DOI:
10.1039/D3MO00167A
(Research Article)
Mol. Omics, 2024,
20, 48-63
Cronobacter sakazakii infection implicates multifaceted neuro-immune regulatory pathways of Caenorhabditis elegans†
Received
23rd August 2023
, Accepted 25th September 2023
First published on 28th September 2023
Abstract
The neural pathways of Caenorhabditis elegans play a crucial role in regulating host immunity and inflammation during pathogenic infections. To understand the major neuro-immune signaling pathways, this study aimed to identify the key regulatory proteins in the host C. elegans during C. sakazakii infection. We used high-throughput label-free quantitative proteomics and identified 69 differentially expressed proteins. KEGG analysis revealed that C. sakazakii elicited host immune signaling cascades primarily including mTOR signaling, axon regeneration, metabolic pathways (let-363 and acox-1.4), calcium signaling (mlck-1), and longevity regulating pathways (ddl-2), respectively. The abrogation in functional loss of mTOR-associated players deciphered that C. sakazakii infection negatively regulated the lifespan of mutant worms (akt-1, let-363 and dlk-1), including physiological aberrations, such as reduced pharyngeal pumping and egg production. Additionally, the candidate pathway proteins were validated by transcriptional profiling of their corresponding genes. Furthermore, immunoblotting showed the downregulation of mTORC2/SGK-1 during the later hours of pathogen exposure. Overall, our findings profoundly provide an understanding of the specificity of proteome imbalance in affecting neuro-immune regulations during C. sakazakii infection.
1 Introduction
Understanding the molecular mechanisms involved in the host-pathogen interaction (HPI) is important for identifying the immune effectors potentially acting towards the pathogen.1 Generally, the host's innate immune response is the first line of defense against pathogen attacks. The nematode Caenorhabditis elegans is a highly considered model to study many human pathogens, as a wealth of data has proven that various cell biological, neuronal, and developmental processes are highly conserved between C. elegans and humans.2,3 In this perspective, C. elegans is widely used as a model organism to study host–pathogen interactions and elucidate the host innate immunity.4C. elegans can be controlled precisely over the presence of microbes, thereby enabling robust and simple infection assays that allow unbiased exploration of the interactions between bacterial pathogens and C. elegans can be developed.5
The present study investigated the pathogenicity of C. sakazakii using the model organism C. elegans. The members of the genus Cronobacter (formerly Enterobacter) belonging to the Enterobacteriaceae family are a class of Gram-negative facultative anaerobic bacteria that are all considered potentially pathogenic according to FAO/WHO.6 The consumption of a C. sakazakii—contaminated formula often results in life-threatening necrotizing enterocolitis and meningitis in preterm neonates, and neurodegenerative diseases by translocating their virulence factors through the blood–brain barrier (BBB) of the host bloodstream.7–9 The virulence of this pathogen is predominantly strengthened by its ability to exhibit various adaptation mechanisms to extreme environments, such as heat, acidity, and osmotic stress. The pathogenicity of C. sakazakii is mostly associated with its outer membrane protein A (OmpA), OmpX, InvA, and zinc metalloprotease. These factors are responsible for the bacterial adherence and invasion of human intestinal and brain epithelial cells.10–12 Despite the plethora of studies on virulence factors of C. sakazakii using whole-genome sequencing and other omics methods, the exact molecular mechanism of C. sakazakii in the host remains unclear. Diagnosing the etiology and identifying the causative pathogen are vital to make strides in control measures.
The human brain is composed of about 86 billion neurons and millions of synapses and gap junctions, and thus, studying neuro-immune interactions in humans is difficult with available technologies.13 The problems associated with neurological diseases caused by infection remain lacking, a vacuity of knowledge to humans. C. elegans provides greater accessibility to invertebrate neuroscience studies, especially with the limited number of neurons activating the neuro-specific immune signaling pathways during pathogen infections.14 Unlike vertebrates, C. elegans lacks an adaptive immune system, relying solely on several evolutionary conserved innate immune signal transduction pathways that are involved in pathogen defense including the p38/PMK-1 mitogen-activated protein kinase (MAPK) pathways, DAF-2/DAF-16 insulin-like signaling pathways, DBL-1/transforming growth factor β pathway, Unfolded Protein Responses (UPRs), TGF-β pathway, and programmed cell death.14–17 However, the complete analysis of these immune pathways for the invaded pathogens is still uncovered due to the lack of understanding of exacerbating infections during insufficient or excessive immune responses. Also, the downstream alterations result in prolonged inflammation, tissue damage, or even death in various pathogenic infections.
C. elegans has a limited number of neurons with completely explored and well-mapped neuronal connections, producing a large repertoire of neurotransmitters, neuropeptides, and neurohormones.18 Consequently, it confers remarkable specificity to different pathogens, indicating that the innate immune responses can generate high levels of specificity during host–pathogen interactions. A fine example, neuronal GPCRs controlling the p38/PMK-1 MAPK pathway independently depend on the pathogen-specific defense responses.19,20 The neuronal bioamines are considered a potential target for treating infectious diseases. Further studies have shown that the inhibition of biogenic amines such as dopamine and serotonin in the nervous system triggers the immune response against pathogen infections.21,22 In addition, the intertwined functions of the nervous and immune systems parse out possible confounding variables by regulating the immune signaling pathways upon pathogen infection.
In studies of C. sakazakii interactions, C. elegans has been used as a host.23–26 Albeit the transcriptional and metabolomic level studies have been reported by our research group, we aimed to approach more specifically to the functional molecular players involved in crucial neuro-immune signaling pathways. The protein players integrated into the immune system via neuronal signals rapidly contribute to the defense mechanism during the HPI.27 Although earlier studies provided less information on host immune regulation, proteomics profiling that executes the expression of genetic information will enable to assess the required information on how bacterial virulence manifests the neuro-immune communication of the host organism. The HPI-induced proteostasis involves a complex network of factors that mitigate aberrant native protein functions and this network is progressively challenged between the neurons and innate immune signaling pathways.28
To better understand the infectious nature of C. sakazakii, the protein profiling of C. elegans during the prolonged exposure of C. sakazakii using a label-free quantitative LC-MS/MS approach was followed. In this study, we tried to answer the following questions: (a) What are the major neuro-immune signaling pathway proteins and their respective genes that get regulated during infection? and (b) What happens to the host when the major neuro-immune signalling pathway proteins are either up or downregulated? Undeniably, this study aims to give more insights into the relationship between the molecular mechanisms of the neuro-immune signaling pathway and inflammatory response proteins during C. sakazakii infection in a well-established animal model system.
2 Materials and methods
2.1 Bacterial strains and growth conditions
The starter culture of Escherichia coli OP50 was procured from Caenorhabditis Genetics Center (CGC, Minneapolis, MN, USA) and was maintained in Luria Bertani (LB) agar. The pathogen of interest C. sakazakii ATCC 29544 was obtained from the American Type Culture Collection (ATCC), Manassas, Virginia, USA, and was maintained in tryptic soy (TS) agar. For routine experiments, E. coli was sub-cultured in LB broth and C. sakazakii in the TS broth and was grown at 37 °C and 30 °C, respectively. Further, bacterial cultures with an OD600 of ∼0.2 were used for all the performed experiments.
2.2 Maintenance of C. elegans strains
The growth and maintenance of all the C. elegans strains were followed as previously described by Brenner.29 Briefly, the CGC obtained wild-type Bristol N2 and other mutant strains were grown and maintained at 20 °C and 15 °C respectively in the standard nematode growth medium (NGM) seeded with E. coli as a food source. For all the experiments, the worms were age synchronized at larval stage 4 (L4) by bleaching (using a 1
:
1 ratio of NaOCl and 5 M KOH) the hermaphrodite worms in their gravid stage. The details of the different C. elegans strains used in this study are BQ1 (akt-1), VC2312 (let-363), and CZ5730 (dlk-1).
2.3 Infection process and protein sample preparation
The total proteome extraction from C. sakazakii and E. coli—fed C. elegans was performed as described previously.30 Briefly, the L4 stage-synchronized wild-type N2 worms (∼1000 per sample) were exposed to 20% inoculum of bacteria (E. coli or C. sakazakii) in a 80% sterile M9 buffer (3 g KHPO4, 6 g Na2HPO4, 5 g NaCl, 1 ml 1 M MgSO4 and 1000 ml H2O) and incubated at 20 °C for 24 and 48 h. After the incubation period, the worms were washed using an M9 buffer and collected as compact pellets, which were then resuspended in a urea buffer (7 M urea, 20 mM Tris–HCl, pH 8.5, 2 M thiourea, and 2% (w/v) CHAPS) containing 1
:
100 ratio of 1 mM phenylmethanesulphoyl fluoride (PMSF) as a protease inhibitor. The samples were sonicated using a Vibracell ultrasonic processor-VCX 750 for about 1 min (5 s on, 10 s off), and the homogenate was centrifuged at 10
000g for 5 min. The supernatant was retained in a new sterile microfuge tube and the total protein concentration was determined using Bradford's method with bovine serum albumin as the standard.31 All the steps were carried out under ice-cold conditions to prevent protein denaturation or degradation.
2.4 In-solution digestion for mass spectrometry
The samples’ concentration used was ∼100 μg per sample, and a 2D cleanup kit from GE Healthcare was used to precipitate them according to the manufacturer's instructions. The lysates were then reduced with 100 mM DTT at 55 °C for 30 min in the darkness and alkylated with 200 mM iodoacetamide at 37 °C. The samples were then digested overnight (in a wet chamber at 37 °C) with sequencing grade trypsin (Sigma-Aldrich) in 50 mM ammonium bicarbonate buffer, with a protein-to-trypsin proportion of 100
:
1. The digestion was stopped by adding trifluoroacetic acid (TFA) to a final concentration of 1% (v/v).32 The digested peptide mixture from both the control and infected samples was desalted using Pierce™ C18 Spin Tips from Thermo Fisher Scientific, according to the manufacturer's protocol. This step involves passing the sample through a C18 resin column to remove impurities and salts, leaving behind the purified peptides.
2.5 LC-MS/MS analysis
The LC-MS/MS analysis was performed using the parameters described earlier.33 Briefly, 10 μl of samples were injected for the separation of peptides in liquid chromatography–mass spectrometry (Agilent 6545 ESI-LC/qToF-MS/MS system). The separation was achieved using a gradient method with a solvent system consisting of 0.1% (v/v) formic acid and 90% ACN, and the total run time was 90 minutes. The resulting data were processed using the Mass Hunter Workstation software (version B.08.00), and the post-processing of data was performed using the Spectrum Mill software (version B.06.00). The database searching was performed using the C. elegans database, and the precursor mass tolerance was set to 20 ppm, while the product mass tolerance was set to 600 mDa. Trypsin was selected as the primary enzyme with a specificity of at least one missed cleavage site. Additionally, carbamidomethylation (C) of cysteine was selected as a fixed modification, and oxidation of methionine (M) was selected as the variable modification for database searching.
The mass spectrometry proteomics data have been deposited to the ProteomeXchange Consortium via the PRIDE34 partner repository with the dataset identifiers PXD041315.
2.6 Bioinformatics analysis
The high-throughput LC-MS/MS identified differentially regulated proteins were further subjected to the construction of protein–protein interaction (PPI) networks using the STRINGV11.5 database.35 The minimal interaction score was set to “high confidence”. The interactome network was selected for both the functional (indirect) and physical (direct) protein associations to predict the differentially regulated proteins and their association. STRING-generated networks were further analyzed for Gene Ontology (GO) groups, and the GO term subcategories according to biological processes, cellular components, molecular functions, and KEGG pathways were also plotted. Further, to identify the commonly regulated proteins, a Venn diagram was constructed using Venny 2.1.0 (https://bioinfogp.cnb.csic.es/tools/venny/).
2.7 Lifespan, pharyngeal pumping, and egg-laying assays
The methodology to determine the lifespan, pharyngeal pumping and egg-laying of the control and infected worms was followed as described previously.36 Briefly, 10–30 numbers of age-synchronized L4 populations (both wild-type and mutant worms) were transferred to a 24-well microtiter plate (MTP) containing 400 μl of M9 buffer with ∼0.2 OD of 20% bacterial culture and 50 μM of 5-fluoro-2′-deoxyuridine (FUdR, Sigma; to prevent self-fertilization) and incubated at 20 °C. Here, the wild-type worm (N2 Bristol) was used as a control for C. sakazakii infection. For an experiment, the day worms were transferred to the MTP was set as day 0 and the assay was monitored every 24 h and the live worms were counted. Worms were considered dead if they failed to respond to physiological stimuli either by gentle tapping at the side of the MTP or by gently prodding using a platinum wire worm picker.
For the pharyngeal pumping assay, the pharyngeal pumping rate from control and infected worms was observed using a stereomicroscope with a focus on the pharynx. The number of contractions of the pharyngeal bulb of individual worms was counted over 10 consecutive seconds. The age-synchronized worms (n = 30) were transferred to fresh NGM plates with bacterial lawn (E. coli: control; C. sakazakii: infected). The worms were allowed to lay eggs and were transferred to a fresh NGM plate every 24 h until egg-laying had ceased because of the pathogen. All the experiments and counting were performed in three independent biological triplicates.
2.8 RNA isolation and quantitative PCR (qPCR)
To find the change in expression at transcriptional levels, the total RNA was isolated and qPCR was performed as described earlier.37 Briefly, the L4 stage-synchronized worms were fed with either E. coli or C. sakazakii for durations of 12, 24, 48, and 72 h as mentioned above. The control and infected worms were gently washed with an M9 buffer, and the total RNA was extracted using an RNA X-Press reagent (HiMedia) and was reverse transcribed into cDNA using an RT-PCR kit (Invitrogen) according to the manufacturer's instructions. The qPCR analysis was performed for the candidate genes (listed in Table 1) with the transcribed cDNA using an SYBR™ Green kit (Applied Biosystems) in an Applied Biosystems® 7500 Real-Time system (USA). The β-act gene of C. elegans was used as an internal control. Thermo cycling conditions for qPCR are as follows: initial denaturation at 95 °C for 10 min followed by 40 cycles of 95 °C for 20 s, annealing at 60 °C for 20 s, extension at 72 °C for 30 s, followed by melt curve analysis. The experiments were independently performed at least three times. The differential gene expression (DEG) level was calculated by the 2−ΔΔCT method.38 The gene expression analysis was carried out in biological triplicate.
Table 1 List of primers used for real-time qPCR in this study
Target gene |
Forward primers (5′-3′) |
Reverse primers (5′-3′) |
Ref. |
let-363
|
GGTGTTGAATGGCTTGTCCT |
ATCGAGCTTTGCTGTTTCGT |
Zhang and Zhang 2013 |
mlck-1
|
CCAGTGACTCAAGACGCAAA |
CTGCAGCCAAAAGACTTTCC |
Wang et al. 2018 and Gao et al. 2022 |
acox-1.4
|
AACACCCCAACGACTACAGC |
GGCACTCGGAATTTATCGAA |
Shimizu et al. 2022 |
ddl-2
|
ATCAGCAAGCTCCTCCGTAA |
ACGACTCAAGAATCCCATCG |
Chiang et al. 2012 |
act-2
|
ACGCCAACACTGTTCTTTCC |
TTCATGGTTGATGGGGCAAG |
Kamaladevi and Balamurugan. 2017 |
2.9 Cell death staining during the infection
Propidium iodide was used to stain late necrosis or dead cells and the assay was conducted according to the reported protocol with slight modifications.39 Briefly, L4 stage-synchronized N2 worms were exposed to C. sakazakii or E. coli for 48 and 72 h and washed thoroughly with an M9 buffer. The worms were stained with propidium iodide (Sigma) at a final concentration of 10 μM in M9 buffer and incubated for 2 hours at room temperature in a horizontal shaker at 50 rpm. The images of individual worms from both control and infected were acquired using a Fluorescence Microscope (Eclipse Ts2R, Nikon, Tokyo, Japan) at 20× magnification equipped with a Nikon DS-Fi3 Color Camera and the NIS-Elements F capture software with identical parameter settings.
2.10 Western blotting
To validate the proteomics data, western blot analysis was carried out as described previously.40,41 In brief, 50 μg of total protein from the L4 stage wild-type worms exposed to C. sakazakii or E. coli during different time periods were separated by 12% SDS-PAGE gels and transferred onto a polyvinylidene fluoride (PVDF) membrane using a semidry system according to the manufacturer's instructions (Amersham Biosciences). The membranes were probed with the following commercially available antibodies: anti-β-actin (4967L-Cell Signaling Technology, Inc.), Anti-SGK-1 (Sc-33774, Santa Cruz Biotechnology, Inc.), and Anti-Rabbit IgG (A8025, Sigma -Aldrich). The working concentration of primary antibodies was kept at 1
:
1000 dilutions and for secondary antibodies at 1
:
5000 dilutions. The PVDF membranes were blocked with 5% skimmed milk in TBS plus 0.1% Tween 20 (TBST) overnight, and then incubated with their specific primary antibodies for 8 h, followed by the secondary antibody at 4 °C for 4 h. The membranes were developed by transferring to 1× AP buffer (alkaline phosphatase) developing solutions.42 The blots were imaged using Image Lab 4.0 (Bio-Rad), and quantitative analysis was performed using the ImageJ software. The mean values and standard deviation were calculated and normalized against β-actin. All of the experiments were repeated with at least three independent biological replicates.
2.11 Statistical analysis
All the experiments were performed in three biological replicates with a minimum of three experimental triplicates. All the data shown are presented as mean ± standard deviation (SD). Analysis of variance (ANOVA) followed by Duncan's post hoc test was performed using GraphPad Prism 8.0 (GraphPad Prism Software Inc., San Diego, CA, USA) to determine the significance among groups and within groups, respectively. Differences were considered significant at *p
<
0.05, **p
<
0.01, and ***p
<
0.001.
3 Results
3.1 Label-free quantitative proteomic analysis of C. sakazakii—infected C. elegans charts the immune regulatory pathway players
Initially, we aimed to catalogue the proteins involved in neuro-signaling pathways associated with immune homeostasis of control and C. sakazakii—infected worms upon 24 and 48 h of infection. The protein profiling of both control and infected worms by LC-MS/MS analysis revealed a total of 174 proteins in control (Table S1, ESI†), 206 in C. sakazakii (CS) infected (Table S2, ESI†) at 24 h, and 172 proteins in control (Table S3, ESI†) and 226 in C. sakazakii infected (Table S4, ESI†) at 48 h samples, respectively. All the obtained proteins were curated using the UniProt (UniProtKB) database. The identified proteins of control and infected worms were subjected to STRINGv11.5 analysis, and protein–protein interaction networks were constructed for both 24 and 48 h samples from control and infection (Fig. 1A and B). Both networks were constructed with the highest confidence threshold value of 0.900 to identify the possible functional and physical protein associations. The PPI network of 24 h had more network edges with a higher number of nodes, and nearly all the proteins exhibited multiple interactions with their associated proteins. In contrast, the 48 h network was observed to have a lesser number of nodes and edges. This may be due to the loss of several functional proteins in the 48 h sample albeit 48 h C. sakazakii—infected samples had had more proteins (226) than 24 h C. sakazakii—infected samples (206). Further, the common proteins from both time points were identified by Venn analysis. A threshold value ≥2.00 for upregulated proteins and ≤2.00 for downregulated proteins was assigned. The Venn diagram analysis revealed the presence of 42 differentially expressed proteins (DEPs) in 24 h (Fig. 2A), including 10 (23.8%) upregulated and 32 (76.1%) downregulated proteins (Table 2) in common. Meanwhile, 27 DEPs were observed in 48 h (Fig. 2B), including 6 (22.2%) upregulated and 21 (77.7%) downregulated proteins (Table 3). Further, the commonly regulated proteins were analysed for stable groups of similar proteins especially for functional annotations. The 24 h network analysis revealed a higher clustering coefficient of 0.3 and an average node degree of ∼0.96. Meanwhile, for 48 h, the average higher clustering coefficient is 0.40 and the average node degree is ∼0.91. Totally, three tight clusters (green, blue, and red) were generated from STRING. In 24 h, DEPs revealed the cluster red color has 16, green has 24 and blue has 10 members (Fig. 2C). In 48 h DEPs revealed the cluster members as 15 from green, 10 from blue and 12 from red clusters (Fig. 2D). The gene ontology (GO) classification of all identified high-confidence common proteins from 24 and 48 h was simultaneously analyzed (Fig. 3A and B). The proteome composition of the cellular component (CC) of a major group of annotated proteins at different time points (24 and 48 h) was found to predominantly encode the cellular anatomical entity (CC, GO: 0110165), intracellular organelle (CC, GO: 0043229), cytoplasm (CC, GO: 0005737), protein-containing complex (CC, GO: 0032991) and membrane-bounded organelle (CC, GO: 0043227), as well as cytoplasm (CC, GO: 0005737) (Tables S5b and S6b, ESI†), whereas GO of DEPs revealed that these proteins are involved in various biological processes (BP) (Tables S5c and S6c, ESI†), and molecular functions (MF) (Tables S5a and S6a, ESI†) exhibited specific functions such as binding function (MF, GO: 0005488), catalytic activity (MF, GO: 0003824), organic cyclic compound binding (MF, GO: 0097159), heterocyclic compound binding (MF, GO: 1901363), and ion binding (MF, GO: 0043167). Based on the biological process (BP), the identified proteins and their interacting partners were highly enriched in the determination of adult lifespan (BP, GO: 0008340), metabolic (BP, GO: 0008152), cellular (BP, GO: 0009987), organic substance metabolic (BP, GO: 0071704), and other primary metabolic (BP, GO: 0044238) processes.
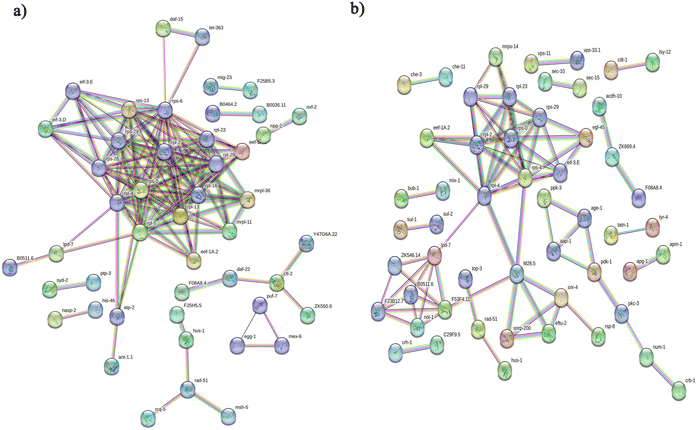 |
| Fig. 1 Protein–protein interaction (PPI) network analysis of functional proteins identified from LC-MS/MS. PPI was identified using the STRINGv11.5 software with the highest confidence score (0.9) and disconnected nodes were hidden. The protein networks were constructed for both control and C. sakazakii-exposed worms at 24 h (A) and 48 h (B) time points. Nodes are proteins, and the known interactions of the nodes in the image represent experimentally determined and curated databases. Each node linked with different color-coded lines represents six types of evidence used in prediction associations including neighborhood evidence (green), fusions evidence (red), co-occurrence evidence (blue), textmining evidence (yellow), co-expression evidence (black) and protein homology evidence (light-blue). | |
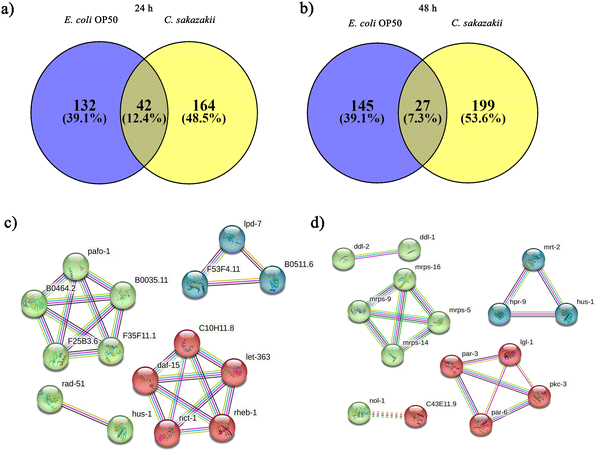 |
| Fig. 2 Analysis of differentially regulated proteins from E. coli OP50 and C. sakazakii. Venn diagram to compare the compositions of proteins identified in worms fed on E. coli OP50 and C. sakazakii. The numbers in the Venn diagram indicate the number of proteins identified at least once in each protein sample (OP50 or C. sakazakii). The representative diagram suggests that 42 common elements were present among the 24 h interactome (A) and 27 common elements were present between the 48 h interactome (B). Each color (green, red, and blue) of the PPI clusters from 24 h (C) and 48 h (D) time points suggests the functional link between the proteins. The average local clustering coefficient is 0.3 for 24 h and 0.405 for 48 h. | |
Table 2 List of upregulated proteins (more than 2 folds) at 24 h identified by LC–MS/MS during C. sakazakii exposure when compared to the control
Accession |
Max fold change |
Mass (Da) |
Protein name |
Gene name |
Q17984 |
9.16 |
22635.2 |
Uncharacterized protein |
C14E2.3
|
O16237 |
8.97 |
231090 |
LysM Domain (Peptidoglycan binding) protein |
lmd-4
|
B1Q250 |
7.43 |
60142.4 |
UDP-glucuronosyltransferase (EC 2.4.1.17) |
lmd-4
|
Q95Q95 |
3.25 |
308805.6 |
Target of rapamycin homolog (EC 2.7.11.1) (CeTOR) (lethal protein 363) |
let-363
|
P91061 |
3.18 |
30976.8 |
Uncharacterized protein |
C16C8.18
|
Q09506 |
2.68 |
13532.4 |
Tax1-binding protein 3 homolog |
txbp-3
|
Q4TT88 |
2.56 |
108004.1 |
Puromycin-sensitive aminopeptidase (PSA) (EC 3.4.11.14) (cytosol alanyl aminopeptidase) (AAP-S) |
pam-1
|
Q09560 |
2.17 |
109608.3 |
Uncharacterized protein F36G3.1 |
F36G3.1
|
H2L075 |
2.07 |
461741.4 |
Kettin homolog |
ketn-1
|
H2L034 |
2.01 |
69685.1 |
ABC transmembrane type-1 domain-containing protein |
pmp-5
|
Q23142 |
1.55 |
29865.5 |
Uncharacterized protein |
CELE_W03B1.3
|
Q95Y89 |
1.23 |
61967 |
Pescadillo homolog (lipid depleted protein 7) |
lpd-7
|
G5EGG8 |
1.15 |
39478.2 |
DNA repair protein RAD51 homolog |
rad-51
|
Q09633 |
1.14 |
55695.2 |
RNase H type-1 domain-containing protein |
rnh-1.1
|
H2KZ70 |
0.98 |
37978.9 |
Coiled-coil domain-containing protein 51 |
CELE_ZC477.3
|
O62427 |
0.95 |
29261.6 |
RING-type domain-containing protein |
CELE_Y38H8A.2
|
Q09400 |
0.95 |
53610.1 |
Uncharacterized protein F58F12.3 |
F58F12.3
|
Q18020 |
0.92 |
46791.3 |
Aspartic protease 10 (EC 3.4.23.-) (Heme transporter hrg-7) (Heme-responsive gene 7 protein) |
hrg-7
|
P0DM41 |
0.85 |
42137.4 |
Actin-1 (EC 3.6.4.-) |
act-1
|
Q19683 |
0.85 |
46529.3 |
Uncharacterized protein F21D5.5 |
F21D5.5
|
Q27GV2 |
0.83 |
20595.3 |
Uncharacterized protein ZK688.12 |
ZK688.12
|
O62139 |
0.83 |
74997.9 |
Acyl-coenzyme A oxidase acox-1.4 (EC 1.3.3.-) |
acox-1.4
|
Q22790 |
0.82 |
17905.7 |
DUF4604 domain-containing protein |
CELE_T25G3.1
|
P34454 |
0.82 |
48273.5 |
Uncharacterized protein F54F2.9 |
F54F2.9
|
Q9U374 |
0.80 |
11421.3 |
Uncharacterized protein |
CELE_T10B10.9
|
Q7YXD2 |
0.76 |
35646.7 |
Delta-like protein |
dsl-7
|
Q17431 |
0.74 |
48062.5 |
RNA polymerase-associated protein LEO1 |
leo-1
|
Q20085 |
0.72 |
54501 |
Membrane-associated tyrosine- and threonine-specific cdc2-inhibitory kinase wee-1.1 (EC 2.7.11.1) (Myt1 kinase) |
wee-1.1
|
D7SFK4 |
0.71 |
136188.4 |
Lin-12 and Glp-1 X-hybridizing |
lgx-1
|
Q18563 |
0.53 |
80126.6 |
Regulator of G-protein signaling rgs-6 |
rgs-6
|
Q9N4P3 |
0.51 |
63688.4 |
R3H domain-containing protein |
CELE_ZK121.2
|
V6CLB7 |
0.49 |
38009.2 |
G Protein, alpha subunit |
gpa-18
|
Q8MNV7 |
0.49 |
78385 |
SWI/SNF-related matrix-associated actin-dependent regulator of chromatin subfamily A-like protein 1 homolog (EC 3.6.4.-) |
smrc-1
|
Q7YXB5 |
0.47 |
42523.7 |
Carbohydrate sulfotransferase |
CELE_Y87G2A.16
|
Q17930 |
0.44 |
40860 |
DUF19 domain-containing protein |
C12D5.9
|
Q09989 |
0.44 |
55502.8 |
SynDaPiN (synaptic dynamin binding protein) homolog |
sdpn-1
|
A0A163UTE6 |
0.39 |
95989.7 |
Dilute domain-containing protein |
hum-2
|
H2KZ09 |
0.38 |
55271.1 |
Serpentine receptor, class SX |
srsx-34
|
G5EFI9 |
0.37 |
31791.1 |
Checkpoint protein |
hus-1
|
F5GU62 |
0.29 |
37959.1 |
Uncharacterized protein |
CELE_T01B6.1
|
G5EEA7 |
0.28 |
85072.4 |
Anion transporter SULP-4 (STAS domain-containing protein) |
sulp-4
|
O17744 |
0.27 |
94171.3 |
Uncharacterized protein |
CELE_E03H4.4
|
Table 3 List of upregulated proteins (more than 2 folds) at 48 h identified by LC–MS/MS during C. sakazakii exposure when compared to the control
Accession |
Max fold change |
Mass (Da) |
Protein name |
Gene name |
Q23260 |
10.2 |
136062 |
Protein kinase domain-containing protein |
mlck-1
|
P91061 |
7.86 |
30976.8 |
Uncharacterized protein |
C16C8.18
|
Q19022 |
5.72 |
22641.4 |
Uncharacterized protein |
CELE_E01G6.2
|
B7FAS5 |
2.66 |
87766.2 |
Rab-GAP TBC domain-containing protein |
tbc-17
|
Q9N5I2 |
2.59 |
37267.8 |
Serpentine receptor, class Z |
CELE_K09D9.3
|
Q19266 |
2.58 |
68832.6 |
Protein kinase C-like 3 (EC 2.7.11.13) (Atypical protein kinase C-3) (aPKC3) |
pkc-3
|
Q09400 |
1.98 |
53610.1 |
Uncharacterized protein F58F12.3 |
F58F12.3
|
Q9N5U6 |
1.94 |
29003.3 |
Uncharacterized protein |
CELE_F35F11.2
|
O62139 |
1.49 |
74997.9 |
Acyl-coenzyme A oxidase acox-1.4 (EC 1.3.3.-) |
acox-1.4
|
O17744 |
1.34 |
94171.3 |
Uncharacterized protein |
CELE_E03H4.4
|
Q19812 |
1.33 |
29126.7 |
Uncharacterized protein |
CELE_F26F12.2
|
Q9N4P3 |
1.32 |
63688.4 |
R3H domain-containing protein |
CELE_ZK121.2
|
Q9XXS2 |
1.26 |
40441.2 |
SET domain-containing protein |
set-22
|
G5ECX9 |
0.955 |
32727.4 |
CaDmium responsive (Cadmium-inducible lysosomal protein CDR-2) |
cdr-2
|
H2L075 |
0.929 |
461741.4 |
Kettin homolog |
ketn-1
|
Q09633 |
0.772 |
55695.2 |
RNase H type-1 domain-containing protein |
rnh-1.1
|
O62116 |
0.495 |
40667.8 |
Nuclear hormone receptor family |
nhr-81
|
G5EBH3 |
0.468 |
460349.8 |
SMAll |
sma-1
|
Q09506 |
0.452 |
13532.4 |
Tax1-binding protein 3 homolog |
txbp-3
|
O18195 |
0.439 |
54636.5 |
WASH complex subunit homolog 1 |
ddl-2
|
G5EFI9 |
0.4 |
31791.1 |
Checkpoint protein |
hus-1
|
Q09989 |
0.348 |
55502.8 |
SynDaPiN (synaptic dynamin binding protein) homolog |
sdpn-1
|
Q9TYV5 |
0.335 |
74303.1 |
26S rRNA (cytosine-C(5))-methyltransferase nsun-1 (EC 2.1.1.-) (5-methylcytosine rRNA methyltransferase nsun-1) (NOL1/NOP2/Sun domain family member 1) (RNA cytosine C(5)-methyltransferase nsun-1) (rRNA cytosine C(5)-methyltransferase nsun-1) |
nsun-1
|
H2L0B0 |
0.283 |
46776.7 |
NR LBD domain-containing protein |
nhr-104
|
Q09560 |
0.24 |
109608.3 |
Uncharacterized protein F36G3.1 |
F36G3.1
|
Q9TZE1 |
0.15 |
51746.1 |
MFS domain-containing protein |
CELE_W04C9.6
|
P49391 |
0.0595 |
23470.7 |
Probable 40S ribosomal protein S14, mitochondrial (MRP-S14) (S14mt) |
mrps-14
|
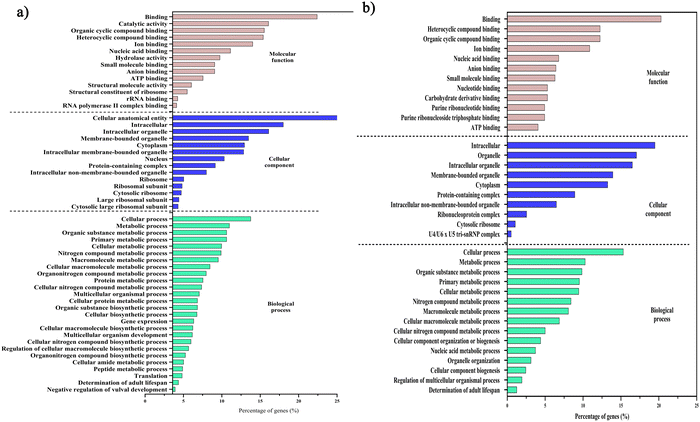 |
| Fig. 3 Gene ontology classifications of the high-confidence proteins from PPI network analysis. The GO analysis of 24 h (A) and 48 h (B) samples between E. coli OP50 and C. sakazakii includes biological processes (light-green), cellular components (blue), and molecular functions (apple blossom). The percentage of proteins enriched in each category is indicated. Raw information on GO terms and their respective false discovery rates are provided in ESI,† Tables S5 and S6. | |
Additionally, the unique protein cluster network analysis of C. sakazakii—infected samples (Fig. S3A and B, ESI†) revealed a high clustering coefficient of 0.342 (24 h) and 0.285 (48 h), and the average node degree of 1.39 (24 h) and 1.24 (48 h). The higher clustering coefficient suggests that those unique proteins showed interaction between protein metabolic process, nucleic acid binding, microtubule cytoskeleton, and ribonucleoprotein complex proteins. Overall, GO analysis suggests that the C. sakazakii infection interferes with the fundamental biological and molecular processes of the host system to make the host more susceptible to the infection (Fig. S3C, ESI†).
3.2 Pathway analysis of immune-regulated proteins during C. sakazakii infection
The DEPs were further investigated by the Kyoto Encyclopedia of Genes and Genomes (KEGG) pathway enrichment analysis, and accordingly, 18 pathways were observed represented by four unique proteins (acyl-coenzyme A oxidase (ACOX-1.4), uncharacterized protein (F54F2.9), mammalian target of rapamycin homolog (LET-363), and ABC transmembrane type-1 domain-containing protein (PMP-5) in the 24 h sample (Table S5d, ESI†). Further, the overall fold change at the protein level is provided in Tables 2 and 3. Of the four, two proteins, namely, ACOX-1.4 and PMP-5 belonged to the peroxisome pathway. One of the regulated proteins, the target of rapamycin homolog coded by LET-363 was predicted to be occurring in the mTOR signaling, ErbB signaling, autophagy, axon regeneration, and longevity regulating in worms and multiple other species pathways. In addition, ACOX-1.4 was found to be involved in the pathways associated with fatty acid, unsaturated fatty acids, carbon metabolism, alpha-linolenic acid, beta-alanine, and propanoate metabolisms. Interestingly, two of the identified major regulating proteins, LET-363 and ACOX-1.4, were found to have a major contribution in KEGG-identified pathway proteins, which suggests that C. sakazakii infection makes the host system susceptible by regulating the both neuronal signaling and the immune pathways. Hence, these proteins were selected for further characterization studies.
Meanwhile, in the 48 h samples, 13 pathways were predicted with the KEGG pathway-associated proteins (Table 3). Notably, ACOX-1.4 was found to be predominantly expressed in nine of the total pathways (Table S6, ESI†). Additionally, the protein kinase domain-containing protein (MLCK-1) was regulated in the calcium signaling pathway, which is associated with the intestinal immune barrier function. The daf-16-dependent longevity gene (DDL-2) may regulate via the longevity regulating pathway of the worm and endocytosis pathways. These pathway analyses suggest that C. sakazakii infection induced the host proteome by regulating the neuronal signaling and immune pathways such as mTOR, axon regeneration and longevity regulating pathways.
3.3 LET-363/mTOR negatively regulates C. elegans lifespan through the mTOR signaling and Axon regeneration pathways
We further investigated the functions of DEPs in neuro-immune signaling-associated pathways during C. sakazakii infection. The candidate protein LET-363 was selected to further validate its function on longevity and involvement in the PI3K/AKT/mTOR signaling pathway. The mTOR has two complexes (mTORC1 and mTORC2) that regulate several cellular and metabolic processes in a normal system.43 However, the neuro-immune communication via mTOR signaling remains unclear. To evaluate whether the candidate pathogen C. sakazakii influenced the host immune defense, C. elegans mutants BQ-1(akt-1), VC2312 (let-363), and CZ5730 (dlk-1) were fed with C. sakazakii or E. coli, and their longevity was measured. As demonstrated in Fig. 4A–C, mutants showed lower survival rates than those of wild-type strains during C. sakazakii infection. We analyzed the egg-laying behavior of mutants, where BQ1 completely stopped laying eggs at day 5, while VC2312 was at day 4 (Fig. S1A and B, ESI†). Further, these mutant worms showed a significant increase in pharyngeal pumping during the initial 24 h and declined later with an increase in bacterial colonization (Fig. S2A and B, ESI†).
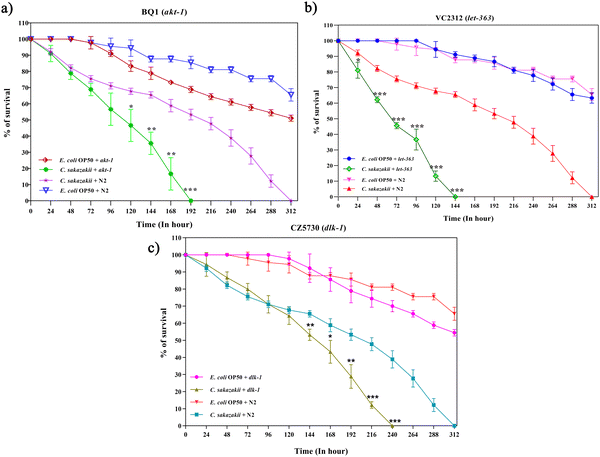 |
| Fig. 4 Protein misfolding of PI3K/AKT/mTOR and axon regeneration pathway proteins differentially affects C. elegans survival during C. sakazakii infection. Wild-type (N2), BQ1 (akt-1), VC2312 (let-363), and CZ5730 (dlk-1) worms shown in the graphs denote the killing kinetics of worms exposed to E. coli OP50 and C. sakazakii (CS) and scored for survival over time. The representative graphs shown are (A) N2 + CS versus BQ1 (akt-1) + CS, (B) N2 + CS versus VC2312 (let-363) + CS, and (C) N2 + CS versus CZ5730 (dlk-1) + CS. Each graph is a combination of three biological triplicate experiments. The P-values represent the significance level of mutant survival relative to N2, during C. sakazakii infection, *p < 0.05, **p < 0.01, and ***p < 0.001. | |
3.4
C. sakazakii infection upregulates innate-immune response genes through candidate immune regulatory players
We then intended to identify the expression changes of the KEGG pathway-identified genes (acox-1.4, ddl-2, mlck-1, and let-363) at the mRNA level using qPCR analysis (Fig. 5). The mRNA expression level showed upregulation of all the targeted genes by 0.5 to 6.5 times the fold differences during 12 to 72 h of C. sakazakii infection. Compared to that of the control, the expression of genes was found slightly upregulated in the early hours of infection (12 h). After 24 h of infection, the expressions of ddl-2 upregulated drastically, a 1.99-fold difference than 24 and 48 h. At 48 h, the expressions of acox-1.4, mlck-1, and let-363 were upregulated. During the late infection stage (72 h), the expressions of acox-1.4, mlck-1 and let-363 were upregulated by 3.50, 4.68, 3.83, respectively, followed by ddl-2 upregulated by 1.99 fold. Overall, we observed that acox-1.4 and ddl-2 regulated contrariwise and mlck-1 and let-363 regulated reciprocally. For example, acox-1.4 2 was found to have a significant increase in fold change during 12 and 24 h, whereas the fold change of ddl-2 was downregulated in 24 and 48 h and upregulated in 72 h though no significant differences were observed. However, mlck-1 and let-363 were upregulated in parallel during all the analyzed time duration. As a result, it is found that transcriptional regulation of most of the validated genes (acox-1.4, ddl-2, mlck-1, and let-363) were not similarly expressed to the protein level expression identified using LC-MS/MS analysis (Fig. 5, Tables 2 and 3). Thus, it is inferred that functional dissimilarity between genes and their proteins against infection-triggered host defense may have undergone alternative splicing at the translational level.
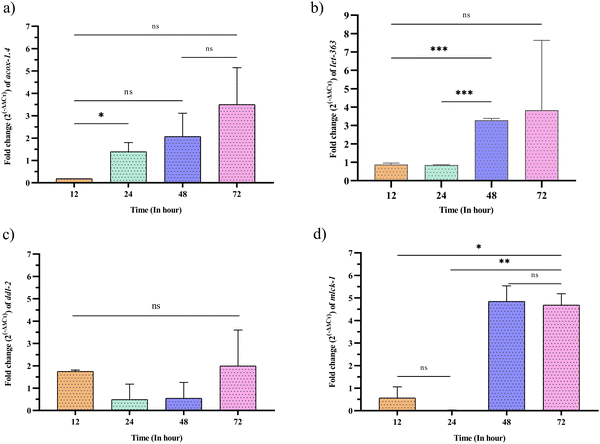 |
| Fig. 5 Real-Time qPCR analysis of DEPs gene expression in C. sakazakii infected C. elegans. The relative expression level of the KEGG pathway has identified protein-coding genes involved in the mTOR signaling pathway; axon regeneration, metabolic pathways -acox-1.4 (A), let-363 (B), longevity regulating pathway -ddl-2 (C), and calcium signaling pathway -mlck-1 (D), in worms infected with C. sakazakii at various time periods (12, 24, 48, and 72 h). The bars represent mean ± s.d.; three independent biological triplicate experiments. Here, ≥1 is considered as upregulated and ≤1 is considered as downregulated. The level of significance was analyzed by one-way ANOVA followed by Duncan's post-hoc analysis (*p < 0.05, **p < 0.01, and ***p < 0.001; ns – no significance). | |
3.5
C. sakazakii infection induces necrotic cell death and modulation of SGK-1 expression
To circumvent the inflammatory response of necrotic cell death during C. sakazakii infection, we exposed N2 worms to C. sakazakii for 48 and 72 h. Later, the worms were stained them with propidium iodide, a red fluorescent dye that enters into several tissues through plasma membrane pores and stains the host cells. The microscopic analysis of C. elegans exposed to C. sakazakii for 72 h showed higher uptake of propidium iodide than 48 h infection, which indicated the higher number of dying cells during the course of infection (Fig. 6A and B). Therefore, the examination of cell death is evidence to the killing assay, where the C. sakazakii infection is able to compromise the immunity and make a first kill at 24 h of exposure.
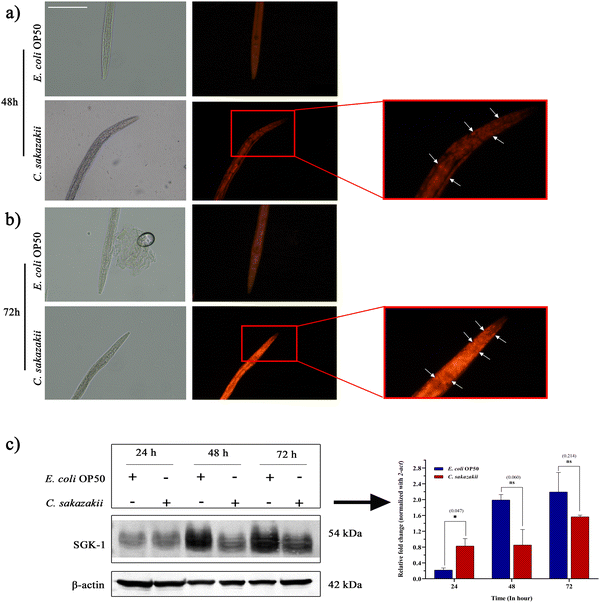 |
| Fig. 6 Loss of cell membrane integrity during C. sakazakii infection triggers an imbalance of proteostasis in C. elegans. The representative fluorescence microscopic images show the worms stained with propidium iodide (PI) in E. coli OP50 and C. sakazakii. The C. sakazakii exposed worms showing membrane integrity (red fluorescence marked with arrow) upon infection at 72 h compared to 48 h (10× magnification). The bar denotes 50 μm. (C) Western blot analysis of C. sakazakii infection on the expression levels of SGK-1. β-actin protein was used as the internal control. The histograms representing the fold change in the regulation of total SGK-1 in C. elegans during C. sakazakii infection. Bars represent mean ± s.d. | |
Further, the relative protein expression of mTORC2-activated SGK-1 kinase was measured by immunoblotting analysis with β-actin as the internal control (Fig. 6C). Though SGK-1 was not directly identified in the MS analysis but in the PI3K/Akt/mTOR signaling pathway, it is well known for its regulation for cell survival, and the disturbance of their proteostasis would enhance autophagic or necrotic machinery.44 Hence, we tried to uncover the regulation of potential immune regulatory protein kinases of mTORC2/SGK-1 during the C. sakazakii infection. The blotting analysis showed a significant difference in the upregulation of C. sakazakii—infected SGK-1 protein expression level at 24 h, while during 48 and 72 h of infection, the SGK-1 protein expression level revealed negligible downregulation when compared to E. coli OP50. The image visualization software (Bio-Rad) analysis also confirmed protein level expression variation in the graph revealing the quantitative differentiation of SGK-1 regulation (Fig. 6C). The variations in the protein expression level may be due to the pathogenic effect of C. sakazakii infection that might have triggered the host immune player SGK-1 via the mTOR pathway during initial 24 h of infection to regulate the autophagy properly.
4 Discussion
Proteomics strategies have been used to investigate the protein regulations and their associated pathways that cause neuro signaling modulation and immune homeostasis during inflammatory reactions during HPI. Several reports have shown that in an HPI, both Gram-positive (Staphylococcus aureus and Bacillus thuringiensis) and Gram-negative pathogens (Vibrio alginolyticus and Pseudomonas aeruginosa) regulate transcriptional (gene) and translational (protein) changes in the immune system.30,37,45,46 Further, these studies have reported that the pathogenic mechanisms affect the host by inhibiting the immune response, causing prolonged damage to the nervous system, and increasing the risk of neurodegenerative diseases.
Our research group has already reported that C. elegans is a model organism for understanding the infections of C. sakazakii, in which, the response of C. elegans against 24 h of C. sakazakii infection at the physiological level was analyzed for the first time.24 Later, Muthubharathi et al. reported that the metabolites of C. elegans respond negatively to C. sakazakii infection.25 Further studies also proved that C. sakazakii infection altered the genes of C. elegans involved in pathogen recognition and kinase activity.26 Despite this limited number of studies, no reports are available regarding the neuro-immune protein change in C. elegans during C. sakazakii infection to date. Hence, in this study, we sought to elucidate the changes in host neuro-immune translation (protein) caused by C. sakazakii infection using a label-free quantitative proteomics approach (LC-MS/MS). The C. sakazakii strain (ATCC 29544) used in this study was also found to regulate host proteomes within 24 and 48 h of infection. This study was initiated with the objective of identifying major differentially regulated and neuro-immunity-related C. elegans proteins in response to C. sakazakii infection. In this regard, LC-MS/MS-based proteome analysis of C. sakazakii—infected (24 and 48 h) and E. coli—fed C. elegans was performed. The analysis revealed a higher total number of proteins in both 24 and 48 h infected samples. STRING analysis of the whole proteins from both 24 and 48 h samples provided representative networks of both well-known and predicted protein interactions with physical and functional associations. Further, the Gene Ontology (GO) annotation of the distribution of differentially regulated proteins suggested that the major group of proteins belonged to different cellular components, molecular functions, and biological processes. Since the study focused on neuronal signaling-associated immune pathways, only the proteins associated with those pathways were specifically pruned. The proteins were LET-363 (mTOR signaling, axon regeneration, autophagy), MLCK-1 (calcium signaling), DDL-2 (endocytosis, longevity regulating pathway), and ACOX- 1.4 (peroxisome, metabolic pathways such as fatty acid metabolism, carbon metabolism, and biosynthesis of unsaturated fatty acids). Hence, with respect to the results of LCMS/MS analysis, this study considered four major pathways, namely, mTOR signaling, axon regeneration, calcium signaling, and longevity regulating pathway.
Many HPI studies have reported that significantly regulated proteins have perturbed immune responses and disturb the neuronal signaling mechanisms. One study reported that NPR-1, a G-protein coupled receptor (GPCR) expressed in neurons, has deleterious consequences to immune responses in non-neural tissues by the p38 MAPK pathway and OCTR-1 inhibits the ribosomal protein RPS-1 and the translation initiation factor EIF-3 during infection with P. aeruginosa.19,47 Similarly, LET-363, an axonal mammalian target of rapamycin (mTOR) involved in the translation regulation of the proteostasis of neuronal signaling cascades and the long-lasting synaptic plasticity against a wide-range infections such as Leishmania major and herpes simplex virus-1 (HSV1), induces neurological diseases that infiltrate mTORC1, which is downstream to PI3K-Akt receptors and affects the cell growth via upstream to SGK-1 phosphorylation (mTORC2).48,49 Moreover, it has a major role in maintaining the anabolism and catabolism of a cell50 and itself is a kinase protein that acts as a mediator for a diverse number of cellular metabolism and immune activation.51 The detailed analysis of GO revealed that host inflammatory responses such as rRNA binding and catalytic activity, or primary metabolic processes involving autophagy promoting LET-363 in the cell biosynthesis process, improve cell protein metabolism, translation, and lifespan determination in adult worms. Due to the immune stress, mTOR activity decreases, resulting in reduced protein synthesis and increased autophagic cell death. Previous reports have shown that the uptake of a pathogen by C. elegans has downregulated the expression of mTOR due to deprived nutrient signals.52,53 Unlike other pathogens, wild-type C. elegans were attracted to C. sakazakii and their virulence factors disrupt serotonin regulations and induce abnormal egg production,25 and it is evidenced that the absence of the neuronal signaling associated proteins AKT-1 and LET-363 has adversely regulated the lifespan of mutants during C. sakazakii infection, also reducing the development rate of animals, and therefore, it reduced the egg production in the mutants (BQ1 and VC2312). However, mTOR was found to be upregulated at protein level (by 3.25 fold at 24 h) and significantly upregulated at mRNA level expression (by 3.28 fold at 48 h and 3.83 fold at 72 h), also downregulated during 12 (0.88 fold) and 24 h (0.84 fold) conditions. Interestingly, the mutants BQ1 (akt-1) and VC2312 (let-363) were dramatically suppressed, with shorter lifespan (8 days for BQ1 and 6 days for VC2312) than wild-type worms (13 days) during the C. sakazakii infection. In the mTOR downregulation scenario, the slower uptake of pathogens as a food source by the mutants was due to the virulence factors that made the mutant worms to starve for nutrients in the later hours of exposure. This observation was supported by the reduction in the pharyngeal pumping movement of mutants BQ1 and VC2312. Hence, the upregulation in mTOR might be caused due to the higher uptake of pathogens as a food source, implicating that other mechanisms of host defense might exist.
Axon degeneration caused by chronic infection via mTOR protein regulation is considered to be an important pathogenesis of neurodegenerative diseases. In addition, the parallel regulation of conserved DLK-1 is plausible, which acts downstream of DAF-2 (IIS pathway),54 and the p38MAP kinase pathway plays a key signaling modulation role leading to the collapse of proteostasis and the progression of axonal degeneration.55,56 We performed the lifespan assay using the CZ5730 mutant (suppressor of rpm-1- a regulator of synaptic vesicles), which provides experimental evidence that the dysregulation of the host's proteostasis occurs through axotomy and may have downregulated the immune response,57 and thus, the infection reduced the lifespan of mutant worms.
An infection can trigger several professional immune players in the host, which employs their cells to function autonomously in order to normalize their neuronal synaptogenesis. Considering the given fact that proteins are major mediators and regulators of immune responses, our present findings also emphasize the importance of mRNA level expression as a surrogate for proteins. Therefore, we further investigated the gene expression of mlck-1 by real-time qPCR analysis. Myosin light-chain kinase (MLCK) regulates smooth muscles by inducing the actin–myosin interaction, where it binds to Ca2+via phosphorylation of light chains and contraction.58 The impaired Ca2+-encoded signals involved in intestinal inflammation through mlck facilitate the internal colonization by opportunistic pathogens.59,60 Therefore, the activation of mlck is crucial to regulate the tight junction (TJ) of both epithelial and endothelial cells. In addition, this TJ complex provides a hub for the Ca2+ signaling pathway, and thus, it protects the intestinal cells from invading pathogenic factors.61 The higher expression (10.2 fold changes at 48 h) of mlck-1 at protein level is functionally related to its gene expression, where the fold expression at 48 h revealed to be significantly higher than that at 12 and 24 h. The functional similarity merely suggests that C. sakazakii infection at initial exposure time might have impaired the intestinal barrier function via dysfunction of the mlck-1 signaling pathway.
It is a well-known fact that an increase in mTOR decreases autophagy.62 Autophagy is considered one of the important pathways to eliminate cellular waste and toxins.63,64 The inhibition or absence of autophagy prevents the cells from removing toxins, thereby accumulating unwanted proteins and other compounds, which leads to necrotic cell death due to the infection.65 However, the mechanism by which autophagy regulates innate immunity via mTORC2 to SGK-1 remains largely unknown. The cell death during the infection time was validated in our study using propidium iodide staining that confirmed the increase in necrosis-induced organismal cell death triggered due to C. sakazakii infection at 72 h in wild-type worms. The impairment of autophagy might have induced intestinal necrosis as shown in the propidium iodide—stained worm images.65 During the course of longer expression time at 48 and 72 h, the infection also leads to a reduction in protein levels of SGK-1, which could not be able to fight against the pathogenesis and possibly might have phosphorylated which resulted in the impairment of autophagy or necrosis, and proteostasis.66 Additionally, a spatiotemporal examination of autophagic activity in sgk-1 mutants may provide greater mechanistic understanding of how autophagy contributes to C. sakazakii infection-induced proteostasis.
C. sakazakii infection can penetrate the blood–brain barrier (BBB) by translocating proteins associated with the endocytic pathway to human brain microvascular endothelial cells (HBMEC).67 One of the HSF-1 regulators, DDL-2 (the C. elegans homolog of the human Wiskott-Aldrich syndrome protein and SCAR homolog: (WASH2) protein), (daf-16-dependent longevity) is a key player in the longevity regulating pathway.68 HSF-1 is a molecular chaperone that forms a protein complex with HSB-1 (heat-shock factor binding protein-1) and DDL-2, and this complex reduces the lifespan of C. elegans.69,70 This induces the HSPs chaperones from HSF-1 which reduces the proteotoxicity.71 Thereby, the protein expression of ddl-2 from LC-MS/MS data revealed the downregulation at 0.439 fold difference during 48 h condition and their KEGG pathway identification potentially inflicts damage in host proteostasis by involving several signaling pathways including the endocytic pathway in neuronal dysfunction (KEGG ID: cel04144) and longevity regulating pathways (KEGG ID: cel04212) (Table S6D, ESI†). The mRNA level expression of this translated protein is eventually studied and the observation revealed that C. sakazakii infection increased the ddl-2 expression to 1.99-fold in 72 h albeit no significant regulations were observed when compared to 12 (1.75 fold), 24 (0.49 fold) and 48 h (0.55 fold). The gene expression level results suggest that C. elegans senses C. sakazakii virulence factors, which are imported via endocytosis. The significant reduction in lifespan in wild-type and mutant strains during C. sakazakii infection in our study may be attributed to this phenomenon in parallel regulation of longevity pathway (ddl-2).
Other major finding during C. sakazakii infection is the increase in lipid droplets in the pharynx, intestinal region, and tail.25 Lipid droplets that generally store excess fats (triglycerides and cholesterol esters) are used by C. elegans during nutritional demand.72 However, excess lipid droplets also accumulate fat and cause metabolic dysregulation. Their subunits are reported to participate in axon regeneration by producing a dauer-inducing ascaroside.73acox-1.4 is a major gene involved in lipid degradation. In line with the findings of Muthubharathi et al., acox-1.4 was found to be significantly upregulated during 12–24 h of infection. Meanwhile, the protein expression revealed the upregulated fold change from 24 to 48 h condition. The host system might have enhanced acox-1.4 to deplete the overproduction of lipid droplets upon infection. After 48 h of infection, the collapse of the system due to infection might have reduced acox-1.4 expression as evidenced in the gene expression at 72 h. Our findings are consistent with recent studies showing that some pathogens, including Stenotrophomonas maltophilia require C. elegans metabolites for survival through their pathogenesis and increase their virulence by suppressing acox-1.74 However, these reports were available till the L3 stage, and the exact mechanism of acox subunits in the L4 stage is yet to be known.
5 Conclusion
The label-free quantitative technique (LC-MS/MS) for studying the pathophysiological features of host-specific proteins regulating potential neuro-immune signaling pathways of C. elegans is foreseeable. Based upon our results, we hypothesized that the identified candidate players have revealed the relationship between the neuronal signaling, metabolic pathways and inflammatory immune responses. Notably, for the first time, mTOR are targeted to unravel the relation between neuronal signaling and immune pathway, which provides an augmented understanding of C. sakazakii infection to host. Later, as C. sakazakii utilizes the host proteins and metabolites, the upregulated proteins of C. elegans act as a negative feedback loop by inducing other phenomena such as necrotic cell death and lipid droplet aggregations (Fig. 7). Future research will be needed to determine the functional connectomes of identified protein's role in neuro-immune communication during the C. sakazakii pathogenesis.
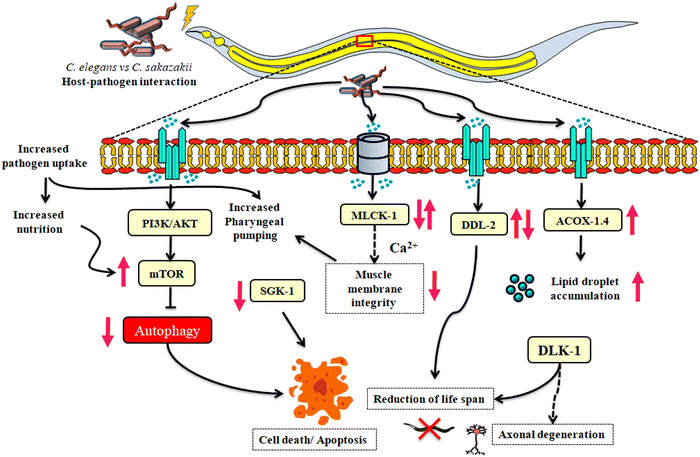 |
| Fig. 7 Multifaceted neuro-immune regulatory signaling pathways of C. elegans against C. sakazakii infection. The infection of C. elegans with C. sakazakii was found to upregulate four key neuro-immune signaling pathways. In response to C. sakazakii being taken up more readily by C. elegans, the nutrients were increased, resulting in an increase in the expression of mTOR. The parallel upregulation of MLCK-1 (a regulator of muscle contraction movements from the calcium signaling pathway) might have supported the pathogen uptake by increasing the pharyngeal pumping at earlier exposure. The upregulation of mTOR and subsequent downregulation of SGK-1 would have caused autophagy inhibition, thereby inducing necrotic cell death and proteostasis. The differential regulation of DDL-2 from the longevity-regulating pathway directly indicates the reduction of lifespan in C. elegans. In addition, the accumulation of lipid droplets during infection may have contributed to the upregulation of ACOX-1.4, resulting in axonal damage later supported with lifespan depletion of dlk-1 mutants. | |
Author contributions
Conceptualization: LMV and KB; data curation: LMV; formal analysis: LMV, BB and TJS; methodology: LMV; resources: VR and KB; software: LMV; supervision: KB; visualization: LMV; writing – original draft: LMV and TJS; writing – review & editing: LMV and KB.
Data availability
The mass spectrometry proteomics data have been deposited to the ProteomeXchange Consortium via the PRIDE partner repository with the data set identifier, PXD041315.
Conflicts of interest
There are no conflicts to declare.
Acknowledgements
The authors thankfully acknowledge the Caenorhabditis Genetics Centre, University of Minnesota (funded by National Institutes of Health) for providing us with the C. elegans wild-type (N2), BQ1, VC2312, and CZ5730 strains. LMV and KB acknowledges with appreciation to the Department of Biotechnology, India (DBT, India) (Ref. No. BT/PR17367/MED/122/44/2016 dated 09.06.2017), ITC-AU Collaborative Projects, RUSA 2.0 [grant no. F. 24-51/2014-U, Policy (TN Multi-Gen) and DST-SERB (CRG/2021/000914) for the financial support. LMV acknowledges DBT, India (Ref. No. BT/PR17367/MED/122/44/2016 dated 09.06.2017) for the financial support in the form of Junior Research Fellowship (JRF). KB and LMV acknowledge the NIPER, Kolkata for providing access to the LC-MS/MS facility available at the Institute. Technical assistance in LC-MS/MS analysis rendered by Gajbhiye Rahul Laxman is greatly appreciated. Special thanks to Dr Shanmugam Marudhupandiyan, PhD, for technical assistance in western blotting analysis.
References
- C. Treitz, L. Cassidy, A. Höckendorf, M. Leippe and A. Tholey, Quantitative proteome analysis of Caenorhabditis elegans upon exposure to nematicidal Bacillus thuringiensis, J. Proteomics, 2015, 113, 337–350, DOI:10.1016/j.jprot.2014.09.027.
- J. E. Irazoqui, J. M. Urbach and F. M. Ausubel, Evolution of host innate defence: insights from Caenorhabditis elegans and primitive invertebrates, Nat. Rev. Immunol., 2010, 10(1), 47–58, DOI:10.1038/nri2689.
- L. Zhang, Y. Wang, C. Cao, Y. Zhu, W. Huang, Y. Yang, H. Qiu, S. Liu and D. Wang, Beneficial effect of Xuebijing against Pseudomonas aeruginosa infection in Caenorhabditis elegans, Front. Pharmacol., 2022, 13, 949608, DOI:10.3389/fphar.2022.949608.
- E. K. Marsh and R. C. May, Caenorhabditis elegans, a model organism for investigating immunity, Appl. Environ. Microbiol., 2012, 78(7), 2075–2081, DOI:10.1128/AEM.07486-11.
- W. Kim, R. S. Underwood, I. Greenwald and D. D. Shaye, OrthoList 2: a new comparative genomic analysis of human and Caenorhabditis elegans genes, Genetics, 2018, 210(2), 445–461, DOI:10.1534/genetics.118.301307.
- World Health Organization, Enterobacter Sakazakii (Cronobacter Spp.) in Powdered Follow-up Formula: Meeting Report, World Health Organization, 2008.
- O. Holý and S. Forsythe, Cronobacter spp. as emerging causes of healthcare-associated infection, J. Hosp. Infect., 2014, 86(3), 169–177, DOI:10.1016/j.jhin.2013.09.011.
-
S. J. Forsythe, New insights into the emergent bacterial pathogen Cronobacter, Food Safety, Academic Press, 2015, pp. 265–308 Search PubMed.
- P. Ogrodzki and S. J. Forsythe, DNA-sequence based typing of the Cronobacter genus using MLST, CRISPR-cas array and capsular profiling, Front. Microbiol., 2017, 1875, DOI:10.3389/fmicb.2017.01875.
- J. P. Mange, R. Stephan, N. Borel, P. Wild, K. S. Kim, A. Pospischil and A. Lehner, Adhesive properties of Enterobacter sakazakii to human epithelial and brain microvascular endothelial cells, BMC Microbiol., 2006, 6(1), 1, DOI:10.1186/1471-2180-6-58.
- M. K. Mohan Nair, K. Venkitanarayanan, L. K. Silbart and K. S. Kim, Outer membrane protein A (OmpA) of Cronobacter sakazakii binds fibronectin and contributes to invasion of human brain microvascular endothelial cells, Foodborne Pathog. Dis., 2009, 6(4), 495–501, DOI:10.1089/fpd.2008.0228.
- S. Kim, H. Hwang, K. P. Kim, H. Yoon, D. H. Kang and S. Ryu, Hfq plays important roles in virulence and stress adaptation in Cronobacter sakazakii ATCC 29544, Infect. Immun., 2015, 83(5), 2089–2098, DOI:10.1128/iai.03161-14.
- F. A. Azevedo, L. R. Carvalho, L. T. Grinberg, J. M. Farfel, R. E. Ferretti, R. E. Leite, W. J. Filho, R. Lent and S. Herculano-Houzel, Equal numbers of neuronal and nonneuronal cells make the human brain an isometrically scaled-up primate brain, J. Comp. Neurol., 2009, 513(5), 532–541, DOI:10.1002/cne.21974.
- X. Zhang and Y. Zhang, Neural-immune communication in Caenorhabditis elegans, Cell Host Microbe, 2009, 5(5), 425–429, DOI:10.1016/j.chom.2009.05.003.
- D. H. Kim, R. Feinbaum, G. Alloing, F. E. Emerson, D. A. Garsin, H. Inoue, M. Tanaka-Hino, N. Hisamoto, K. Matsumoto, M. W. Tan and F. M. Ausubel, A conserved p38 MAP kinase pathway in Caenorhabditis elegans innate immunity, Science, 2002, 297(5581), 623–626, DOI:10.1126/science.1073759.
- M. J. Gravato-Nobre and J. Hodgkin, Caenorhabditis elegans as a model for innate immunity to pathogens, Cell. Microbiol., 2005, 7(6), 741–751, DOI:10.1111/j.1462-5822.2005.00523.x.
- J. J. Ewbank, Signaling in the immune response, WormBook, 2006, 1–2, DOI:10.1895/wormbook.1.83.1.
- L. Frooninckx, L. Van Rompay, L. Temmerman, E. Van Sinay, I. Beets, T. Janssen, S. J. Husson and L. Schoofs, Neuropeptide GPCRs in C. elegans, Front. Endocrinol., 2012, 3, 167, DOI:10.3389/fendo.2012.00167.
- K. L. Styer, V. Singh, E. Macosko, S. E. Steele, C. I. Bargmann and A. Aballay, Innate immunity in Caenorhabditis elegans is regulated by neurons expressing NPR-1/GPCR, Science, 2008, 322(5900), 460–464, DOI:10.1126/science.1163673.
- K. C. Reddy, E. C. Andersen, L. Kruglyak and D. H. Kim, A polymorphism in npr-1 is a behavioral determinant of pathogen susceptibility in C. elegans, Science, 2009, 323(5912), 382–384, DOI:10.1126/science.1166527.
- A. Anderson, H. Laurenson-Schafer, F. A. Partridge, J. Hodgkin and R. McMullan, Serotonergic chemosensory neurons modify the C. elegans immune response by regulating G-protein signaling in epithelial cells, PLoS Pathog., 2013, 9(12), e1003787, DOI:10.1371/journal.ppat.1003787.
- X. Cao and A. Aballay, Neural inhibition of dopaminergic signaling enhances immunity in a cell-non-autonomous manner, Curr. Biol., 2016, 26(17), 2329–2334, DOI:10.1016/j.cub.2016.06.036.
- B. S. Sivamaruthi, M. I. Prasanth and K. Balamurugan, Alterations in Caenorhabditis elegans and Cronobacter sakazakii lipopolysaccharide during interaction, Arch. Microbiol., 2015, 197, 327–337, DOI:10.1007/s00203-014-1064-1.
- B. S. Sivamaruthi, A. Ganguli, M. Kumar, S. Bhaviya, S. K. Pandian and K. Balamurugan, Caenorhabditis elegans as a model for studying Cronobacter sakazakii ATCC BAA-894 pathogenesis, J. Basic Microbiol., 2011, 51(5), 540–549, DOI:10.1002/jobm.201000377.
- B. C. Muthubharathi, B. Balasubramaniam, D. A. Mir, V. Ravichandiran and K. Balamurugan, Physiological and Metabolite Alterations Associated with Neuronal Signals of Caenorhabditis elegans during Cronobacter sakazakii Infections, ACS Chem. Neurosci., 2021, 12(22), 4336–4349, DOI:10.1021/acschemneuro.1c00559.
- B. C. Muthubharathi, V. Ravichandiran and K. Balamurugan, Cronobacter sakazakii Cue for the Attraction and Its Impact on the Immunity of Caenorhabditis elegans, Infect. Immun., 2022, 90(12), e00281, DOI:10.1128/iai.00281-22.
- T. Kawli, F. He and M. W. Tan, It takes nerves to fight infections: insights on neuro-immune interactions from C. elegans, Dis. Models Mech., 2010, 3(11–12), 721–731, DOI:10.1242/dmm.003871.
- P. Wibisono and J. Sun, Neuro-immune communication in C. elegans defense against pathogen infection, Curr. Res. Immunol., 2021, 2, 60–65, DOI:10.1016/j.crimmu.2021.04.002.
- S. Brenner, The genetics of Caenorhabditis elegans, Genetics, 1974, 77(1), 71–94, DOI:10.1093/genetics/77.1.71.
- V. Balasubramanian, D. Sellegounder, K. Suman and B. Krishnaswamy, Proteome analysis reveals translational inhibition of Caenorhabditis elegans enhances susceptibility to Pseudomonas aeruginosa PAO1 pathogenesis, J. Proteomics, 2016, 145, 141–152, DOI:10.1016/j.jprot.2016.04.025.
- M. M. Bradford, A rapid and sensitive method for the quantitation of microgram quantities of protein utilizing the principle of protein-dye binding, Anal. Biochem., 1976, 72(1–2), 248–254, DOI:10.1006/abio.1976.9999.
- D. A. Mir and K. Balamurugan, Global proteomic response of Caenorhabditis elegans against PemKSa toxin. Frontiers in Cellular and Infection, Microbiology, 2019, 9, 172, DOI:10.3389/fcimb.2019.00172.
- B. Balasubramaniam, T. Vinitha, S. Meenal, L. M. VenkataKrishna, V. Ravichandiran and K. Balamurugan, p38-MAPK recruits the proteolytic pathways in Caenorhabditis elegans during bacterial infection, Int. J. Biol. Macromol., 2022, 204, 116–135, DOI:10.1016/j.ijbiomac.2022.01.191.
- E. W. Deutsch, N. Bandeira, V. Sharma, Y. Perez-Riverol, J. J. Carver, D. J. Kundu, D. García-Seisdedos, A. F. Jarnuczak, S. Hewapathirana, B. S. Pullman and J. Wertz, The ProteomeXchange consortium in 2020: enabling ‘big data’ approaches in proteomics, Nucleic Acids Res., 2020, 48(D1), D1145–D1152, DOI:10.1093/nar/gkz984.
- A. Franceschini, D. Szklarczyk, S. Frankild, M. Kuhn, M. Simonovic, A. Roth, J. Lin, P. Minguez, P. Bork, C. Von Mering and L. J. Jensen, STRING v9. 1: protein-protein interaction networks, with increased coverage and integration, Nucleic Acids Res., 2012, 41(D1), D808–D815, DOI:10.1093/nar/gks1094.
- S. Marudhupandiyan, U. Prithika, B. Balasubramaniam and K. Balamurugan, RACK-1, a multifaceted regulator is required for C. elegans innate immunity against S. flexneri M9OT infection, Dev. Comp. Immunol., 2017, 74, 227–236, DOI:10.1016/j.dci.2017.05.008.
- B. Wang, H. Wang, J. Xiong, Q. Zhou, H. Wu, L. Xia, L. Li and Z. Yu, A proteomic analysis provides novel insights into the stress responses of Caenorhabditis elegans towards nematicidal Cry6A Toxin from Bacillus thuringiensis, Sci. Rep., 2017, 7(1), 14170, DOI:10.1038/s41598-017-14428-3.
- E. L. Greer, D. Dowlatshahi, M. R. Banko, J. Villen, K. Hoang, D. Blanchard, S. P. Gygi and A. Brunet, An AMPK-FOXO pathway mediates longevity induced by a novel method of dietary restriction in C. elegans, Curr. Biol., 2007, 17(19), 1646–1656, DOI:10.1016/j.cub.2007.08.047.
- N. Kourtis, V. Nikoletopoulou and N. Tavernarakis, Small heat-shock proteins protect from heat-stroke-associated neurodegeneration, Nature, 2012, 490(7419), 213–218, DOI:10.1038/nature11417.
- D. Grün, M. Kirchner, N. Thierfelder, M. Stoeckius, M. Selbach and N. Rajewsky, Conservation of mRNA and protein expression during development of C. elegans, Cell Rep., 2014, 6(3), 565–577, DOI:10.1016/j.celrep.2014.01.001.
- D. A. Mir, B. Balasubramaniam, L. M. VenkataKrishna, B. C. Muthubharathi and K. Balamurugan, Proteomic analysis of Caenorhabditis elegans against Salmonella Typhi toxic proteins. Genes &, Immunity, 2021, 22(2), 75–92, DOI:10.1038/s41435-021-00132-w.
- M. I. Prasanth, G. S. Santoshram, J. P. Bhaskar and K. Balamurugan, Ultraviolet-A triggers photoaging in model nematode Caenorhabditis elegans in a DAF-16 dependent pathway, Age, 2016, 38, 1–3, DOI:10.1007/s11357-016-9889-y.
- R. A. Saxton and D. M. Sabatini, mTOR signaling in growth, metabolism, and disease, Cell, 2017, 168(6), 960–976, DOI:10.1016/j.cell.2017.02.004.
- U. Prithika, V. Deepa and K. Balamurugan, External induction of heat shock stimulates the immune response and longevity of Caenorhabditis elegans towards pathogen exposure, Innate Immun., 2016, 22(6), 466–478, DOI:10.1177/1753425916654557.
- K. T. Simonsen, J. Møller-Jensen, A. R. Kristensen, J. S. Andersen, D. L. Riddle and B. H. Kallipolitis, Quantitative proteomics identifies ferritin in the innate immune response of C. elegans, Virulence, 2011, 2(2), 120–130, DOI:10.4161/viru.2.2.15270.
- S. Durai, N. Singh, S. Kundu and K. Balamurugan, Proteomic investigation of Vibrio alginolyticus challenged Caenorhabditis elegans revealed regulation of cellular homeostasis proteins and their role in supporting innate immune system, Proteomics, 2014, 14(15), 1820–1832, DOI:10.1002/pmic.201300374.
- Y. Liu, D. Sellegounder and J. Sun, Neuronal GPCR OCTR-1 regulates innate immunity by controlling protein synthesis in Caenorhabditis elegans, Sci. Rep., 2016, 6(1), 36832, DOI:10.1038/srep36832.
- N. Hay and N. Sonenberg, Upstream and downstream of mTOR, Genes Dev., 2004, 18(16), 1926–1945, DOI:10.1101/gad.1212704.
- M. Kobayashi, A. C. Wilson, M. V. Chao and I. Mohr, Control of viral latency in neurons by axonal mTOR signaling and the 4E-BP translation repressor, Genes Dev., 2012, 26(14), 1527–1532, DOI:10.1101/gad.190157.112.
- A. S. Dossou and A. Basu, The emerging roles of mTORC1 in macromanaging autophagy, Cancers, 2019, 11(10), 1422, DOI:10.3390/cancers11101422.
- R. C. Russell, C. Fang and K. L. Guan, An emerging role for TOR signaling in mammalian tissue and stem cell physiology, Development, 2011, 138(16), 3343–3356, DOI:10.1242/dev.058230.
- A. Kamaladevi and K. Balamurugan, Global proteomics revealed Klebsiella pneumoniae induced autophagy and oxidative stress in Caenorhabditis elegans by inhibiting PI3K/AKT/mTOR pathway during infection. Frontiers in Cellular and Infection, Microbiology, 2017, 7, 393, DOI:10.3389/fcimb.2017.00393.
- L. Cabral-Fernandes, S. Goyal, A. Farahvash, J. Tsalikis, D. J. Philpott and S. E. Girardin, Invading Bacterial Pathogens Activate Transcription Factor EB in Epithelial Cells through the Amino Acid Starvation Pathway of mTORC1 Inhibition, Mol. Cell. Biol., 2022, 42(9), e00241, DOI:10.1128/mcb.00241-22.
- S. Belin, M. Norsworthy and Z. He, Independent control of aging and axon regeneration, Cell Metab., 2014, 19(3), 354–356, DOI:10.1016/j.cmet.2014.02.014.
- D. Yan, Z. Wu, A. D. Chisholm and Y. Jin, The DLK-1 kinase promotes mRNA stability and local translation in C. elegans synapses and axon regeneration, Cell, 2009, 138(5), 1005–1018, DOI:10.1016/j.cell.2009.06.023.
- M. Hammarlund, P. Nix, L. Hauth, E. M. Jorgensen and M. Bastiani, Axon regeneration requires a conserved MAP kinase pathway, Science, 2009, 323(5915), 802–806, DOI:10.1126/science.1165527.
- K. Nakata, B. Abrams, B. Grill, A. Goncharov, X. Huang, A. D. Chisholm and Y. Jin, Regulation of a DLK-1 and p38 MAP kinase pathway by the ubiquitin ligase RPM-1 is required for presynaptic development, Cell, 2005, 120(3), 407–420, DOI:10.1016/j.cell.2004.12.017.
- K. A. Steger and L. Avery, The GAR-3 muscarinic receptor cooperates with calcium signals to regulate muscle contraction in the Caenorhabditis elegans pharynx, Genetics, 2004, 167(2), 633–643, DOI:10.1534/genetics.103.020230.
- H. Wang, N. Zhai, Y. Chen, C. Fu and K. Huang, OTA induces intestinal epithelial barrier dysfunction and tight junction disruption in IPEC-J2 cells through ROS/Ca2+-mediated MLCK activation, Environ. Pollut., 2018, 242, 106–112, DOI:10.1016/j.envpol.2018.06.062.
- J. Gao, S. Cao, H. Xiao, S. Hu, K. Yao, K. Huang, Z. Jiang and L. Wang, Lactobacillus reuteri 1 Enhances Intestinal Epithelial Barrier Function and Alleviates the Inflammatory Response Induced by Enterotoxigenic Escherichia coli K88 via Suppressing the MLCK Signaling Pathway in IPEC-J2 Cells, Front. Immunol., 2022, 13, 897395, DOI:10.3389/fimmu.2022.897395.
- A. A. Bhat, S. Uppada, I. W. Achkar, S. Hashem, S. K. Yadav, M. Shanmugakonar, H. A. Al-Naemi, M. Haris and S. Uddin, Tight junction proteins and signaling pathways in cancer and inflammation: a functional crosstalk, Front. Physiol., 2019, 9, 1942, DOI:10.3389/fphys.2018.01942.
- S. Chakrabarti, P. Liehl, N. Buchon and B. Lemaitre, Infection-induced host translational blockage inhibits immune responses and epithelial renewal in the Drosophila gut, Cell Host Microbe, 2012, 12(1), 60–70, DOI:10.1016/j.chom.2012.06.001.
- E. S. Hars, H. Qi, S. V. Jin, L. Cai, C. Hu and L. F. Liu, Autophagy regulates ageing in C. elegans, Autophagy, 2007, 3(2), 93–95, DOI:10.4161/auto.3636.
- A. Meléndez and B. Levine, Autophagy in C. elegans, WormBook, 2009, 1–26, DOI:10.1895/wormbook.1.147.1.
- C. G. Zou, Y. C. Ma, L. L. Dai and K. Q. Zhang, Autophagy protects C. elegans against necrosis during Pseudomonas aeruginosa infection, Proc. Natl. Acad. Sci. U. S. A., 2014, 111(34), 12480–12485, DOI:10.1073/pnas.1405032111.
- P. de la Cruz-Ruiz, B. Hernando-Rodríguez, M. M. Pérez-Jiménez, M. J. Rodríguez-Palero, M. D. Martínez-Bueno, A. Pla, R. Gatsi and M. Artal-Sanz, Prohibitin depletion extends lifespan of a TORC2/SGK-1 mutant through autophagy and the mitochondrial UPR, Aging Cell, 2021, 20(5), e13359, DOI:10.1111/acel.13359.
- T. Jin, N. Guan, Y. Du, X. Zhang, J. Li and X. Xia, Cronobacter sakazakii ATCC 29544 translocated human brain microvascular endothelial cells via endocytosis, apoptosis Induction, and disruption of tight junction, Front. Microbiol., 2021, 12, 675020, DOI:10.3389/fmicb.2021.675020.
- A. L. Hsu, C. T. Murphy and C. Kenyon, Regulation of aging and age-related disease by DAF-16 and heat-shock factor, Science, 2003, 300(5622), 1142–1145, DOI:10.1126/science.1083701.
- W. C. Chiang, T. T. Ching, H. C. Lee, C. Mousigian and A. L. Hsu, HSF-1 regulators DDL-1/2 link insulin-like signaling to heat-shock responses and modulation of longevity, Cell, 2012, 148(1), 322–334, DOI:10.1016/j.cell.2011.12.019.
- M. Rodriguez, L. B. Snoek, M. De Bono and J. E. Kammenga, Worms under stress: C. elegans stress response and its relevance to complex human disease and aging, Trends Genet., 2013, 29(6), 367–374, DOI:10.1016/j.tig.2013.01.010.
- D. Garigan, A. L. Hsu, A. G. Fraser, R. S. Kamath, J. Ahringer and C. Kenyon, Genetic analysis of tissue aging in Caenorhabditis elegans: a role for heat-shock factor and bacterial proliferation, Genetics, 2002, 161(3), 1101–1112, DOI:10.1093/genetics/161.3.1101.
- A. Dichlberger, S. Schlager, P. T. Kovanen and W. J. Schneider, Lipid droplets in activated mast cells–a significant source of triglyceride-derived arachidonic acid for eicosanoid production, Eur. J. Pharmacol., 2016, 785, 59–69, DOI:10.1016/j.ejphar.2015.07.020.
- T. Shimizu, K. Sugiura, Y. Sakai, A. R. Dar, R. A. Butcher, K. Matsumoto and N. Hisamoto, Chemical signaling regulates axon regeneration via the GPCR–Gqα pathway in Caenorhabditis elegans, J. Neurosci., 2022, 42(5), 720–730, DOI:10.1523/jneurosci.0929-21.2021.
- L. J. Radeke and M. A. Herman, Identification and characterization of differentially expressed genes in Caenorhabditis elegans in response to pathogenic and nonpathogenic Stenotrophomonas maltophilia, BMC Microbiol., 2020, 20, 1–20, DOI:10.1186/s12866-020-01771-1.
|
This journal is © The Royal Society of Chemistry 2024 |
Click here to see how this site uses Cookies. View our privacy policy here.