DOI:
10.1039/D3MA00671A
(Paper)
Mater. Adv., 2024,
5, 2218-2229
Organohalogenochromism (OHC) of D–π–A pyridinium dye polymer films and the colorimetric detection of volatile organic halogen compounds†
Received
7th September 2023
, Accepted 28th October 2023
First published on 30th October 2023
Abstract
Volatile organohalogen compounds (VOHCs) are commonly used in manufacturing; however, most of them are extremely toxic to human health, biological systems and the environment even when exposed to small quantities. Meanwhile, organohalogenochromism (OHC) is a photophysical phenomenon that induces a significant hypsochromic or bathochromic shift of photoabsorption bands of organic dyes in halogenated solvents compared with those in non-halogenated solvents. Thus, in this study, to ensure the expression of OHC and to develop optical polymeric materials based on OHC for the visualization and detection of VOHCs, we designed and synthesized a D–π–A type pyridinium dye monomer OD2-V possessing intramolecular charge transfer (ICT) characteristics and prepared a copolymer poly(OD2-co-S) composed of OD2-V and styrene. It was found that poly(OD2-co-S) and OD2-V exhibit bathochromic shift-type OHC (b-OHC) in the solution state. However, the D–π–A type pyridine dye NI2-V and its copolymer poly(NI2-co-S) composed of NI2-V and styrene did not exhibit such OHC. Furthermore, drop-casted poly(OD2-co-S) film exhibits the bathochromic shift of the ICT-based photoabsorption band in the halogenated solvent–vapor atmosphere, that is, a visual change in color from yellow to orange upon exposure to VOHCs, including dichloromethane (CH2Cl2) and chloroform (CHCl3). Indeed, the poly(OD2-co-S) film exhibits a good reversible switching of the ICT-based photoabsorption band in the air and VOHC-vapor atmosphere process. The optical sensing properties of poly(OD2-co-S) film to VOHCs, 1H NMR spectral measurements and semi-empirical molecular calculations for OD2-V demonstrate that the enhanced ICT characteristics of OD2 chromophore induced by the intermolecular interaction between the dye and the organohalogen molecules are responsible for the b-OHC of D–π–A type pyridinium dyes. Consequently, we propose that polymeric organohalogenochromic dyes are one of the most promising and convenient functional materials for the colorimetric detection of VOHCs.
Introduction
Organohalogenochromism (OHC) has recently been recognized as a photophysical phenomenon that induces a significant hypsochromic or bathochromic shift of the photoabsorption bands of organic dyes in halogenated solvents compared with those in non-halogenated solvents.1–4 Hence, OHC is a specific solvatochromism observed only in halogenated solvents that is different from a common solvatochromism depending on the dielectric constant (εr) of solvent and solvent polarity parameter ET(30); it can be actually classified into two types, positive solvatochromism (p-SC) and negative solvatochromism (n-SC), which correspond to a bathochromic shift and a hypsochromic shift, respectively, with increasing solvent polarity.5–12 Bathochromic shift-type OHC (b-OHC) has been occasionally found in donor–π–acceptor (D–π–A) type dyes, which are composed of dialkyl or diaryl amino groups as a strong electron-donating moiety (D) and pyridinium, benzothiazolium, dicyanomethylene, and barbituric moieties as a strong electron-withdrawing moiety (A) connected by a π-conjugated bridge, and it can exhibit a photoabsorption band based on the intramolecular charge transfer (ICT) from the D moiety to the A moiety.1–4,13–28 However, few studies have been carried out on OHC although the phenomenon is of great scientific interest and practical importance, such as the development of optical sensors for the detection of toxic organohalogen compounds.29–35 Actually, volatile organohalogen compounds (VOHCs) are commonly used in manufacturing,36–43 but most of them are extremely toxic to human health, biological systems and the environment even when exposed to small quantities. Several detection techniques for VOHCs have been developed, including gas chromatography–mass spectrometry (GC–MS) and gas chromatography-flame ionization detector (GC-FID) methods.44–47 Although these techniques provide sufficient qualitative and quantitative accuracy, they generally require time-consuming procedures for sample preparation and sophisticated experimental skills for operation and analysis as well as expensive instrumentation. The development of optical detection techniques for VOHCs based on organic dyes, which involve facile operation and simple analysis, allows not only sufficient accuracy, high sensitivity, and fast response but also allows the visualization and real-time monitoring of VOHCs. Thus far, we have focused on the elucidation of OHC and the creation of functional dye materials based on OHC for visualization and detection of organohalogen compounds.1–4 In our previous work,2 we developed D–π–A type pyridinium dyes OD1–3 bearing a halide anion (X− = Cl−, Br−, or I−) as the counter anion and investigated their photophysical properties in various solvents (Fig. 1a). It was found that OD1–3 showed a b-OHC, that is, the significant bathochromic shift of the ICT-based photoabsorption band in halogenated solvents although the semi-empirical molecular orbital (MO) calculations predicted that OD1–3 will show n-SC. Based on the 1H NMR spectroscopic studies of OD1–3 in deuterated non-halogenated solvents and deuterated halogenated solvent, it was suggested that the enhanced ICT characteristics of OD1–3 in halogenated solvents, which might be induced by the formation of halogen–halide anion interaction48–50 between the halogen atoms of halogenated solvents and the counter halide anion of the dye, are responsible for the b-OHC of D–π–A type pyridinium dyes bearing a counter anion. Moreover, the b-OHC is observed for non-ionic D–π–A dyes with dicyanomethylene and barbituric moieties as a strong electron-withdrawing group,18–20 but their OHC remains poorly elucidated. Therefore, to create organohalogenochromic dye materials for the visualization and detection of VOHCs, it is necessary to further investigate the effects of intermolecular interaction between the dyes and the organohalogen molecules, including halogen–π interaction51–55 between halogen atoms of halogenated solvents and aromatic π-systems of dye molecules as well as halogen–halide anion interaction.48–50
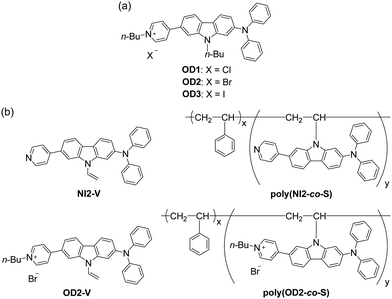 |
| Fig. 1 Chemical structures of (a) D–π–A type pyridinium dyes OD1–3 possessing organohalogenochromic characteristics (previous work) and (b) D–π–A type pyridine dye monomer NI2-V, D–π–A type pyridinium dye monomer OD2-V, and their copolymer poly(NI2-co-S) and poly(OD2-co-S) composed of the dye monomer and styrene (in this work). | |
Thus, in this work, to ensure the expression of OHC and to develop optical polymeric materials based on OHC for the colorimetric detection of VOHCs, we designed and synthesized a D–π–A type pyridinium dye monomer OD2-V with a vinyl group on the carbazole skeleton as a derivative of OD2 and prepared a copolymer poly(OD2-co-S) composed of OD2-V and styrene (Fig. 1b). It was found that poly(OD2-co-S) exhibits b-OHC in the solution state, and its drop-casted film shows the bathochromic shift of the ICT-based photoabsorption band in halogenated solvent–vapor atmosphere, that is, a visual change in color when exposed to VOHCs, including dichloromethane (CH2Cl2) and chloroform (CHCl3). The poly(OD2-co-S) film exhibits good reversible switching of the ICT-based photoabsorption band in the air and VOHC-vapor atmosphere process. Herein, we report the b-OHC of polymeric organohalogenochromic dye based on the intermolecular interaction between the dye and the organohalogen molecules and the optical sensing properties of its film to VOHCs, which were revealed by comparison with the D–π–A type pyridine dye monomer NI2-V and its copolymer poly(NI2-co-S) composed of NI2-V and styrene.
Results and discussion
Synthesis
D–π–A type pyridinium dye OD2-V and a copolymer poly(OD2-co-S) composed of OD2-V and styrene were prepared according to a stepwise synthetic protocol (Scheme 1). First, NI2-V was obtained in moderate yield (64%) by the reaction of NI256 with n-BuLi, followed by treatment with vinyl bromide in the presence of Pd(dba)2/2P(t-Bu)3. Next, the reaction of NI2-V with n-butyl bromide gave OD2-V in a relatively high yield (78%). Then, polymerization was carried out at a ratio of NI2-V to styrene of 1
:
99 using 2,2′-azobis(isobutyronitrile) (AIBN) as a free radical initiator to give poly(NI2-co-S) as a white solid (Mn = 59
200, Mw/Mn = 1.55, 8% yield). The 1H NMR spectrum indicated that the molar ratio (x/y) of styrene unit (x) and NI2-V unit (y) and the weight percentage (wt%) of NI2-V in the obtained poly(NI2-co-S) were determined to be ca. 667 and ca. 0.62 wt%, respectively. Finally, the reaction of poly(NI2-co-S) with n-butyl bromide gave poly(OD2-co-S) as a yellow solid in high yield (84%). The preparation of poly(OD2-co-S) was demonstrably confirmed by the FT-IR, the 1H NMR (Fig. S3b and S5b, ESI†) and the photoabsorption spectra (Fig. 3c and d).
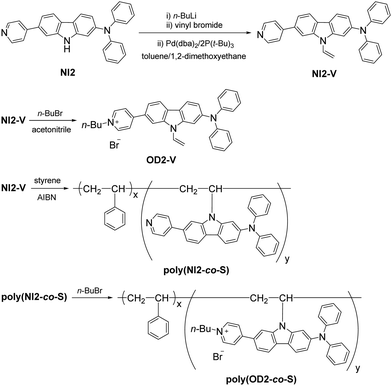 |
| Scheme 1 Synthesis of NI2-V, OD2-V, poly(NI2-co-S), and poly(OD2-co-S). | |
Photoabsorption properties
The photoabsorption spectra of NI2-V, OD2-V, poly(NI2-co-S), and poly(OD2-co-S) in various solvents are shown in Fig. 2 and 3, and their photophysical data are summarized in Tables 1 and 2. The photoabsorption bands in the visible region appeared at around 350–400 nm for NI2-V and poly(NI2-co-S) and 400–550 nm for OD2-V and poly(OD2-co-S), which are attributed to ICT excitation from the (diphenylamino)carbazole unit as a D–π moiety to a pyridine ring or pyridinium ring as an A moiety, which is supported by the semi-empirical MO calculations, as discussed later. The ICT-based photoabsorption maximum wavelength λabs-solutionmax(exp) (ca. 430–490 nm) of OD2-V and poly(OD2-co-S) showed a large bathochromic shift owing to the strong electron-withdrawing ability of the pyridinium ring compared with those (λabs-solutionmax(exp) = 370–380 nm) of NI2-V and poly(NI2-co-S), and the ε values (ca. 20
000–40
000 M−1 cm−1) for NI2-V are higher than those (ca. 10
000–25
000 M−1 cm−1) for OD2-V. The photoabsorption spectra of NI2-V and poly(NI2-co-S) are nearly independent of the kinds of solvents (Fig. 2a, b and 3a, b), but those of OD2-V and poly(OD2-co-S) depend on the categories of solvents. In particular, it is worth noting that OD2-V and poly(OD2-co-S) show a significant bathochromic shift of λabs-solutionmax(exp) in halogenated solvents compared with those in non-halogenated solvents (Fig. 2c, d and 3c, d), that is, a pronounced b-OHC as with the previously reported D–π–A pyridinium dye OD22 (Fig. 1a). Indeed, for NI2-V, the rage of λabs-solutionmax(exp) is from 369 nm in acetone or acetonitrile to 381 nm in iodobenzene (PhI) for all the solvents and from 369 nm in acetone or acetonitrile to 376 nm in pyridine for non-halogenated solvents, but for OD2-V, it is from 433 nm in DMSO to 491 nm in CH2I2 for all the solvents and from 433 nm in DMSO to 461 nm in pyridine for non-halogenated solvents. Therefore, for NI2-V, the difference (Δλabs-solutionmax(exp)) between the shortest and longest λabs-solutionmax(exp) values is 12 nm (853 cm−1) for all the solvents and 7 nm (504 cm−1) for non-halogenated solvents, but for OD2-V, it is 58 nm (2728 cm−1) for all the solvents and 28 nm (1402 cm−1) for non-halogenated solvents (Table 1). Similarly, the Δλabs-solutionmax(exp) values for all the solvents and non-halogenated solvents are, respectively, 7 nm (488 cm−1) and 4 nm (281 cm−1) for poly(NI2-co-S) and 45 nm (2081 cm−1) and 26 nm (1251 cm−1) for poly(OD2-co-S) (Table 2).
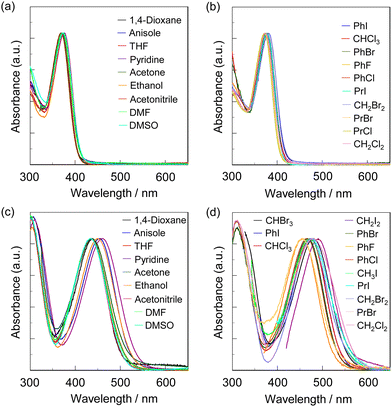 |
| Fig. 2 Photoabsorption spectra of (a) NI2-V and (c) OD2-V in non-halogenated solvents and (b) NI2-V and (d) OD2-V in halogenated solvents. | |
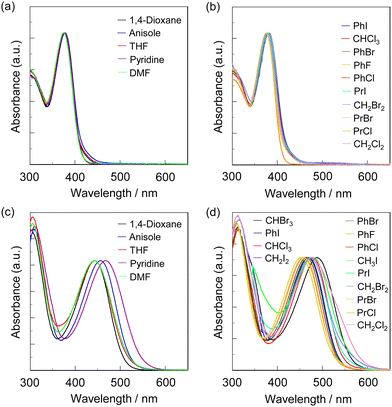 |
| Fig. 3 Photoabsorption spectra of (a) poly(NI2-co-S) and (c) poly(OD2-co-S) in non-halogenated solvents and (b) poly(NI2-co-S) and (d) poly(OD2-co-S) in halogenated solvents. | |
Table 1 Experimental and calculational photophysical data of NI2-V and OD2-V in various solvents
No. |
Solvents |
ε
r
|
E
T(30)b/kcal mol−1 |
NI2-V
|
OD2-V
|
λ
abs-solutionmax(exp)/nm (ε/M−1 cm−1) |
λ
abs-solutionmax(calc)/nm (ff) |
λ
abs-solutionmax(exp)/nm (ε/M−1 cm−1) |
λ
abs-solutionmax(calc)/nm (ff) |
Dielectric constant (ref. 57).
Solvent polarity parameter (ref. 5 and 58).
No data.
NI2-V reacts with the solvent to form the pyridinium salts with the ICT-based λabs-solutionmax(exp) at 460–480 nm (Fig. S7a, ESI).
Poorly soluble.
Oscillator strength.
|
1 |
1,4-Dioxane |
2.22 |
36.0 |
373 (27 000) |
353 (0.88) |
439 (—e) |
440 (1.01) |
2 |
Anisole |
4.30 |
37.1 |
375 (32 000) |
356 (0.88) |
454 (20 900) |
398 (1.14) |
3 |
CHBr3 |
4.40 |
37.7 |
—d |
356 (0.88) |
481 (25 900) |
396 (1.14) |
4 |
PhI |
4.59 |
36.2 |
381 (20 700) |
357 (0.88) |
473 (23 200) |
394 (1.15) |
5 |
CHCl3 |
4.81 |
39.1 |
376 (26 600) |
355 (0.89) |
472 (26 100) |
396 (1.15) |
6 |
CH2I2 |
5.32 |
36.5 |
—d |
358 (0.88) |
491 (10 900) |
387 (1.18) |
7 |
PhBr |
5.45 |
36.6 |
378 (16 000) |
357 (0.88) |
467 (—e) |
390 (1.18) |
8 |
PhF |
5.47 |
37.0 |
373 (32 800) |
356 (0.89) |
455 (—e) |
391 (1.17) |
9 |
PhCl |
5.69 |
36.8 |
376 (23 800) |
356 (0.89) |
466 (—e) |
388 (1.18) |
10 |
CH3I |
6.97 |
—c |
—d |
357 (0.89) |
473 (14 000) |
383 (1.21) |
11 |
1-Iodopropane (PrI) |
7.07 |
35.7 |
377 (26 700) |
357 (0.89) |
475 (—e) |
383 (1.21) |
12 |
THF |
7.52 |
37.4 |
372 (20 800) |
356 (0.89) |
434 (24 000) |
382 (1.21) |
13 |
CH2Br2 |
7.77 |
39.4 |
380 (26 600) |
357 (0.89) |
473 (18 000) |
380 (1.22) |
14 |
1-Bromopropane (PrBr) |
8.09 |
36.9 |
374 (42 000) |
356 (0.89) |
456 (—e) |
382 (1.21) |
15 |
1-Chloropropane (PrCl) |
8.59 |
37.4 |
372 (28 300) |
356 (0.89) |
—e |
382 (1.22) |
16 |
CH2Cl2 |
8.93 |
40.7 |
373 (18 100) |
356 (0.89) |
477 (16 400) |
383 (1.21) |
17 |
Pyridine |
13.3 |
40.5 |
376 (25 000) |
358 (0.89) |
461 (21 600) |
373 (1.25) |
18 |
Acetone |
21.0 |
42.2 |
369 (28 400) |
356 (0.89) |
436 (25 000) |
372 (1.27) |
19 |
Ethanol |
25.3 |
51.9 |
374 (32 600) |
357 (0.89) |
449 (20 000) |
371 (1.27) |
20 |
Acetonitrile |
36.6 |
45.6 |
369 (22 100) |
357 (0.89) |
435 (23 800) |
369 (1.28) |
21 |
DMF |
38.3 |
43.2 |
373 (29 800) |
358 (0.89) |
434 (17 400) |
367 (1.29) |
22 |
DMSO |
47.2 |
45.1 |
375 (30 000) |
358 (0.89) |
433 (21 500) |
365 (1.31) |
Table 2 Experimental photophysical data of poly(NI2-co-S) and poly(OD2-co-S) in various solvents
No. |
Solvents |
ε
r
|
E
T(30)b/kcal mol−1 |
Poly(NI2-co-S)
|
Poly(OD2-co-S)
|
λ
abs-solutionmax(exp)/nm |
λ
abs-solutionmax(exp)/nm |
Dielectric constant (ref. 57).
Solvent polarity parameter (ref. 5 and 58).
No data.
Poly(NI2-co-S) reacts with the solvent to form the pyridinium salts with the ICT-based λabs-solutionmax(exp) at 460–480 nm (Fig. S7b, ESI).
Poorly soluble.
|
1 |
1,4-Dioxane |
2.22 |
36.0 |
375 |
443 |
2 |
Anisole |
4.30 |
37.1 |
379 |
456 |
3 |
CHBr3 |
4.40 |
37.7 |
—d |
488 |
4 |
PhI |
4.59 |
36.2 |
382 |
470 |
5 |
CHCl3 |
4.81 |
39.1 |
380 |
479 |
6 |
CH2I2 |
5.32 |
36.5 |
—d |
—e |
7 |
PhBr |
5.45 |
36.6 |
379 |
467 |
8 |
PhF |
5.47 |
37.0 |
377 |
460 |
9 |
PhCl |
5.69 |
36.8 |
379 |
464 |
10 |
CH3I |
6.97 |
—c |
—d |
477 |
11 |
1-Iodopropane (PrI) |
7.07 |
35.7 |
380 |
474 |
12 |
THF |
7.52 |
37.4 |
376 |
445 |
13 |
CH2Br2 |
7.77 |
39.4 |
382 |
473 |
14 |
1-Bromopropane (PrBr) |
8.09 |
36.9 |
378 |
456 |
15 |
1-Chloropropane (PrCl) |
8.59 |
37.4 |
376 |
454 |
16 |
CH2Cl2 |
8.93 |
40.7 |
377 |
475 |
17 |
Pyridine |
13.3 |
40.5 |
378 |
469 |
18 |
Acetone |
21.0 |
42.2 |
—e |
—e |
19 |
Ethanol |
25.3 |
51.9 |
—e |
—e |
20 |
Acetonitrile |
36.6 |
45.6 |
—e |
—e |
21 |
DMF |
38.3 |
43.2 |
375 |
444 |
22 |
DMSO |
47.2 |
45.1 |
—e |
—e |
Furthermore, it was found that the λabs-solutionmax(exp) of OD2-V and poly(OD2-co-S) in halopropanes (PrCl, PrBr, and PrI) shows a hypsochromic shift compared to those in halomethanes (CHBr3, CHCl3, CH2I2, CH2Br2, CH2Cl2, and CH3I) and halobenzenes (PhI, PhBr, PhCl, and PhF) although the λabs-solutionmax(exp) of OD2-V in PrI shows a slight bathochromic shift by 2 nm compared to those in CH3I and PhI (Tables 1 and 2). Meanwhile, the λabs-solutionmax(exp) of OD2-V and poly(OD2-co-S) in pyridine as a protophilic solvent and ethanol as a polar protic solvent appear at a longer wavelength region by ca. 30 nm and ca. 15 nm, respectively, than those in other non-halogenated solvents. As shown in Fig. 4, the colors of NI2-V and poly(NI2-co-S) are nearly colorless in all the solvents. Interestingly, the colors of OD2-V and poly(OD2-co-S) are yellow in non-halogenated solvents but orange in halogenated solvents, especially in CHBr3, CH3I, CH2Br2, and CH2Cl2.
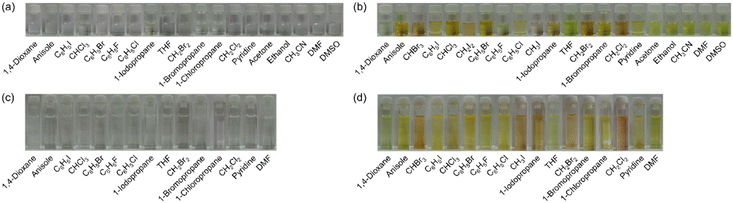 |
| Fig. 4 Photographs of (a) NI2-V, (b) OD2-V, (c) poly(NI2-co-S), and (d) poly(OD2-co-S) in various solvents. | |
To clarify the influence of solvent polarity on the ICT-based photoabsorption bands of NI2-V, OD2-V, poly(NI2-co-S), and poly(OD2-co-S), the wavenumbers (ṽ) of the λabs-solutionmax(exp) are plotted against the dielectric constant (εr) of the solvent (Fig. 5a, b and 6a, b) or solvent polarity parameter ET(30) (Fig. 5e, f and 6c, d). The plots for NI2-V and poly(NI2-co-S) demonstrate that the ṽ shows no systematic change and remains on a near plateau in the range of εr or ET(30) value between 1,4-dioxane (no. 1) and DMSO (no. 22) or ethanol (no. 19) (Fig. 5a, e and 6a, c). Thus, the fact indicates that NI2-V and poly(NI2-co-S) do not possess appreciable solvatochromic properties because for D–π–A type pyridine dyes (NI2 chromophore), the structural and electronic characteristics of both the ground and Franck–Condon photoexcited states do not differ much with a change in solvent polarity. Meanwhile, the plots for OD2-V and poly(OD2-co-S) did not show a systematic change in ṽ with increasing ET(30) or εr value and remained on a near plateau between non-halogenated solvents except for pyridine and ethanol, but obviously, the ṽ in halogenated solvents are located in the low-wavenumber range compared with those in non-halogenated polar and non-polar solvents (Fig. 5b, f and 6b, d); the εr value (7.77) of CH2Br2 is very similar to that (7.52) of THF, and the λabs-solutionmax(exp) (473 nm) of OD2-V and poly(OD2-co-S) in CH2Br2 appears at a longer wavelength region by 39 nm and 28 nm, respectively, than those (434 nm and 445 nm, respectively) in THF. Therefore, the fact evidently indicates that the D–π–A type pyridinium dyes (OD2 chromophore) possess remarkable organohalogenochromic properties. Moreover, one can see that the λabs-solutionmax(exp) of OD2-V and poly(OD2-co-S) in iodinated solvents, such as PhI, CH2I2, and 1-iodopropane (PrI), occurs at a lower wavenumber range (a longer wavelength region) compared to those in the corresponding chlorinated and brominated solvents. Furthermore, we performed the photoabsorption spectral measurements of OD2-V in a mixture of THF as a non-halogenated solvent and CH2Br2 as a halogenated solvent and plotted the ṽ of the λabs-solutionmax(exp) against the THF/CH2Br2 mixture composition (Fig. S8, ESI†). If the plot shows a curve, the formation of a specific interaction between the dye and the solvent molecules is anticipated.59,60 However, the plot was almost linear, and thus we could not obtain useful information for intermolecular interactions between the dye and the organohalogen molecules.
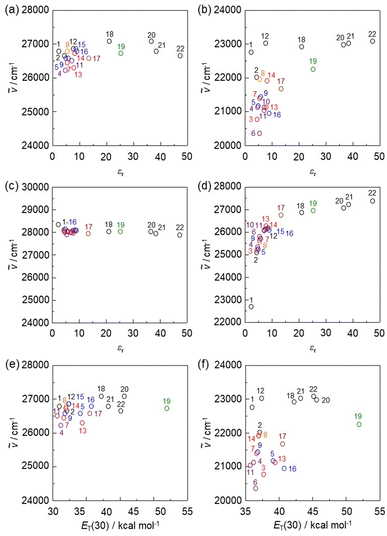 |
| Fig. 5 Plots of the experimental photoabsorption maximum wavenumber (ṽ) of (a) NI2-V and (b) OD2-V against the εr value of the solvent. Plots of the calculated ṽ of (c) NI2-V and (d) OD2-V against the εr value of the solvent. Plots of the experimental ṽ of (e) NI2-V and (f) OD2-V against solvent polarity parameter ET(30). The numbers correspond to those of Table 1. The circles in black, orange, blue, red, purple, brown, and green show non-halogenated solvents, fluorobenzene, chlorinated solvents, brominated solvents, iodinated solvents, pyridine, and ethanol, respectively. | |
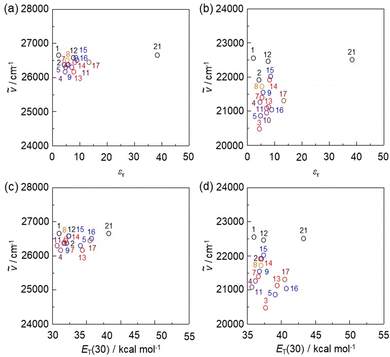 |
| Fig. 6 Plots of the experimental photoabsorption maximum wavenumber (ṽ) of (a) poly(NI2-co-S) and (b) poly(OD2-co-S) against the εr value of the solvent. Plots of the experimental ṽ of (c) poly(NI2-co-S) and (d) poly(OD2-co-S) against solvent polarity parameter ET(30). The numbers correspond to those in Table 2. The circles in black, orange, blue, red, purple, brown, and green represent non-halogenated solvents, fluorobenzene, chlorinated solvents, brominated solvents, iodinated solvents, pyridine, and ethanol, respectively. | |
Semi-empirical molecular calculations
To reveal the solvatochromism of the D–π–A type pyridine and pyridinium dyes, semi-empirical MO calculations of NI2-V and the dye cation for OD2-V were performed by applying the INDO/S method, with the SCRF Onsager Model applied after performing geometrical optimizations using the MOPAC/AM1 method. For NI2-V and OD2-V in all the solvents, the MO calculations show that the first excitation bands are mainly assigned to a transition from the highest occupied molecular orbital (HOMO) to the lowest unoccupied molecular orbital (LUMO) with CI (configuration interaction) component of ca. 60% for NI2-V and ca. 70% for OD2-V, where the HOMOs of NI2-V and OD2-V are mostly localized on the (diphenylamino)carbazole moiety and the LUMOs are mostly localized on the carbazole moiety containing the pyridine ring for NI2-V and the pyridinium ring for OD2-V (Fig. 7a, b for CH2Cl2, see Fig. S9 and S10 for 1,4-dioxane and DMSO, respectively, ESI†). Thus, the HOMO and LUMO distributions for the molecular structure of OD2-V are adequately separated. The changes in the calculated electron density accompanying the first electron excitation demonstrate that for OD2-V, the ICT upon photoexcitation occurs from the (diphenylamino)carbazole unit as a D–π moiety to the pyridinium ring as an A moiety, but for NI2-V, the ICT upon photoexcitation occurs from the diphenylamino group as a D moiety to the pyridine ring as an A moiety through the carbazole skeleton as a π-conjugated bridge (Fig. 7c, see Fig. S9 and S10 for 1,4-diocxane and DMSO, respectively, ESI†). The calculated ICT-based photoabsorption maxima (λabs-solutionmax(calc) = ca. 360 nm) of NI2-V appear in a shorter wavelength region compared to those (365–440 nm) of OD2-V (Table 1). The corresponding oscillator strength (f) values (ca. 1.0–1.3) for OD2-V are higher than those (ca. 0.9) for NI2-V. The plot of the λabs-solutionmax(calc) (ṽ) for NI2-V against the εr value of solvent shows that the ṽ is constant in the range of εr value between 1,4-dioxane (2.22) and DMSO (47.2) (Fig. 5c), indicating that the calculated ICT-based photoabsorption bands of NI2-V are independent of solvent polarity. Thus, for NI2-V, these calculated results agree with their experimental results. However, for OD2-V, with increasing solvent polarity from 1,4-dioxane to DMSO, the calculated ICT-based photoabsorption bands show hypsochromic shifts from 440 nm to 365 nm (Table 1). The plot of the λabs-solutionmax(calc) (ṽ) for OD2-V against the εr value demonstrates that the ṽ dramatically increases with the increase in εr value from 1,4-dioxane (2.22) to pyridine (13.3), while the ṽ gradually increases with the increase in εr value from pyridine to DMSO (47.2) (Fig. 5d). Obviously, for OD2-V, the profile of the calculational plot shows a general n-SC, which is significantly different from that of the experimental plot (Fig. 5b).
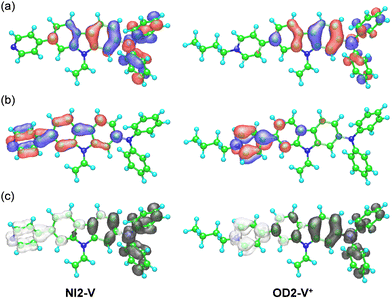 |
| Fig. 7 (a) HOMO and (b) LUMO of NI2-V and the dye cation (OD2-V+) for OD2-V. The red and blue lobes denote the positive and negative phases of the coefficients of the molecular orbitals. The size of each lobe is proportional to the MO coefficient. (c) Calculated electron density changes accompanying the first electronic excitation of NI2-V and OD2-V+ (by the SCRF Onsager model (solvent = CH2Cl2)). The black and white lobes signify decrease and increase in electron density accompanying the electronic transition, respectively. Their areas indicate the magnitude of the electron density change. | |
1H NMR spectra
To investigate the solvent effect on the electronic structures of NI2-V and OD2-V in the ground state, we performed 1H NMR spectral measurements in DMSO-d6 with a high εr value, acetone-d6 with a moderate εr value, and THF-d8 with a relatively low εr value as a non-halogenated solvent, ethanol-d6 as a polar protic solvent, and pyridine-d5 as a protophilic solvent, and in CD2Cl2 and CDCl3 as a halogenated solvent (Fig. 8 and 9). There is a slight difference in the chemical shifts of the aromatic protons for NI2-V between the seven solvents, while in pyridine-d5, the signals for aromatic protons (Ha–h) of the carbazole skeleton and the pyridyl group show downfield shifts compared to those in other solvents. However, for OD2-V, considerable differences in the chemical shifts were observed between halogenated and non-halogenated solvents. With decreasing solvent polarity between DMSO-d6, acetone-d6, and THF-d8. The signal for Ha of the pyridinium ring shows downfield shifts, while those for Hc, Hd and He of the carbazole skeleton near the pyridinium ring show downfield and upfield shifts. Moreover, the signals for Ha, Hb, and Hc in ethanol-d6 show upfield shifts, whereas the signal of Ha in pyridine-d5 shows a downfield shift, compared to those in other non-halogenated solvents. Based on the results of 1H NMR spectral measurements, the bathochromic shifts of the ICT-based photoabsorption band of OD2-V in ethanol as a polar protic solvent and pyridine as a protophilic solvent may be due to the enhanced ICT characteristics, which are triggered, respectively, by the formation of hydrogen bonding between the bromide ion of OD2-V and the hydroxyl group of ethanol molecules and by the interaction of pyridine molecules with the pyridinium ring of OD2-V. It is worth mentioning here that the signals of Hb, Hc, and Hd in CD2Cl2 and CDCl3 show considerably upfield shifts compared to those in non-halogenated solvents. Additionally, because the εr value of CH2Cl2 is similar to that of THF, this result obviously indicates that the chemical shifts for OD2-V are not dependent on the solvent polarity but depend on the intermolecular interaction between the D–π–A type pyridinium dyes and the solvent molecules.
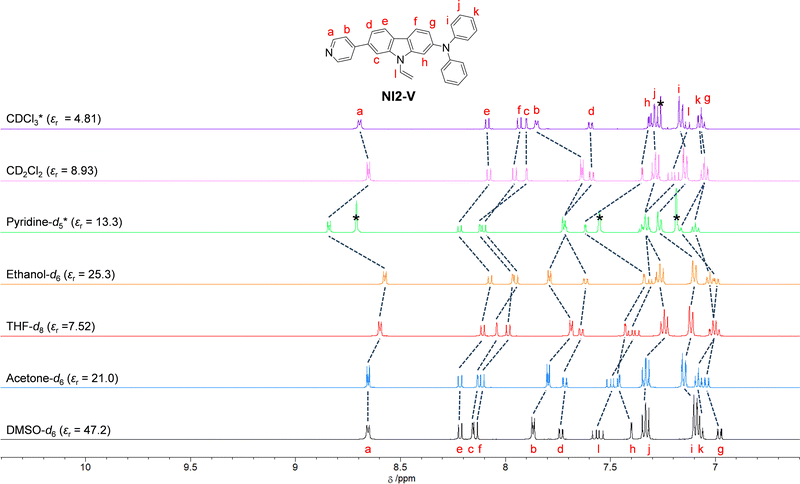 |
| Fig. 8
1H NMR spectra of NI2-V in CDCl3, CD2Cl2, pyridine-d5, ethanol-d6, THF-d8, acetone-d6, and DMSO-d6. | |
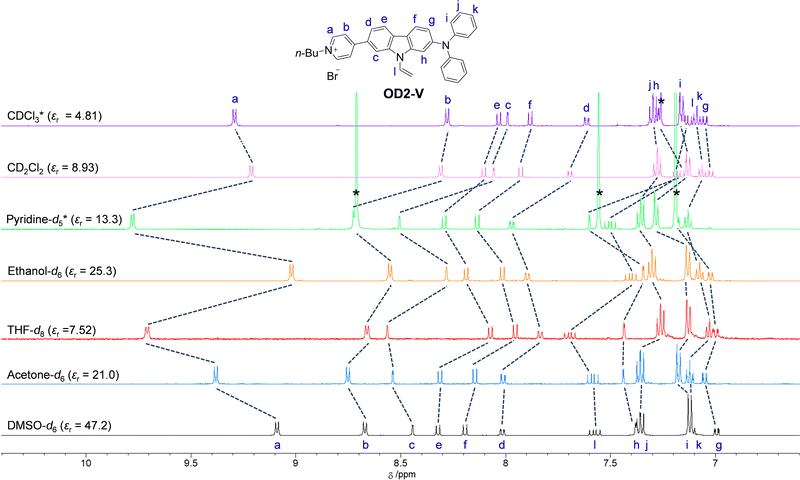 |
| Fig. 9
1H NMR spectra of OD2-V in CDCl3, CD2Cl2, pyridine-d5, ethanol-d6, THF-d8, acetone-d6, and DMSO-d6. | |
Awwadi et al. investigated the halogen–halide anion interaction between the halogen atoms of halogenated solvents and the counter halide anion of pyridinium salt based on theoretical and crystallographic studies. They demonstrated that the energy of interaction between the iodine atom of iodinated solvents and halide anion is greater than that of interaction between the chlorine atom in chlorinated solvents or the bromine atom in brominated solvents and the halide anion.48–50 In fact, the ICT-based photoabsorption bands of OD2-V and poly(OD2-co-S) in iodinated solvents occur at a lower wavenumber range (a longer wavelength region) compared to those in chlorinated and brominated solvents (Tables 1 and 2), which is accompanied by an increase in the energy of halogen–halide anion interaction. Meanwhile, Matter et al. demonstrated the presence of interaction between halogen atoms of the organohalogen compound and aromatic π-systems, that is, halogen–π interaction,51–55 which plays a crucial role in forming biological, supramolecular, and nanomaterial systems based on biding affinities, X-ray crystal structures, 3D database searches, and ab initio calculations.51 Thus, for OD2-V, the large upfield shifts of the signals for protons on the pyridinium and its neighboring aromatic rings from non-halogenated solvent to halogenated solvent are attributable to a change in the electronic structure of OD2-V owing to the halogen–halide anion interaction and/or the intermolecular halogen–π interaction between the periphery of the pyridinium moiety of the dyes and the halogen atoms of the organohalogen molecules.
Detection of VOHCs
Next, to evaluate the capability of organohalogenochromic dyes to act in polymer matrices for the visualization and detection of VOHCs, we prepared drop-casted poly(OD2-co-S) films on quartz glass substrates, in which the surfaces were treated with piranha solution and then modified with a hydrophobic monolayer using 1,1,1,3,3,3-hexamethyldisilazane (HMDS) to obtain stable polymer thin films prior to the drop casting, and performed the photoabsorption spectral measurement of the drop-casted poly(OD2-co-S) films before and after exposure to non-halogenated and halogenated solvent vapors. In addition, the poly(NI2-co-S) films were prepared on the piranha- and HMDS-treated quartz glass substrates using a drop-casting process for comparison with the drop-casted poly(OD2-co-S) films. The as-prepared poly(NI2-co-S) or poly(OD2-co-S) films (in the air) showed an ICT-based photoabsorption band with a λabs-filmmax of ca. 380 nm and ca. 450 nm, respectively (Fig. 10), as with the cases of poly(NI2-co-S) in non-halogenated and halogenated solvents and poly(OD2-co-S) in non-halogenated solvents (Fig. 3). When the poly(NI2-co-S) films were exposed to THF, DMF, CHCl3, CH2Cl2, or 1-bromopropane (PrBr) vapor (for 2 min in solvent–vapor atmosphere), the ICT-based photoabsorption band did not undergo appreciable changes in the spectral shape and the λabs-filmmax (Fig. 10a). However, the poly(OD2-co-S) films showed a relatively large bathochromic shift of the λabs-filmmax by ca. 10 nm upon exposure to CHCl3 or CH2Cl2 vapor (Fig. 10b). When the poly(OD2-co-S) films after exposure to the halogenated solvent vapors were placed in the air (on drying), the photoabsorption spectra recovered the original spectral shapes before exposure to the halogenated solvent vapors. Therefore, for poly(OD2-co-S) film, the reversibility of the λabs-filmmax in the CHCl3- and CH2Cl2-vapor atmosphere processes was investigated (Fig. 11). It was found that the poly(OD2-co-S) films showed good reversible switching of λabs-filmmax even in the five-time air–vapor cycles, indicating that it is a suitable polymeric functional dye material for the visualization and detection of VOHCs. Actually, one can see that the color of the as-prepared poly(OD2-co-S) films is yellow in air or THF-, PrBr- and DMF-vapor atmosphere but is transformed into orange in CH2Cl2- and CHCl3-vapor atmosphere (Fig. 12). Moreover, it is worth mentioning here that the poly(OD2-co-S) film shows a quick response to VOHCs: the color change in the poly(OD2-co-S) film by exposure to CH2Cl2- or CHCl3-vapor was completed within 30 s, and then the film recovered the original color within 10 s upon exposure to air at room temperature (see Movie S1 for poly(OD2-co-S) film upon exposure to CH2Cl2 vapor, ESI†). Evidently, this result revealed that the D–π–A type pyridinium dyes in polymer matrices can also express b-OHC based on the enhanced ICT characteristics, leading to the destabilization of the HOMO energy level and/or the stabilization of the LUMO energy level, which is induced by the intermolecular interaction between the dye and the organohalogen molecules produced by contact between them. Meanwhile, our previous work demonstrated that OD3 bearing an iodide ion (I−) as the counter anion shows an obvious b-OHC, compared to OD1 and OD2 bearing a chloride anion (Cl−) and a bromide anion (Br−), respectively (Fig. 1a).2 Thus, to improve the visualization of VOHCs by a huge change in the photoabsorption wavelength in air and the VOHC-vapor atmosphere process, further studies on b-OHC of D–π–A type pyridinium dye polymers bearing various counter anions are required.
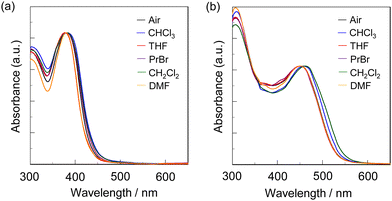 |
| Fig. 10 Photoabsorption spectra of (a) poly(NI2-co-S) film and (b) poly(OD2-co-S) film in air and solvent–vapor atmosphere. | |
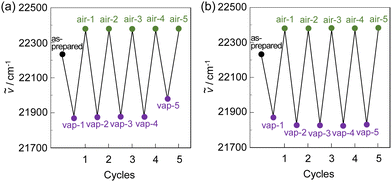 |
| Fig. 11 Reversible switching of photoabsorption maximum wavenumber (ṽ) of poly(OD2-co-S) film in air and (a) CHCl3- and (b) CH2Cl2-vapor atmosphere processes. | |
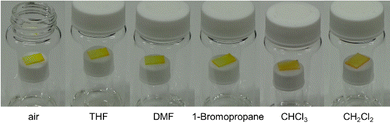 |
| Fig. 12 Photographs of poly(OD2-co-S) films in air and solvent–vapor atmosphere. | |
Conclusions
We designed and synthesized a D–π–A type pyridinium dye monomer OD2-V possessing intramolecular charge transfer (ICT) characteristics and prepared a copolymer poly(OD2-co-S) composed of OD2-V and styrene to elucidate organohalogenochromism (OHC) and to develop optical polymeric materials for the visualization and detection of volatile organohalogen compounds (VOHCs). It was found that poly(OD2-co-S) and OD2-V exhibit bathochromic shift-type OHC (b-OHC) in halogenated solvents: OD2-V and poly(OD2-co-S) show a significant bathochromic shift of ICT-based photoabsorption bands in halogenated solvents, such as dichloromethane (CH2Cl2) and dibromomethane (CH2Br2), compared with those in non-halogenated solvents. Furthermore, the drop-casted poly(OD2-co-S) film shows the bathochromic shift of the ICT-based photoabsorption band in the halogenated solvent–vapor atmosphere, that is, a visual change in color from yellow to orange upon exposure to VOHCs, including chloroform (CHCl3) and CH2Cl2. The poly(OD2-co-S) film exhibits good reversible switching of the ICT-based photoabsorption band in the air and VOHC-vapor atmosphere process. The optical sensing properties of poly(OD2-co-S) film to VOHCs, and the 1H NMR spectral measurements in non-halogenated and halogenated solvents and semi-empirical molecular calculations for OD2-V, revealed that the enhanced ICT characteristics of OD2 chromophore induced by the intermolecular interaction between the dye and the organohalogen molecules are responsible for the b-OHC of D–π–A type pyridinium dyes. Therefore, this work sufficiently elucidated the b-OHC of D–π–A type pyridinium dyes and demonstrated that polymeric organohalogenochromic dyes are one of the most promising and convenient functional dye materials for colorimetric detection of VOHCs.
Experimental
General
Melting points were measured with an AS ONE ATM-02. IR spectra were recorded using a SHIMADZU IRTracer-100 spectrometer by the ATR method. 1H and 13C NMR spectra were recorded using a Varian-500 FT NMR spectrometer. High-resolution mass spectral data were obtained using APCI and ESI with a Thermo Fisher Scientific LTQ Orbitrap XL. Photoabsorption spectra were observed with a SHIMADZU UV-3600 plus. Polymer number-average molecular weights (Mn) and molecular weight distributions (Mw/Mn) were determined by size exclusion chromatography (SEC) at 40 °C using a SHIMADZU Prominence-i LC-2030 plus with a guard column (LF-G, Shodex), two series-connected columns (LF-804, Shodex), a UV detector, and a differential refractive index detector (RID-20A). THF was used as the eluent, and polystyrene standards were used to calibrate the SEC system.
Synthesis
N,N-Diphenyl-7-(pyridin-4-yl)-9-vinyl-9H-carbazol-2-amine (NI2-V).
A solution of NI2 (0.765 g, 1.86 mmol) in dry toluene (30.6 mL) and 1,2-dimethoxyethane (DME) (6.5 mL) under a nitrogen atmosphere was added to a 1.6 M hexane solution of n-BuLi (1.74 mL, 2.79 mmol) dropwise and stirred for 30 min at room temperature. Then, bis(dibenzylideneacetone)palladium(0) (Pd(dba)2) (0.043 g, 0.074 mmol), a 10 wt% hexane solution of tri-t-butyl phosphine (0.444 mL, 0.149 mmol), and a 1.0 M ethyl ether solution of vinyl bromide (5.58 mL, 5.58 mmol) were added, and the solution was stirred at 90 °C for 2 h. The resulting mixture was cooled to room temperature, passed through a short column with silica gel (ethyl acetate as eluent) and concentrated. The residue was chromatographed on silica gel (dichloromethane
:
ethyl acetate = 4
:
1 as eluent) to give NI2-V (0.522 g, yield 64%) as a yellow solid; m.p. 104–106 °C; FT-IR (ATR): ṽ = 3032, 1589, 1487, 1463, 1433, 1273, 1230, 800, 750, 694 cm−1; 1H NMR (500 MHz, acetone-d6): 5.13 (dd, J = 1.1 and 9.3 Hz, 1H), 5.47 (dd, J = 1.1 and 15 Hz, 1H), 7.04 (dd, J = 1.9 and 8.4 Hz, 1H), 7.06–7.10 (m, 2H), 7.14–7.16 (m, 4H), 7.31–7.35 (m, 4H), 7.46 (d, J = 1.7 Hz, 1H), 7.49 (dd, J = 9.3 and 16 Hz, 1H), 7.72 (dd, J = 1.6 and 8.1 Hz, 1H), 7.80 (dd, J = 1.7 and 4.5 Hz, 2H), 8.11 (d, J = 8.4 Hz, 1H), 8.13 (d, J = 1.0 Hz, 1H), 8.22 (d, J = 8.4 Hz, 1H), 8.65 (dd, J = 1.7 and 4.4 Hz, 2H) ppm; 13C NMR (125 MHz, acetone-d6): δ = 103.20, 107.05, 109.74, 119.14, 119.83, 120.85, 121.17, 122.20, 122.46, 123.96, 125.15, 125.52, 130.29, 130.51, 136.33, 141.24, 141.97, 148.51, 148.98, 149.16, 151.22 ppm; HRMS (APCI): m/z (%): [M + H]+ calcd for C31H24N3, 438.19647; found 438.19632.
1-Butyl-4-(7-(diphenylamino)-9-vinyl-9H-carbazol-2-yl)pyridinium bromide (OD2-V).
A solution of NI2-V (0.200 g, 0.457 mmol) and 1-bromobutane (3.95 mL, 36.6 mmol) in dry acetonitrile (50 mL) was stirred for 16 h at 80 °C. After concentrating under reduced pressure, the resulting residue was subjected to reprecipitation from CH2Cl2/hexane to give OD2-V (0.206 g, yield 78%) as orange solids; m.p. 130–132 °C; FT-IR (ATR): ṽ = 3034, 1616, 1523, 1487, 1465, 1433, 1315, 1263, 802, 752, 694 cm−1; 1H NMR (500 MHz, acetone-d6): 1.00 (t, J = 7.5 Hz, 3H), 1.46–1.54 (m, 2H), 2.10–2.17 (m, 2H), 4.92 (t, J = 7.5 Hz, 2H), 5.16 (dd, J = 1.1 and 9.2 Hz, 1H), 5.49 (dd, J = 1.2 and 16 Hz, 1H), 7.05 (dd, J = 1.9 and 8.5 Hz, 1H), 7.10–7.14 (m, 2H), 7.17–7.19 (m, 4H), 7.34–7.37 (m, 4H), 7.44 (d, J = 1.8 Hz, 1H), 7.58 (dd, J = 9.2 and 15.9 Hz, 1H), 8.01 (dd, J = 1.7 and 8.3 Hz, 1H), 8.15 (d, J = 8.5 Hz, 1H), 8.31 (d, J = 8.2 Hz, 1H), 8.54 (d, J = 0.1 Hz, 1H), 8.75 (d, J = 7 Hz, 2H), 9.38 (d, J = 7.1 Hz, 2H) ppm; 13C NMR (125 MHz, CDCl3): δ = 13.68, 19.54, 33.57, 60.80, 104.89, 105.33, 109.76, 117.58, 118.23,120.36, 120.76, 121.81, 123.70, 124.61, 124.99, 127.85, 129.07, 129.55, 129.58, 140.25, 142.24, 144.59, 147.74, 149.00, 156.78 ppm; HRMS (ESI†): m/z (%): [M–Br]+ calcd for C35H32N3, 494.25907; found 494.25894.
Preparation of poly(NI2-co-S)
NI2-V (0.378 g, 0.864 mmol), styrene (9.40 mL, 99.6 mmol), and azobis(isobutyronitrile) (0.071 mg, 0.43 mmol) were degassed with nitrogen bubbling, and then the solution was stirred for 5 h at 60 °C under nitrogen atmosphere. The reaction mixture was dissolved in dichloromethane. The dichloromethane solution was poured into n-hexane, and the resulting precipitate was collected to give poly(NI2-co-S) (0.902 g, yield 8%) as a white solid; m.p. 131–150 °C; FT-IR (ATR): ṽ = 3024, 1600, 1492, 1452, 1028, 906, 754, 696 cm−1; 1H NMR (500 MHz, THF-d8): 1.49 (br, CH2-C
for styrene and NI2-V unit), 1.89 (br, CH-C![[H with combining low line]](https://www.rsc.org/images/entities/char_0048_0332.gif)
for styrene and NI2-V unit), 6.61 (br, C
on Ph for styrene), 7.07 (br, C
on Ph for styrene), 7.89 (br, aromatic protons for NI2-V unit), and 8.57 (br, C
on pyridine ring for NI2-V unit) ppm. The molar ratio (x/y) of styrene unit (x) and NI2-V unit (y) and the weight percentage (wt%) of NI2-V unit were determined to be ca. 667 and ca. 0.62 wt%, respectively, from the 1H NMR spectrum; SEC Mn = 59
200, Mw/Mn = 1.55.
Preparation of poly(OD2-co-S)
Poly(NI2-co-S) (0.500 g, 8.5 × 10−3 mmol) and 1-bromobutane (5.0 mL, 47 mmol) were stirred for 1 day at 100 °C. The reaction mixture was concentrated, and the resulting residue was dissolved in dichloromethane. The dichloromethane solution was poured into n-hexane, and the resulting precipitate was collected to give poly(OD2-co-S) (0.423 g, yield 84%) as a yellow solid; m.p. 140–174 °C; FT-IR (ATR): ṽ = 3024, 1600, 1492, 1452, 1028, 906, 754, 694 cm−1; 1H NMR (500 MHz, THF-d8): 1.49 (br, CH2-C
for styrene and OD2-V unit), 1.90 (br, CH-C![[H with combining low line]](https://www.rsc.org/images/entities/char_0048_0332.gif)
for styrene and OD2-V unit), 5.01 (br, N-C
2-CH2 of butyl group for OD2-V unit), 6.61 (br, C
on Ph for styrene), 7.07 (br, C
on Ph for styrene), 7.78 (br, aromatic protons for OD2-V unit), and 9.56 (br, C
on pyridinium ring for OD2-V unit) ppm.
Preparation of poly(NI2-co-MMA) and poly(OD2-co-S) films
A solution of poly(NI2-co-S) (0.100 g) in THF (2.0 mL) or poly(OD2-co-S) (0.075 g) in THF (1.5 mL) was stirred for 15 h at room temperature. To prepare a polymer film, 250 μL of the polymer solution was drop-casted on a treated quartz glass substrate, where the surfaces of the quartz glass substrate were treated with piranha solution and then modified with a hydrophobic monolayer using 1,1,1,3,3,3-hexamethyldisilazane (HMDS) to obtain stable polymer thin films prior to drop casting. The drop-casted films were dried under a nitrogen atmosphere for 30 min. The resulting poly(NI2-co-S) or poly(OD2-co-S) films were exposed to solvent vapor for 2 min (in the solvent–vapor atmosphere) to perform the photoabsorption spectral measurements with a calibrated integrating sphere system.
Computational methods
The semi-empirical calculations were carried out with the WinMOPAC Ver. 3.9 package (Fujitsu, Chiba, Japan). The geometry calculation of compounds in the ground state was performed using the AM1 method. The geometry was completely optimized (keyword PRECISE) by the eigenvector following routine (keyword EF). The experimental absorption spectra of the compound were compared with their absorption data using the semi-empirical method INDO/S (intermediate neglect of differential overlap/spectroscopic) and the SCRF Onsager Model. All INDO/S calculations were performed using single excitation full SCF/CI (self-consistent field/configuration interaction), which includes the configuration with one electron excited from any occupied orbital to any unoccupied orbital, where 225 configurations were considered [keyword CI (15 15)].
Author contributions
Y. O. conceived the project and directed the experimental studies. K. I. conducted the preparation and analysis of polymer. K. K. performed almost all the experiments and carried out MO calculations. The manuscript was written through contributions of all authors.
Conflicts of interest
There are no conflicts to declare.
Acknowledgements
This work was supported by Grant-in-Aid for Challenging Research (Pioneering) from the Japan Society for the Promotion of Science (JSPS) KAKENHI Grant Number 23K18522 and by Fuso Innovative Technology Fund.
References
- T. Higashino and Y. Ooyama, Chem. Lett., 2021, 50, 1530–1533 CrossRef CAS.
- Y. Ooyama, Y. Oda, T. Mizumo and J. Ohshita, Tetrahedron, 2013, 69, 1755–1760 CrossRef CAS.
- Y. Ooyama, K. Kushimoto, Y. Oda, D. Tokita, N. Yamaguchi, S. Inoue, T. Nagano, Y. Harima and J. Ohshita, Tetrahedron, 2012, 68, 8577–8580 CrossRef CAS.
- Y. Ooyama, R. Asada, S. Inoue, K. Komaguchi, I. Imae and Y. Harima, New J. Chem., 2009, 33, 2311–2316 RSC.
-
C. Reichardt, Solvents and Solvent Effects in Organic Chemistry, Wiley-VCH, Weinheim, 2003 Search PubMed.
- I. Bolz, D. Schaarschmidt, T. Rüffer, H. Lang and S. Spange, Angew. Chem., Int. Ed., 2009, 48, 7440–7443 CrossRef CAS PubMed.
- L. Giordano, V. V. Shvadchak, J. A. Fauerbach, E. A. Jares-Erijman and T. M. Jovin, J. Phys. Chem. Lett., 2012, 3, 1011–1016 CrossRef CAS PubMed.
- M. Vidal, C. Pastenes, M. Caroli Rezende, C. Aliaga and M. Domínguez, Org. Chem. Front., 2019, 6, 3896–3901 RSC.
- J. A. Balam-Villarreal, B. J. López-Mayorga, D. Gallardo-Rosas, R. A. Toscano, M. P. Carreón-Castro, V. A. Basiuk, F. Cortés-Guzmán, J. G. López-Cortés and M. C. Ortega-Alfaro, Org. Biomol. Chem., 2020, 18, 1657–1670 RSC.
- V. Castro-Castillo, J. Gajardo, C. Sandoval-Altamirano, E. Gratton, S. Sanchez, L. Malacrida and G. Gunther, J. Mater. Chem. B, 2020, 8, 88–99 RSC.
- T. Mukherjee, R. J. Martinez-Sanchez, K. T. Fam, S. Bou, L. Richert, D. Garnier, Y. Mély, S. Kanvah, A. S. Klymchenko and M. Collot, Mater. Chem. Front., 2021, 5, 2459–2469 RSC.
- M. Piejko, B. Patrahau, K. Joseph, C. Muller, E. Devaux, T. W. Ebbesen and J. Moran, J. Am. Chem. Soc., 2023, 145, 13215–13222 CrossRef CAS PubMed.
- T. Katoh, Y. Inagaki and R. Okazaki, J. Am. Chem. Soc., 1998, 120, 3623–3628 CrossRef CAS.
- T. Katoh, K. Ogawa, Y. Inagaki and R. Okazaki, Bull. Chem. Soc. Jpn., 1997, 70, 1109–1114 CrossRef CAS.
- B. J. Coe, J. A. Harris, J. J. Hall, B. S. Brunschwig, S.-T. Hung, W. Libaers, K. Clay, S. J. Coles, P. N. Horton, M. E. Light, M. B. Hursthouse, J. Garín and J. Orduna, Chem. Mater., 2006, 18, 5907–5918 CrossRef CAS.
- F. Dumur, C. R. Mayer, E. Dumas, F. Miomandre, M. Frigoil and F. Sécheresse, Org. Lett., 2008, 10, 321–324 CrossRef CAS.
- T. Kolev, T. Tsanev, S. Kotov, H. Mayer-Figge, M. Spiteller, W. S. Sheldrick and B. Koleva, Dyes Pigm., 2009, 82, 95–101 CrossRef CAS.
- T. Dentani, Y. Kubota, K. Funabiki, J. Jin, T. Yoshida, H. Minoura and M. Matsui, New J. Chem., 2009, 33, 93–101 RSC.
- A. Baheti, P. Singh and K. R. J. Thomas, Dyes Pigm., 2011, 88, 195–203 CrossRef CAS.
- M.-A. Tehfe, F. Dumur, B. Graff, F. Morlet-Savary, D. Gigmes, J.-P. Fouassier and J. Lalevée, Polym. Chem., 2013, 4, 3866–3875 RSC.
- M. Hagimori, N. Mizunuma, K. Yokota, Y. Nishimura, M. Suzuta, C.-K. Tai, B.-C. Wang, S.-L. Wang, T.-L. Shih, H.-H. Wu, K. Kawashima, S. Kawashima and Y. Tominaga, Dyes Pigm., 2012, 92, 1069–1074 CrossRef CAS.
- M. Fakis, P. Hrobárik, E. Stathatos, V. Giannetas and P. Persephonis, Dyes Pigm., 2013, 96, 304–312 CrossRef CAS.
- A. Gandioso, M. Palau, R. Bresolí-Obach, A. Galindo, A. Rovira, M. Bosch, S. Nonell and V. Marchán, J. Org. Chem., 2018, 83, 11519–11531 CrossRef CAS.
- M. Vidal, M. C. Rezende, C. Pastene, C. Aliaga and M. Domínguez, New J. Chem., 2018, 42, 4223–4231 RSC.
- A. Cesaretti, P. Foggi, C. G. Fortuna, F. Elisei, A. Spalletti and B. Carlotti, J. Phys. Chem. C, 2020, 124, 15739–15748 CrossRef CAS.
- S. Karmakar, A. Ambastha, A. Jha, A. Dharmadhikari, J. Dharmadhikari, R. Venkatramani and J. Dasgupta, J. Phys. Chem. Lett., 2020, 11, 4842–4848 CrossRef CAS.
- G. Prévot, T. Bsaibess, J. Daniel, C. Genevois, G. Clermont, I. Sasaki, S. Marais, F. Couillaud, S. Crauste-Manciet and M. Blanchard-Desce, Nanoscale Adv., 2020, 2, 1590–1602 RSC.
- A. Kayama, A. Shimizu and R. Shintani, Synthesis, 2021, 4042–4047 CAS.
- W. Zeng and J. Wu, Mater. Chem. Front., 2019, 3, 2668–2672 RSC.
- T.-C. Lin, Z.-Y. Liu, S.-H. Liu, I. O. Koshevoy and P.-T. Chou, JACS Au, 2021, 1, 282–293 CrossRef CAS PubMed.
- R. W. C. Li, L. Ventura, J. Gruber, Y. Kawano and L. R. F. Carvalho, Sens. Actuators B, 2008, 131, 646–651 CrossRef CAS.
- T. Kakuta, R. Nakanishi, T. Ogoshi and T. Yamagishi, RSC Adv., 2020, 10, 12695–12698 RSC.
- R. M. El-Shishtawy, F. A. M. Al-Zahrani, S. M. Afzal, M. A. N. Razvi, Z. M. Al-amshany, A. H. Bakry and A. M. Asiri, RSC Adv., 2016, 6, 91546–91556 RSC.
- K. Ponnusamy, S. Chellappan, C. M. Singaravelu and J. Kandasamy, J. Lumin., 2018, 202, 253–262 CrossRef CAS.
- M. Shibayama, Y. Uehashi, S. Ajioka, Y. Kubota, T. Inuzuka and K. Funabiki, New J. Chem., 2023, 47, 5262–5269 RSC.
- W. Yin, H. Li, A. S. R. Chesman, B. Tadgell, A. D. Scully, M. Wang, W. Huang, C. R. McNeill, W. W. H. Wong, N. V. Medhekar, P. Mulvaney and J. J. Jasieniak, ACS Nano, 2021, 15, 1454–1464 CrossRef CAS.
- I. Lee, J. E. Kwon, Y. Kang, K. C. Kim and B.-G. Kim, ACS Sens., 2018, 3, 1831–1837 CrossRef CAS.
- M. M. Bordbar, J. Tashkhourian and B. Hemmateenejad, ACS Sens., 2019, 4, 1442–1451 CrossRef CAS PubMed.
- N. Meher, D. Barman, R. Parui and P. K. Lyer, J. Mater. Chem. C, 2022, 10, 10224–10254 RSC.
- A. D. Tjandra, A.-H. Pham and R. Chandrawati, Chem. Mater., 2022, 34, 2853–2876 CrossRef CAS.
- T. Eaidkong, R. Mungkarndee, C. Phollookin, G. Tumcharern, M. Sukwattanasinitt and S. Wacharasindhu, J. Mater. Chem., 2012, 22, 5970–5977 RSC.
- S. Dolai, S. K. Bhunia, S. S. Beglaryan, S. Kolusheva, L. Zeiri and R. Jelinek, ACS Appl. Mater. Interfaces, 2017, 9, 2891–2898 CrossRef CAS.
- H. Lin, M. Jang and K. S. Suslick, J. Am. Chem. Soc., 2011, 133, 16786–16789 CrossRef CAS.
- C. Lu, S. Liu, J. Xu, Y. Ding and G. Ouyang, Anal. Chim. Acta, 2016, 902, 205–211 CrossRef CAS PubMed.
- P. Chen, Y. Tseng, Y. Chuang and J. Chen, J. Chromatogr. A, 2015, 1395, 41–47 CrossRef CAS PubMed.
- S. Das, P. Sharma, P. M. Badani and R. K. Vatsa, RSC Adv., 2015, 5, 8887–8894 RSC.
- S. Ampuero and J. O. Bosset, Sens. Actuators B, 2003, 94, 1–12 CrossRef CAS.
- F. F. Awwadi, R. D. Willett, K. A. Peterson and B. Twamley, Chem. – Eur. J., 2006, 12, 8952–8960 CrossRef CAS.
- F. F. Awwadi, R. D. Willet, K. A. Peterson and B. Twamley, J. Phys. Chem. A, 2007, 111, 2319–2328 CrossRef CAS PubMed.
- R. D. Willett, F. F. Awwadi and R. Butcher, Cryst. Growth Des., 2003, 3, 301–311 CrossRef CAS.
- H. Matter, M. Nazaré, S. Güssregen, D. W. Will, H. Schreuder, A. Bauer, M. Urmann, K. Ritter, M. Wagner and V. Wehner, Angew. Chem., Int. Ed., 2009, 48, 2911–2916 CrossRef CAS.
- H. G. Wallnoefer, T. Fox, K. R. Liedl and C. S. Tautermann, Phys. Chem. Chem. Phys., 2010, 12, 14941–14959 RSC.
- A. Forni, S. Pieraccini, S. Rendine, F. Gabas and M. Sironi, ChemPhysChem, 2012, 13, 4224–4234 CrossRef CAS PubMed.
- A. Forni, S. Pieraccini, S. Rendine and M. Sironi, J. Comput. Chem., 2014, 35, 386–394 CrossRef CAS.
- I. S. Youn, D. Y. Kim., W. J. Cho, J. M. L. Madridejos, H. M. Lee, M. Kolaski, J. Lee, C. Baig, S. K. Shin, M. Filatov and K. S. Kim, J. Phys. Chem. A, 2016, 120, 9305–9314 CrossRef CAS PubMed.
- Y. Ooyama, S. Inoue, T. Nagano, K. Kushimoto, J. Ohshita, I. Imae, K. Komaguchi and Y. Harima, Angew. Chem., Int. Ed., 2011, 50, 7429–7433 CrossRef CAS.
-
CRC Handbook of Chemistry and Physics, ed. J. R. Rumble, CRC Press, Boca Raton, 103rd edn, 2022 Search PubMed.
- J. P. Cerón-Carrasco, D. Jacquemina, C. Laurence, A. Planchat, C. Reichardt and K. Sraïdi, J. Phys. Org. Chem., 2014, 27, 512–518 CrossRef.
- J. O. Morley, R. M. Morley, R. Docherty and M. H. Charlton, J. Am. Chem. Soc., 1997, 119, 10192–10202 CrossRef CAS.
- P. Jacques, J. Phys. Chem., 1986, 90, 5535–5539 CrossRef CAS.
|
This journal is © The Royal Society of Chemistry 2024 |