CrossRef CAS PubMed.
- P. R. Brown, D. Kim, R. R. Lunt, N. Zhao, M. G. Bawendi, J. C. Grossman and V. Bulović, Energy level modification in lead sulfide quantum dot thin films through ligand exchange, ACS Nano, 2014, 8, 5863–5872 CrossRef CAS PubMed.
- X. S. Lin, D. Y. Cui, X. H. Luo, C. Y. Zhang, Q. F. Han, Y. B. Wang and L. Y. Han, Efficiency progress of inverted perovskite solar cells, Energy Environ. Sci., 2020, 13, 3823–3847 RSC.
- W. Chen, Y. Z. Wu, J. Liu, C. J. Qin, X. D. Yang, A. Islam, Y.-B. Cheng and L. Y. Han, Hybrid interfacial layer leads to solid performance improvement of inverted perovskite solar cells, Energy Environ. Sci., 2015, 8, 629–640 RSC.
- M. A. Haque, A. D. Sheikh, X. W. Guan and T. Wu, Metal oxides as efficient charge transporters in perovskite solar cells, Adv. Energy Mater., 2017, 7, 1602803 CrossRef.
- B. B. Liu, H. Bi, D. M. He, L. Bai, W. Q. Wang, H. K. Yuan, Q. L. Song, P. Y. Su, Z. G. Zang, T. W. Zhou and J. Z. Chen, Interfacial defect passivation and stress release via multi-active-site ligand anchoring enables efficient and stable methylammonium-free perovskite solar cells, ACS Energy Lett., 2021, 6, 2526–2538 CrossRef CAS.
- H. M. A. Javed, M. Sarfaraz, Z. Nisar, A. A. Qureshi, M. Fakhar e Alam, W. X. Que, X. T. Yin, H. S. M. Abd-Rabboh, A. Shahid, M. I. Ahmad and S. Ullah, Plasmonic dye-sensitized solar cells: Fundamentals, recent developments, and future perspectives, ChemistrySelect, 2021, 62021, 9337–9350 CrossRef.
- O. A. Balitskii, Recent Energy Targeted Application of Localized Surface Plasmon Resonance Semiconductor Nanocrystals: A Mini-Review, Mater. Today Energy, 2021, 20, 100629 CrossRef CAS.
- B. S. Richards, Enhancing the performance of silicon solar cells via the application of passive luminescence conversion layers, Sol. Energy Mater. Sol. Cells, 2006, 90, 2329–2337 CrossRef CAS.
- A. H. G. Niaki, A. M. Bakhshayesh and M. R. Mohammadi, Double-layer dye-sensitized solar cells based on Zn-doped TiO2 transparent and light scattering layers: Improving electron injection and light scattering effect, Sol. Energy, 2014, 103, 210–222 CrossRef.
- W. An, J. R. Wu, T. Zhu and Q. Z. Zhu, Experimental investigation of a concentrating PV/T collector with Cu9S5 nanofluid spectral splitting filter, Appl. Energy, 2016, 184, 197–206 CrossRef CAS.
- I. Zarma, M. Ahmed and S. Ookawara, Enhancing the performance of concentrator photovoltaic systems using nanoparticle-phase change material heat sinks, Energy Convers. Manage., 2019, 179, 229–242 CrossRef CAS.
- Z. K. Liu, Y. X. Zhong, I. Shafei, R. Borman, S. Jeong, J. Chen, Y. Losovyj, X. F. Gao, N. Li, Y. P. Du, E. Sarnello, T. Li, W. L. Ma and X. C. Ye, Tuning infrared plasmon resonances in doped metal-oxide nanocrystals through cation-exchange reactions, Nat. Commun., 2019, 10, 1394 CrossRef PubMed.
- M. G. Gong, D. Ewing, M. Casper, A. Stramel, A. Elliot and J. Z. Wu, Controllable synthesis of monodispersed Fe1-xS2 nanocrystals for high-performance optoelectronic devices, ACS Appl. Mater. Interfaces, 2019, 11, 19286–19293 CrossRef CAS PubMed.
- C. Beynis, Indium insertions in non-stoichiometric copper sulfides, CuxS, and their effect on the localized surface plasmon resonance of the nanocrystals, Appl. Phys. A, 2019, 125, 310 CrossRef CAS.
- A. Verma and S. K. Sharma, Down-conversion from Er3+-Yb3+ codoped CaMoO4 phosphor: A spectral conversion to improve solar cell efficiency, Ceram. Int., 2017, 43, 8879–8885 CrossRef CAS.
- Y. Q. Yin, M. Q. Wang, V. Malgras and Y. Yamauchi, Stable and efficient Tin-based perovskite solar cell via semiconducting-insulating structure, ACS Appl. Energy Mater., 2020, 3, 10447–10452 CrossRef CAS.
- Y. X. Ye, Y. Q. Yin, Y. Chen, S. Li, L. Li and Y. Yamauchi, Metal-organic framework materials in perovskite solar cells: Recent advancements and perspectives, Small, 2023, 19, 2208119 CrossRef CAS PubMed.
|
This journal is © The Royal Society of Chemistry 2024 |
Click here to see how this site uses Cookies. View our privacy policy here.