DOI:
10.1039/D3LC00876B
(Critical Review)
Lab Chip, 2024,
24, 1293-1306
Progress in developing microphysiological systems for biological product assessment
Received
13th October 2023
, Accepted 26th December 2023
First published on 17th January 2024
Abstract
Microphysiological systems (MPS), also known as miniaturized physiological environments, have been engineered to create and study functional tissue units capable of replicating organ-level responses in specific contexts. The MPS has the potential to provide insights about the safety, characterization, and effectiveness of medical products that are different and complementary to insights gained from traditional testing systems, which can help facilitate the transition of potential medical products from preclinical phases to clinical trials, and eventually to market. While many MPS are versatile and can be used in various applications, most of the current applications have primarily focused on drug discovery and testing. Yet, there is a limited amount of research available that demonstrates the use of MPS in assessing biological products such as cellular and gene therapies. This review paper aims to address this gap by discussing recent technical advancements in MPS and their potential for assessing biological products. We further discuss the challenges and considerations involved in successful translation of MPS into mainstream product testing.
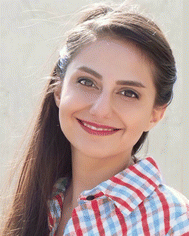 Mona Mansouri | Mona Mansouri is a postdoctoral researcher in the Office of Therapeutic Products in the Center for Biologics Evaluation and Research at FDA, where she studies the microenvironment's impact on cellular function. Specifically, her work focuses on understanding how an inflamed microenvironment influences the differentiation capacity of mesenchymal stem cells for treating cartilage defects. Additionally, she investigates the impact of matrix stiffness on the effectiveness of natural killer cells against tumors, revealing therapeutic potential for cancer treatment. Mona holds a Ph.D. in Chemical and Biomolecular Engineering from the University of Akron, where she explored replicating organ-level responses by manipulating the cell microenvironment with biomaterials and fluidic devices. |
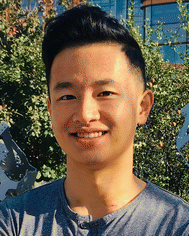 Johnny Lam | Johnny Lam is a biomedical engineer in the Office of Therapeutic Products in the Center for Biologics Evaluation and Research at FDA, where he performs both lead product review and research activities. Johnny's main research interests involve studying complex cell-based therapies and how their product quality attributes relate to functionally relevant bioactivities. His research focuses on the development and adaptation of wide-ranging microphysiological systems as platforms to evaluate various functional outcomes of various cell types toward improving the quality and potency of manufactured cell-based products. Johnny received his Ph.D. in Bioengineering in 2015 at Rice University, where he developed and evaluated injectable multi-layered hydrogel composites for cell and controlled growth factor delivery for in vivo orthopedic tissue repair. |
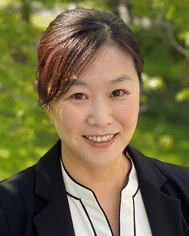 Kyung Sung | Kyung Sung is the Chief of the Cellular and Tissue Therapies Branch in the Office of Therapeutic Products in the Center for Biologics Evaluation and Research, US Food and Drug Administration. She is responsible for overseeing both the regulatory science research programs and the regulatory review process for cell and tissue therapeutic products and medical devices. The research team focuses on studying the impact of interactions between living cells and biomaterials on product quality. They achieve this by developing innovative quantitative assays using a range of biomedical engineering tools. The main objective is to gain a comprehensive understanding of how these interactions impact the production and characterization of cellular products used in regenerative medicine. She obtained her Ph.D. in Chemical Engineering from the University of Michigan, Ann Arbor, and subsequently finished her postdoctoral training at the University of Wisconsin, Madison. Prior to joining the FDA in 2015, she gained experience as a patent examiner in Biotechnology at the US Patent and Trademark Office. |
Introduction
Over the past few decades, researchers have made remarkable progress in developing accessible and informative in vitro models that can potentially reduce, refine, or even replace the use of traditional animal models in nonclinical studies. These models also offer new approaches for testing medical products. In addition to ethical concerns, the main reason for the limitations of animal models is their inherent differences when compared to humans.1 For example, a report indicates that drug failures primarily result from either a lack of efficacy (56%) or safety issues (28%) where failures are attributed to an insufficient therapeutic index, which is a comparison of the amount of a therapeutic agent that causes the therapeutic effect to the amount that causes toxicity.2 These disparities may be attributed to variations in drug-metabolizing enzyme activity among species.3 Even when a model seems to accurately represent a disease, variations in finer details such as cellular receptors and immune signaling pathways can adversely affect the assessment of potential therapies.4 The FDA Modernization Act 2.0 was passed on December 29th, 2022 as part of the broader Consolidated Appropriations Act to acknowledge the emergence of alternative methods that can offer new insights and additional information alongside traditional methods. This amendment to Section 505 of the Federal Food, Drug, and Cosmetic Act (21 U.S.C. 355) replaces the term “pre-clinical tests (including tests on animals)” with the term “nonclinical tests”. Although the definition of nonclinical tests, as stated in the act, still encompasses animal tests, it is important to note that the term ‘nonclinical test’ include various alternative methods. These alternative methods include cell-based assays, microphysiological systems, and bioprinted, or computer models.5 This clarification comes as a response to the evolving discussions regarding the limitations and ethical issues surrounding the use of animal models. It is important to note that regulatory authorities and industries have been accepting alternative methods that are scientifically well qualified. For example, the FDA guidance “Nonclinical safety evaluation of the immunotoxic potential of drugs and biologics”6 recommends that, due to immunological differences in expression and sensitivity between humans and nonclinical test species, additional safety considerations may be required for therapeutics intended to modulate the immune response, which can lead to adverse reactions such as excessive cytokine release. Therefore, the guidance document recommends that sponsors provide specific immune system pharmacological data using human cells before initiating clinical trials.
Studying cells grown in 2D has provided valuable insights into intracellular processes, but this approach has limitations when it comes to understanding the complex interactions that occur between cells, the extracellular matrix (ECM), tissues, and organs. To address these limitations, developing 3D cell cultures, such as MPS, capable of creating specific physiological conditions, tissue-like perfusion, mechanical cues, and chemical environments have become important. It is worth noting that there are different definitions of MPS that have been proposed, each providing different levels of coverage. For example, the FDA's Alternative Methods Working Group (AMWG) provides a broad definition of MPS. They describe it as in vitro platforms consisting of human or animal cells, tissue/organ-derived explants, and/or self-assembling organoids that are designed to create microenvironmental niches that facilitate appropriate biochemical, electrical, and mechanical responses at the organ/tissue level.7 It also defines organ-on-chip as a subset of MPS consisting of a miniaturized physiological environment engineered to yield functional tissue units. The Innovation & Quality Microphysiological Systems (IQ MPS) Affiliate, which consists of pharmaceutical and biotechnology companies, uses the term, complex in vitro models (CIVM). CIVM refers to models of broad complexity, including micropatterned co-culture systems, 3D microtissues such as spheroids and organoids, 3D bioprinted tissues, and/or organ-on-chip.8 The IQ MPS affiliate defines MPS as a model representing the more complex side of the CIVM spectrum, such as 3D bioprinted tissues and organ-on-chip. The applications of MPS are vast, encompassing the modeling of both healthy and diseased conditions in human biology, as well as translational research aimed at assessing the efficacy, specificity, and potency of therapeutic candidates, such as pharmaceutical drugs or biological products.9 Although MPS have made significant progress in developing models that closely mimic in vivo conditions, it is important to acknowledge that they still face certain challenges. These challenges include the use of complex culture techniques, difficulties in imaging caused by cell growth in the z-direction, problems with extended cell culture, and the development of necrosis in the engineered tissue due to the lack of a fully functional vasculature within it.10,11 Researchers aim to minimize the need for animal testing; however, it is probable that some degree of it will remain necessary to support the development of various medical products before they can be brought to market.
The development of MPS was motivated by the need to enhance the effectiveness, efficiency, and safety of pharmaceutical development and testing. Pilot studies have demonstrated this in drug pharmacokinetics (PKs), including bioavailability prediction, investigation of low-clearance compounds, and drug–drug interactions studies.12 One fascinating example is the use of in vitro lung models for drug testing that aim to replicate the complexities of the human lung such as lung epithelial cells, vascular endothelial cells, an air-liquid interface, and cyclic mechanical stretching. These lung models have demonstrated substantial utility as relevant analytical tools in clinically relevant research. For instance, they recapitulate key aspects of COVID-19-induced conditions, such as fibrosis and inflammation, and assist in identifying potential therapy candidates.13 Another noteworthy application of MPS involves the study of drug toxicity, particularly within heart and liver models. Cardiotoxicity accounts for approximately one-third of pharmaceutical withdrawals due to safety concerns, while the liver plays a crucial role in drug metabolism, significantly influencing the overall effect of drug. The combination of heart and liver tissue in vitro models has the potential to improve the predictability and efficiency of drug toxicity screening.14,15 Several research laboratories, such as those in the laboratories in the FDA Division of Applied Regulatory Science in the Center for Drug Evaluation and Research (CDER), currently focus on the evaluation of MPS for generating consistent experimental results in drug toxicity, metabolism, and pharmacokinetics assays for specific contexts of use.16–18
The application of MPS extends beyond screening or testing of pharmaceuticals. There are ongoing efforts to develop alternative platforms for evaluating different types of products, such as biological products. As per the definition outlined by the Public Health Service (PHS) Act, biological products refer to a wide range of substances employed to prevent, treat, or cure of human diseases. These substances include viruses, serums, toxins, vaccines, blood derivatives, and proteins/peptides.19 In contrast to well-defined chemically synthesized drugs, biological products, particularly those involving human cells, tissues, or ex vivo gene-modified cells, exhibit inherent heterogeneity. It is important to note that these products are often influenced by variations in the manufacturing process, wherein even minor modifications can substantially affect their quality and effectiveness.8 These challenges underscore the importance of improving testing methods to ensure that biological products are properly evaluated with greater sensitivity, accuracy, and predictability. MPS technologies can be beneficial in testing various biological products, spanning from simple isolated and expanded cells (e.g., autologous chondrocytes for cartilage repair) to more intricate genetically modified cells (e.g., gene-edited allogeneic CAR T cells transduced with a viral vector). In the following sections, we discuss advanced technologies that are used to integrate MPS for testing biological products in specific contexts of use. Furthermore, we discuss important technical considerations that are necessary for effectively incorporating these technologies into the development and testing processes of biological products.
Technical advancements in developing MPS
When discussing MPS, the organ-on-a-chip is the most widely recognized system. However, it is essential to acknowledge that there is a wide range of model systems that may fall under this umbrella term depending on the specific definition being used. The overarching goal of these technologies is to meticulously control the behavior of cells within the system, thus facilitating the generation of more pertinent and precise data for various applications. In this review article, we categorized MPS into four distinct technologies as illustrated in Fig. 1. It's worth highlighting that these technological advancements are constantly evolving, and the focus of this section is to spotlight the latest developments in technology.
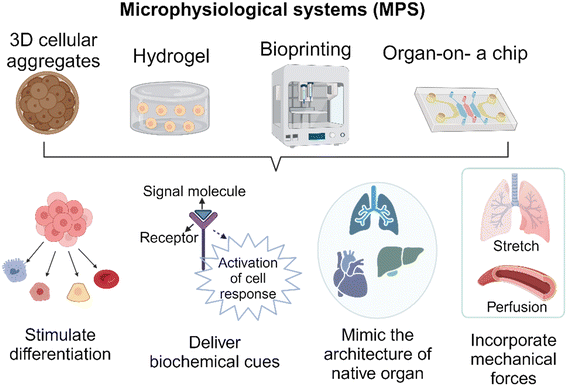 |
| Fig. 1 Schematic illustration of four MPS technologies discussed in this review and their applications in creating a biomimetic environment through the stimulation of differentiation, delivery of biochemical cues, mimicry of native organ architecture, and incorporation of mechanical forces. | |
A) Organoids/cellular aggregates
In comparison to 2D models, spheroids or cellular aggregates serve as 3D in vitro platforms capable of capturing biological complexities. Cellular aggregates are not only utilized as a testing platform, but they are also employed in the development of implantable biological products. The preservation and activation of cellular functions within 3D niche microenvironments are of utmost importance during the development of cellular aggregates. Complex interactions between ECM molecules play a significant role in this regard.20 The study conducted by Choi et al.21 investigated the effectiveness of a FGF2-tethered substrate in improving the survival of transplanted adipose-derived stem cell spheroids (FECS-Ad) as a mean to mitigate the challenge of low cell survival in cell therapy. Additionally, it was observed that the upregulation of tissue inhibitor of metalloproteinase 1 (TIMP1) had a significant role in improving the survival and therapeutic efficacy of transplanted FECS-Ad in a mouse model of critical limb ischemia. Moreover, when developing a testing platform, it is possible to generate spheroids from primary cells that have been isolated from patients. This allows for the replication of specific pathological conditions, thereby creating a model that is specific to each patient.22
Organoids are another type of cellular aggregates that are typically derived from adult stem cells (ASCs), embryonic stem cells (ESCs), or induced pluripotent stem cells (iPSCs). The formation of organoids involves providing suitable physical and biochemical cues that stimulate differentiation and maturation of organ-like structures and characteristics.23 To facilitate the development of larger and more intricate tissues, it is necessary for stem cell aggregates/organoids to undergo a transformation into a complex system with heterogeneous components and cell-type patterns.24 Recently, researchers developed a three-part system that involved organoids resembling the cerebral cortex and spinal cord, along with a 3D spheroid of skeletal muscle. This system demonstrated the successful connection of corticofugal projection neurons with spinal motor neurons, resulting in the formation of neuromuscular contacts capable of inducing muscle twitching upon cortical neuron stimulation.25 The size of organoids is another factor that contributes to the maturity. Smaller free-floating organoids tend to not fully mature,26 whereas larger ones create necrotic cores, which in turn restricts their progression into mature stages. The use of endothelial-lined vessels and dynamic culture conditions can help eliminate the necrosis observed in these models. This approach also allows the model to mature into a larger and more complex construct.27 In addition, flattened organoids were developed to provide adequate nutrient and oxygen supply, which helps prevent conditions such as hypoxia and necrosis.28 The flattened organoids also ensure a consistent exposure to inducing factors throughout the development of organoids, resulting in a more uniform differentiation process and the formation of homogeneous tissue. To overcome the diffusion limit and develop cortical organoids that closely resemble the late-stage human cortex, the sliced neocortical organoid (SNO) system was introduced. Through this innovation, continuous growth of organoids without internal cell death was achieved, resulting in the emergence of fresh neurons and the formation of an expanded cortical plate that faithfully replicates the neocortex development seen in a human embryo during the third trimester.29 In conclusion, 3D cellular aggregates represent a remarkable advancement in biomedical research. They provide a valuable platform for studying and recapitulating the complex characteristics of human organs and diseases in vitro.
B) Cell-laden hydrogels
Cells in the human body are surrounded by 3D ECM, which have a critical role in regulating essential cellular functions such as growth, proliferation, and migration.30 Hydrogels are polymer networks that are hydrophilic and can be designed to mimic the properties of ECM. A study conducted by Mooney's research group31 developed an immunomodulatory extracellular matrix (iECM) hydrogel to enhance the immunomodulatory properties of bone marrow-derived primary human mesenchymal stromal cells (hMSCs). This system combines sustained inflammatory licensing with 3D encapsulation in hydrogels with tunable mechanical properties. This novel material allows for the investigation of its capacity to inhibit human T cell proliferation. The 3D hydrogel comprises an interpenetrating network of click-functionalized alginate and fibrillar collagen, with incorporated heparin-coated beads loaded with interferon-γ. In addition, the development of stimulus-responsive hydrogels that can provide on-demand responses in response to specific stimuli is currently ongoing. For example, Tsui et al.32 developed tissue engineering scaffolds using electroconductive hydrogels composed of reduced graphene oxide (rGO) dispersed within decellularized porcine myocardial extracellular matrix (dECM). The degree of graphene oxide (GO) was carefully adjusted to modulate the electrical and mechanical properties of the hydrogels, which in turn had a direct impact on promoting functional improvements in engineered cardiac MPS. In this experiment, human induced pluripotent stem cell-derived cardiomyocytes (hiPSC-CMs) and human bone marrow-derived stromal cells were mixed and cultured within the dECM-rGO hydrogel scaffold. The resulting cardiac tissues closely resembled the native myocardium and exhibited increased expression of genes involved in regulating contractile and electrophysiological function, including calcium-handling, action potential duration, and conduction velocity. The use of hydrogels in the construction of microfluidics-based MPS is growing. However, it is important to carefully select and modify hydrogels to ensure compatibility with the microfluidics-based system. For example, it was demonstrated that controlling hydrogel swelling is important in achieving vasculogenesis of human iPSC-derived endothelial cells (hiPSC-ECs) encapsulated in a poly (ethylene glycol) (PEG)-based hydrogel within a confined microfluidic channel. The PEG-based hydrogel, when used in a traditional cell culture setup, was able to support the formation of a vascular network. However, the hydrogel did not exhibit the same property when used in a microfluidic system. This failure was attributed to a higher level of effective polymer and crosslinking density in the constrained state compared to the unconstrained state. Incorporating PEG-grafted poly(propargyl-L-glutamate) resulted in neutral swelling properties, which allowed for the successful vascular network formation within the confined MPS.33
C) Bioprinting
The emergence of 3D bioprinting presents a remarkably viable method for crafting physiologically pertinent tissue models. This is achieved through precise cell and ECM component deposition, facilitating the development of 3D models that replicate the architecture of native tissue. Similar to the cellular aggregates discussed above, this technique serves a dual purpose. It is utilized in the construction of MPS and the development of tissue-engineered products.34 Horvath et al.35 conducted a study where they utilized a valve-based bioprinting approach to recreate the structure of the human air-blood tissue barrier. This was achieved by employing a layer-by-layer printing technique to separate the printed epithelial cell layer from the underlying endothelium using a thin basement membrane created using Matrigel™. The constructed model facilitated complex cell–cell interactions, leading to a closer resemblance to the intricate cell networks found at the air-blood barrier in living organisms, both structurally and functionally. Another example involves the promotion of alignment and elongation of cardiomyocytes. The researchers fabricated multilayer cantilevers with microgrooves using 3D micro-extrusion bioprinting to guide the growth of hiPSCs-derived cardiomyocytes.36 Through this process, the cardiomyocytes were aligned on microgrooves with a filament spacing of 60 μm, resulting in the formation of an anisotropic laminar tissue with electrical propagation similar to that of the native ventricle. Bioprinting has also gained considerable attention in assembling 3D cellular aggregates into tissue, especially for engineering complex in vitro systems, with a specific focus on the nervous system. One noteworthy study conducted by Bowser and Moore,37 focused on the development of a neural MPS by combining two distinct approaches. Initially, they fabricated spinal cord spheroids using magnetic nanoparticles, which had a size range of 300–400 μm. Subsequently, these spheroids were strategically positioned within a 3D poly-ethylene glycol diacrylate (PEGDA) hydrogel construct using magnetic bioprinting. The findings of the study demonstrated that the photopatterned hydrogel not only guided but also provided support for long-distance 3D neural projections. This led to the successful production of neural MPS.
Bioprinting is currently being utilized to develop vascularized MPS. In order to culture larger 3D tissue models, it is essential to have vasculature. However, the complexity of vasculature presents challenges when attempting to replicate it using traditional fabrication methods. Byambaa et al.38 demonstrated the creation of a biomimetic in vitro niche for engineering vasculogenic and osteogenic environments. This was achieved by utilizing gelatin methacryloyl (GelMA) hydrogel loaded with silicate nanoplatelets to create cell-laden cylinder elements. Human umbilical vein endothelial cells and bone marrow-derived human mesenchymal stromal cells were co-cultured within these elements to enhance vascular spreading. They also introduced varying concentrations of vascular endothelial growth factor (VEGF) that were chemically conjugated in the adjacent bone niches. This study demonstrates the feasibility of creating bone-like structures that include vascular channels. Thus, the utilization of bioprinting in constructing organ-on-a-chip models offers numerous advantages in comparison to the traditional method of depositing cells and hydrogels to create intricate structures in a controlled manner.39
D) Organ-on-a-chip
In recent years, there has been a remarkable surge in experimental research that utilizes organ-on-a-chip platforms. This surge is primarily due to advancements in microfluidics technologies. These technologies enable the manipulation of the cellular microenvironment, allowing for the creation of functional tissue and organ interactions on a smaller scale that more closely resemble the interactions occurring in the human body than 2D models. In addition, organ-on-a-chip technology can be tailored to accommodate high-throughput assay workflows, which are essential for evaluating a large number of potential candidates with multiple replicates. The study by Song et al.40 presents a 3D tumor model designed for in vitro testing of immune-cell-mediated cytotoxicity against colorectal cancer cells using a high-throughput injection-molded microfluidic platform. The uniformity of the devices led to reproducible experiments, allowing for 10 times more experiments compared to conventional microfluidic devices. Yu and colleagues41 also introduced a microfluidic platform called MicroVascular Injection-Molded Plastic Array 3D Culture (MV-IMPACT), to generate an array of micro-vascularized tissues for studying tumor invasion and circulating tumor extravasation. The same device was used to examine the infiltration, migration, and cytotoxic activity of NK-92 cells against HeLa cells within the collagen matrix, which was reported as a high-throughput 3D co-culture system of cytotoxic lymphocytes with cancer cells.42 Another advantage is the integration of patient-specific cells into these organ chips, enabling the development of personalized models.43 Yada et al.44 developed the microfluidic platform to investigate lymphatic spread in head and neck cancer. The study utilized a controlled tumor microenvironment (TME) consisting of cancer-associated fibroblasts, a tumor spheroid, and a lymphatic microvessel to investigate metastasis. Through this study, the researchers discovered alterations in the metabolic states of lymphatic endothelial cells and cancer cells in the TME. Additionally, they identified a previously unknown molecule called macrophage migration inhibitory factor (MIF) secreted by lymphatic endothelial cells in the TME. This platform provided valuable insights into the migration and metastasis of head and neck cancer, thereby enhancing our understanding of disease progression. In another study, researchers developed a microengineered human alveolus chip that recapitulated lung injury and immune responses caused by SARS-CoV-2, providing a representation of these processes at an organ level in a laboratory setting.45 To mimic the critical features of the human alveolar-capillary barrier, the researchers co-cultured human alveolar epithelium, microvascular endothelium, and circulating immune cells under fluidic flow conditions. Following SARS-CoV-2 (strain 107) infection, the epithelium showed higher susceptibility to the virus compared to the endothelium. Transcriptional analyses revealed activated innate immune responses in the epithelium and cytokine-dependent pathways in the endothelium. The organ-on-a-chip technology also enables the monitoring of genetic mutations in small populations of cells. Yu et al.46 conducted a study where they employed a CRISPRi-microfluidics screening platform to identify genes that have the potential to be modified to improve the secretion of r-proteins in Corynebacterium glutamicum. Thousands of single-guide RNAs from a CRISPR interference library were employed to target and suppress various genes. Another intriguing application of the organ-on-a-chip technology lies in its ability to replicate the persistent cyclic stretching encountered by specific organs using programmable pumps. An example of this concept can be found in the work of Otomo et al.,47 where they utilized electro-conjugated fluid (ECF) micropumps integrated into a microfluidic system to stretch a microporous membrane. They applied a voltage of 1.1–1.6 kV to the ECF micropump, allowing them to control the strain of the microporous membrane. The result of study demonstrated the device's capability to stretch the microporous membrane with a strain range of 5–15% at a frequency of 0.2 Hz, which closely resembles the in vivo conditions of organs. The examples discussed here demonstrate the wide range of applications for organ-on-chip technologies in various areas of research. These applications span from cancer metastasis and atherosclerosis to genetic screening and immunotherapy.
MPS-based assays for testing biological products
As stated above, the primary focus of MPS technology has been on studying how cells respond to pharmaceuticals and assessing the toxicity of various substances. There has been a recent increase in recognition of the broader range and potential applications of this technology in the analysis and characterization of biological products. In this section, we delve into the advantages and challenges associated with implementing MPS technology within the field of biological product testing. As illustrated in Fig. 2, our primary focus is on assessing the bioactivity and safety of biological products, evaluating immune responses, and assessing tumorigenicity using MPS platforms.
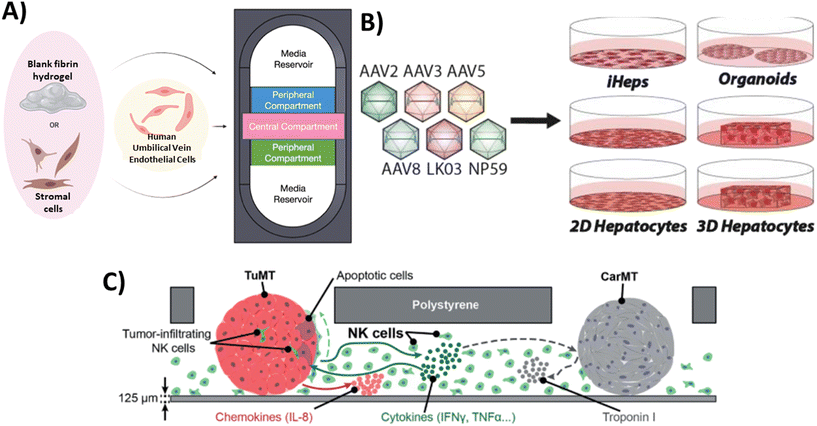 |
| Fig. 2 Examples of MPS that have been used for the assessment of biological products. A) Bioactivity testing: Endothelial cells and stromal cells (such as human lung fibroblasts and MSCs) were embedded in fibrin hydrogels and co-cultured within individual compartments of the device as a potential test system for assessing stromal cell bioactivity for inducing vasculogenesis.52 B) Safety assessment: The safety of six AAV variants in preclinical models of the human liver was studied to uncover which combination of models is the most relevant for the identification of AAV capsid variant for safe and efficient transgene delivery to primary human hepatocytes.70 C) Immune response: MPS that supports a triple culture of three-dimensional (3D) colorectal tumor microtissues, 3D cardiac microtissues, and human-derived natural killer (NK) cells in the same microfluidic network to study the possible interactions among components.71 | |
Bioactivity testing
The presence of heterogeneity in biological products can lead to significant variations in potency or bioactivity, even when biological products are produced through the same manufacturing processes. According to the regulations outlined in 21 CFR 610 and 21 CFR 600.3(s), potency refers to the specific ability or capacity of the product. This can be determined through appropriate laboratory tests or by adequately controlled clinical data obtained from the administration of the product in the manner intended, to effect a given result.48 Assessing potency is important to ensure the consistent reliability and comparability of the manufactured products in terms of clinical safety and effectiveness. However, determining potency poses a significant challenge when it comes to advancing some cellular therapy products into pivotal clinical trials. The choice of assay(s) for measuring bioactivity should ideally be based on the intended biological effect, which can be linked to the clinical response.49–51 In this context, MPS may offer a useful platform for assessing the potency of cellular products. For example, Lam et al.52 developed a physiologically relevant test platform for measuring the specific paracrine effect of MSCs in stimulating vasculogenesis, serving as a measure of their specific bioactivity (Fig. 2a). When MSCs from different donors and passages were co-cultured with human umbilical vein endothelial cells (HUVECs) as part of a cell-based assay built using an MPS platform, there were significant variability in the MSC vasculogenic potency among donors and passages. In another study, they investigated the chondrogenic capacity of MSCs derived from different donors subjected to a spectrum of manufacturing conditions. By characterizing the morphology of MSC aggregates in 3D culture, they identified potential quality attributes that could predict the cells' chondrogenic potential. This study also revealed unique morphological dynamics of MSC aggregates dependent on both the cell line and passage. By developing a simple nondestructive approach that demonstrated a strong correlation between early MSC aggregate morphology and chondrogenic potential, they provided a valuable tool for estimating MSC chondrogenic differentiation capacity at an early stage.53
Characterizing and measuring the potency of vectors in gene therapy also present certain challenges. The European Medicines Agency (EMA) guidelines state that the potency assay should evaluate the efficiency of gene transfer (infectivity/transduction/delivery) and the effectiveness of the therapeutic sequence in demonstrating its intended activity54 which aligns with recommendations from US FDA.55 In one example, researchers have developed a specialized microfluidic platform called the human retina-on-chip (RoC) model to examine the potency of various types of adeno-associated viral (AAV) vectors, a non-enveloped virus engineered to deliver DNA to target cells, using induced pluripotent stem cell-derived retinal organoids (iPSC-ROs) and retinal pigment epithelium (RPE). The system was designed to mimic physiological conditions through compartmentalization and a vasculature-like perfusion. Nutrient supply was provided through a choroidal-like vasculature. Prior to testing on the RoC model, the transduction efficacy of the AAV vectors was confirmed in an animal model in vivo. Subsequently, these vectors were tested on iPSC-ROs at different maturity levels (differentiated for 80 days as immature ROs and for 300 days as mature ones containing all cell types) to assess the impact of maturation on AAV transduction efficacy. The assessment of AAV vectors' impact on the growth and morphology of the organoids indicated that day 300 organoids did not exhibit detrimental changes after viral transduction, whereas a significant percentage of day 80 organoids showed signs of degeneration or disorganization. The presented data in this study demonstrated the potential of iPSC-based RoC as an innovative approach for evaluating AAV vectors' bioactivity within cell-based systems of different maturities, holding significant implications for advancing gene therapy research.56 Immunotherapies targeting cancers is another field that could greatly benefit from the development of targeted potency assays. For example, a key to a successful immunotherapy lies in the ability of genetically modified T cells, known as chimeric antigen receptor (CAR) T cells and T-cell receptor (TCR) T cells, to target specific antigens or markers on tumor cells. CAR T cells achieve this through direct targeting, while TCR T cells use histocompatibility leukocyte antigen (HLA) mediation for specific targeting of tumor cells. Several variables, including the variety of tumor-associated antigens, design of immunoreceptors, and the specific T-cell subset(s) to be modified, all collectively determine the therapeutic potency of these genetically engineered T cells.57 A publication by Wolf and coworkers58 has summarized various potency assays that either directly measure immune cell cytotoxic activity or use surrogate markers like cytokine release. Recently, a 3D microfluidic platform that replicates the hepatitis B virus-associated hepatocellular carcinoma (HBV-HCC) environment has been developed. This platform enabled the investigation of how human primary monocytes influence the killing efficacy of HBV-specific TCR T cells generated through several methods and analyzed the role of PD-L1/PD-1 expression during their interactions.59 Overall, these MPS-based assays may contribute to enhancing the potency evaluation strategy by incorporating additional physiologically relevant cells and structures that were not feasible in traditional assays.
Safety assessment
MPS has the potential to be used in testing the toxicity of gene therapy products, such as CAR T cells.60 CAR T cells may face challenges related to specificity and safety, particularly when there is an effort to artificially enhance the affinity of T cells in order to enhance the effectiveness of the product. This heightened affinity could lead to CAR T reactivity with self-peptides found in other tissues, potentially causing severe cross-reactive toxicity.61,62 This toxicity has the potential to lead to cardiogenic shock and has unfortunately resulted in fatalities among patients.63 Currently, in vivo models and in silico pipelines are used to assess cross-reactive toxicity.64–67 However, the development of MPS that can replicate specific tissue conditions could have potential applications in evaluating safety concerns related to cross-reactivity. In addition, MPS technology can be utilized to assess the potential toxicity risks associated with AAV vectors. While wild type AAV does not generally induce illness on its own, employing it as a gene therapy vector may introduce new risks associated with uncontrolled transgene expression and impurities during manufacturing. These risk could lead to inflammatory reactions within host tissues.68 A research group created a platform known as Human Liver Tissue Equivalent (hLTE) Platform and utilized it to introduce clinically relevant serotypes AAV5 (hLTE-5) and AAV3b (hLTE-3b).69 This approach aimed to enhance the understanding of the effectiveness as well as safety of AAV gene therapy in the human liver. Through chromogenic assays, it was revealed that the production of urea in cell culture supernatants was reduced in the groups transduced with AAV in comparison to the non-transduced hLTEs, indicating a decline in the functionality of hepatocytes. Additionally, PCR assay for human fibrosis identified the disruption of multiple genes associated with fibrosis and inflammation in both hLTE-5 and hLTE-3b. In conclusion, the study demonstrated that while AAV5 displayed greater transduction efficiency in hLTEs than AAV3b, both groups exhibited compromised self-aggregation and diminished hepatocyte functionality, warranting further attention and investigation. Similarly, Westhaus and colleagues70 conducted a functional evaluation of six AAV vectors in twelve in vitro models of the human liver including immortalized cells, iPSC-derived and primary hepatocytes, as well as primary human hepatic organoids, and in vivo models Fig. 2b. Their objective was to determine the most relevant model for selecting an AAV capsid variant that ensures safe and efficient transgene delivery to primary human hepatocytes. Their study produced different results across different in vitro liver models. For example, while AAV2 performed exceptionally well in immortalized liver cancer cell lines in terms of cell entry and transgene expression levels, its performance declined when tested in iPSC-derived and primary cells. This highlights the importance of selecting an appropriate in vitro liver model when testing AAV variants.
Immune response
Evaluating the immune response directed towards biologics holds a pivotal role in comprehensively understanding the interactions between biologics and the immune system. Liu et al.72 developed a co-culture system where CAR-T cells, tumor cells, and macrophages was incorporated in a ratio of 1
:
3
:
1. This study demonstrated that CAR-T treatment activates macrophage AIM2 inflammasomes, resulting in the release of bioactive IL-1β. Simultaneously, the release of tumor cell DNA prompted by CAR-T treatment further intensified the activation of AIM2 inflammasomes within macrophages. Interestingly, there is a specific focus on using MPS to study immune responses by replicating particular functions through the incorporation of immune components.73 For instance, a proof-of-concept study was conducted to develop an immunocompetent microphysiological system (iMPS). This system supported a triple culture of 3D colorectal tumor microtissues, 3D cardiac microtissues, and human-derived natural killer (NK) cells within the same microfluidic network (Fig. 2c). Using this system, the authors studied different aspects of the interactions between tumors and NK cells. These included studying the direct interaction between the two, examining how NK cells can kill tumors, observing how the presence of NK cells can lead to the production of pro-inflammatory chemokines and cytokines, and analyzing the impact of these interactions on healthy cardiac microtissues. The findings demonstrated that NK cells have the specific ability to kill tumors, and there were increased levels of chemokines and cytokines produced by both tumor and NK cells. This suggests that there is communication between the two and an inflammatory milieu is being created. While the viability and morphological integrity of cardiac microtissues remained largely unaffected, alterations in their beating behavior were observed. This highlights the potential of iMPS for bioactivity and early safety testing of novel cell therapy candidates, providing valuable insights into the interactions between tumor cells and NK cells, as well as their impact on cardiac microtissues.71
Understanding and mitigating immune responses against viral vectors and their transgene products is another a crucial aspect of advancing safe and effective gene therapy strategies.74 The immune responses and related adverse events are closely tied to the dosages of AAV vectors and, to some extent, the method of application. When administering low to moderate doses, like those used for treating ocular diseases or hemophilia B with an optimized transgene product, noticeable T cell responses are often absent, although they may prompt AAV capsid-specific antibody responses.75 Several research groups have employed organoids in studying potential immunotoxicity of liver-directed AAV gene delivery. These ongoing studies utilize human liver organoids comprising all major cell types present in the human liver, with frequencies that are physiologically relevant, enabling the evaluation of innate immune responses.76 The recent developments in creating 3D immune organoids show promise for studying the pathways of AAV-mediated immune activation in greater detail. Functional studies on repeated dosing and therapy-induced immunity to AAV can be conducted by integrating liver and lymphoid microtissues, such as lymph nodes and spleen, into these models. This presents a remarkable opportunity to leverage the advancements in bioengineering to enhance our comprehension of AAV immunity and explore novel strategies for mitigating safety concerns.77
Tumorigenicity
Due to the intricate and heterogeneous characteristics of biological products, it is imperative to carefully assess their tumorigenicity. Stem cell-derived products in particular, warrant significant attention in this regard due to the potential presence of mutations inherited from the parental cells used for reprogramming. One example is related to the presence of residual undifferentiated iPSCs in iPS-Cartilage, even after chondrogenic differentiation, which could lead to teratoma formation following implantation.78 Prolonged culture of MSCs have also been associated with a decrease in their ability to differentiate and an increase in chromosomal abnormalities, which can eventually lead to the development of malignant cells.79 The assessment of tumorigenicity often relies on animal models, although their sensitivity can vary depending on the animal species used and the level of immunosuppression applied. Currently, in vitro evaluations employ agar colony formation, flow cytometry, and PCR to detect impurities. However, there has been relatively less attention paid on the development of MPS for assessing the tumorigenicity risk associated with cell-based products. Cultivating human tissues in vitro on a chip and integrating immune cells provide alternative strategies for assessing tumorgenicity. However, in order to achieve this goal, it is necessary to conduct comprehensive studies and make enhancement. This is because there are various factors, such as cell type, donor variations, incubation period, and exposure profile differences, that can still introduce variability in these in vitro models. It is important to acknowledge that each cell-based product and its intended patient population possess distinct characteristics and unique properties. These properties can potentially lead to immune rejection and genetic instability after being cultured.80 Furthermore, it is important to consider that the reprogramming and differentiation processes may pose a potential risk of mutagenesis.81 This highlights the need for careful consideration of these factors when conducting tumorigenicity assessments.
Translation of MPS technology, challenges and outlook
In the previous section, we discussed recent technological advances in the field of MPS and their applications in the testing of biological products. To effectively incorporate this technology into product manufacturing, it is important to carefully evaluate these new methodologies and determine their level of readiness. The suitability of MPS for a clinical product development program can be evaluated through qualification and validation processes as shown in Fig. 3.82 Although some people may use the terms “validation” and “qualification” interchangeably, it is important to recognize the difference between the two terms, especially when considering the context of qualifying and validating new methods. The definitions provided in the draft document titled “Validation, Qualification, and Regulatory Acceptance of New Approach Methodologies”,83 drafted by the Interagency Coordinating Committee on the Validation of Alternative Methods (ICCVAM), are as follows:
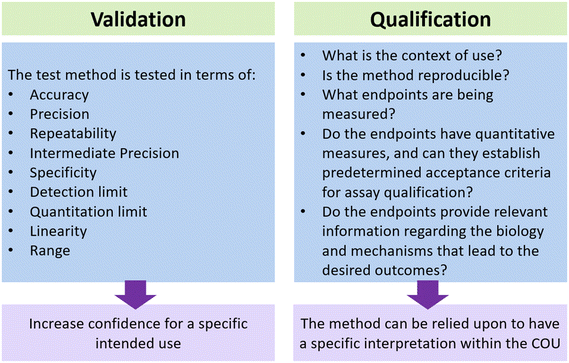 |
| Fig. 3 Illustration depicting the difference between qualification and validation. Both approaches are utilized to assess the capacity of MPS in ensuring the safety and effectiveness of biological products within the given context. | |
• Validation: the process by which the accuracy, reliability, and relevance of a procedure are established for a specific purpose. Validation for one specific purpose does not imply validation for other specific purposes. Further qualification may be needed for a particular context of use.
• Qualification: a conclusion that the results of an assessment using a validated model or assay can be relied upon to have a specific interpretation and application in product development and regulatory decision-making.
It is crucial to consider the context of use (COU) when determining the necessary level of qualification and validation. According to the ICCVAM document, the COU is a clearly articulated description delineating the manner and purpose of use for a particular method or approach. Having a more specific COU can greatly assist in the qualification process. This is because a specific COU establishes clear and straightforward requirements for validation and qualification. Consequently, the qualification process becomes more precise and specific. The scope of the COU can be expanded to include qualification of the assay for additional uses. It is important to recognize that different assays serve different purposes depending on the COU, which will serve a crucial role in determining the extent of qualification and validation required. For example, as stated in the FDA guidance document, “Potency tests for cellular and gene therapy product”,55 it is necessary to properly qualify an assay in order to determine if its performance is sufficient for ensuring the potency of the product. Before initiating a pivotal study, the potency assay must be qualified for assessing relevant specific bioactivities of the medical product; such assay(s) will need to be validated prior to submitting a licensing application (BLA) for marketing approval.
To qualify a model, it is essential to clearly define the specific research questions and experiments in which these models will be applied within the given COU. It is equally important to have a clear understanding of the aspects of the biological system being recapitulated and to determine the appropriate level of complexity required to obtain the necessary information. Scientists should aim to develop organ constructs and integrated MPS that are as simple as possible while minimizing unnecessary complexity. Rather than developing multiorgan systems that are difficult to use and analyze, some effort has been put into creating dynamic cellular microenvironments and geometrical arrangements to achieve cell polarization, direct cell–cell interaction, and the propagation of biological signaling.84 For example, immunocompetent cancer-on-chip models have allowed researchers to develop perfused structures in a tightly controlled microenvironment to observe the direct interactions of single tumor cells with immune cells.85 While not discouraging the development of complex systems, it's important to note that complex systems demand a higher investment in characterization and validation to mitigate overall variability.
Having confidence in the data obtained from MPS is important for consumers. The development of animal cell-based MPS may contribute to this goal by demonstrating the correlation between in vivo and in vitro data.86 In addition, these models can enhance confidence in producing findings that have not been directly assessed in clinical trials. For example, they can be used to study the cellular processes occurring within a specific organ or to examine the functional and phenotypic characteristics associated with a particular disease.87,88 Furthermore, it enhances our understanding of the translational potential of various findings and the reasons behind the different sensitivities of different species.89 Enhancing access to high-quality and well-defined data is of utmost importance in the qualification process. An example of this is organ-on-a-chip technology, which requires accurate and careful estimation of parameters such as shear stress, fluid flow, and cell ratios.90 Similarly, for hydrogel-based MPS, it is crucial to adequately and thoroughly characterize and report the composition, degradation profile, biomechanical performance, and biocompatibility of the hydrogel.91 If bioprinting is employed, the composition of the bioink and the impact of printing parameters such as pressure and shear stress on cell function should be measured to ensure the quality and biological relevance of the printed tissue construct. In order to ensure the generation of high-quality data, it is essential to promote thorough data recording, establish standardized operating procedures (SOPs) and guidelines, and encourage the sharing of data across various sectors. There is a growing emphasis on enhancing the capacity of bioinformatics and computational tools as well in order to effectively analyze the substantial amount of data generated by MPS and draw meaningful insights. Moreover, enhancing the system's compatibility with physical assessments, such as histology, could offer valuable insights into spatial cellular organization and histological analysis.
Additional aspects to consider during development of MPS
The greatest source of variability in MPS is the cells used in their construction.92 Establishing acceptance criteria for reproducible results is a foremost challenge. For example, variations in iPSCs and differentiated cells arise from diverse sources, resulting in heterogeneity. To minimize these variations, one can establish specific selection criteria, such as evaluating the expression of cell-specific markers or creating gene expression signatures.93 Another study found that the ability of epithelial cells to change to mesenchymal cells is varied and donor-dependent, illustrating why it is critical to thoroughly examine the cells before use and use only those that meet the established acceptance criteria.94
Media and hydrogels are another source of variability that affects the progress and success of MPS platforms. There are many undefined components in cell culture media which might impact the reproducibility of data. For example, the use of animal-derived serum in human models warrants careful consideration due to batch-to-batch variability.95 To ensure accurate and reliable results, it is crucial to have a clear understanding of how serum components can potentially impact the behavior of the biological product. The careful selection of growth factors, along with their precise quantities, holds paramount importance in facilitating robust and reproducible cell growth and maintaining desired functionalities. The inclusion of cells that secrete specific cytokines can wield a profound influence on the biological responses observed within the model. Moreover, in certain cases, the development of a universal media formulation capable of nurturing diverse cell types while upholding their anticipated functionalities would help simplify the experimental setup while ensuring consistent results across a wide range of cell types. When designing matrices and scaffolds, it is important to carefully consider the source, understand their biological relevance and how they interact with cells. The variability in composition, whether it is within or between different batches, can significantly influence cell response. The selection of the polymer may vary depending on the organ model and the specific experimental question. Additionally, it is essential to ensure that the biomaterial is easily accessible and does not elicit unexpected or undesirable responses.
Improving the robustness of endpoint measurements is another area that can benefit from enhancements. Utilizing techniques such as metabolomics, transcriptomics, and proteomics at the single-cell level can offer comprehensive characterization of models, thereby increasing confidence in their ability to replicate in vivo tissue.96 When selecting a model to investigate the modulation of a specific biochemical pathway or for target validation, it is necessary to confirm the expression levels of proteins and genes in that pathway, as well as the biological target of interest, in the relevant cell types. Additionally, whenever feasible, conducting measurements of cell-specific physiological functions is advisable. For example, using multi-electrode arrays to record the generation of action potentials can be a useful way to identify neuronal phenotypes. Similarly, conducting metabolic activity assessments for hepatocytes or measuring contraction in cardiomyocytes can provide valuable information about their functionality specific to the organ they belong to. Finally, efforts should be made to enhance fabrication and operational management. When constructing microfluidics-based MPS, it is crucial to take into account several important factors. These factors include ensuring the generation of adequate shear stress at physiologically relevant rates to maintain stable protein and oxygen gradients within the system for extended periods of time. Additionally, researchers must also take into account the fact that materials used in platform construction, such as polydimethylsiloxane (PDMS), are known to absorb hydrophobic small molecules.97,98 It is important to thoroughly investigate the interaction between critical molecules and other materials present in the platform. In both plate-based setups (under stationary conditions) and chip-based configurations (with dynamic conditions), incorporating automated systems with electrical, optical, and mechanical transduction is important for monitoring and managing intricate small-scale tissue cultures. Incorporating advanced imaging capabilities would enhance the ability to track specific targeted receptors and off-target activities in real-time. This would greatly improve operational supervision and enable label-free long-term experiments.
In summary, to ensure the reliability and applicability of MPS, it is important to carefully select suitable models, exercise control over fabrication processes, and conduct comprehensive characterization of materials and cells.
Conclusion
In this paper, we discussed the recent advances of MPS technologies in enhancing the recapitulation of organ biology. Additionally, we discussed the potential applications of these technologies in evaluating biological therapeutic products, such as cell and gene therapies. While MPS technologies offer several advantages over conventional in vitro models, including increasing spatial and temporal control, as well as enhanced controls of biochemical and biomechanical characteristics, it is crucial to acknowledge the challenges that come with their implementation. Material choice, fabrication, analysis, standardization, and reproducibility are ongoing challenges for researchers working with MPS platforms. Despite these challenges, in specific contexts, these platforms may enhance the prediction of bioactivity and toxicity risks, and they also yield valuable information about the immune reactions and tumorigenicity of biologic products. Notably, MPS have the potential to facilitate the emergence of platforms capable of preserving the phenotypic characteristics of individual patients. This, in turn, would allow for a better understanding of interindividual differences. We believe that embracing and furthering the advancements in MPS will undoubtedly lead us into an era of more effective and safer therapeutic interventions. This will greatly benefit numerous patients, reduce the need for animal experimentation, and ultimately affect the future of healthcare.
Author contributions
All authors contributed to the writing, discussion, and correction of the review.
Conflicts of interest
There are no conflicts to declare.
References
- J. Criscione,
et al., Heart-on-a-chip platforms and biosensor integration for disease modeling and phenotypic drug screening, Biosens. Bioelectron., 2023, 220, 114840 CrossRef CAS PubMed.
- J. Arrowsmith and P. Miller, Trial watch: phase II and phase III attrition rates 2011-2012, Nat. Rev. Drug Discovery, 2013, 12(8), 569 CrossRef CAS PubMed.
- L. Donoghue,
et al., Tissue Chips and Microphysiological Systems for Disease Modeling and Drug Testing, Micromachines, 2021, 12(2) CrossRef PubMed.
- Z. B. Bjornson-Hooper,
et al., A Comprehensive Atlas of Immunological Differences Between Humans, Mice, and Non-Human Primates, Front. Immunol., 2022, 13, 867015 CrossRef CAS PubMed.
-
S. R. Paul, S.5002 - FDA Modernization Act 2.0. 2022; Available from: https://www.congress.gov/bill/117th-congress.
- Nonclinical Evaluation of the Immunotoxic Potential of Pharmaceuticals, Guidance for Industry. 2023, US Food and Drug Administration, Center for Drug Evaluation and Research (CDER).
- Advancing Alternative Methods at FDA. 2022; Available from: https://www.fda.gov/science-research/about-science-research-fda/advancing-alternative-methods-fda.
- S. W. Baran,
et al., Perspectives on the evaluation and adoption of complex in vitro models in drug development: Workshop with the FDA and the pharmaceutical industry (IQ MPS Affiliate), Altex Chromatogram, 2022, 39(2), 297–314 Search PubMed.
- C. P. Austin, Opportunities and challenges in translational science, Clin. Transl. Sci., 2021, 14(5), 1629–1647 CrossRef PubMed.
- C. B. Zhao, Cell culture: model system and a promising path to applications, J. Histotechnol., 2023, 46(1), 1–4 CrossRef PubMed.
- M. Virumbrales-Muñoz and J. M. Ayuso, From microfluidics to microphysiological systems: Past, present, and future, Organs-on-a-Chip, 2022, 4, 100015 CrossRef.
- H. Wang,
et al., 3D cell culture models: Drug pharmacokinetics, safety assessment, and regulatory consideration, Clin. Transl. Sci., 2021, 14(5), 1659–1680 CrossRef PubMed.
- A. M. Sun,
et al., Application of lung microphysiological systems to COVID-19 modeling and drug discovery: a review, Bio-Des. Manuf., 2021, 4(4), 757–775 CrossRef CAS PubMed.
- A. Mathur,
et al., Human induced pluripotent stem cell-based microphysiological tissue models of myocardium and liver for drug development, Stem Cell Res. Ther., 2013, 4(1), S14 CrossRef PubMed.
- S. Yang,
et al., Liver three-dimensional cellular models for high-throughput chemical testing, Cells Rep. Methods, 2023, 3(3), 100432 CrossRef CAS PubMed.
- Q. Shi,
et al., Co-Culture of Human Primary Hepatocytes and Nonparenchymal Liver Cells in the Emulate(R) Liver-Chip for the Study of Drug-Induced Liver Injury, Curr. Protoc., 2022, 2(7), e478 CrossRef CAS PubMed.
- K. Eckstrum,
et al., Utilization of a model hepatotoxic compound, diglycolic acid, to evaluate liver Organ-Chip performance and in vitro to in vivo concordance, Food Chem. Toxicol., 2020, 146, 111850 CrossRef CAS PubMed.
- A. Rubiano,
et al., Characterizing the reproducibility in using a liver microphysiological system for assaying drug toxicity, metabolism, and accumulation, Clin. Transl. Sci., 2021, 14(3), 1049–1061 CrossRef CAS PubMed.
- Biological products regulated under Section 351 of the Public Health Service Act; implementation of biologics license; elimination of establishment license and product license; public workshop--FDA. Proposed rule; notice of workshop, Fed. Regist., 1998, 63, 42773–42774 Search PubMed.
- W. Kim,
et al., Therapeutic strategies of three-dimensional stem cell spheroids and organoids for tissue repair and regeneration, Bioact. Mater., 2023, 19, 50–74 CAS.
- J. K. Choi,
et al., Functionally enhanced cell spheroids for stem cell therapy: Role of TIMP1 in the survival and therapeutic effectiveness of stem cell spheroids, Acta Biomater., 2023, 454–469 CrossRef CAS PubMed.
- M. Virumbrales-Munoz,
et al., Microphysiological model of renal cell carcinoma to inform anti-angiogenic therapy, Biomaterials, 2022, 283, 121454 CrossRef CAS PubMed.
- S. Kim,
et al., Organoids for Advanced Therapeutics and Disease Models, Adv. Ther., 2019, 2(1), 1800087 CrossRef.
- X. Yin, B. E. Mead, H. Safaee, R. Langer and J. M. Karp, Levy, O, Engineering stem cell organoids, Cell Stem Cell, 2016, 18(1), 25–38 CrossRef CAS PubMed.
- J. Andersen,
et al., Generation of Functional Human 3D Cortico-Motor Assembloids, Cell, 2020, 183(7), 1913–1929 e26 CrossRef CAS PubMed.
- J. Takahashi,
et al., Suspension culture in a rotating bioreactor for efficient generation of human intestinal organoids, Cells Rep. Methods, 2022, 2(11), 100337 CrossRef PubMed.
- V. Velasco, S. A. Shariati and R. Esfandyarpour, Microtechnology-based methods for organoid models, Microsyst. Nanoeng., 2020, 6, 76 CrossRef CAS PubMed.
- H. Cai,
et al., Engineering human spinal microphysiological systems to model opioid-induced tolerance, Bioact. Mater., 2023, 22, 482–490 CAS.
- X. Qian,
et al., Sliced Human Cortical Organoids for Modeling Distinct Cortical Layer Formation, Cell Stem Cell, 2020, 26(5), 766–781 e9 CrossRef CAS PubMed.
- S. Chawla and A. Das, Preclinical-to-clinical innovations in stem cell therapies for liver regeneration, Curr. Res. Transl. Med., 2023, 71(1), 103365 CAS.
- A. Gonzalez-Pujana,
et al., Multifunctional biomimetic hydrogel systems to boost the immunomodulatory potential of mesenchymal stromal cells, Biomaterials, 2020, 257, 120266 CrossRef CAS PubMed.
- J. H. Tsui,
et al., Tunable electroconductive decellularized extracellular matrix hydrogels for engineering human cardiac microphysiological systems, Biomaterials, 2021, 272, 120764 CrossRef CAS PubMed.
- A. Brown,
et al., Engineering PEG-based hydrogels to foster efficient endothelial network formation in free-swelling and confined microenvironments, Biomaterials, 2020, 243, 119921 CrossRef CAS PubMed.
- W. Peng, D. Unutmaz and I. T. Ozbolat, Bioprinting towards Physiologically Relevant Tissue Models for Pharmaceutics, Trends Biotechnol., 2016, 34(9), 722–732 CrossRef CAS PubMed.
- L. Horvath,
et al., Engineering an in vitro air-blood barrier by 3D bioprinting, Sci. Rep., 2015, 5, 7974 CrossRef CAS PubMed.
- H. Yuan,
et al., Instrumented cardiac microphysiological devices via multi-material 3D printing, Nat. Mater., 2017, 16, 303–308 CrossRef PubMed.
- D. A. Bowser and M. J. Moore, Biofabrication of neural microphysiological systems using magnetic spheroid bioprinting, Biofabrication, 2019, 12(1), 015002 CrossRef PubMed.
- B. Byambaa,
et al., Bioprinted Osteogenic and Vasculogenic Patterns for Engineering 3D Bone Tissue, Adv. Healthcare Mater., 2017, 6(16), 1700015 CrossRef PubMed.
- H. Lee and D. W. Cho, One-step fabrication of an organ-on-a-chip with spatial heterogeneity using a 3D bioprinting technology, Lab Chip, 2016, 16(14), 2618–2625 RSC.
- J. Song,
et al., High-Throughput 3DTumor Vasculature Model for Real-Time Monitoring of Immune Cell Infiltration and Cytotoxicity, Front. Immunol., 2021, 12, 733317 CrossRef CAS PubMed.
- J. Yu,
et al., Perfusable micro-vascularized 3D tissue array for high-throughput vascular phenotypic screening, Nano Convergence, 2022, 9(1), 16 CrossRef CAS PubMed.
- D. Park,
et al., High-Throughput Microfluidic 3D Cytotoxicity Assay for Cancer Immunotherapy (CACI-IMPACT Platform), Front. Immunol., 2019, 10, 1133 CrossRef CAS PubMed.
- S. Mughal,
et al., Organs-on-Chips: Trends and Challenges in Advanced Systems Integration, Adv. Mater. Interfaces, 2022, 9(33), 2201618 CrossRef.
- R. C. Yada,
et al., Microphysiological head and neck cancer model identifies novel role of lymphatically secreted monocyte migration inhibitory factor in cancer cell migration and metabolism, Biomaterials, 2023, 298, 122136 CrossRef CAS PubMed.
- M. Zhang,
et al., Biomimetic Human Disease Model of SARS-CoV-2-Induced Lung Injury and Immune Responses on Organ Chip System, Adv. Sci., 2021, 8(3), 2002928 CrossRef CAS PubMed.
- X. Yu,
et al., CRISPRi-microfluidics screening enables genome-scale target identification for high-titer protein production and secretion, Metab. Eng., 2023, 75, 192–204 CrossRef CAS PubMed.
- T. Otomo,
et al., A microfluidic device integrated with a stretchable microporous membrane controlled by electro-conjugate fluid, Sens. Actuators, A, 2023, 114332 CrossRef CAS.
- 21 CFR Part 610-GENERAL BIOLOGICAL PRODUCTS STANDARDS. 1973; Available from: https://www.ecfr.gov/current/title-21/part-610.
- P. Salmikangas,
et al., Potency testing of cell and gene therapy products, Front. Med., 2023, 10, 1190016 CrossRef PubMed.
- J. P. Wikswo, The relevance and potential roles of microphysiological systems in biology and medicine Introduction, Exp. Biol. Med., 2014, 239(9), 1061–1072 CrossRef PubMed.
- D. A. Tagle, The NIH microphysiological systems program: developing in vitro tools for safety and efficacy in drug development, Curr. Opin. Pharmacol., 2019, 48, 146–154 CrossRef CAS PubMed.
- J. Lam,
et al., A microphysiological system-based potency bioassay for the functional quality assessment of mesenchymal stromal cells targeting vasculogenesis, Biomaterials, 2022, 290, 121826 CrossRef CAS PubMed.
- J. Lam,
et al., Functional Profiling of Chondrogenically Induced Multipotent
Stromal Cell Aggregates Reveals Transcriptomic and Emergent Morphological Phenotypes Predictive of Differentiation Capacity, Stem Cells Transl. Med., 2018, 7(9), 664–675 CrossRef CAS PubMed.
- Guideline on quality, non-clinical and clinical requirements for investigational advanced therapy medicinal products in clinical trials. 2018; Available from: https://www.ema.europa.eu/en/documents/scientific-guideline/guideline-quality-non-clinical-clinical-aspects-gene-therapy-medicinal-products_en.pdf.
- Guidance for Industry, Potency Tests for Cellular and Gene Therapy Products. 2011; Available from: https://www.fda.gov/files/vaccines,%20blood%20%26%20biologics/published/Final-Guidance-for-Industry--Potency-Tests-for-Cellular-and-Gene-Therapy-Products.pdf.
- K. Achberger,
et al., Human stem cell-based retina on chip as new translational model for validation of AAV retinal gene therapy vectors, Stem Cell Rep., 2021, 16(9), 2242–2256 CrossRef CAS PubMed.
- I. Liadi,
et al., Defining potency of CAR(+) T cells: Fast and furious or slow and steady, Oncoimmunology, 2019, 8(10), e1051298 CrossRef PubMed.
- C. De Wolf, M. De Bovenkamp and M. Hoefnagel, Regulatory perspective on in vitro potency assays for human dendritic cells used in anti-tumor immunotherapy, Cytotherapy, 2018, 20(11), 1289–1308 CrossRef CAS PubMed.
- S. W. L. Lee,
et al., Corrigendum: Characterizing the Role of Monocytes in T Cell Cancer Immunotherapy Using a 3D Microfluidic Model, Front. Immunol., 2018, 9, 1719 CrossRef PubMed.
- X. Si,
et al., Preclinical Evaluation of CAR T Cell Function: In Vitro and In Vivo Models, Int. J. Mol. Sci., 2022, 23(6), 3154 CrossRef CAS PubMed.
- F. Jacob,
et al., A Patient-Derived Glioblastoma Organoid Model and Biobank Recapitulates Inter- and Intra-tumoral Heterogeneity, Cell, 2020, 180(1), 188–204 e22 CrossRef CAS PubMed.
- K. Petrovic,
et al., TEM8/ANTXR1-specific CAR T cells mediate toxicity in vivo, PLoS One, 2019, 14(10), e0224015 CrossRef CAS PubMed.
- G. P. Linette,
et al., Cardiovascular toxicity and titin cross-reactivity of affinity-enhanced T cells in myeloma and melanoma, Blood, 2013, 122(6), 863–871 CrossRef CAS PubMed.
- S. Yagyu,
et al., A lymphodepleted non-human primate model for the assessment of acute on-target and off-tumor toxicity of human chimeric antigen receptor-T cells, Clin. Transl. Immunol., 2021, 10(6), e1291 CrossRef CAS PubMed.
- C. Gouttefangeas, R. Klein and A. Maia, The good and the bad of T cell cross-reactivity: challenges and opportunities for novel therapeutics in autoimmunity and cancer, Front. Immunol., 2023, 14, 1212546 CrossRef CAS PubMed.
- M. Castellarin,
et al., A rational mouse model to detect on-target, off-tumor CAR T cell toxicity, JCI Insight, 2020, 5(14), e136012 CrossRef PubMed.
- J. A. Hammill,
et al., A Cross-Reactive Small Protein Binding Domain Provides a Model to Study Off-Tumor CAR-T Cell Toxicity, Mol. Ther.--Oncolytics, 2020, 17, 278–292 CrossRef CAS PubMed.
- B. T. Assaf and L. O. Whiteley, Considerations for Preclinical Safety Assessment of Adeno-Associated Virus Gene Therapy Products, Toxicol. Pathol., 2018, 46(8), 1020–1027 CrossRef CAS PubMed.
- R. M. Ramamurthy,
et al., Using a Human Liver Tissue Equivalent (hLTE) Platform to Define the Functional Impact of Liver-Directed AAV Gene Therapy, Blood, 2021, 138, 2938 CrossRef.
- A. Westhaus,
et al., Assessment of pre-clinical liver models based on their ability to predict the liver-tropism of adeno-associated virus vectors, Hum. Gene Ther., 2023, 34(7–8), 273–288 CrossRef CAS PubMed.
- O. T. P. Nguyen,
et al., An Immunocompetent Microphysiological System to Simultaneously Investigate Effects of Anti-Tumor Natural Killer Cells on Tumor and Cardiac Microtissues, Front. Immunol., 2021, 12, 781337 CrossRef CAS PubMed.
- D. Liu,
et al., Blockade of AIM2 inflammasome or alpha1-AR ameliorates IL-1beta release and macrophage-mediated immunosuppression induced by CAR-T treatment, J. Immunother. Cancer., 2021, 9(1), e001466 CrossRef PubMed.
- J. H. Hammel,
et al., Modeling Immunity In Vitro: Slices, Chips, and Engineered Tissues, Annu. Rev. Biomed. Eng., 2021, 23, 461–491 CrossRef CAS PubMed.
- F. Mingozzi and K. A. High, Immune responses to AAV vectors: overcoming barriers to successful gene therapy, Blood, 2013, 122(1), 23–36 CrossRef CAS PubMed.
- H. C. J. Ertl, Immunogenicity and toxicity of AAV gene therapy, Front. Immunol., 2022, 13, 975803 CrossRef CAS PubMed.
- R. M. Ramamurthy,
et al., Organoids and microphysiological systems: Promising models for accelerating AAV gene therapy studies, Front. Immunol., 2022, 13, 1011143 CrossRef CAS PubMed.
- G. A. Duncan, Integrative approaches to enhance adeno-associated viral gene delivery, J. Controlled Release, 2022, 341, 44–50 CrossRef CAS PubMed.
- Y. Takei,
et al., Quality assessment tests for tumorigenicity of human iPS cell-derived cartilage, Sci. Rep., 2020, 10(1), 12794 CrossRef CAS PubMed.
- L. Barkholt,
et al., Risk of tumorigenicity in mesenchymal stromal cell-based therapies-Bridging scientific observations and regulatory viewpoints, Cytotherapy, 2013, 15(7), 753–759 CrossRef PubMed.
- Y. Sato,
et al., Tumorigenicity assessment of cell therapy products: The need for global consensus and points to consider, Cytotherapy, 2019, 21(11), 1095–1111 CrossRef CAS PubMed.
- S. Yamanaka, Pluripotent Stem Cell-Based Cell Therapy- Promise and Challenges, Cell Stem Cell, 2020, 27(4), 523–531 CrossRef CAS PubMed.
- U. Marx,
et al., Biology-inspired microphysiological systems to advance patient benefit and animal welfare in drug development, Altex, 2020, 37(3), 365 Search PubMed.
- Validation, Qualification, and Regulatory Acceptance of New Approach Methodologies. 2023; Available from: https://ntp.niehs.nih.gov/sites/default/files/2023-08/VWG%20Report%20Draft_for%20public%20comment_08Aug2023.pdf.
- C. M. Leung,
et al., A guide to the organ-on-a-chip, Nat. Rev. Methods Primers, 2022, 2(1), 33 CrossRef CAS.
- T. I. Maulana,
et al., Immunocompetent cancer-on-chip models to assess immuno-oncology therapy, Adv. Drug Delivery Rev., 2021, 173, 281–305 CrossRef CAS PubMed.
- M. Kawasaki, T. Goyama, Y. Tachibana, I. Nagao and Y. M. Ambrosini, Farm and Companion Animal Organoid Models in Translational Research: A Powerful Tool to Bridge the Gap Between Mice and Humans, Front. Med. Technol., 2022, 895379 CrossRef PubMed.
- T. Usui,
et al., Establishment of a dog primary prostate cancer organoid using the urine cancer stem cells, Cancer Sci., 2017, 108(12), 2383–2392 CrossRef CAS PubMed.
- J. Zhou,
et al., Infection of bat and human intestinal organoids by SARS-CoV-2, Nat. Med., 2020, 26(7), 1077–1083 CrossRef CAS PubMed.
-
A. Khan, et al., In Vitro and In Vivo Animal Models: The Engineering Towards Understanding Human Diseases and Therapeutic Interventions. Omics Technologies and Bio-Engineering: Towards Improving Quality of Life, Emerging Fields, Animal and Medical Biotechnologies, 2018, vol. 1, pp. 431–448 Search PubMed.
- M. Piergiovanni,
et al., Standardisation needs for organ on chip devices, Lab Chip, 2021, 21(15), 2857–2868 RSC.
- A. R. Harris, M. J. Walker and F. Gilbert, Ethical and regulatory issues of stem cell-derived 3-dimensional organoid and tissue therapy for personalised regenerative medicine, BMC Med., 2022, 20(1), 499 CrossRef PubMed.
- C. Sakolish,
et al., Technology Transfer of the Microphysiological Systems: A Case Study of the Human Proximal Tubule Tissue Chip, Sci. Rep., 2018, 8(1), 14882 CrossRef PubMed.
- Z. Y. Guo,
et al., Building a microphysiological skin model from induced pluripotent stem cells, Stem Cell Res. Ther., 2013, 4, 1–7 CrossRef PubMed.
- S. Fowler,
et al., Microphysiological systems for ADME-related applications: current status and recommendations for system development and characterization, Lab Chip, 2020, 20(3), 446–467 RSC.
- J. van der Valk,
et al., Fetal Bovine Serum (FBS): Past - Present - Future, ALTEX, 2018, 35(1), 99–118 CrossRef PubMed.
- J. E. Ekert,
et al., Recommended Guidelines for Developing, Qualifying, and Implementing Complex In Vitro Models (CIVMs) for Drug Discovery, SLAS Discovery, 2020, 25(10), 1174–1190 CrossRef CAS PubMed.
- B. J. van Meer,
et al., Small molecule absorption by PDMS in the context of drug response bioassays, Biochem. Biophys. Res. Commun., 2017, 482(2), 323–328 CrossRef CAS PubMed.
- M. W. Toepke and D. J. Beebe, PDMS absorption of small molecules and consequences in microfluidic applications, Lab Chip, 2006, 6(12), 1484–1486 RSC.
|
This journal is © The Royal Society of Chemistry 2024 |