Facile and eco-friendly synthesis of hydrogen bonding-rich bio-based bisbenzoxazine resins with low surface free energy, strong adhesion strength and high thermal stability†
Received
21st April 2023
, Accepted 16th October 2023
First published on 17th October 2023
Abstract
A facile and eco-friendly synthetic strategy has been developed to achieve a series of hydrogen bonding-rich bio-based thermosetting resins in this study. Using both safe and green solvents, we successfully synthesized target bio-based bisbenzoxazines (DcTa-fa, DcTa-sa, and DcTa-da) with high purity from five different naturally resourced raw materials. The chemical structures of the obtained bisbenzoxazine monomers were verified by nuclear magnetic resonance technology (including 1H and 13C NMR, two-dimensional 1H–1H nuclear Overhauser effect spectroscopy (NOESY), and 1H–13C heteronuclear multiple quantum coherence (HMQC)) and Fourier transform infrared spectroscopy (FT-IR). The polymerization processes were systematically investigated by differential scanning calorimetry (DSC) and in situ FT-IR analysis. Contact angle measurements were conducted and the corresponding results revealed tunable surface properties during the polymerization process of each bio-based bisbenzoxazine resin. In order to understand the relationship between the chemical structure and surface properties, more detailed FT-IR analyses were carried out to investigate the hydrogen bonding networks in the resulting polybenzoxazines. Notably, poly(DcTa-fa) presented excellent thermal stability (Td10 of 377 °C, Yc of 53.7 wt%) and strong adhesion strength (5.232 ± 0.26 MPa), while poly(DcTa-sa) and poly(DcTa-da) showed outstanding surface properties with very low surface free energy values (22.91 and 22.84 mJ m−2). These results highlight the utility of smart and sustainable benzoxazine chemistry and offer a facile and green synthetic approach to access hydrogen bonding-rich bio-based benzoxazine resins with many attractive properties.
Design, System, Application
The exceptionally flexible molecular design capability of benzoxazine resins enabled us to develop a sustainable synthetic route that concurrently considered the principles of green chemistry. Herein, renewable dihydrocoumarin and tyramine have been used as raw materials to develop a series of bio-based bisbenzoxazines via a phenol-free approach. Besides, a one-pot synthetic method is conducted, and only green solvents including ethanol and ethyl acetate are used throughout the whole synthesis and purification procedure. Moreover, neither the reaction yield nor the purity of the newly synthesized bisbenzoxazine resins is compromised. The findings achieved in the current work demonstrate the value of environmentally friendly benzoxazine chemistry and provide a simple and sustainable synthetic method to obtain multiple functional bio-based benzoxazine thermosetting resins.
|
Introduction
Nowadays, materials scientists have been pushed to prioritize green chemistry for a more sustainable future due to the worldwide energy crisis and contemporary environmental issues. Since a substantial amount of effort has been put into discovering alternative resources to petroleum-derived ones to ensure future sustainability, biomass resources are considered to be very desirable precursors for developing next-generation materials.1,2 In the last few decades, an abundance of bio-based raw materials has been used to replace their petroleum counterparts.3,4 Consequently, various bio-based polymeric materials have been synthesized from biomass resources, including plastics,5,6 thermosets,7,8 rubbers,9 and other functional polymers.10
As rapidly emerging thermosetting resins, both academia and industry have given benzoxazines much attention owing to their superior comprehensive properties, including almost nil volumetric expansion or contraction,11 extended shelf life,12 outstanding thermal and mechanical characteristics,13,14 and minimal water absorption.15 In addition, their derived polybenzoxazines generally show high glass transition temperature (Tg),16,17 excellent electrical properties,18,19 and hydrophobicity.20,21 In particular, the most appealing aspect of benzoxazine resins is that they can be easily synthesized from three different starting ingredients, i.e., phenol, primary amine, and formaldehyde, by means of the Mannich condensation. Therefore, such unique molecular characteristics provide a very versatile structural design capability for benzoxazine resins.
Polybenzoxazines are a type of hydrogen bonding-rich material because of the extensive presence of phenolic groups and tertiary amine in their structure. Consequently, hydrogen bonding plays a significant role in the properties of polybenzoxazines,22 and it also brings them a variety of unexpected features. For instance, polybenzoxazines generally possess high modulus at room temperature, high Tg, and good thermal stability. Although a large number of phenolic hydroxyl groups exist in the cross-linked network, polybenzoxazines can still exhibit very low surface energy, low water absorption, and excellent resistance to weak acids and various organic solvents.23,24 Recently, amide-containing benzoxazines have been synthesized and studied, and their involved both intra- and intermolecular hydrogen bonds were found to contribute an obvious property enhancement for the resulting polybenzoxazines.22,25
In the last decade, designing benzoxazine resins using green chemistry has been widely investigated in response to sustainable development. Various biomass resources, including renewable phenolic compounds such as sesamol,26 guaiacol,27,28 coumarins,29,30 apigenin,31,32 and resveratrol,33,34 as well as naturally derived primary amines such as furfurylamine,32,34,35 stearylamine,36 dehydroabietylamine37,38 and isohexide derived amines,39,40 have been employed in the synthesis of novel bio-benzoxazine resins. Among them, coumarins are a broad family of natural chemicals that have been exploited in the manufacture of polymeric materials, including fluorescent chemosensors41 and antifouling coatings.42 On the other hand, some coumarin derivatives, such as umbelliferone and aesculetin, were used to synthesize benzoxazine structures with excellent performances.29,30 Interestingly, dihydrocoumarin, as a coumarin derivative without bearing any phenolic and amino groups, could also be used to synthesize benzoxazine resins.43 Specifically, the amide functionality can be incorporated into the benzoxazine structure through a ring-opening reaction between dihydrocoumarin and amines.
It should also be noted that the green synthesis approach is not equal to the synthesis process only using natural renewable raw compounds. The reaction conditions used during the synthesis must be taken into consideration according to the principles of green chemistry proposed by Paul and Warner (principle numbers 5 and 7).44 Therefore, solvents used for both reaction and purification processes should be carefully chosen in terms of the green synthesis of benzoxazine resins. However, so far only 23 cases of natural renewable benzoxazines have been synthesized and purified using green and safe solvents (SCI database by January 1, 2023).26,30,35,36,45–57
The exceptionally flexible molecular design capability of benzoxazine resins enabled us to develop a sustainable synthetic route that concurrently considered the principles of green chemistry. Herein, renewable dihydrocoumarin and tyramine were used as raw materials to develop a series of bio-based bisbenzoxazines via a phenol-free approach. Tyramine is a kind of tyrosine derivative that widely exists in cheese. Besides, a recent report has confirmed that it is a promising green alternative to p-aminophenol for preparing thermosets possessing excellent processability and thermal stability.58 In addition, a one-pot synthetic method was conducted, and only green solvents including ethanol and ethyl acetate were used throughout the whole synthesis and purification procedure. Moreover, neither the reaction yield nor the purity of the newly synthesized bisbenzoxazine resins was compromised in the current study. The detailed synthetic strategy and the physical properties of the resulting amide-containing bio-polybenzoxazines with rich hydrogen bonding have been discussed in this article.
Experimental
Materials
Dihydrocoumarin (99%), tyramine (98%), furfurylamine (99%), stearylamine (97%), and paraformaldehyde (99%) were purchased from Aladdin Reagent, China. Dehydroabietylamine (90%) was purchased from Macklin Reagent, China. Absolute ethyl alcohol and ethyl acetate were purchased from Shanghai First Chemical Co., Shanghai, China. All the reagents and solvents were used as received. BA-a was synthesized according to a previous report.59
Characterization methods
The FT-IR spectra of bisbenzoxazine monomers and each polybenzoxazine sample were recorded using a Fourier transform infrared spectrophotometer (Bomem, Michelson MB 110). The 1H nuclear magnetic resonance (NMR) spectra were recorded using a Bruker AVANCE II 400 MHz NMR spectrometer. The internal standard was tetramethylsilane, while the solvent for NMR experiments was CDCl3 or DMSO-d6. The average number of transients for obtaining the 1H NMR spectrum was 64. To confirm the NMR assignments, two-dimensional 1H–1H NOESY and 1H–13C HMQC spectra were also obtained. HR-MS (high resolution mass spectrometry) was performed on a Thermo Scientific Q Exactive Orbitrap, and elemental analysis for the benzoxazine monomer was conducted on an Elementar Vario EL-III analyzer. In terms of the measurement of differential scanning calorimetry, NETZSCH DSC equipment (type 204f1) was used in N2 with a flow rate of 60 mL min−1. The temperature ramp rate was 10 °C min−1. For the lap shear test, the process was performed to evaluate the adhesion strength of the coating to the substrate using an LFM-300KN universal tester. A sample was clamped at one end and pulled from another clamped end at a constant rate (3 mm min−1) and the tests were performed at room temperature, and five different points on each sample were tested to obtain an average value. Dynamic mechanical analysis (DMA) was performed using a NETZSCH DMA/242E analyzer at a frequency of 5 Hz and a heating rate of 3 °C min−1. TGA was performed using a NETZSCH STA449-C apparatus under N2 from ambient temperature to 800 °C (heating rate 10 °C min−1). A contact angle testing apparatus was used to assess the contact angles of bio-resin coatings (TBU 100, DataPhysics). At least three drops were applied at various locations on the coatings to ensure accurate data. The surface free energy was calculated using the average of the obtained contact angles.
Synthesis of N-(2-(3-(furan-2-ylmethyl)-3,4-dihydro-2H-benzo[e][1,3]oxazin-6-yl)ethyl)-3-(3-(furan-2-ylmethyl)-3,4-dihydro-2H-benzo[e][1,3]oxazin-8-yl)propanamide (abbreviated as DcTa-fa).
Dihydrocoumarin (0.740 g, 0.005 mol), tyramine (0.685 g, 0.005 mol) and 45 mL of ethanol were successively added into a 100 mL round-bottom flask. The entire mixture was stirred for 10 minutes at RT, the reaction was then heated to 80 °C, and the mixture was stirred for 6 hours. Afterward, furfurylamine (0.971 g, 0.010 mol) and paraformaldehyde (0.660 g, 0.022 mol) were added into the flask and stirred for another 8 h. Ethyl acetate and ethanol were used for recrystallization in a 1
:
1 volume ratio. White crystals were eventually obtained (yield ca. 82%).
1H NMR (400 MHz, CDCl3), ppm: δ = 7.43 (q, J = 1.5 Hz, 2H), 7.03 (dd, J = 6.5, 2.7 Hz, 1H), 6.91 (dd, J = 8.3, 2.2 Hz, 1H), 6.88–6.72 (m, 4H), 6.36 (td, J = 3.5, 1.9 Hz, 2H), 6.26 (t, J = 3.2 Hz, 2H), 5.52 (t, J = 5.4 Hz, 1H), 4.88 (d, J = 7.1 Hz, 4H), 4.00 (d, J = 7.2 Hz, 4H), 3.92 (d, J = 8.8 Hz, 4H), 3.47 (q, J = 6.7 Hz, 2H), 2.92 (dd, J = 8.7, 6.8 Hz, 2H), 2.69 (t, J = 7.0 Hz, 2H), 2.47 (dd, J = 8.6, 6.9 Hz, 2H).
13C NMR (101 MHz, CDCl3), ppm: δ = 172.50, 152.56, 151.68, 151.54, 142.59, 131.16, 128.32, 128.16, 128.09, 127.74, 125.86, 120.39, 119.74, 119.30, 116.56, 110.24, 110.21, 108.98, 108.94, 81.93, 81.81, 77.34, 77.23, 77.02, 76.71, 49.65, 49.56, 48.28, 48.23, 40.77, 36.78, 34.99, 26.10.
Elemental analysis: calculated for C31H33N3O5: C, 70.57; H, 6.30; N, 7.96. Found: C, 70.51; H, 6.34; N, 7.99.
HR-MS (ESI) (m/z), [M + H]+ calcd. for C31H34N3O5+, 528.24930; found, 528.24902.
Synthesis of N-(2-(3-octadecyl-3,4-dihydro-2H-benzo[e][1,3]oxazin-6-yl)ethyl)-3-(3-octadecyl-3,4-dihydro-2H-benzo[e][1,3]oxazin-8-yl)propanamide (abbreviated as DcTa-sa).
Dihydrocoumarin (0.740 g, 0.005 mol), tyramine (0.685 g, 0.005 mol) and 80 mL of ethanol were added into a 250 mL round-bottom flask. The entire mixture was stirred for 10 minutes at RT, the reaction was then heated to 80 °C, and the mixture was stirred for 6 hours. Afterward, stearylamine (2.695 g, 0.010 mol) and paraformaldehyde (0.660 g, 0.022 mol) were added into the flask and stirred for another 8 h. Ethyl acetate and ethanol were used for recrystallization in a 1
:
1 volume ratio. White crystals were eventually obtained (yield ca. 75%).
1H NMR (400 MHz, CDCl3), ppm: δ = 7.00 (dd, J = 7.3, 1.9 Hz, 1H), 6.90–6.75 (m, 2H), 6.70 (d, J = 8.3 Hz, 1H), 5.53 (t, J = 5.9 Hz, 0H), 4.85 (s, 2H), 3.97 (d, J = 6.9 Hz, 2H), 3.45 (q, J = 6.7 Hz, 1H), 2.89 (dd, J = 8.7, 6.7 Hz, 1H), 2.79–2.59 (m, 3H), 2.45 (dd, J = 8.6, 6.8 Hz, 1H), 1.71–0.77 (m, 38H).
13C NMR (101 MHz, CDCl3), ppm: δ =172.52, 152.87, 151.99, 130.79, 128.11, 128.01, 127.86, 127.64, 125.72, 120.43, 120.03, 119.97, 116.37, 82.66, 82.44, 77.35, 77.03, 76.71, 51.56, 51.49, 50.36, 40.78, 36.81, 35.01, 31.92, 29.69, 29.66, 29.63, 29.56, 29.55, 29.35, 28.15, 27.29, 27.26, 26.07, 22.68, 14.09.
Elemental analysis: calculated for C57H97N3O3: C, 78.47; H, 11.21; N, 4.82. Found: C, 78.43; H, 11.28; N, 4.85.
HR-MS (ESI) (m/z), [M + H]+ calcd. for C57H98N3O3+, 872.76027; found, 872.75861.
Synthesis of N-(2-(3-((7-isopropyl-1,4a-dimethyl-1,2,3,4,4a,9,10,10a-octahydrophenanthren-1-yl)methyl)-3,4-dihydro-2H-benzo[e][1,3]oxazin-6-yl)ethyl)-3-(3-((7-isopropyl-1,4a-dimethyl-1,2,3,4,4a,9,10,10a-octahydrophenanthren-1-yl)methyl)-3,4-dihydro-2H-benzo[e][1,3]oxazin-8-yl)propanamide (abbreviated as DcTa-da).
Dihydrocoumarin (0.740 g, 0.005 mol), tyramine (0.685 g, 0.005 mol) and 80 mL of ethanol were added into a 250 mL round-bottom flask. The entire mixture was stirred for 10 minutes at RT, the reaction was then heated to 80 °C, and the mixture was stirred for 6 hours. Afterward, dehydroabietylamine (2.855 g, 0.010 mol) and paraformaldehyde (0.660 g, 0.022 mol) were added into the flask and stirred for another 8 h. Ethyl acetate and ethanol were used for recrystallization in a 1
:
1 volume ratio. Light-brown crystals were eventually obtained (yield ca. 73%).
1H NMR (400 MHz, CDCl3), ppm: δ = 7.22 (dd, J = 8.2, 2.3 Hz, 1H), 7.07–6.97 (m, 2H), 6.94 (s, 1H), 6.97–6.85 (m, 1H), 6.88–6.68 (m, 2H), 5.57 (q, J = 6.8, 6.3 Hz, 0H), 4.81–4.72 (m, 2H), 3.97–3.90 (m, 2H), 3.48 (q, J = 6.7 Hz, 1H), 3.02–2.76 (m, 4H), 2.69 (q, J = 6.9 Hz, 1H), 2.49 (dd, J = 8.7, 6.8 Hz, 1H), 2.42–2.27 (m, 2H), 1.81–1.70 (m, 3H), 1.74–1.55 (m, 1H), 1.52–1.36 (m, 2H), 1.27 (d, J = 7.0 Hz, 10H), 1.01 (dd, J = 8.2, 3.1 Hz, 1H), 0.95–0.85 (m, 4H), 0.85 (s, 1H).
13C NMR (101 MHz, CDCl3), ppm: δ = 172.64, 153.54, 152.73, 147.65, 145.53, 134.62, 130.56, 128.24, 128.16, 127.96, 127.57, 127.42, 126.92, 125.62, 124.20, 123.87, 121.22, 120.75, 119.77, 116.63, 85.24, 77.43, 77.11, 76.79, 62.73, 62.68, 54.13, 43.68, 40.88, 39.13, 38.50, 37.46, 36.88, 35.98, 35.96, 35.06, 33.49, 30.26, 26.13, 25.68, 24.05, 19.36, 19.34, 18.86, 18.82.
Elemental analysis: calculated for C61H81N3O3: C, 81.02; H, 9.03; N, 4.65. Found: C, 81.00; H, 9.07; N, 4.66.
HR-MS (ESI) (m/z), [M + H]+ calcd. for C61H82N3O3+, 904.63507; found, 904.63312.
Polymerization of bio-based benzoxazine resins
DcTa-fa, DcTa-sa and DcTa-da were poured into a glass sample bottle, then put into a furnace and cured following the heating process of 120 °C/1 h + 140 °C/1 h + 160 °C/1 h + 180 °C/1 h + 200 °C/1 h + 220 °C/1 h + 240 °C/1 h to obtain bio-based benzoxazine resins, namely poly(DcTa-fa), poly(DcTa-sa) and poly(DcTa-da). In addition, BA-a was cured using the same method to form poly(BA-a).
Computational methods
The optimized structure of all monomers was calculated using the Gaussian 09 program.60 Specifically, the calculation method was set as DFT with the B3LYP hybrid function, while the basis set used was 6-31G(d).
Results and discussion
Green design, eco-friendly synthesis, and structural characterization of bio-based bisbenzoxazine monomers
Herein we chose a series of biomass resources, including dihydrocoumarin, tyramine, furfurylamine, stearylamine and dehydroabietylamine, for the synthesis of new bio-benzoxazines. Furfurylamine, which is a furfural derivative from corn, was a reagent for producing polybenzoxazines with high thermal stability owing to the extra sites of cross-linking provided by the furan group.61 Stearylamine and dehydroabietylamine, which are derived from soybean oil and rosin, can improve the filming processability.38,62 Kaya et al. first reported the synthesis of amide-containing benzoxazines from dihydrocoumarin. However, the phenolic precursors should be prepared via a ring-opening reaction between dihydrocoumarin and primary amines.43 As shown in Fig. 1, a readily one-pot synthetic method has been designed for obtaining each bio-benzoxazine in the current work, which enables direct access to products from accessible raw materials without separating any intermediates. After the reaction between dihydrocoumarin and tyramine for 6 h, we took out a small amount of reaction solution and evaporated the reaction solvent for the 1H NMR measurement (see Fig. S1†). The high conversion confirmed from the NMR spectrum grants the high yield of the amide-containing phenolic intermediate obtained in this work. On the other hand, both ethanol and ethyl acetate were used between the synthesis and purification steps, which are considered as green solvents.63
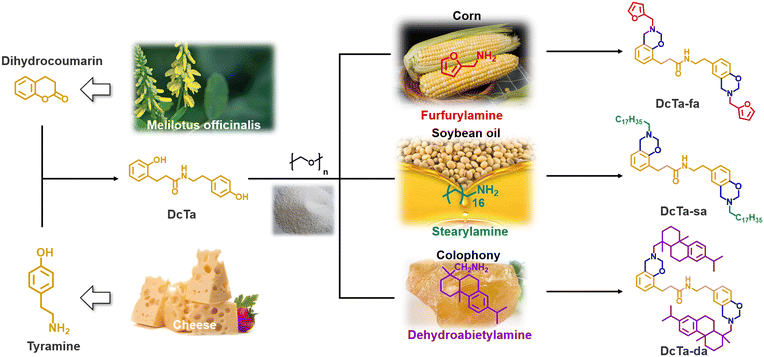 |
| Fig. 1 Design and synthetic strategy of bisbenzoxazine monomers using renewable raw materials. | |
To establish the precise structure of each benzoxazine monomer, a combined analysis of NMR (Fig. 2 and S2–S7†), FT-IR (Fig. S8†), and HR-MS (Fig. S9–S11†) spectroscopy was carried out. The detailed 1H and 13C assignments and 2D 1H–13C HMQC NMR spectra are shown in Fig. 2. In general, the two distinct methylene groups in the oxazine ring are often ascribed to two different resonances with equal intensity in the 1H NMR spectrum for 1,3-benzoxazine. Both methylene signals were found at around 4.0 and 4.8 ppm for this series of bio-based bisbenzoxazines. Besides, the resonance for the typical proton of the amide group was observed at around 5.5 ppm. As can be seen from the 1H NMR spectrum of DcTa-fa, three protons corresponding to Hd, He, and Hf are located at 6.26, 6.36, and 7.43 ppm, respectively. Another resonance centered at 3.92 ppm is the characteristic methylene signal for the RN-CH2-furan. Additionally, the distinctive resonances of the aliphatic chains can be found in the region of 0.5 to 2.8 ppm for DcTa-sa as shown in Fig. 2(b). Similarly, the protons assigned to the methyl and methylene groups derived from dehydroabietylamine can also be found between 0.5 and 3.0 ppm.
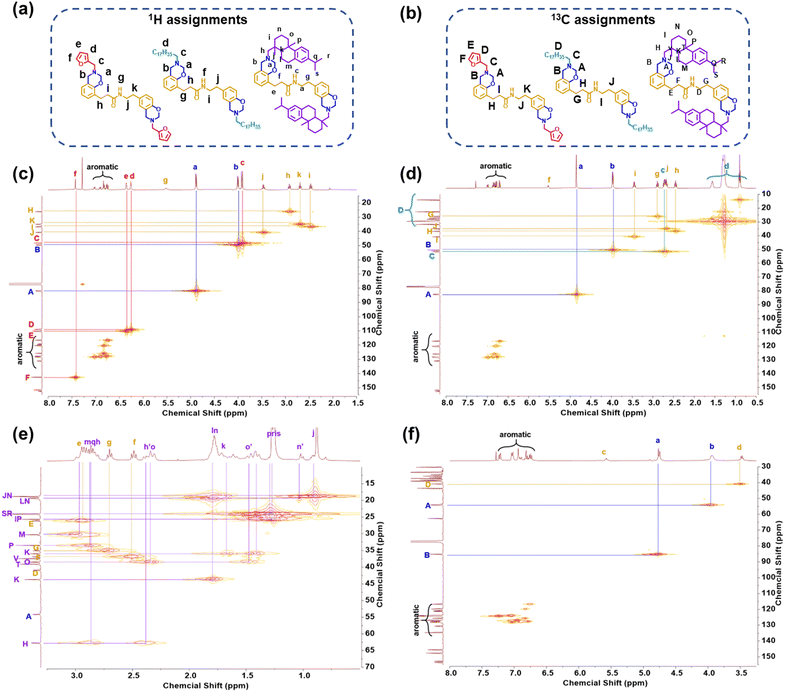 |
| Fig. 2
1H assignments (a) and 13C assignments (b) of benzoxazine monomers. 2D 1H–13C HMQC NMR spectra of DcTa-fa (c), DcTa-sa (d) and DcTa-da (e and f) in CDCl3. | |
The 13C NMR spectra of the obtained bisbenzoxazine monomers are shown in Fig. S5–S7.† The characteristic carbon resonances are found around 50 and 82 ppm, which can be assigned to Ar–CH2–N- and –O–CH2–N-groups, respectively, confirming the presence of the oxazine ring fused to the benzene group. The signals that appeared at 108.94, 110.21, and 142.59 ppm can be assigned to CD, CE and CF from the furan structure in DcTa-fa. In addition, the carbon atoms for the methyl and methylene groups in DcTa-sa and DcTa-da can be found in the range of 10 to 45 ppm. The precise protons and carbon assignments for each benzoxazine monomer are also shown in Fig. 2.
FT-IR spectra (Fig. S8†) were obtained in order to further confirm the presence of the characteristic functionalities in each benzoxazine monomer. The characteristic oxazine ring absorption bands, which are attributed to the antisymmetric stretching mode of C–O–C and the oxazine ring-related mode, respectively, were found to occur at 1235 and 932 cm−1. Additionally, the typical bands at 1501, 1078, and 745 cm−1 are due to the stretching of the C
C double bond, the stretching of the C–O antisymmetric bond, and the wagging of the C–H out-of-plane in phase, respectively. These typical bands strongly support the successful introduction of the furan structure in DcTa-fa. The C–H stretching from aliphatic chains at 2922 and 2849 cm−1 for DcTa-sa exhibited very high intensity. Meanwhile, DcTa-da showed distinctive absorption peaks at 2926 and 2865 cm−1, which are attributed to the symmetric and asymmetric stretching of methyl groups. Moreover, the HR-MS spectra (Fig. S9–S11†) indicate that the experimental m/z values of protonated species are in accordance with the theoretical outcomes, which further supports the successful synthesis of each target bio-based bisbenzoxazine structure.
Thermal polymerization behaviors of bisbenzoxazine monomers
DSC was conducted to keep track of the polymerization behavior of each bio-based bisbenzoxazine. Two thermal events can be seen in the DSC thermograms of all monomers as illustrated in Fig. 3(a). The melting process can be supported by the endothermic phase, which showed peak temperatures centered at 160.6, 102.3 and 105.6 °C for DcTa-fa, DcTa-sa and DcTa-da, respectively. Besides, the DSC thermograms of DcTa-fa, DcTa-sa and DcTa-da also exhibited exothermic peaks centered at 224.4, 251.3 and 264.3 °C, respectively, which are due to thermally triggered ring-opening polymerization of benzoxazines. Specifically, DcTa-fa showed a second overlapping exothermic event, which results from cross-linking of the furan structure at elevated temperatures.34,61 Additionally, DcTa-fa exhibited a relatively lower polymerization temperature compared with other bio-based benzoxazine monomers,26,34,53,64 which is possibly owing to the cooperative electrical impact of the adjacent oxazine ring and furfurylamine. The furan ring is easier to be substituted because the energy barrier between furan substituted transition state and iminium intermediate is much smaller than the energy barrier between transition state and iminium intermediate of other substituted structure,65 which result in the relative lower curing temperature of furan-based benzoxazine than others derived from aniline and aliphatic amine. On the other hand, both DcTa-sa and DcTa-da exhibited a great temperature gap between endothermic and exothermic peaks. Such a wider processing window suggests their excellent processability. Although DcTa-sa and DcTa-da possess amide functionality, both benzoxazines show unexpectedly higher polymerization temperatures compared with DcTa-fa and other amide-containing benzoxazine resins.19,66,67 In general, the electrical effect from the surrounding groups and steric effects have strong influences on ring-opening polymerization.68 In order to understand this anomalous polymerization behavior, we performed DFT calculations to optimize the structure of each monomer by the DFT B3LYP/6-31G(d) method. As shown in Fig. 3(b)–(d), DcTa-fa shows a quite different configuration in comparison with DcTa-sa and DcTa-da, which is owing to the large chain segments of stearylamine and dehydroabietylamine. Clearly, the steric effects existed in the configurations of DcTa-sa and DcTa-da resulting in higher ring-opening polymerization temperatures. Particularly, the oxazine rings in DcTa-da are wrapped by the multivariate ring structure derived from dehydroabietylamine, which leads to the highest polymerization temperature of DcTa-da.
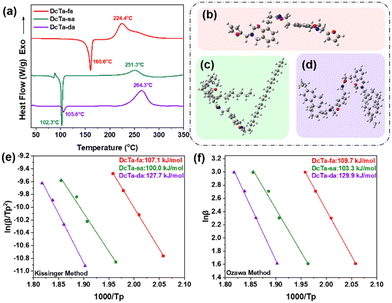 |
| Fig. 3 (a) DSC curves of monomers. The optimized structures of (b) DcTa-fa, (c) DcTa-sa and (d) DcTa-da; representations of the Kissinger method (e) and Ozawa method (f) for the calculation of activation energy. | |
DSC tests using different heating rates were conducted to study the curing kinetics by both Kissinger and Ozawa theories.69,70 The following are representations of the Kissinger and modified Ozawa equations, which were used to calculate activation energy (Ea) values, respectively.
| 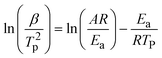 | (1) |
| 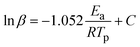 | (2) |
where
β stands for the heating rate,
A is the pre-exponential factor,
Tp stands for the temperature at the exothermic peak maximum,
R stands for the gas constant, and
C is a constant.
Fig. S12–S14† show the DSC curves of the monomers at various heating ramp rates (5, 10, 15, 20 °C min−1), and Fig. 3(e) and (f) show the plots of ln(β/T2p) and ln(β) versus 1/Tp for all monomers utilizing the Kissinger and Ozawa methods. According to the Kissinger hypothesis, the Ea values for DcTa-fa, DcTa-sa and DcTa-da were calculated to be 107.1, 100.0 and 127.7 kJ mol−1, while other results were 109.7, 103.3 and 129.9 kJ mol−1 based on the calculation from the Ozawa method. The results of the DSC analysis are summarized in Table 1.
Table 1 DSC analysis of monomers
Monomer |
DSC temperature value (°C) |
Kissinger activation energy (kJ mol−1) |
Ozawa activation energy (kJ mol−1) |
T
m
|
T
o
|
T
p
|
DcTa-fa
|
160.6 |
202.2 |
224.4 |
107.1 |
109.7 |
DcTa-sa
|
102.3 |
219.7 |
251.3 |
100.0 |
103.3 |
DcTa-da
|
105.6 |
232.4 |
264.3 |
127.7 |
129.9 |
To further understand the thermal polymerization process of these bio-based bisbenzoxazine monomers, in situ FT-IR spectra were then obtained. The infrared absorption spectra exhibit no fluctuations from ambient temperature to 160 °C for all three monomers as illustrated in Fig. 4(a)–(c). As the heating temperature further increased, the characteristic bands attributed to the C–O–C antisymmetric stretching mode (around 1225 cm−1), C–O antisymmetric stretching mode (around 1075 cm−1), and oxazine ring-related mode (around 923 cm−1) all decreased gradually. At 200 °C, the aforementioned distinctive bands of DcTa-fa completely vanished, signifying that the oxazine ring has been fully cured. On the other hand, the completion for the polymerization of DcTa-sa and DcTa-da was found to be at 220 and 240 °C, which is in accordance with the above DSC result. As the polymerization temperature further increased, the usual band of –OH (a broad band at approximately 3300 cm−1) started to emerge. This characteristic band with a broad background generally appeared between 2600 and 3700 cm−1, which proves the generation of different intra- or inter-hydrogen bonding.22 The band at 730 cm−1 in DcTa-fa (C– H out-of-plane wagging deformation from the furan structure) started to diminish when the polymerization temperature reached 180 °C, showing more cross-linking polymerization of the furan structure. This band completely disappeared as the temperature rose to 240 °C, signifying that the polymerization of the furan group in DcTa-fa was also completed. Therefore, the hypothesized thermally activated ring-opening polymerization processes for these bio-based bisbenzoxazines are shown in Fig. 4(d and e) according to the results gathered from DSC and in situ FT-IR investigations.
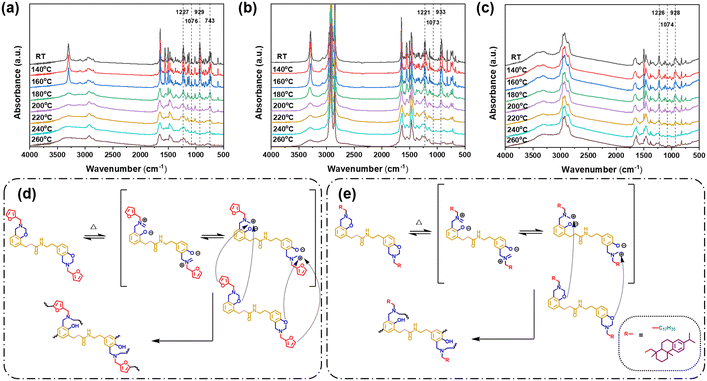 |
| Fig. 4
In situ FT-IR spectra of benzoxazine monomers: (a) DcTa-fa, (b) DcTa-sa and (c) DcTa-da. Proposed polymerization behaviors of (d) DcTa-fa and (e) DcTa-sa and DcTa-da. | |
Hydrogen bonding structures in bio-based polybenzoxazines
According to previous research, the overall hydrogen bonding significantly influences the properties of the resulting polybenzoxazine.22 The various types of hydrogen bonding involved in the obtained bio-polybenzoxazines are depicted in the FT-IR spectra as shown in Fig. 5(a). Based on the chemical structures of the cured bio-polybenzoxazines, the typical bands at around 2925 cm−1, 3120 cm−1 and 3600 cm−1 can be ascribed to intramolecular hydrogen bonding of RCON–H⋯N/O, intramolecular hydrogen bonding of ArOH⋯N, and intramolecular hydrogen bonding of –OH⋯π or free –OH, respectively. Besides, the typical bands at 3350 cm−1 can be assigned to the combination of the inter- and intramolecular hydrogen bonding of ArOH⋯O and ArOH⋯H since all typical bands are heavily overlapped in this range. In addition, the splitting FT-IR spectra between 2650 and 3700 cm−1 of poly(DcTa-fa), poly(DcTa-sa) and poly(DcTa-da) are shown in Fig. 5(b)–(d). The nitrogen atom in the Mannich bridge could have a great impact on the distribution of hydrogen bonding.71Poly(DcTa-da) showed almost no intramolecular hydrogen bonding of –OH⋯π in its structure. The ratio of intramolecular hydrogen bonding of RCON–H⋯N/O in poly(DcTa-sa) and poly(DcTa-da) was much higher than that in poly(DcTa-fa), which is probably owing to the steric effect derived from their amine resources. Moreover, an illustration of different types of hydrogen bonding and the hydrogen bonding-rich structure of the resulting bio-polybenzoxazine is shown in Fig. 5(e). Since the extensive existence of hydrogen bonding in these bio-polybenzoxazines can play a special role in the surface properties, the corresponding surface property evaluation has also been performed in the following section.
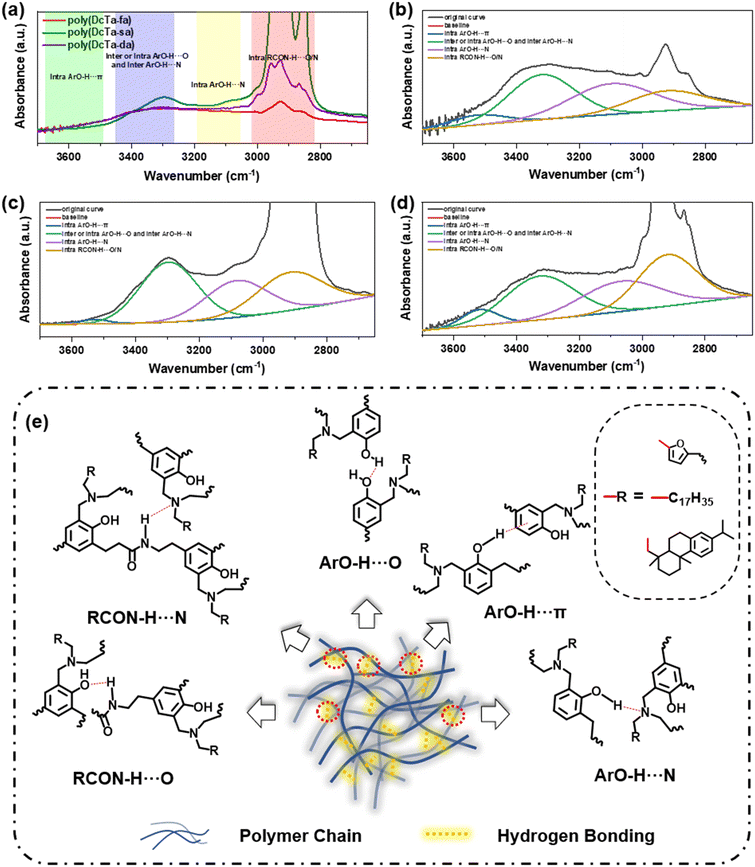 |
| Fig. 5 (a) Various types of hydrogen bonding in the obtained bio-polybenzoxazines depicted in FT-IR spectra. Splitting FT-IR spectra between 2650 and 3700 cm−1 of poly(DcTa-fa) (b), poly(DcTa-sa) (c) and poly(DcTa-da) (d). (e) Proposed illustration for different types of hydrogen bonding in bio-polybenzoxazines. | |
The surface behavior of bio-based benzoxazine coatings
The bio-based resin coatings were prepared through brush coating processes. Specifically, each bisbenzoxazine monomer was dissolved in ethyl acetate to obtain a solution with 10 wt% resin content. Then it was applied to a glass slide substrate that has been cleaned using ultrasonication. The coated glass slides were heated at 50 °C for 8 hours in a vacuum oven in order to remove the organic solvent, and a layer of the bio-benzoxazine coating film was then formed. The water contact angles for all the coated surfaces after various thermal treatments are shown in Fig. 6(a)–(c). The coatings based on DcTa-fa, DcTa-sa and DcTa-da all showed water contact angles (CAs) that were greater than 90° after various thermal treatments, demonstrating the good surface hydrophobicity of these hydrogen bonding-rich bio-benzoxazine coatings. Even though the polybenzoxazine network contained large amounts of both the phenolic groups generated from the ring-opening of the oxazine ring, all the bio-resin coatings exhibited excellent hydrophobicity after thermal treatments. The robust intramolecular hydrogen bond, in particular for the ArOH⋯O/N link, can prevent the phenolic groups in the polymer network from interacting with water, hence yielding the hydrophobic character of this series of bio-polybenzoxazines. Additionally, DcTa-sa and DcTa-da have substantially greater water CA values than DcTa-fa due to the abundant existence of alkyl groups in their structures.
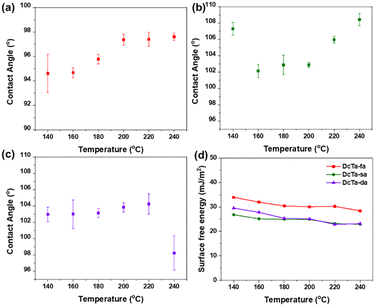 |
| Fig. 6 Water contact angles of (a) DcTa-fa, (b) DcTa-sa and (c) DcTa-da after various thermal treatments. (d) Surface free energies of bio-based bisbenzoxazine coatings after various thermal treatments calculated by the OWRK mode. | |
The OWRK mode based on Owen's three-liquid method72 was employed to determine the surface free energy of these three bio-benzoxazine-derived coatings after various thermal treatments by using the contact angles of water, ethylene glycol (EG), and diiodomethane (DIM). The surface property testing results are shown in Fig. 6(d) and Table 2. In general, the hydrogen bonding network plays significant roles in the value of surface-free energy. With the increase in heating temperature, the hydrogen bonding was generated gradually, which resulted in a gradual downward trend of surface free energy. The lowest values of surface free energy were obtained for DcTa-sa (22.91 mJ m−2) and DcTa-da (22.84 mJ m−2) after they were thermally treated at 240 °C and 220 °C, while DcTa-fa showed the lowest surface free energy after its thermal treatment at 240 °C. The relatively lower surface free energy values for poly(DcTa-sa) and poly(DcTa-da) are probably due to the joint impact of alkyl and hydrogen bonding. Therefore, all the hydrogen bonding-rich bio-polybenzoxazine coatings exhibit low surface free energy values after curing, which are even much lower than those of our recently reported Priamine 1074 bio-based benzoxazine resins.73
Table 2 Surface properties of bio-benzoxazine coatings after various thermal treatments
Sample name |
Terminal curing temperature (°C) |
Contact angle (°) |
Surface free energy (mJ m−2) |
H2O |
EG |
DIM |
DcTa-fa
|
140 °C |
94.60 |
66.57 |
47.67 |
33.93 |
160 °C |
94.67 |
69.33 |
51.30 |
31.95 |
180 °C |
95.77 |
71.53 |
53.77 |
30.38 |
200 °C |
97.37 |
71.53 |
54.77 |
30.03 |
220 °C |
97.40 |
72.57 |
53.87 |
30.2 |
240 °C |
97.60 |
75.07 |
56.93 |
28.37 |
DcTa-sa
|
140 °C |
107.30 |
74.10 |
64.30 |
26.8 |
160 °C |
102.13 |
81.10 |
62.27 |
25.11 |
180 °C |
102.87 |
83.00 |
61.87 |
24.89 |
200 °C |
102.87 |
83.70 |
61.50 |
24.87 |
220 °C |
105.97 |
83.87 |
66.43 |
23.16 |
240 °C |
108.43 |
82.73 |
68.67 |
22.91 |
DcTa-da
|
140 °C |
102.97 |
74.47 |
55.87 |
29.52 |
160 °C |
103.00 |
76.83 |
58.90 |
27.76 |
180 °C |
103.13 |
81.67 |
61.67 |
25.33 |
200 °C |
103.83 |
81.47 |
62.63 |
25.09 |
220 °C |
104.23 |
84.53 |
66.43 |
22.84 |
240 °C |
98.23 |
82.43 |
66.03 |
23.14 |
Adhesion properties of bio-based polybenzoxazines
The adhesion to the substrate of low surface energy-based coatings is a major barrier for practical applications.74,75 The surface energy of the coating should be low enough and within the acceptable range of the Baier curve, but it should not be as low as to compromise the adhesive strength to target surfaces. The interactions between the adhesive and the substrate, as well as the wettability and viscosity of the adhesive, all affect the adhesive strength to a surface.76 The lap shear test was performed to evaluate the adhesion strength of all the newly obtained bio-based polybenzoxazines. Schematic diagrams of the sample size and lap shear test, as well as the test results, are shown in Fig. 7. A commercialized benzoxazine resin, BA-a (Fig. S15†), was also synthesized and investigated for comparison. The adhesion strength of poly(BA-a), poly(DcTa-fa), poly(DcTa-sa) and poly(DcTa-da) was found to be 4.032 ± 0.45, 5.232 ± 0.26, 1.770 ± 0.21, and 0.443 ± 0.22 MPa, respectively. The adhesion strength of poly(DcTa-fa) was improved by ∼30% compared to that of poly(BA-a). However, when the soft aliphatic segment was incorporated into the polybenzoxazine structure, the adhesion strength decreased remarkably. Nevertheless, such polybenzoxazines bearing aliphatic chains still showed some potential application in marine environments.42
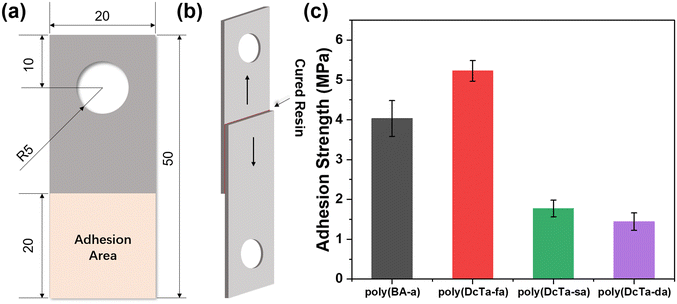 |
| Fig. 7 Schematic diagrams of the sample size (unit of length: mm) (a) and lap shear test (b). (c) Adhesion properties of polybenzoxazines. | |
Thermal properties of bio-based polybenzoxazines
The thermal stability of bio-polybenzoxazines was evaluated by thermogravimetric analysis (TGA), and the corresponding thermograms are shown in Fig. 8(a) and (b). As shown in Fig. 8(a), negligible weight loss can be observed below 250 °C for all these bio-polybenzoxazines. When the temperature was further raised, the Td5 values (the temperature at a weight loss of 5 wt%) occurred at 319.2, 278.8 and 297.3 °C for poly(DcTa-fa), poly(DcTa-sa) and poly(DcTa-da), respectively. On the other hand, the Td10 values (the temperature at a weight loss of 10 wt%) occurred at 377.1, 299.4 and 319.9 °C for poly(DcTa-fa), poly(DcTa-sa) and poly(DcTa-da), respectively. The fact that poly(DcTa-fa) has a higher initial degradation temperature than the other two bio-polybenzoxazines suggests its higher cross-linking density which is derived from the further cross-linking of the furan moiety. The additional electrophilic sites at the ortho-oxygen position in the furan ring may provide an extra cross-linking point which contributes to the higher cross-linking density.32,34,35 In addition, the steric effect of a large aliphatic chain derived from stearylamine and dehydroabietylamine may hinder the formation of hydrogen bonding, which results in relatively lower thermal stability. Moreover, the char yield obtained at 800 °C was found to be 53.7%, 19.1%, and 19.8%, respectively. Fig. 8b shows the derivative weight loss (DTG) curves of this new series of bio-polybenzoxazines during heating. According to previous research,77 polybenzoxazines typically undergo three procedures during heat breakdown: firstly, the amine may depart from the chain ends or branches; secondly, amine derivatives evaporate from the main chain; finally, the phenolic linkage breaks followed by the Mannich base degradation. The initial degradation stage was reported to be conducted before 300 °C.78Poly(DcTa-fa) showed a very low degradation rate before 300 °C (0.082%/°C), which indicates the presence of degradation for the relatively small molecular chain ends and branches as compared to other recently reported high performance polybenzoxazines (both higher than 0.1%/°C).73,79 As the temperature increased to 350 °C, poly(DcTa-sa) and poly(DcTa-da) showed a rapidly increasing degradation rate which may be owing to the evaporation of stearylamine, dehydroabietylamine and their derivatives. The whole degradation process of poly(DcTa-da) seemed more homogeneous, while it showed a lower degradation temperature for its main degradation stage, which can be owing to the unstable Mannich bridge in poly(DcTa-da). Moreover, the DTG curve of poly(DcTa-fa) exhibited relatively low decomposition rates throughout its whole degradation process. Such a low decomposition rate during thermal degradation is quite advantageous from the perspective of flammability.
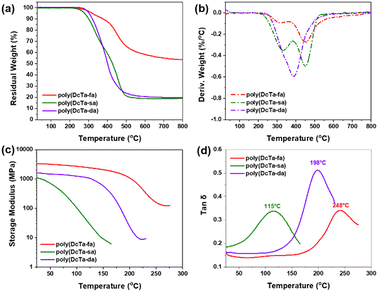 |
| Fig. 8 TGA (a and b) and DMA curves (c and d) of derived bio-polybenzoxazines. | |
Dynamic mechanical analysis (DMA) was conducted to assess the thermo-mechanical characteristics of all the bio-polybenzoxazines. The storage modulus of poly(DcTa-fa) at RT was found to be 3400 MPa, which indicates its high rigidity. However, the storage modulus of poly(DcTa-sa) and poly(DcTa-da) was found to be 1700 and 1100 MPa at RT, respectively. The relatively lower stiffness of poly(DcTa-sa) and poly(DcTa-da) should be owing to the presence of a large amount of aliphatic series in their networks. The glass transition temperature (Tg) was obtained using the peak temperature of the tan
δ from the DMA curve. The Tg values of poly(DcTa-fa), poly(DcTa-sa) and poly(DcTa-da) were found to be 248, 115 and 198 °C. The high Tg of poly(DcTa-fa) is derived from the high cross-linking density that comes from the ring-opening of the oxazine ring and the additional cross-linking of the furan ring. In addition, the flexible alkylene chains included in polybenzoxazine networks provide a plasticization effect that results in the relatively lower Tg values of poly(DcTa-sa) and poly(DcTa-da). The cross-linking density of poly(DcTa-fa), poly(DcTa-sa) and poly(DcTa-da) was also calculated by the DMA method based on a classical formula Ve = E/6RT. To be more precise, E represents the storage modulus of the rubber platform region, typically measured at Tg + 40 °C based on the DMA findings, R denotes the standard gas constant, and T signifies the absolute temperature of Tg.
The data corresponding to the thermal properties of the derived bio-polybenzoxazines are summarized in Table 3.
Table 3 The data corresponding to the thermal properties of bio-polybenzoxazines
Samples |
T
d5 (°C) |
T
d10 (°C) |
Y
c (%) |
T
g (°C) (DMA) |
Cross-linking density (mol m−3) |
Poly(DcTa-fa)
|
319.2 |
377.1 |
53.7 |
248 |
3.35 × 105 |
Poly(DcTa-sa)
|
278.8 |
299.4 |
19.1 |
115 |
0.31 × 105 |
Poly(DcTa-da)
|
297.3 |
319.9 |
19.8 |
198 |
0.42 × 105 |
Conclusions
A new series of bio-based bisbenzoxazine resins were successfully synthesized in the current work using a facile one-pot reaction. The whole preparation process of all resins also complied with the principles of green chemistry. Besides, bio-polybenzoxazines with rich hydrogen bonding networks were revealed by incorporation of an amide functionality into the benzoxazine structure via a smart phenol-free synthetic approach. The resulting bio-polybenzoxazine, poly(DcTa-fa), showed exceptionally high thermal stability (Td10 of 377 °C, Tg of 248 °C) and strong adhesion strength (5.232 ± 0.26 MPa), while the other two bio-polybenzoxazines, poly(DcTa-sa) and poly(DcTa-da), exhibited very low surface free energy values (22.91 and 22.84 mJ m−2). The whole work demonstrates the effectiveness of intelligent and sustainable benzoxazine chemistry, providing a simple and environmentally friendly synthetic method for producing bio-based benzoxazine resins with abundant hydrogen bonding and numerous appealing properties including exceptionally high thermal stability and strong adhesion strength. The outstanding performance achieved in the current work grants the polybenzoxazines great potential to be used as flame-resistant materials and anti-corrosion coatings. These findings demonstrate the value of environmentally friendly benzoxazine chemistry and provide a simple and sustainable synthetic method to obtain multiple functional bio-based benzoxazine thermosetting resins.
Conflicts of interest
The authors declare no competing financial interest.
Acknowledgements
The authors acknowledge the financial support from the National Natural Science Foundation of China (52073125) and the Qinglan Project of Jiangsu Province of China.
Notes and references
- V. Froidevaux, C. Negrell, S. Caillol, J.-P. Pascault and B. Boutevin, Chem. Rev., 2016, 116, 14181–14224 CrossRef CAS PubMed
.
- C. Zhang, J. Xue, X. Yang, Y. Ke, R. Ou, Y. Wang, S. A. Madbouly and Q. Wang, Prog. Polym. Sci., 2022, 125, 101473 CrossRef CAS
.
- R. M. Cywar, N. A. Rorrer, C. B. Hoyt, G. T. Beckham and E. Y.-X. Chen, Nat. Rev. Mater., 2022, 7, 83–103 CrossRef CAS
.
- P. Yuan, Y. Sun, X. Xu, Y. Luo and M. Hong, Nat. Chem., 2022, 14, 294–303 CrossRef CAS PubMed
.
- M. Parit and Z. Jiang, Int. J. Biol. Macromol., 2020, 165, 3180–3197 CrossRef CAS PubMed
.
- S. Walker and R. Rothman, J. Cleaner Prod., 2020, 261, 121158 CrossRef CAS
.
- G. Liu, C. Jin, S. Huo, Z. Kong and F. Chu, Int. J. Biol. Macromol., 2021, 193, 1400–1408 CrossRef CAS PubMed
.
- J. Liu, S. Wang, Y. Peng, J. Zhu, W. Zhao and X. Liu, Prog. Polym. Sci., 2021, 113, 101353 CrossRef CAS
.
- Y. Chen, J. Nie, C. Xu, W. Wu and Z. Zheng, ACS Sustainable Chem. Eng., 2019, 8, 1285–1294 CrossRef
.
- Z. Zhang, C. Kang, S. B. Peh, D. Shi, F. Yang, Q. Liu and D. Zhao, J. Am. Chem. Soc., 2022, 144, 14992–14996 CrossRef CAS PubMed
.
- H. Ishida and H. Y. Low, Macromolecules, 1997, 30, 1099–1106 CrossRef CAS
.
- M. G. Mohamed, S. W. Kuo, A. Mahdy, I. M. Ghayd and K. I. Aly, Mater. Today Commun., 2020, 25, 101418 CrossRef CAS
.
- Y. Lu, X. Yu, C. J. Evans, S. Yang and K. Zhang, Polym. Chem., 2021, 12, 5059–5068 RSC
.
- K. Zhang and X. Yu, Macromolecules, 2018, 51, 6524–6533 CrossRef CAS
.
- R. Yang, L. Xie, N. Li, P. Froimowicz and K. Zhang, Polym. Chem., 2022, 12, 3639–3649 RSC
.
- Y. Lu, X. Yu, L. Han and K. Zhang, Polymers, 2021, 13, 1417 CrossRef CAS PubMed
.
- M. G. Mohamed, C.-J. Li, M. A. R. Khan, C.-C. Liaw, K. Zhang and S.-W. Kuo, Macromolecules, 2022, 55, 3106–3115 CrossRef CAS
.
- J. Chen, M. Zeng, Z. Feng, T. Pang, Y. Huang and Q. Xu, ACS Appl. Polym. Mater., 2019, 1, 625–630 CrossRef CAS
.
- K. Zhang, L. Han, P. Froimowicz and H. Ishida, Macromolecules, 2017, 6552–6560 CrossRef CAS
.
- A. Adjaoud, L. Puchot, C. E. Federico, R. Das and P. Verge, Chem. Eng. J., 2023, 453, 139895 CrossRef CAS
.
- Z. Zhou, C. Li, R. Wang, H. Tao, D. Yang, S. Qin, X. Meng and C. Zhou, Prog. Org. Coat., 2022, 171, 107059 CrossRef CAS
.
- X. Shen, L. Cao, Y. Liu, J. Dai, X. Liu, J. Zhu and S. Du, Macromolecules, 2018, 51, 4782–4799 CrossRef CAS
.
- J. Liu, X. Lu, Z. Xin and C. Zhou, Langmuir, 2013, 29, 411–416 CrossRef CAS PubMed
.
- L. Qu and Z. Xin, Langmuir, 2011, 27, 8365–8370 CrossRef CAS PubMed
.
- K. Zhang, L. Han, P. Froimowicz and H. Ishida, Macromolecules, 2017, 50, 6552–6560 CrossRef CAS
.
- M. A. L. Salum, D. Iguchi, C. R. Arza, L. Han, H. Ishida and P. Froimowicz, ACS Sustainable Chem. Eng., 2018, 6, 13096–13106 CrossRef CAS
.
- A. M. Chong, S. A. Salazar and J. F. Stanzione III, ACS Sustainable Chem. Eng., 2021, 9, 5768–5775 CrossRef CAS
.
- A. Trejo-Machin, A. Adjaoud, L. Puchot, R. Dieden and P. Verge, Eur. Polym. J., 2020, 124, 109468 CrossRef CAS
.
- P. Froimowicz, C. R. Arza, L. Han and H. Ishida, ChemSusChem, 2016, 9, 1921–1928 CrossRef CAS PubMed
.
- Y. Lu, Y. Zhang and K. Zhang, Chem. Eng. J., 2022, 137670 CrossRef CAS
.
- B. Hao, R. Yang and K. Zhang, RSC Adv., 2020, 10, 25629–25638 RSC
.
- K. Zhang, Y. Liu, M. Han and P. Froimowicz, Green Chem., 2020, 22, 1209–1219 RSC
.
- K. Zhang, M. Han, L. Han and H. Ishida, Eur. Polym. J., 2019, 116, 526–533 CrossRef CAS
.
- K. Zhang, M. Han, Y. Liu and P. Froimowicz, ACS Sustainable Chem. Eng., 2019, 7, 9399–9407 CrossRef CAS
.
- Y. Lu and K. Zhang, Eur. Polym. J., 2021, 156, 110607 CrossRef CAS
.
- C. Wang, J. Sun, X. Liu, A. Sudo and T. Endo, Green Chem., 2012, 14, 2799–2806 RSC
.
- X. Liu, R. Zhang, T. Li, P. Zhu and Q. Zhuang, ACS Sustainable Chem. Eng., 2017, 5, 10682–10692 CrossRef CAS
.
- X. Piao, M. Xie, X. Duan, C. Jin and Z. Wang, Constr. Build. Mater., 2022, 319, 126123 CrossRef CAS
.
- N. Amarnath, S. Shukla and B. Lochab, ACS Sustainable Chem. Eng., 2019, 7, 18700–18710 CrossRef CAS
.
- N. Amarnath, S. Mukherjee and B. Lochab, ACS Sustainable Chem. Eng., 2021, 9, 7550–7560 CrossRef CAS
.
- D. Cao, Z. Liu, P. Verwilst, S. Koo, P. Jangjili, J. S. Kim and W. Lin, Chem. Rev., 2019, 119, 10403–10519 CrossRef CAS PubMed
.
- H. Guo, L. Song, J. Hu, T. Lin, X. Li, H. Yu, D. Cheng, Y. Hou, X. Zhan and Q. Zhang, Chem. Eng. J., 2021, 420, 127676 CrossRef CAS
.
- G. Kaya, B. Kiskan and Y. Yagci, Polym. Chem., 2019, 10, 1268–1275 RSC
.
-
A. Paul, T. Warner and C. John, Green chemistry: theory and practice, Oxford University Press, Oxford [England], New York, 1998, vol. 11, p. 1394013941 Search PubMed
.
- N. Amarnath, S. Shukla and B. Lochab, ACS Sustainable Chem. Eng., 2018, 6, 15151–15161 CrossRef CAS
.
- L. Dumas, L. Bonnaud, M. Olivier, M. Poorteman and P. Dubois, Eur. Polym. J., 2016, 75, 486–494 CrossRef CAS
.
- L. Dumas, L. Bonnaud, M. Olivier, M. Poorteman and P. Dubois, Green Chem., 2016, 18, 4954–4960 RSC
.
- Y. Liu, L. Yuan, G. Liang and A. Gu, Eur. Polym. J., 2022, 111320 CrossRef CAS
.
- J. R. Oliveira, L. R. V. Kotzebue, S. E. Mazzetto and D. Lomonaco, Eur. Polym. J., 2019, 116, 534–544 CrossRef CAS
.
- T. Periyasamy, S. P. Asrafali, S. Muthusamy and S.-C. Kim, New J. Chem., 2016, 40, 9313–9319 RSC
.
- Z. Qian, Q. Li, L. Wang, F. Fu and X. Liu, J. Polym. Sci., 2021, 59, 2057–2068 CrossRef CAS
.
- S. Ren, X. Miao, W. Zhao, S. Zhang and W. Wang, Mater. Today Commun., 2019, 20, 100568 CrossRef CAS
.
- X.-L. Sha, L. Yuan, G. Liang and A. Gu, ACS Sustainable Chem. Eng., 2020, 8, 18696–18705 CrossRef CAS
.
- P. Thirukumaran, R. Sathiyamoorthi, A. S. Parveen and M. Sarojadevi, Polym. Compos., 2016, 37, 573–582 CrossRef CAS
.
- P. Thirukumaran, A. S. Parveen and M. Sarojadevi, ACS Sustainable Chem. Eng., 2014, 2, 2790–2801 CrossRef CAS
.
- C. Wang, C. Zhao, J. Sun, S. Huang, X. Liu and T. Endo, J. Polym. Sci., Part A: Polym. Chem., 2013, 51, 2016–2023 CrossRef CAS
.
- S.-M. Zhang, J.-Q. Zhao, Y. Liu, Y.-X. Liu and C.-M. Liu, React. Funct. Polym., 2021, 165, 104957 CrossRef CAS
.
- M. Chen, X. He, Y. Guo, J. Hu, B. Liang, K. Zeng and G. Yang, Polym. Chem., 2021, 12, 408–422 RSC
.
-
H. Ishida and P. Froimowicz, Advanced and emerging polybenzoxazine science and technology, Elsevier, 2017 Search PubMed
.
-
M. J. Frisch, G. W. Trucks, H. B. Schlegel, G. E. Scuseria, M. A. Robb, J. R. Cheeseman, J. A. Montgomery Jr., T. Vreven, K. N. Kudin and J. C. Burant, Gaussian 13, revision C 02, Gasussian, Inc., Wallingford, CT, 2004 Search PubMed
.
- L. R. Kotzebue, J. S. R. de Oliveira, J. B. da Silva, S. E. Mazzetto, H. Ishida and D. Lomonaco, ACS Sustainable Chem. Eng., 2018, 6, 5485–5494 CrossRef CAS
.
- Y. Cao, C. Chen, X. Lu, D. Xu, J. Huang and Z. Xin, Surf. Coat. Technol., 2021, 405, 126569 CrossRef CAS
.
- C. Capello, U. Fischer and K. Hungerbühler, Green Chem., 2007, 9, 927–934 RSC
.
- K. Zhang, X. Tan, Y. Wang and H. Ishida, Polymer, 2019, 168, 8–15 CrossRef CAS
.
- L. Zhang, Y. Zhu, D. Li, M. Wang, H. Chen and J. Wu, RSC Adv., 2015, 5, 96879–96887 RSC
.
- J. Liu, N. Safronava, R. E. Lyon, J. Maia and H. Ishida, Macromolecules, 2018, 51, 9982–9991 CrossRef CAS
.
- K. Zhang and H. Ishida, Polym. Chem., 2015, 6, 2541–2550 RSC
.
-
H. Ishida, in Handbook of benzoxazine resins, Elsevier, 2011, pp. 3–81 Search PubMed
.
- H. E. Kissinger, Anal. Chem., 1957, 29, 1702–1706 CrossRef CAS
.
- T. Ozawa, Polymer, 1971, 12, 150–158 CrossRef CAS
.
- C. F. Wang, Y. C. Su, S. W. Kuo, C. F. Huang, Y. C. Sheen and F. C. Chang, Angew. Chem., Int. Ed., 2006, 45, 2248–2251 CrossRef CAS PubMed
.
- D. K. Owens and R. Wendt, J. Appl. Polym. Sci., 1969, 13, 1741–1747 CrossRef CAS
.
- W. Zhao, B. Chen and K. Zhang, ACS Sustainable Chem. Eng., 2022, 10, 14783–14793 CrossRef CAS
.
- B. Jin, G. Zhang, J. Lian, Q. Zhang, X. Zhan and F. Chen, J. Mater. Chem. A, 2019, 7, 12266–12275 RSC
.
- J. A. Callow and M. E. Callow, Nat. Commun., 2011, 2, 1–10 Search PubMed
.
- V. Duhan, N. Amarnath, S. Yadav and B. Lochab, ACS Appl. Polym. Mater., 2023, 5, 2971–2982 CrossRef CAS
.
- H. Y. Low and H. Ishida, Polymer, 1999, 40, 4365–4376 CrossRef CAS
.
- J. Liu and H. Ishida, Macromolecules, 2014, 47, 5682–5690 CrossRef CAS
.
- Y. Lu, J. Liu, W. Zhao and K. Zhang, Chem. Eng. J., 2023, 457, 141232 CrossRef CAS
.
|
This journal is © The Royal Society of Chemistry 2024 |