DOI:
10.1039/D3MA01162C
(Paper)
Mater. Adv., 2024,
5, 3832-3837
Synthesis of heat storage ceramic λ-Ti3O5 using titanium chloride as the starting material†
Received
22nd December 2023
, Accepted 11th March 2024
First published on 13th March 2024
Abstract
This study reports a simple method for synthesizing λ-Ti3O5 through hydrogen reduction calcination using titanium chloride as the starting material. Upon applying 300 MPa pressure, λ-Ti3O5 transitions to β-Ti3O5 as a pressure-induced phase transformation. The β-Ti3O5 produced under pressure reverts to λ-Ti3O5 at 462 K upon heating, accumulating 7.78 kJ mol−1 of heat energy. An investigation of the influence of the crystalline size on the threshold pressure required for the phase transition and the values of transition enthalpy of the transition between λ-Ti3O5 and β-Ti3O5 reveals that larger crystalline sizes correlate with lower threshold pressures and higher transition enthalpies. Understanding the interplay between the crystalline size of λ-Ti3O5 and its heat storage properties is crucial for designing phase transition materials tailored to specific heat storage requirements. This research paves the way for the development of more efficient heat storage materials, potentially impacting various industrial applications and energy conservation methods.
Introduction
In recent years, the intensifying issues of climate change and environmental deterioration have emerged as prominent global concerns. Advancing technological innovation is imperative to safeguard the Earth and ensure its preservation for future generations.1–8 To this end, energy reuse technology—a critical component of energy conservation—has garnered special attention, especially the recovery of waste heat energy. Currently, approximately 40% of the energy obtained from sources such as oil, gas, and coal is emitted as waste heat into the atmosphere. Note that about 80% of this waste heat is <200 °C (473 K).9 The development of high-performance thermal storage materials, capable of capturing and transforming sub-200 °C waste heat into usable thermal energy, could significantly mitigate these challenges. Established thermal storage materials include sensible heat storage materials, such as bricks and concrete, and latent heat storage materials, such as water, paraffin, and polyethylene glycol, which utilize solid–liquid phase transitions.2,10–17 Generally, thermal storage materials release stored heat energy gradually over time. However, materials that can store heat for extended periods and release it on demand are promising for various practical applications.
Recently, we developed a pressure-responsive thermal storage material that discharges stored heat when subjected to external pressure.18,19 This material—λ-phase trititanium pentoxide (λ-Ti3O5)—is a metastable phase present only in nanoscale materials and not in bulk forms.18,20 Furthermore, λ-Ti3O5 undergoes a reversible phase transition with beta-phase trititanium pentoxide (β-Ti3O5) under external stimuli such as light, pressure, or temperature.18–21 Moreover, λ-Ti3O5 is an environmentally friendly material purely composed of titanium and oxygen.18–22 To effectively use this material, scalable synthesis methods for mass production and strategies for enhancing thermal storage properties are crucial. Accordingly, various synthesis techniques for λ-Ti3O5 have been explored. One method involves nanocrystalline λ-Ti3O5 synthesis through calcination reduction of TiO2 nanoparticles in a hydrogen atmosphere.18,20–25 Another approach uses titanium chloride as the Ti source, employing the sol–gel process to produce λ-Ti3O5 nanoparticles within a silica matrix.20,26–28 Additionally, carbon thermal reduction,29–32 electrochemical methods,33,34 and pulse laser deposition (PLD)35,36 are other notable synthesis methods.
In this research, we detail a method for synthesizing λ-Ti3O5 through hydrogen reduction calcination of a precursor, utilizing titanium(IV) chloride as the starting material. This technique enables the efficient production of λ-Ti3O5 without employing matrices such as silica. At a pressure of 300 MPa, λ-Ti3O5 undergoes a pressure-induced phase transformation to β-Ti3O5. During the reverse transition from β-Ti3O5 to λ-Ti3O5 upon heating, the material stores 7.78 kJ mol−1 of heat energy at 462 K. We investigated the relationship between the crystalline size of λ-Ti3O5 and the threshold pressure for pressure-induced phase transition and the transition enthalpy values between λ-Ti3O5 and β-Ti3O5, demonstrating the correlation between crystalline size and heat storage capabilities.
Experimental
Material synthesis
A mixture solution of 420 mL of H2O, 1.40 mL of TiCl4 (Wako), and 9.6 mL of NH3 (25 wt%, Wako, ammonia solution) was prepared in a 1-L container. The solution was stirred for 20 h at 50 °C in an oil bath to produce Ti(OH)4 precipitates. These precipitates were separated via centrifugation, washed with ethanol, and dried at 60 °C for 24 h (Fig. 1) (eqn (1)). | TiCl4 + 4H2O + 4NH3 → Ti(OH)4 + 4NH4Cl | (1) |
 |
| Fig. 1 Synthesis of λ-Ti3O5 using titanium(IV) chloride as the starting material. Schematic of the synthesis used to prepare the precursor and the calcination procedure. | |
The resulting precipitate underwent calcination under a hydrogen flow rate of 0.5 dm3 min−1 at 1100 °C for 5 h, forming a black powder sample (eqn (2)).
| 3Ti(OH)4 + H2 → Ti3O5 + 7H2O | (2) |
Measurements
Elemental analysis was conducted via X-ray fluorescence (XRF) spectroscopy using the Rigaku ZSX Primus IV. The morphology of the synthesized samples was examined using scanning electron microscopy (SEM) (JSM-7500FA), manufactured by JEOL. Powder X-ray diffraction (PXRD) patterns were obtained using a Rigaku Ultima IV instrument with Cu–Kα radiation (λ = 1.5418 Å). Rietveld analyses of the PXRD patterns were performed using Rigaku PDXL software. The heat absorption during the phase transition from β-Ti3O5 to λ-Ti3O5 was measured using a Rigaku differential scanning calorimetry (DSC) Thermo plus EVO2, with liquid nitrogen (N2) as the cooling agent. Magnetic measurements were conducted using a Quantum Design MPMS superconducting quantum interference device magnetometer.
Results and discussion
Material
According to the XRF measurements, the composition of the synthesized black powder was Ti3.00(3)O5.00(3) (calculated: Ti: 64.22; O: 35.78 wt%; Observed: Ti: 64.53; O: 35.47 wt%). Fig. 2 presents an SEM image of the sample. The sample displayed a flake-like morphology with an average width of 299 ± 54 nm (Fig. S1, ESI†). The PXRD and Rietveld analysis confirmed the samples as monoclinic λ-Ti3O5 with the space group C2/m (lattice parameters: a = 9.8332(2) Å, b = 3.78568(7) Å, c = 9.9688(2) Å, β = 91.2589(14)°), and the crystalline size was estimated to be 57 ± 0.3 nm (Fig. 3 and Fig. S2 and Table S1, ESI†). In other words, this sample was an aggregate with a flake-like structure, approximately 300 nm in width, composed of crystallites of about 57 nm.
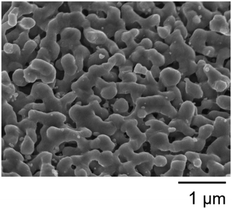 |
| Fig. 2 SEM image of the obtained λ-Ti3O5 particles. | |
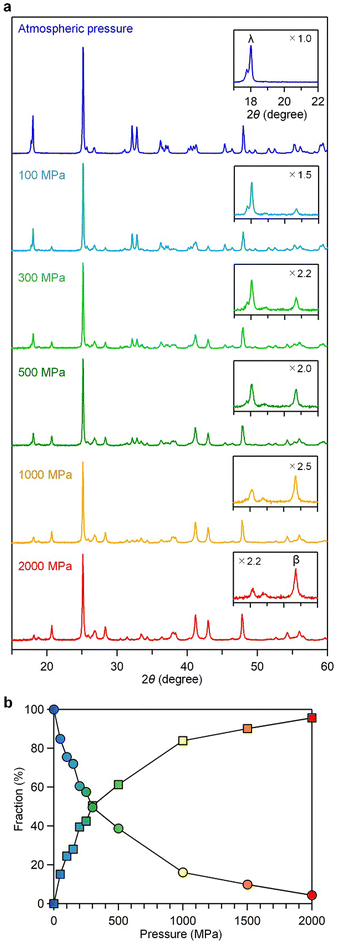 |
| Fig. 3 (a) PXRD patterns without applying pressure (blue), and after releasing pressures of 100 MPa (light blue), 300 MPa (light green), 500 MPa (green), 1000 MPa (orange), and 2000 MPa (red). (b) Phase fraction of λ-Ti3O5 (circle) and β-Ti3O5 (square) as a function of applied pressure. | |
In the magnetic susceptibility (χ) versus temperature (T) curve, λ-Ti3O5 displayed a χ-value of approximately 2 × 10−4 emu per Ti atom over the entire temperature range (Fig. S3, ESI†). This aligns with prior findings identifying λ-Ti3O5 as a Pauli paramagnet, stable across the temperature spectrum. The gradual decrease in χ value below 150 K is attributed to the spin–orbit interaction of Ti3+ ions. The abrupt increase in magnetic susceptibility below 40 K is possibly because of Curie paramagnetism resulting from minor defects in the sample.20
Pressure-induced phase transition
We investigated the effects of pressure on the crystal structure. The pellets subjected to varying uniaxial pressures were analyzed, and their PXRD patterns were measured. As demonstrated in Fig. 3 and Fig. S4–S13 and Tables S2–S11 (ESI†), increasing pressure led to a decrease in the λ-Ti3O5 fraction and an increase in β-Ti3O5. The threshold pressure value (Pth) for converting 50% of λ-Ti3O5 to β-Ti3O5 was approximately 300 MPa (Fig. 3b). The crystal structure of β-Ti3O5 formed after a 2000 MPa pressure was monoclinic with lattice parameters a = 9.7540(6) Å, b = 3.7993(2) Å, c = 9.4428(6) Å, β = 91.528(3)° (space group C2/m) (Fig. S13 and Table S11, ESI†).
Accumulated heat energy
To investigate the heat energy accumulated during the phase transition from β-Ti3O5 to λ-Ti3O5, DSC measurements were conducted using β-Ti3O5 samples after applying a pressure of 300 MPa and releasing the pressure (Fig. 4). The transition from β-Ti3O5 to λ-Ti3O5 occurred at 462 K, and the observed transition enthalpy (ΔHtrans) during this temperature-induced phase transition was 7.78 ± 0.26 kJ mol−1 (Fig. 4, i). Subsequent cooling of the sample during DSC measurements revealed no peaks (Fig. 4, ii), indicating that the heat energy accumulated during the β-Ti3O5 to λ-Ti3O5 phase transition was not released upon cooling. Moreover, once transformed into λ-Ti3O5, the sample exhibited no peaks in repeated heating and cooling cycles (Fig. 4, iii–vi), highlighting the stability of λ-Ti3O5.
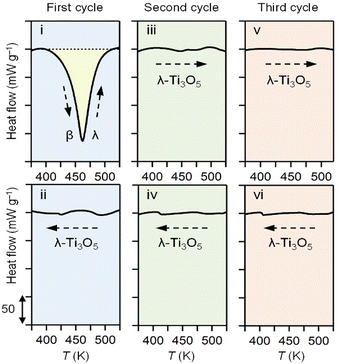 |
| Fig. 4 DSC measurements for pressure-produced β-Ti3O5 by heating (i) and cooling (ii). Second cycle of heating (iii) and cooling (iv), and third cycle of heating (v) and cooling (vi). | |
Thermodynamic interpretation of the threshold pressure
The threshold pressure for the phase transition from λ-Ti3O5 to β-Ti3O5 was analyzed using the Slichter and Drickamer mean-field thermodynamic model (SD model).18,20,37 In this model, the Gibbs free energy (G) is expressed as
G = xΔH + γx(1 − x) + T{R[x ln x + (1 − x)ln(1 − x)] − xΔS}, |
where x represents the fraction of charge delocalization units corresponding to λ-Ti3O5; ΔH denotes the transition enthalpy, and ΔS indicates the transition entropy. γ represents the interaction parameter between the λ-Ti3O5 and β-Ti3O5 phases, and R denotes the gas constant. The origin of the energy is set to be the Gibbs free energy of β-Ti3O5 (x = 0). Using the experimentally observed ΔHtrans value (7.78 kJ mol−1) and ΔStrans value (21.0 J K−1 mol−1) from the DSC measurements, the Gibbs free energy curve for the bistable state was calculated at 300 K, as depicted in Fig. 5a (S6, ESI†). This equilibrium state reveals an energy barrier between λ-Ti3O5 and β-Ti3O5, suggesting that λ-Ti3O5, synthesized via high-temperature calcination and cooled to room temperature, does not spontaneously convert to β-Ti3O5. Conversely, applying pressure at 300 K reduces this energy barrier between λ-Ti3O5 and β-Ti3O5 dec (Fig. 5b), which vanishes at 300 MPa. The pressure at which this energy barrier disappears is identified as the theoretical threshold pressure.
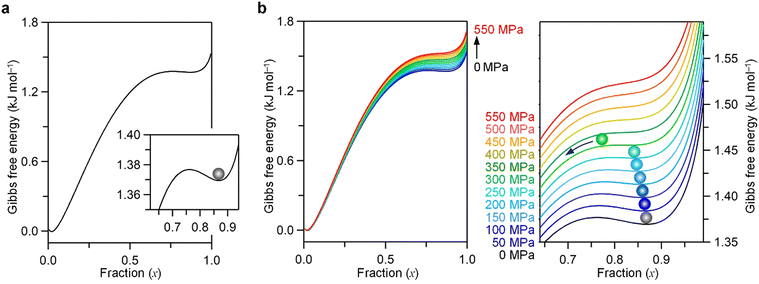 |
| Fig. 5 (a) Gibbs free energy versus fraction curve at 300 K for pressure of 0. Ball indicates the thermal population. λ-Ti3O5 cannot transit to β-Ti3O5 since the energy barrier exists. (b) Pressure dependence of Gibbs free energy versus fraction curves. λ-Ti3O5 transits to β-Ti3O5 by applying pressure because the energy barrier disappears. | |
Relationship between crystalline size, ΔHtrans and Pth
Subsequently, we investigated the relationship between crystalline size, ΔHtrans and Pth, wherein crystalline size was estimated as 57 nm with a spherical shape, ΔHtrans is obtained as 7.78 kJ mol−1, and Pth was experimentally observed at 300 MPa. In previous reports, various values of Pth with different crystalline sizes have been reported. For instance, the Pth for flake-shaped λ-Ti3O5 (crystal size: 25 nm, cubic shape) was 400 MPa,20 whereas the Pth for stripe-shaped λ-Ti3O5 (crystal size: 200 × 30 nm, rectangular prism shape) was 60 MPa.18 The value of Pth for block-shaped λ-Ti3O5 (crystal size: 400 nm, cubic shape) was 7 MPa.24 Furthermore, λ-Ti3O5 synthesized using a block copolymer (crystal size: 54 nm, spherical shape, S5, ESI†) exhibited a Pth of 300 MPa.23 The relationship between the Pth values and the crystalline size (crystalline volume) of λ-Ti3O5 is plotted in Fig. 6a, exhibiting a clear size dependency of Pth, i.e., Pth decreases as the crystalline size increases.
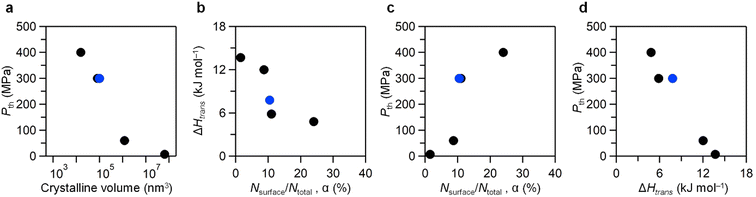 |
| Fig. 6 (a) Pthversus crystalline volume plot, (b) ΔHtransversus Nsurface/Ntotal plot, (c) Pthversus Nsurface/Ntotal plot, and (d) Pthversus ΔHtrans plot. Black circles indicate previously reported data,18,20,23,24 whereas blue circles indicate data from the present study. | |
This trend led us to consider that the Pth diminishes with increasing crystalline size. As the crystalline size decreases, the ratio of surface atoms to total atoms in the crystal (α = Nsurface/Ntotal) increases, enhancing the significance of surface energy in the Gibbs free energy of the crystal. Fig. 6b illustrates the inverse relationship between ΔHtrans and α: a smaller α corresponds to a larger ΔHtrans, and vice versa. Similarly, Fig. 6c demonstrates the direct relationship between Pth and α: a larger α results in a higher Pth. These observations suggest that as the crystalline size reduces, α increases, leading to a heightened surface energy contribution to the Gibbs free energy, consequently reducing ΔHtrans. A decrease in ΔHtrans consequently correlates with an increase in Pth (Fig. 6d). Employing the Slichter and Drickamer model to manipulate ΔH values, we observed that a smaller ΔH aligns with a higher Pth, whereas a larger ΔH associates with a lower Pth (Fig. S15, ESI†). Surface effects influence not only enthalpy but also entropy. Fig. S16 (ESI†) shows the ΔS versus ΔHtrans plot. As ΔHtrans increases, ΔS also increases. Fig. S17 (ESI†) shows the Pthversus ΔS plot. The influence of ΔS on Pth suggests a similar trend to that of ΔHtrans.
Conclusions
In this research, we introduced a simple synthesis technique for producing λ-Ti3O5 without the use of matrices such as silica. This method involves hydrogen reduction calcination of a precursor derived from titanium(IV) chloride. The resultant λ-Ti3O5 had a crystalline size of ∼57 nm, and the threshold pressure required for its phase transition from λ-Ti3O5 to β-Ti3O5 was ca. 300 MPa. The transition from β-Ti3O5 to λ-Ti3O5 occurred at 462 K, leading to the accumulation of 7.78 kJ mol−1 of heat energy.
The examination of the relationship between crystalline size and the threshold pressure and transition enthalpy values between λ-Ti3O5 and β-Ti3O5 demonstrated that a reduction in crystalline size and an increase in the proportion of surface atoms intensified the influence of surface energy on the Gibbs free energy. This decreases the transition enthalpy and consequently increases the threshold pressure. Understanding the relationship between the crystalline size and heat storage properties is essential for developing effective heat storage materials in contemporary society.
The synthesis method utilizing titanium(IV) chloride as the starting material provides an economical and efficient route to produce λ-Ti3O5, suitable for large-scale manufacturing. Given the broad availability of metal chlorides for various metals, this approach is a viable candidate for preparing metal-substituted λ-Ti3O5. Although certain metal-substituted λ-Ti3O5 has been previously reported,38–45 those methods typically involve calcination or melting of titanium oxide particles with different metals. The synthesis technique presented in this study offers potential for homogeneous doping using diverse metal chlorides as starting materials to prepare the precursor, enabling greater versatility in the synthesis of metal-substituted λ-Ti3O5.
Author contributions
T. K. and A. F. F. conducted sample synthesis, characterization, and data analysis and contributed to the preparation of the manuscript. R. S. and A. F. performed sample synthesis, characterization, and DSC measurements. F. J. contributed to sample synthesis and characterization. S. O. contributed to data analysis and coordinated this study. H. T. designed and coordinated the study, contributed to all measurements, performed calculations, and wrote the manuscript. All authors participated in the discussion and editing of the manuscript.
Conflicts of interest
The authors declare no competing financial interests.
Acknowledgements
This research was supported in part by the Japan Science and Technology FOREST Program (JPMJFR213Q), Japan Society for the Promotion of Science Grant-in-Aid for Scientific Research (B) (22H02046), Grant-in-Aid for Scientific Research (A) (20H00369), JST Advanced Technologies for Carbon-Neutral (JPMJAN23A2), and Yazaki Memorial Foundation for Science and Technology. We are grateful to Prof. Asuka Namai for her technical support. We acknowledge the support of the Cryogenic Research Center and Center for Nano Lithography & Analysis at The University of Tokyo, which are supported by MEXT.
References
- D. B. Gingerich and M. S. Mauter, Quantity, Quality, and Availability of Waste Heat from United States Thermal Power Generation, Environ. Sci. Technol., 2015, 49, 8297–8306 CrossRef CAS PubMed.
- I. Gur, K. Sawyer and R. Prasher, Searching for a Better Thermal Battery, Science, 2012, 335, 1454–1455 CrossRef PubMed.
- E. Cartlidge, Saving for a rainy day, Science, 2011, 334, 922–924 CrossRef PubMed.
- D. Lindley, The energy should always work twice, Nature, 2009, 458, 138–141 CrossRef CAS PubMed.
- A. Sharma, V. V. Tyagi, C. R. Chen and D. Buddhi, Review on thermal energy storage with phase change materials and applications, Renewable Sustainable Energy Rev., 2009, 13, 318–345 CrossRef CAS.
- C. W. King, A. S. Holman and M. E. Webber, Thirst for energy, Nat. Geosci., 2008, 1, 283–286 CrossRef CAS.
- D. Butler, Solar power: California's latest gold rush, Nature, 2007, 450, 768–769 CrossRef CAS PubMed.
- G. W. Crabtree and N. S. Lewis, Solar energy conversion, Phys. Today, 2007, 60, 37–42 CrossRef CAS.
-
A. Kondo, NEDO, presented in part the 10th German-Japanese Environment and Energy Dialogue Forum, Tokyo, 2019 https://www.nedo.go.jp/content/100899759.pdf.
- Q. Cao and P. Liu, Hyperbranched polyurethane as novel solid-solid phase change material for thermal energy storage, Eur. Polym. J., 2006, 42, 2931–2939 CrossRef CAS.
- K. S. Al-Jabri, A. W. Hago, A. S. Al-Nuaimi and A. H. Al-Saidy, Concrete blocks for thermal insulation in hot climate, Cem. Concr. Res., 2005, 35, 1472–1479 CrossRef CAS.
- M. M. Farid, A. M. Khudhair, S. A. K. Razack and S. Al-Hallaj, A review on phase change energy storage: materials and applications, Energy Convers. Manage., 2004, 45, 1597–1615 CrossRef CAS.
- W. Li, D. Zhang, T. Zhang, T. Wang, D. Ruan, D. Xing and H. Li, Study of solid–solid phase change of (n-CnH2n+1NH3)2MCl4 for thermal energy storage, Thermochim. Acta, 1999, 326, 183–186 CrossRef CAS.
- M. Barrio, D. O. López, J. L. Tamarit, P. Negrier and Y. Haget, Molecular interactions and packing in molecular alloys between nonisomorphous plastic phases, J. Solid State Chem., 1996, 124, 29–38 CrossRef CAS.
- D. K. Benson, R. W. Burrows and J. D. Webb, Solid state phase transitions in pentaerythritol and related polyhydric alcohols, Sol. Energy Mater., 1986, 13, 133–152 CrossRef CAS.
- V. Busico, C. Carfagna, V. Salerno, M. Vacatello and F. Fittipaldi, The layer perovskites as thermal energy storage systems, Sol. Energy, 1980, 24, 575–579 CrossRef CAS.
- M. K. Nahas and F. H. Constable, Thermal conductivity of mud brick, Nature, 1938, 142, 837 CrossRef.
- H. Tokoro, M. Yoshikiyo, K. Imoto, A. Namai, T. Nasu, K. Nakagawa, N. Ozaki, F. Hakoe, K. Tanaka, K. Chiba, R. Makiura, K. Prassides and S. Ohkoshi, External stimulation-controllable heat-storage ceramics, Nat. Commun., 2015, 6, 7037 CrossRef CAS PubMed.
- S. Ohkoshi, M. Yoshikiyo, J. MacDougall, Y. Ikeda and H. Tokoro, Long-term heat-storage materials based on λ-Ti3O5 for green transformation (GX), Chem. Commun., 2023, 59, 7875–7886 RSC.
- S. Ohkoshi, Y. Tsunobuchi, T. Matsuda, K. Hashimoto, A. Namai, F. Hakoe and H. Tokoro, Synthesis of a metal oxide with a room-temperature photoreversible phase transition, Nat. Chem., 2010, 2, 539–545 CrossRef CAS PubMed.
- C. Mariette, M. Lorenc, H. Cailleau, E. Collet, L. Guérin, A. Volte, E. Trzop, R. Bertoni, X. Dong, B. Lépine, O. Hernandez, E. Janod, L. Cario, V. Ta Phuoc, S. Ohkoshi, H. Tokoro, L. Patthey, A. Babic, I. Usov, D. Ozerov, L. Sala, S. Ebner, P. Böhler, A. Keller, A. Oggenfuss, T. Zmofing, S. Redford, S. Vetter, R. Follath, P. Juranic, A. Schreiber, P. Beaud, V. Esposito, Y. Deng, G. Ingold, M. Chergui, G. F. Mancini, R. Mankowsky, C. Svetina, S. Zerdane, A. Mozzanica, A. Bosak, M. Wulff, M. Levantino, H. Lemke and M. Cammarata, Strain wave pathway to semiconductor-to-metal transition revealed by time-resolved X-ray powder diffraction, Nat. Commun., 2021, 12, 1239 CrossRef CAS PubMed.
- B. Yang, Z. Zhang, P. Liu, X. Fu, J. Wang, Y. Cao, R. Tang, X. Du, W. Chen, S. Li, H. Yan, Z. Li, X. Zhao, G. Qin, X.-Q. Chen and L. Zuo, Flatband λ-Ti3O5 towards extraordinary solar steam generation, Nature, 2023, 622, 499–506 CrossRef CAS PubMed.
- Y. Araki, S. Ohkoshi and H. Tokoro, Synthesis of λ-Ti3O5 nanocrystals using a block copolymer, Mater. Today Energy, 2020, 18, 100525 CrossRef CAS.
- S. Ohkoshi, H. Tokoro, K. Nakagawa, M. Yoshikiyo, F. Jia and A. Namai, Low-pressure-responsive heat-storage ceramics for automobiles, Sci. Rep., 2019, 9, 13203 CrossRef PubMed.
- F. Hakoe, H. Tokoro and S. Ohkoshi, Dielectric and optical constants of λ-Ti3O5 film measured by spectroscopic ellipsometry, Mater. Lett., 2017, 188, 8–12 CrossRef CAS.
- G. Batdembere, Y. Ganchimeg, M. Enkhtuul, O Enkhtsolmon, G. Munkhsaikhan and D. Otgonbayar, Synthesis and Characterization of Nanosized Ti3O5 Crystals under Inert Gas Flow, Nano Hybrids Composites, 2022, 39, 73–79 Search PubMed.
- Y. Cai, Q. Shi, M. Wang, X. Lv, Y. Cheng and W. Huang, Synthesis of nanoscale lambda-Ti3O5 via a PEG assisted sol-gel method, J. Alloys Compd., 2020, 848, 156585 CrossRef CAS.
- T. Nasu, H. Tokoro, K. Tanaka, F. Hakoe, A. Namai and S. Ohkoshi, Sol–gel synthesis of nanosized λ-Ti3O5 crystals, Mater. Sci. Eng., 2014, 54, 012008 CAS.
- Q. Shi, G. Chai, W. Huang, Y. Shi, B. Huang, D. Wei, J. Qi, F. Su, W. Xu and T. Lu, Fabrication of nanocrystalline λ-Ti3O5 with tunable terahertz wave transmission properties across a temperature induced phase transition, J. Mater. Chem. C, 2016, 4, 10279–10285 RSC.
- X. Zhang, W. Liu, H. Yu, X. Zhong, L. Wang, A. Singh and Y. Lin, Preparation and oxygen sensing properties of Ti3O5 submicron rods, Micro Nano Lett., 2016, 11, 811–813 CrossRef CAS.
- D. Wei, W. Huang, Q. Shi, T. Lu and B. Huang, Effect of coating layers on nano-TiO2 particles on the preparation of nanocrystalline λ-Ti3O5 by carbothermal reduction, J. Mater. Sci.: Mater. Electron., 2016, 27, 4216–4222 CrossRef CAS.
- G. Chai, W. Huang, Q. Shi, S. Zheng and D. Wei, Preparation and characterization of λ-Ti3O5 by carbothermal reduction of TiO2, J. Alloys Compd., 2015, 621, 404–410 CrossRef CAS.
- Z. Ertekin, N. Ö. Pekmez and K. Pekmez, One-step electrochemical deposition of thin film titanium suboxide in basic titanyl sulfate solution at room temperature, J. Solid State Electrochem., 2020, 24, 975–986 CrossRef CAS.
- Z. Ertekin, U. Tamer and K. Pekmez, Cathodic electrochemical deposition of Magnéli phases TinO2n−1 thin films at different temperatures in acetonitrile solution, Electrochim. Acta, 2015, 163, 77–81 CrossRef CAS.
- K. Yoshimatsu and H. Kumigashira, Direct Synthesis of Metastable λ-Phase Ti3O5 Films on LaAlO3 (110) Substrates at High Temperatures, Cryst. Growth Des., 2022, 22, 703–710 CrossRef CAS.
- H. Chen, Y. Hirose, K. Nakagawa, K. Imoto, S. Ohkoshi and T. Hasegawa, Non-metallic electrical transport properties of a metastable λ-Ti3O5 thin film epitaxially stabilized on a pseudobrookite seed layer, Appl. Phys. Lett., 2020, 116, 201904 CrossRef CAS.
- C. P. Slichter and H. G. Drickamer, Pressure-induced electronic changes in compounds of iron, J. Chem. Phys., 1972, 56, 2142–2160 CrossRef CAS.
- F. Jia, M. Yoshikiyo, R. Makuta, K. Kawakami, H. Tokoro and S. Ohkoshi, Observation of a Pressure Effect on an Al-substituted λ-Ti3O5 Heat-storage Material, Chem. Lett., 2023, 52, 748–751 CrossRef CAS.
- S. Jütten and T. Bredow, Doping Effect on the Electronic Structure and Heat-Storage Properties of Ti3O5, J. Phys. Chem. C, 2023, 127, 10445–10452 CrossRef.
- S. Ohkoshi, F. Jia, M. Yoshikiyo, K. Imoto, H. Tokoro, K. Nakagawa, Y. Maeno, A. Namai, R. Harada, K. Hattori, K. Kojima, K. Sugiura and T. Suganuma, Pressure effect on long-term heat storage ceramics based on Mg-substituted λ-Ti3O5, Mater. Adv., 2022, 3, 4824–4830 RSC.
- X. Fu, W. Chen, X. Hao, Z. Zhang, R. Tang, B. Yang, X. Zhao and L. Zuo, Preparing high purity λ-Ti3O5 and Li/λ-Ti3O5 as high-performance electromagnetic wave absorbers, J. Mater. Chem. C, 2021, 9, 7976–7981 RSC.
- Y. Nakamura, Y. Sakai, M. Azuma and S. Ohkoshi, Long-term heat-storage ceramics absorbing thermal energy from hot water, Sci. Adv., 2020, 6, eaaz5264 CrossRef CAS PubMed.
- M. Wang, W. Huang, Z. Shen, J. Gao, Y. Shi, T. Lu and Q. Shi, Phase evolution and formation of λ phase in Ti3O5 induced by magnesium doping, J. Alloys Compd., 2019, 774, 1189–1194 CrossRef CAS.
- R. Takahama, T. Ishii, D. Indo, M. Arizono, C. Terakura, Y. Tokura, N. Takeshita, M. Noda, H. Kuwahara, T. Saiki, T. Katsufuji, R. Kajimoto and T. Okuda, Structural, magnetic, transport, and thermoelectric properties of the pseudobrookite AlTi2O5-Ti3O5 system, Phys. Rev. Mater., 2020, 4, 074401 CrossRef CAS.
- Z. Shen, Q. Shi, W. Huang, B. Huang, M. Wang, J. Gao, Y. Shi and T. Lu, Stabilization of microcrystal λ-Ti3O5 at room temperature by aluminum-ion doping, Appl. Phys. Lett., 2017, 111, 191902 CrossRef.
Footnote |
† Electronic supplementary information (ESI) available: SEM image, PXRD patterns, Rietveld analyses, crystallographic data, magnetic properties, details of parameters in theoretical calculations, calculated fraction versus pressure curves, and relationship between ΔHtrans, ΔS and Pth. See DOI: https://doi.org/10.1039/d3ma01162c |
|
This journal is © The Royal Society of Chemistry 2024 |