DOI:
10.1039/D4MA90097A
(Editorial)
Mater. Adv., 2024,
5, 6686-6689
Introduction to order, disorder and ultrafast phenomena in functional solids
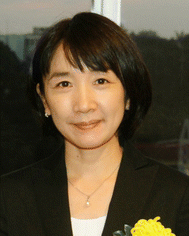
Hiroko Tokoro
| Hiroko Tokoro is a Professor of Materials Science at the University of Tsukuba. She received BS from Keio University, ME from Kyoto University, and PhD from the University of Tokyo. Her career includes working as a JSPS fellowship (2004–2007) and JST researcher (2007–2010), serving as an Assistant Professor at the University of Tokyo (2011–2013), and holding the position of Associate Professor at University of Tsukuba (2013–2018). She has received awards, including the German Innovation Award (2016) and the Yonezawa Memorial Prize of JPS (2020). Her research mainly focuses on bistability and phase transition in solid-state materials. |
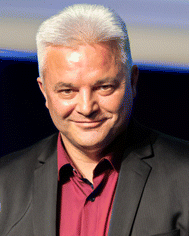
Eric Collet
| Eric Collet is Professor of Physics at the University of Rennes, elected at the Academy of Europe. He has a chair at the Institut Universitaire de France for fundamental research. He is co-directing the International Research Laboratory “Dynamical Control of Materials”, funded by CNRS, the University of Rennes and the University of Tokyo. His research topics are about functional materials, phase transitions and out-of-equilibrium dynamics. He received the 2022 Alajos Kálmán Prize of the European Crystallographic Association, the 2020 Silver Medal of CNRS and the 2017 Louis Ancel prize, condensed matter prize of the French Physical Society. |
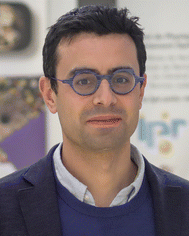
Ernest Pastor
| Dr Ernest Pastor is a CNRS Junior Professor at The Institute of Physics of Rennes (IPR). His research focuses on characterising solids for solar-energy conversion using optical, electrochemical and time-resolved X-ray methods. He is particularly interested in understanding and controlling the role of disorder in catalytic solids. Photo by Frédéric Obé. |
Disorder in solids manifests in the variable extended loss of the translational symmetry.1,2 This change in the crystallographic properties can dramatically impact the solid's functionality by altering its electric conduction, magnetic response or optical properties.3–7 Consequently, manipulating disorder via the targeted control of defect populations is central to developing new functional materials, such as those used for catalysis and magnetic memories,8–13 or in energy and thermal storage, as discussed in this collection by Kubota et al. (https://doi.org/10.1039/D3MA01162C).
Disorder is particularly important for materials that undergo photo-induced phase transformations as, often, light excitation triggers a change in the way the system is ordered.14 For example, photoexcitation of Sr3Ir2O7 or RbMnFe(CN)6 can change magnetic spin order and induce a transition from antiferromagnetic to paramagnetic, or ferromagnetic to antiferromagnetic.15–18 Similarly, in the ferroelectric solid TTF-CA, thermal or photoexcitation can induce a change in polar order and affect the ferroelectric properties.19–23
These light-active phase-change materials hold incredible technological potential as they offer the possibility to achieve transformation speeds not attainable using thermodynamical tools (i.e., heating or increasing pressure) thus opening the door to ultra-fast switches or memories. Currently, great research efforts are devoted to understanding photo-induced transformations with growing evidence highlighting the critical role that disorder plays in enabling control of the phase change.24–28 The development of tools capable of elucidating the impact of defects in the ground state and upon excitation, from microscopic to macroscopic scales, is crucial to enable further technological developments.
Defects can take many different shapes and forms. For example, in Prussian blue analogues (PBA), with structure AxM1y[M2(CN)6]n·xH2O (A = alkaline), disorder might be chemically controlled through M2(CN)6 vacancies and variable quantities of water molecules, as well as due to the presence of different interstitial cations, amongst other sources (Fig. 1).29,30 These defects can partake in the collective intermetallic charge transfer, which can be driven by external stimuli such as temperature or pressure.6,31–34
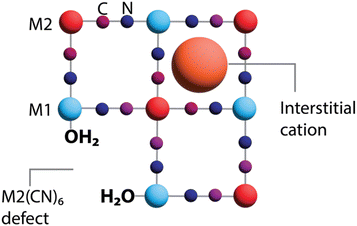 |
| Fig. 1 Two type of defects that can emerge in Prussian blue analogues (PBA) of formula AxM1y[M2(CN)6]n·xH2O (A = alkaline), and which affect the function of the material. | |
Defects can also be generated upon illumination.35–38 As shown by Hervé et al. (https://doi.org/10.1039/D3MA01072D), in the case of PBA, the excitation of the solid can induce a charge transfer at the microscopic scale, causing a local structural distortion around the metal ion known as a polaron (Fig. 2).37,39–42 These photoinduced polarons are important because they are the heart of cooperative photo-active phenomena that might enable the magnification of external stimuli towards a macroscopic charge-transfer phase transition.28,43–45
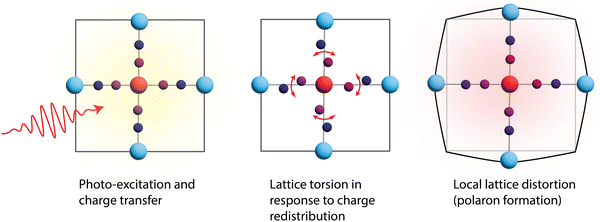 |
| Fig. 2 Formation of a photo-induced polaron in a PBA. The local structural distortion can be the precursor of cooperative phenomena in the solid that magnifies the effect of the external perturbation. | |
Similarly, in bistable molecular solids, such as spin crossover materials, the constituting molecules may be thermally or optically converted from one electronic state to another in a collective way. However, fluctuations in the balance between elastic interactions and entropy can lead to the long-range ordering or disordering of molecules in different spin states, causing stepwise thermal conversion to emerge, and therefore multi-stable phases.46–49 For many spin-transition materials, stepwise transitions are associated with long-range ordering of molecules in different spin states and are therefore symmetry-breaking, which also allows for a magneto-electric effect.50 The case of order–disorder of molecules in different spin states, without symmetry-breaking, is discussed in this collection by Ruzzi et al. (https://doi.org/10.1039/D3MA01057K).
Optical methods, relying on recent advances in laser and detection tools, offer a great platform to characterise the role of disorder and expose the complex interplay of phenomena that leads to phase metastability. The probes can provide information on changes in local and/or long-range order. For example, spectroscopic methods can be used to track the fingerprints of ground-state defects and impulsive spectroscopy can resolve, with unprecedented time resolution, how the electronic and structural systems of the solid couple and become disordered upon excitation.26,51–53 As shown by Sugisawa et al. (https://doi.org/10.1039/D3MA00317E), methods like time-resolved reflectance and second-harmonic generation can be used to reveal how photo-excitation modulates the polarisation structure of hydrogen-bonded ferroelectric materials relevant for technological applications.
Importantly, it is now possible to perform such optical experiments under in-situ-like conditions thus characterising the response of the solid under technologically relevant temperature, pressure and excitation regimes.54 For example, in this collection, Dronova et al. use variable-temperature infrared spectroscopy to learn about the interactions between cations and the cyanide-bridge networks in PBA (https://doi.org/10.1039/D4MA00064A).
Similarly, structural and coherent imaging methods, powered by unprecedented developments in large-scale facilities, offer the possibility to visualise the formation of defects in real time.55,56 In this regard, the measurement of weak diffuse signals that track random displacements in the solid's structure is exposing, not only that defects are important, but that time-dependent, spatial interaction between defects can dictate the phase of the system upon irradiation.57 The use of coherent imaging to capture polaronic states is discussed in this collection by Sarkar et al. (https://doi.org/10.1039/D4MA00154K). Further development of experimental and theoretical methods to understand and analyse such signals promises to reveal unexpected physicochemical phenomena that could be useful to manipulate solids on demand. However, due to the multiscale nature of photoinduced processes, theoretical developments are very challenging.
In this themed collection we highlight works focusing on the implementation of advanced experimental tools to characterize defects and the development of robust theoretical frameworks to understand the role of disorder. Further advances in these areas are needed to push the boundaries of optical control of materials. However, the recent developments in laser techniques, large-scale facilities and computational capabilities offer an exciting outlook for the characterisation and control of disorder in functional solids.
As guest editors, we would like to thank all the authors that have contributed as well as the RSC editorial team for their help in coordinating the effort. We hope the research highlighted in this collection will be useful for the multiple communities seeking to understand and control disorder in solids in order to instil new functionality that powers the technologies of the future.
References
-
A. M. S. W. Hayes, Defects and Defect Processes in Nonmetallic Solids, Dover Publications, 2004 Search PubMed.
-
C. R. A. Catlow, in Defects and Disorder in Crystalline and Amorphous Solids, ed. C. R. A. Catlow, Springer Netherlands, Dordrecht, 1994, pp. 1–23 Search PubMed.
- E. Pastor, M. Sachs, S. Selim, J. R. Durrant, A. A. Bakulin and A. Walsh, Nat. Rev. Mater., 2022, 7, 503–521 CrossRef CAS.
- E. Pastor, L. Montañés, A. Gutiérrez-Blanco, F. S. Hegner, C. A. Mesa, N. López and S. Giménez, Nanoscale, 2022, 14, 15596–15606 RSC.
- A. K. Cheetham, T. D. Bennett, F.-X. Coudert and A. L. Goodwin, Dalton Trans., 2016, 45, 4113–4126 RSC.
- H. Tokoro and S. Ohkoshi, Bull. Chem. Soc. Jpn., 2015, 88, 227–239 CrossRef.
- E. Janod, J. Tranchant, B. Corraze, M. Querré, P. Stoliar, M. Rozenberg, T. Cren, D. Roditchev, V. T. Phuoc, M.-P. Besland and L. Cario, Adv. Funct. Mater., 2015, 25, 6287–6305 CrossRef CAS.
- S. Kim, J. S. Park and A. Walsh, ACS Energy Lett., 2018, 3, 496–500 CrossRef CAS.
- S. Selim, E. Pastor, M. García-Tecedor, M. R. Morris, L. Francàs, M. Sachs, B. Moss, S. Corby, C. A. Mesa, S. Gimenez, A. Kafizas, A. A. Bakulin and J. R. Durrant, J. Am. Chem. Soc., 2019, 141, 18791–18798 CrossRef CAS PubMed.
- Z. Meng, E. Pastor, S. Selim, H. Ning, M. Maimaris, A. Kafizas, J. R. Durrant and A. A. Bakulin, J. Am. Chem. Soc., 2023, 145, 17700–17709 CrossRef CAS.
- S. Bai, N. Zhang, C. Gao and Y. Xiong, Nano Energy, 2018, 53, 296–336 CrossRef CAS.
- H. Tokoro, M. Yoshikiyo, K. Imoto, A. Namai, T. Nasu, K. Nakagawa, N. Ozaki, F. Hakoe, K. Tanaka, K. Chiba, R. Makiura, K. Prassides and S. Ohkoshi, Nat. Commun., 2015, 6, 7037 CrossRef CAS PubMed.
- S. Ohkoshi, K. Nakagawa, M. Yoshikiyo, A. Namai, K. Imoto, Y. Nagane, F. Jia, O. Stefanczyk, H. Tokoro, J. Wang, T. Sugahara, K. Chiba, K. Motodohi, K. Isogai, K. Nishioka, T. Momiki and R. Hatano, Nat. Commun., 2023, 14, 8466 CrossRef CAS PubMed.
- S. Wall, S. Yang, L. Vidas, M. Chollet, J. M. Glownia, M. Kozina, T. Katayama, T. Henighan, M. Jiang, T. A. Miller, D. A. Reis, L. A. Boatner, O. Delaire and M. Trigo, Science, 2018, 362, 572–576 CrossRef CAS PubMed.
- E. Pastor, D. Moreno-Mencía, M. Monti, A. S. Johnson, N. Fleischmann, C. Wang, Y. Shi, X. Liu, D. G. Mazzone, M. P. M. Dean and S. Wall, Phys. Rev. B, 2022, 105, 064409 CrossRef CAS.
- G. Azzolina, H. Tokoro, K. Imoto, M. Yoshikiyo, S. Ohkoshi and E. Collet, Angew. Chem., Int. Ed., 2021, 60, 23267–23273 CrossRef CAS PubMed.
- D. G. Mazzone, D. Meyers, Y. Cao, J. G. Vale, C. D. Dashwood, Y. Shi, A. J. A. James, N. J. Robinson, J. Lin, V. Thampy, Y. Tanaka, A. S. Johnson, H. Miao, R. Wang, T. A. Assefa, J. Kim, D. Casa, R. Mankowsky, D. Zhu, R. Alonso-Mori, S. Song, H. Yavas, T. Katayama, M. Yabashi, Y. Kubota, S. Owada, J. Liu, J. Yang, R. M. Konik, I. K. Robinson, J. P. Hill, D. F. McMorrow, M. Först, S. Wall, X. Liu and M. P. M. Dean, Proc. Natl. Acad. Sci. U. S. A., 2021, 118, e2103696118 CrossRef CAS.
- H. Tokoro, T. Matsuda, T. Nuida, Y. Moritomo, K. Ohoyama, E. D. L. Dangui, K. Boukheddaden and S. Ohkoshi, Chem. Mater., 2008, 20, 423–428 CrossRef CAS.
- E. Collet, Science, 2003, 300, 612–615 CrossRef CAS.
- M. H. Lemée-Cailleau, M. Le Cointe, H. Cailleau, T. Luty, F. Moussa, J. Roos, D. Brinkmann, B. Toudic, C. Ayache and N. Karl, Phys. Rev. Lett., 1997, 79, 1690–1693 CrossRef.
- S. Koshihara, Y. Tokura, T. Mitani, G. Saito and T. Koda, Phys. Rev. B: Condens. Matter Mater. Phys., 1990, 42, 6853–6856 CrossRef CAS.
- S. Koshihara, Y. Takahashi, H. Sakai, Y. Tokura and T. Luty, J. Phys. Chem. B, 1999, 103, 2592–2600 CrossRef CAS.
- S.-Q. Su, S.-Q. Wu, S. Kanegawa, K. Yamamoto and O. Sato, Chem. Sci., 2023, 14, 10631–10643 RSC.
- A. Zong, A. Kogar, Y.-Q. Bie, T. Rohwer, C. Lee, E. Baldini, E. Ergeçen, M. B. Yilmaz, B. Freelon, E. J. Sie, H. Zhou, J. Straquadine, P. Walmsley, P. E. Dolgirev, A. V. Rozhkov, I. R. Fisher, P. Jarillo-Herrero, B. V. Fine and N. Gedik, Nat. Phys., 2019, 15, 27–31 Search PubMed.
- G. A. de la Peña Muñoz, A. A. Correa, S. Yang, O. Delaire, Y. Huang, A. S. Johnson, T. Katayama, V. Krapivin, E. Pastor, D. A. Reis, S. Teitelbaum, L. Vidas, S. Wall and M. Trigo, Nat. Phys., 2023, 19, 1489–1494 Search PubMed.
- A. S. Johnson, D. Moreno-Mencía, E. B. Amuah, M. Menghini, J.-P. Locquet, C. Giannetti, E. Pastor and S. E. Wall, Phys. Rev. Lett., 2022, 129, 255701 CrossRef CAS.
- M. Fechner, M. Först, G. Orenstein, V. Krapivin, A. S. Disa, M. Buzzi, A. von Hoegen, G. de la Pena, Q. L. Nguyen, R. Mankowsky, M. Sander, H. Lemke, Y. Deng, M. Trigo and A. Cavalleri, Nat. Mater., 2024, 23, 363–368 CrossRef CAS.
- M. Hervé, G. Privault, E. Trzop, S. Akagi, Y. Watier, S. Zerdane, I. Chaban, R. G. Torres Ramírez, C. Mariette, A. Volte, M. Cammarata, M. Levantino, H. Tokoro, S. Ohkoshi and E. Collet, Nat. Commun., 2024, 15, 267 CrossRef PubMed.
- H. Tokoro and S. Ohkoshi, Dalton Trans., 2011, 40, 6825–6833 RSC.
- H. L. B. Boström and W. R. Brant, J. Mater. Chem. C, 2022, 10, 13690–13699 RSC.
- S. Ohkoshi and H. Tokoro, Acc. Chem. Res., 2012, 45, 1749–1758 CrossRef CAS PubMed.
- J. Zimara, H. Stevens, R. Oswald, S. Demeshko, S. Dechert, R. A. Mata, F. Meyer and D. Schwarzer, Inorg. Chem., 2021, 60, 449–459 CrossRef CAS PubMed.
- L. Trinh, S. Zerdane, S. Mazérat, N. Dia, D. Dragoe, C. Herrero, E. Rivière, L. Catala, M. Cammarata, E. Collet and T. Mallah, Inorg. Chem., 2020, 59, 13153–13161 CrossRef CAS PubMed.
- C. R. Gros, M. K. Peprah, B. D. Hosterman, T. V. Brinzari, P. A. Quintero, M. Sendova, M. W. Meisel and D. R. Talham, J. Am. Chem. Soc., 2014, 136, 9846–9849 CrossRef CAS PubMed.
- L. M. Carneiro, S. K. Cushing, C. Liu, Y. Su, P. Yang, A. P. Alivisatos and S. R. Leone, Nat. Mater., 2017, 16, 819–825 CrossRef CAS PubMed.
- E. Pastor, J.-S. Park, L. Steier, S. Kim, M. Grätzel, J. R. Durrant, A. Walsh and A. A. Bakulin, Nat. Commun., 2019, 10, 3962 CrossRef PubMed.
- S. Biswas, S. Wallentine, S. Bandaranayake and L. R. Baker, J. Chem. Phys., 2019, 151, 104701 CrossRef PubMed.
- I. J. Porter, S. K. Cushing, L. M. Carneiro, A. Lee, J. C. Ondry, J. C. Dahl, H.-T. Chang, A. P. Alivisatos and S. R. Leone, J. Phys. Chem. Lett., 2018, 9, 4120–4124 CrossRef CAS.
- J. S. P. Cresi, L. D. Mario, D. Catone, F. Martelli, A. Paladini, S. Turchini, S. D’Addato, P. Luches and P. O’Keeffe, J. Phys. Chem. Lett., 2020, 11, 5686–5691 CrossRef.
- C. Franchini, M. Reticcioli, M. Setvin and U. Diebold, Nat. Rev. Mater., 2021, 6, 560–586 CrossRef CAS.
- J. Wiktor and A. Pasquarello, ACS Appl. Mater. Interfaces, 2019, 11, 18423–18426 CrossRef CAS PubMed.
- Y.-J. Kim, J. L. Mendes, J. M. Michelsen, H. J. Shin, N. Lee, Y. J. Choi and S. K. Cushing, Sci. Adv., 2024, 10, eadk4282 CrossRef CAS PubMed.
- G. Azzolina, R. Bertoni, C. Ecolivet, H. Tokoro, S. Ohkoshi and E. Collet, Phys. Rev. B, 2020, 102, 134104 CrossRef CAS.
- R. Bertoni, M. Lorenc, H. Cailleau, A. Tissot, J. Laisney, M.-L. Boillot, L. Stoleriu, A. Stancu, C. Enachescu and E. Collet, Nat. Mater., 2016, 15, 606–610 CrossRef CAS PubMed.
- S. Zerdane, M. Hervé, S. Mazerat, L. Catala, R. Alonso-Mori, J. M. Glownia, S. Song, M. Levantino, T. Mallah, M. Cammarata and E. Collet, Faraday Discuss., 2022, 237, 224–236 RSC.
- G. Molnár, S. Rat, L. Salmon, W. Nicolazzi and A. Bousseksou, Adv. Mater., 2018, 30, 1703862 CrossRef PubMed.
- A. Volte, C. Mariette, R. Bertoni, M. Cammarata, X. Dong, E. Trzop, H. Cailleau, E. Collet, M. Levantino, M. Wulff, J. Kubicki, F.-L. Yang, M.-L. Boillot, B. Corraze, L. Stoleriu, C. Enachescu and M. Lorenc, Commun. Phys., 2022, 5, 168 CrossRef CAS.
- D. Chernyshov, H.-B. Bürgi, M. Hostettler and K. W. Törnroos, Phys. Rev. B: Condens. Matter Mater. Phys., 2004, 70, 094116 CrossRef.
- G. Azzolina, R. Bertoni and E. Collet, J. Appl. Phys., 2021, 129, 085106 CrossRef CAS.
- V. B. Jakobsen, E. Trzop, E. Dobbelaar, L. C. Gavin, S. Chikara, X. Ding, M. Lee, K. Esien, H. Müller-Bunz, S. Felton, E. Collet, M. A. Carpenter, V. S. Zapf and G. G. Morgan, J. Am. Chem. Soc., 2022, 144, 195–211 CrossRef CAS PubMed.
- E. Pastor, Z. Lian, L. Xia, D. Ecija, J. R. Galán-Mascarós, S. Barja, S. Giménez, J. Arbiol, N. López and F. P. García de Arquer, Nat. Rev. Chem., 2024, 8, 159–178 CrossRef PubMed.
- S. Wall, D. Wegkamp, L. Foglia, K. Appavoo, J. Nag, R. F. Haglund, J. Stähler and M. Wolf, Nat. Commun., 2012, 3, 721 CrossRef CAS PubMed.
- K. Barlow and J. O. Johansson, Phys. Chem. Chem. Phys., 2021, 23, 8118–8131 RSC.
- G. Privault, G. Huitric, M. Hervé, E. Trzop, J. Tranchant, B. Corraze, Z. Khaldi, L. Cario, E. Janod, J.-C. Ameline, N. Godin and R. Bertoni, Eur. Phys. J.: Spec. Top., 2023, 232, 2195–2203 CAS.
- A. S. Johnson, D. Perez-Salinas, K. M. Siddiqui, S. Kim, S. Choi, K. Volckaert, P. E. Majchrzak, S. Ulstrup, N. Agarwal, K. Hallman, R. F. Haglund, C. M. Günther, B. Pfau, S. Eisebitt, D. Backes, F. Maccherozzi, A. Fitzpatrick, S. S. Dhesi, P. Gargiani, M. Valvidares, N. Artrith, F. de Groot, H. Choi, D. Jang, A. Katoch, S. Kwon, S. H. Park, H. Kim and S. E. Wall, Nat. Phys., 2023, 19, 215–220 Search PubMed.
- D. A. Keen and A. L. Goodwin, Nature, 2015, 521, 303–309 CrossRef CAS PubMed.
- A. S. Johnson, E. Pastor, S. Batlle-Porro, H. Benzidi, T. Katayama, G. A. de la Peña Muñoz, V. Krapivin, S. Kim, N. López, M. Trigo and S. E. Wall, Nat. Phys., 2024, 20, 970–975 Search PubMed.
|
This journal is © The Royal Society of Chemistry 2024 |
Click here to see how this site uses Cookies. View our privacy policy here.