DOI:
10.1039/D4IM00031E
(Review Article)
Ind. Chem. Mater., 2025,
3, 7-30
Progress on aqueous rechargeable aluminium metal batteries
Received
20th March 2024
, Accepted 13th June 2024
First published on 18th June 2024
Abstract
Aqueous rechargeable aluminium metal batteries (ARAMBs) have advantages of high energy density, cost efficiency and reasonable safety. However, parasitic reactions between the Al anode and electrolyte, sluggish dynamics and low reversibility of the Al anode, and structural instability caused by the high charge density of Al3+ ions lead to a short cycling life and inferior high-rate performance in ARAMBs. Herein, in this review, we summarize the research progress on ARAMBs by emphasizing the reported strategies to address the above-mentioned intractable issues. Initially, we discuss how to regulate the Al anode and interphase to accelerate the kinetics of Al stripping, which mainly includes strategies of ionic liquid analogue-derived solid electrolyte interphases (SEIs), artificial interfacial functional layer and aluminium alloy. Subsequently, the electrolyte modification approaches are highlighted including preparing highly concentrated single-salt/bi-salt electrolytes and designing electrolyte additives to reduce the parasitic reactions of ARAMBs. Finally, we introduce the progress on fabricating cathodes, such as vanadium-based materials, manganese-oxide materials, molybdenum-based materials, Prussian blue analogues, carbon materials, and organic materials to accommodate Al3+ ions. We propose that the further development of ARAMBs requires the cooperation of the above-mentioned strategies to improve their overall electrochemical performance and the development of new methods to illustrate the reaction mechanism of batteries.
Keywords: Aqueous batteries; Interphase design; Al anode; Electrolyte modification; Cathode materials.
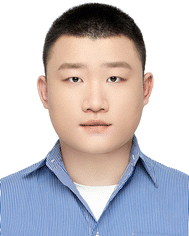 Xiaotian Wang | Xiaotian Wang is currently pursuing his Ph.D. in Inorganic Chemistry at Nankai University under the supervision of Prof. Qing Zhao. He received his B.S. in Chemistry at Nankai University in 2023. His research interests are focused on the design and preparation of electrolytes for aqueous rechargeable aluminum metal batteries. |
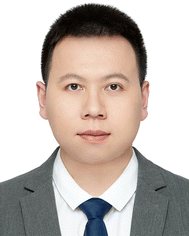 Zihang Xi | Zihang Xi is currently pursuing his M.S. in Inorganic Chemistry at Nankai University under the supervision of Prof. Qing Zhao. He received his B.S. in Chemistry at Nankai University in 2023. His research interest focuses on the design of electrolyte additives for aqueous rechargeable aluminum metal batteries. |
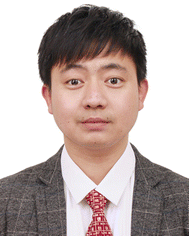 Qing Zhao | Qing Zhao received his B.S. (2012) and Ph.D. (2017) degrees from Nankai University. He was a Postdoctoral Fellow in Cornell University from 2017 to 2021. Presently, he is a Professor in Nankai University. His research interests focus on the interphase chemistry of electrode/electrolyte and advanced battery systems. |
1 Introduction
Given that the non-renewable traditional fossil energy cannot satisfy the long-term demands for energy consumption, environmentally friendly renewable energy sources are gaining increasing prominence in energy supply.1,2 However, these sustainable energy sources such as solar and wind energy are constrained by natural conditions with uneven temporal and spatial distribution, which highlights the importance of developing large-scale energy storage systems (ESSs).3,4 As one of the most successful electrochemical ESSs, lithium-ion batteries (LIBs), which have advantages of less space restriction, higher conversion efficiency/energy density, faster response speed and shorter construction time than pumped storage, have witnessed rapid development in recent years.5 However, the limited/uneven distribution of lithium resources and the safety risk associated with LIBs limit their application in large-scale energy storage.6 Accordingly, significant efforts have been devoted to developing adequate alternatives beyond lithium batteries, such as sodium, potassium, zinc, magnesium, calcium, and aluminium batteries.7 Among them, sodium-ion batteries (NIBs) have been the most widely studied due to the highly abundant Na resource and their similar working principle with LIBs.8,9 Nevertheless, given that flammable organic electrolytes are used in NIBs, their safety is still an issue especially considering the huge electrical energy in ESSs. Therefore, the development of intrinsically safe electrochemical ESSs is urgent.
Rechargeable batteries that operate under the condition of aqueous electrolyte are emerging as potential EESs with merits of high safety and low cost.10,11 Moreover, when using metallic zinc and aluminium as anodes that exhibit low reactivity in the atmosphere and moisture, the constructed aqueous rechargeable batteries can also deliver a high energy density (Fig. 1).12–15 Compared with zinc batteries, aluminium metal batteries (AMBs) show the following merits: 1) high abundance in the Earth's crust (8.21 wt% for Al and 0.0076 wt% for zinc). 2) Large-scale production with low cost. 3) Potential three-electron transfer mechanism with lightweight characteristic allows aluminium metal to achieve extremely high specific capacities (2980 mA h g−1 and 8046 mA h cm−3 for Al, 820 mA h g−1 and 5855 mA h cm−3 for zinc).
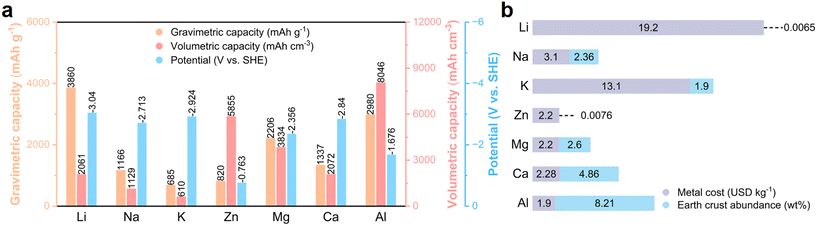 |
| Fig. 1 Advantages of Al as a battery anode. (a) Comparison of theoretical specific capacities and standard electrochemical reduction potentials of different metal anodes. (b) Abundance of different metal elements in the Earth's crust and the cost of metal anodes. Reprinted with permission.16 Copyright 2021, WILEY-VCH VERLAG GMBH. | |
Although aluminium was reported as a battery anode in the Buff battery as early as 1857 and other primary Al batteries such as Al/air, Al/sulphur, and Al/CO2 batteries are also well known, the first rechargeable aluminium battery only appeared in 2011, when Archer et al. applied AlCl3/1-ethyl-3-methylimidazolium chloride ([EMIm]Cl) ionic liquid (IL) electrolyte to achieve the reversible operation of an Al/V2O5 battery.17–23 However, due to the sluggish kinetics and less reversible electrochemistry of the V2O5 cathode, the development of AMBs was still slow until anion intercalation chemistry was found in the graphite cathode in 2015.24 The Al/graphite battery using the AlCl3-[EMIm]Cl IL electrolyte exhibits fast kinetics with an excellent cycling performance (over 7000 charge/discharge cycles), boosting the development of this emerging ESS.24–26 Various cathode materials such as organic compounds, carbon materials, metal chalcogenide, sulphur, metal–organic frameworks and aluminium anode host/current collectors have been reported for AMBs with an increased specific capacity and cycling life.27–38
However, despite the merits of IL-based aluminium batteries, the moisture sensitivity of IL makes it difficult to assemble AMBs under atmospheric conditions, thus greatly increasing the production costs and contradicting the advantages of aluminium-metal anodes.39,40 Meanwhile, due to the large anion (AlCl4−) interaction mechanism, the cathode has a low specific capacity (usually less than 100 mA h g−1).35,41 In comparison, aqueous rechargeable aluminium metal batteries (ARAMBs) using metallic Al as the anode and high-safety aqueous electrolytes have emerged as potential candidates in last five years. However, although the high specific capacity and moderate redox potential of Al3+/Al result in a high theoretical energy density in ARAMBs, there are still intractable issues that need to be overcome before their commercial application. Currently, the most prominent problem associated with ARAMBs is the interface between the aluminium-metal anode and the aqueous electrolyte, which is not present in aqueous Al-ion batteries.42–47 Furthermore, due to the low standard reduction potential of Al (−1.676 V vs. standard hydrogen electrode, SHE), the hydrogen evolution reaction (HER) theoretically occurs prior to the electrochemical stripping of Al.17 Although a dense passivated oxide layer with large band gap can suppress the spontaneous HER, it also significantly reduces the reaction kinetics of the Al anode.48 Moreover, the easy passivation of the oxide layer also prevents the reversible stripping/deposition of aluminium. Meanwhile, the high charge density of Al3+ ions also leads to a strong electrostatic interaction with the cathode material, which predisposes the cathode to structural disintegration, and thus reduces the battery capacity.49
Thus, to overcome the issues associated with ARAMBs, considerable efforts have been devoted to designing anode, electrolyte and cathode materials in last five years (Fig. 2). The rapid increase in the number of publications and the inconsistent conclusions from various works make a review in this field necessary. Herein, we focus on the research progress on ARAMBs that operate under aqueous electrolyte conditions. This review summarizes the modification strategies in terms of three aspects, including Al anode and interphase regulation, modification of aqueous electrolytes, and design of cathode materials. On the anode side, we emphasize that the modification of Al metal anodes and electrolytes needs collaboration to stabilize the operation of batteries. On the cathode side, the storage mechanism is still ambiguous for most cathode materials given that various ions such as Al3+, H+, Al(CF3SO3)2+, and AlCl4− have been reported as charge carriers in ARAMBs. We anticipate that this review can offer fundamental principles for designing advanced electrodes and electrolytes for next-generation energy-dense aqueous batteries.
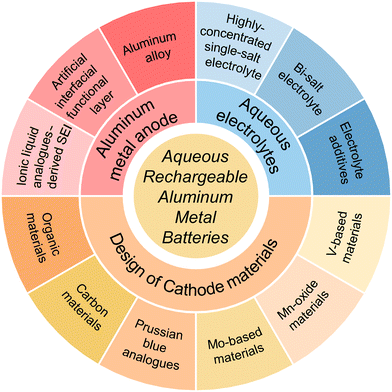 |
| Fig. 2 Modification strategies for aqueous rechargeable Al metal batteries. | |
2 Al anode and interphase regulation
It is difficult for pure Al metal to function as an anode in conventional aqueous electrolytes owing to the sluggish Al stripping process caused by the passivation film on the surface of Al and inevitable HER once fresh Al is exposed to the electrolyte. Thus, to alleviate the above-mentioned issues, it is necessary to modify the interface of Al to inhibit interface passivation and side reactions towards the electrolyte. To date, a few strategies have been proposed to modify the aluminium metal anode, which can be generally summarized as ionic liquid analogue-derived solid electrolyte interphase (SEI), artificial interfacial functional layer, and aluminium alloy anode.
2.1 Ionic liquid analogue-derived SEI
A qualified SEI that is endowed with electronic insulation and ionic conductivity has been well known for enabling reversible stripping/plating of alkali metal anodes.50 In 2018, Zhao et al. proposed a strategy to construct an SEI on an Al anode by pre-treating aluminium foil with an AlCl3-[EMIm]Cl IL electrolyte.48 The IL could erode the Al2O3 passivation film of Al by forming a new interfacial layer rich in IL derivatives, which contained abundant C
N, C
O, and C
C bonds according to the FTIR spectra (Fig. 3a). In comparison, the vibration modes of the imidazolium ring in [EMIm]Cl largely disappeared, indicating that imidazolium may undergo ring-open reactions on the surface of Al, preventing the oxidation of the Al anode. The IL-derived SEI effectively transformed the interfacial chemistry of the metal anode (Fig. 3b and c), enabling fast charge transport at the electrolyte/electrode interface. Since then, more works using Al anodes modified by ionic liquids have been reported to match various cathode materials, such as Prussian blue analogues (PBAs), organic materials, carbon materials and transition metal oxides.49,51–55 Besides the expensive IL-based electrolytes, some low-cost eutectic solvents can also be used to pre-treat Al metal anodes. For example, the AlCl3–acetamide deep eutectic solution (DES, AlCl3/Acm = 1.3 by molar ratio) can also erode the passivation layer of aluminium metal anodes.56 The difference in the surface chemical composition of Al anodes before and after treatment with DES was characterized by XPS tests (Fig. 3d and e, respectively), which showed a significant reduction in oxides and the appearance of a new peak corresponding to Al–Cl bonds, demonstrating that the interfacial chemistry of the metal anode was effectively transformed. This conclusion was also be supported by the cross-section scanning electron microscopy (SEM) images and energy-dispersive X-ray spectroscopy (EDS) (Fig. 3f), where the interphase was rich in Cl, O, and N. Overall, the SEI on the Al anode generated by eutectic electrolytes can largely improve the kinetics of the Al stripping process. In general, organic cations in ionic liquid analogues undergo chemical transformation on the surface of the Al anode. In addition, the residual AlCl3 analogue provides an acidic environment for fast charge transfer kinetics.
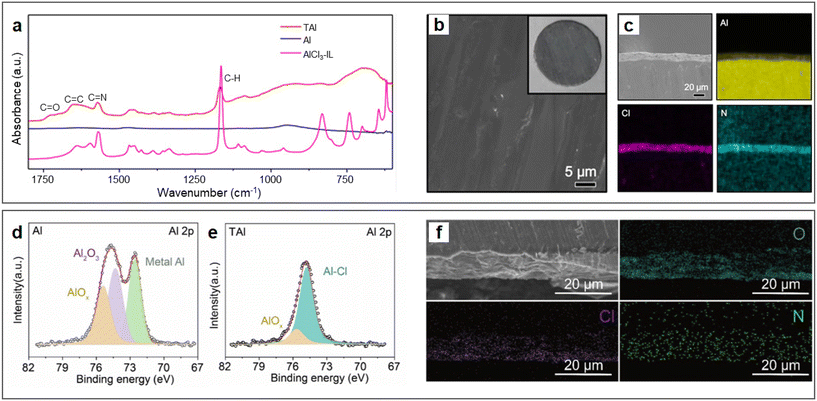 |
| Fig. 3 Characterization of ionic liquid analogue-derived SEI. (a–c) AlCl3-[EMIm]Cl ionic liquid electrolyte-treated Al (TAl) foil. (a) Attenuated total reflection-Fourier transform infrared spectra (ATR-FTIR) of Al and TAl foil. SEM images of (b) surface with inserted digital photo and (c) cross-section with corresponding EDS mapping of Al, Cl, and N of TAl foil. Reproduced with permission.48 Copyright 2018, AMER ASSOC ADVANCEMENT SCIENCE. (d–f) AlCl3–acetamide deep eutectic solution-treated Al (TAl) foil. X-ray photoelectron spectra (XPS) of Al 2p on the surface of (d) Al foil and (e) TAl foil. (f) Cross-sectional SEM image and corresponding EDS mapping of O, Cl and N of TAl foil. Reproduced with permission.56 Copyright 2021, ELSEVIER. | |
Although the stripping/plating kinetics of the Al anode can be significantly accelerated, the long-term stability of the ionic liquid analogue-derived SEI in aqueous electrolyte has been questioned.57 After immersing the IL-derived SEI in highly concentrated Al(CF3SO3)3 (Al(OTF)3) aqueous electrolyte for 30 min, it underwent drastic changes (Fig. 4a). Subsequently, the XPS Al 2p spectra, which were previously dominated by a single AlCl3 peak, exhibited various peaks including Al metal, oxides, hydroxides and AlF3. The chronopotentiometry results indicated that although the IL-treated Al anodes possessed lower “overpotentials” for each half-reaction compared with the pure Al anodes, their superior electrochemical activity was significantly reduced upon prolonged immersion in aqueous electrolyte. This phenomenon was also observed in a urea-AlCl3 eutectic solvent-derived SEI.58 After exposing the Al anode treated by urea-AlCl3 eutectic solvent (UTAl) to air for 48 h, the XPS Al 2p spectra showed that its surface was still covered by chloride salts of Al (Fig. 4b). However, the presence of Al-based fluoride salt and an increase in the relative amount of Al oxide on the surface of UTAl were observed after the first stripping (Fig. 4c), indicating that the partial rupture and dissolution of the SEI may occur in aqueous electrolytes.
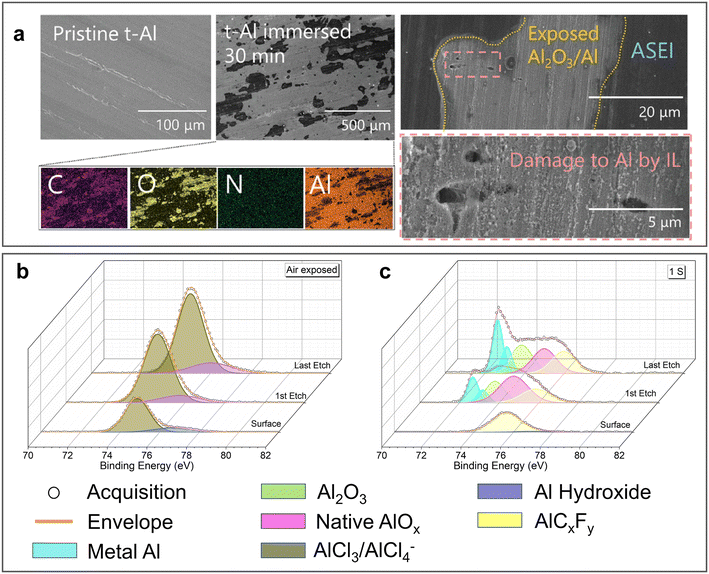 |
| Fig. 4 Long-term stability of ionic liquid analogue-derived SEI. (a) SEM images and corresponding EDX mapping of TAl foil before and after immersion in 5 M Al(OTF)3 electrolyte (left). The magnified image on the right shows the damage of SEI. Reproduced with permission.57 Copyright 2021, WILEY-VCH VERLAG GMBH; Al 2p XPS spectra of UTAl foil (b) after 48 h of exposure to air and (c) after a round of stripping in a symmetric cell. Reproduced with permission.58 Copyright 2022, ELSEVIER SCIENCE SA. | |
Overall, the construction of SEIs for aluminium anodes via ionic liquid analogues can significantly enhance the charge transport kinetics at the anode/electrolyte interface, particularly in symmetric batteries, showing a large reduction in voltage hysteresis compared to pure aluminium anodes under galvanostatic tests. However, the stability of SEIs constructed by this strategy in the atmospheric environment as well as in aqueous electrolytes has been questioned. There are few reports demonstrating the Coulombic efficiencies (CE) of aluminium metal stripping/deposition, and there is also a lack of studies exploring the associated reaction mechanisms.
2.2 Artificial interfacial functional layer
Various strategies have been developed for constructing stable artificial interfacial functional layers on the surface of aluminium metal anodes. Among them, the poly(vinylidene difluoride) (PVDF) coating prepared by the spin coating method not only prevented water and oxygen infiltration but also had the function of guiding the deposition of Al3+ ions on the electrode.59 The working mechanism of the bi-functional PVDF coating can be briefly described as follows: β-PVDF with all-trans structure enabled the strong interaction through the F–Al bond, and thus guided the deposition of Al3+ on the electrode. In addition, the hydrophobic nature of the PVDF coating hindered the contact between the bare Al and free H2O, which inhibited the HER (Fig. 5a). The linear polarization curves of PVDF-Al and bare Al showed that the corrosion current of PVDF-Al (1.31 mA cm−2) was lower than that of bare Al (1.78 mA cm−2), which indicated the slower corrosion rate of the PVDF-Al electrode (Fig. 5b). The linear sweep voltammetry (LSV) studies indicated that PVDF-Al showed a lower initial HER potential of 0.08 V (vs. Al/Al3+) than that of the bare Al anode (0.51 V vs. Al/Al3+) (Fig. 5c). The amorphization strategy has also been applied for the construction of an interfacial functional layer on the surface of aluminium metal anodes. Yan et al. first constructed a porous amorphous metallic Al functional layer on the surface of an aluminium substrate (Al@a–Al) via an in situ lithiation/delithiation method, and further coated a polymer film to increase the overpotential of HER (Fig. 5d).60 The amorphous Al structure endowed the Al anode with the merits of low Al nucleation energy barrier and fast interfacial ions transfer kinetics. The electrochemically active surface area-normalized corrosion current density of Al@a–Al was about 400 times lower than that of bare Al (Fig. 5e), which confirmed the intrinsically restrained self-corrosion of Al@a–Al compared with bare Al. Meanwhile, the LSV studies indicated that the polymer film facilitated a markedly widened cathodic limit (Fig. 5f), ensuring the stable and reversible aluminium stripping/plating in the Al@a–Al@polymer anode. In addition, artificial interfacial functional layers can also be constructed on the surface of aluminium alloy anodes to improve the reversibility of ARAMBs. A eutectic aluminium–cerium alloy uniformly grafted with ultrathin MXene (MXene/E-Al97Ce3) was reported as a highly flexible, reversible, and dendrite-free anode for ARAMBs (Fig. 5g).61 In the composite anode, the grafted ultrathin MXene layer not only suppressed the Al corrosion behaviour (Fig. 5h), but also alleviated the passivating effect of the native oxide layer to facilitate Al3+ transport, resulting in a significant enhancement of Al stripping/plating behaviour. Furthermore, no gas bubbles were observed at the MXene/E-Al97Ce3 electrode due to the negligible hydrogen evolution (Fig. 5i). The symmetric MXene/E-Al97Ce3 cell finally displayed stable stripping/plating voltage profiles over 1000 h.
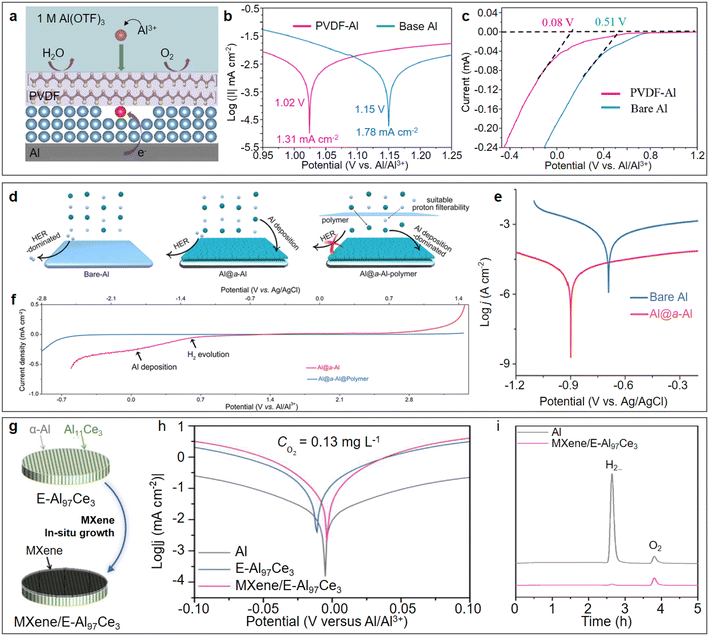 |
| Fig. 5 Design artificial layer on Al anode. (a–c) PVDF-coated Al. (a) Schematic illustration of PVDF-Al. (b) Linear polarization curves presenting the corrosion currents for bare Al and PVDF-Al. (c) Comparison of HER initial potentials between PVDF Al and pure Al. Reproduced with permission.59 Copyright 2022, PERGAMON-ELSEVIER SCIENCE LTD. (d–f) Combination of amorphous Al and polymer coating (Al@a–Al@polymer). (d) Schematic illustration of Al@a–Al@polymer improving the Al stripping/plating reversibility and suppressing HER activity. (e) Comparison of potentiodynamic polarization curves normalized before and after adding amorphous Al layer. (f) Electrochemical stability window (ESW) of aqueous electrolyte (0.5 M Al2(SO4)3) obtained by LSV at a rate of 5 mV s−1 using Al@a–Al and Al@a–Al-polymer working electrodes, respectively. Reproduced with permission.60 Copyright 2022, AMER CHEMICAL SOC. (g and h) Combination of Al97Ce3 alloy and MXene coating. (g) Schematic illustration of the fabrication of MXene/E-Al97Ce3 hybrid electrodes. (h) Tafel polarization curves of MXene/E-Al97Ce3, E-Al97Ce3 alloy and monometallic Al electrodes in 2 M Al(OTF)3 aqueous electrolyte at a scan rate of 5 mV s−1. (i) Gas evolution profiles of Al and MXene/E-Al97Ce3 hybrid electrodes during Al stripping/plating progress. Reproduced with permission.61 Copyright 2023, WILEY-VCH VERLAG GMBH. | |
Obviously, the introduction of artificial interfacial functional layers has an effect on inhibiting gas generation in the operation of ARAMBs, which greatly improves the reliability of ARAMBs. However, due to the diversity and complex composition of artificial interfacial functional layers, it is difficult to investigate the corresponding working mechanism. In addition, although some hydrophobic interfacial layers inhibit the HER activity, they also have negative effects on the charge transfer at the interface, simultaneously. Therefore, it is important to further unveil the working mechanism and develop more effective functional layers for ARAMBs.
2.3 Aluminium alloy
In addition to building SEI or functional layers on the Al anode, the design of Al alloys by rationally selecting the alloying elements can also shift the standard potential of the anode to a more positive value, thereby enabling the deposition on the metal anode through an underpotential deposition process.62,63 For example, an oxidation-resistant and dendrite-suppressed Zn–Al alloy anode was fabricated by depositing Al3+ on a Zn substrate (Fig. 6a).64 The addition of Zn could effectively mitigate the passivation and self-discharge behaviour of the Al anode and improve the CE of the metal stripping/plating process by inhibiting HER. Meanwhile, Zn2+ deposits more easily than Al3+ because of its higher electrochemical redox potential in aqueous media, and thus the non-deposited Al3+ could form a positively charged electrostatic shield to suppress the growth of metal dendrites (Fig. 6b). Given that the co-deposition of Zn2+/Al3+ ions effectively reduced the nucleation barrier of Al deposition on the substrate,47 the Zn–Al alloy anode delivered a small metal stripping/plating voltage polarization for more than 1000 h (Fig. 6c). The battery assembled with the Zn–Al alloy anode and AlxMnO2 cathode delivered a high discharge voltage plateau near 1.6 V and specific capacity of 460 mA h g−1 for over 80 cycles. When using tetrachloro-1,4-benzoquinone (TCQ) as the cathode, the cell afforded good cycling stability with 70.7% capacity retention after 200 charge–discharge cycles at a current density of 2 A g−1.65 In another work, Ran et al. reported the fabrication of a eutectic Al82Cu18 alloy (E-Al82Cu18) anode with a lamellar nanostructure consisting of alternating α-Al and intermetallic Al2Cu nanolamellas.66 The high-resolution transmission electron microscopy (HRTEM) image with element mapping confirmed the phase separation triggered by the eutectic reaction and the periodic distribution of Al and Cu atoms in the alloy anode (Fig. 6d and e), respectively. When using E-Al82Cu18 as the anode, the less-noble α-Al lamellas worked as electroactive materials to supply Al3+ charge carriers, while the more-noble Al2Cu lamellas served as 2D nanopatterns to guide highly reversible Al stripping and plating at low overpotentials (Fig. 6e), which together endowed the electrodes with exceptional Al stripping/plating stability for more than 2000 h.
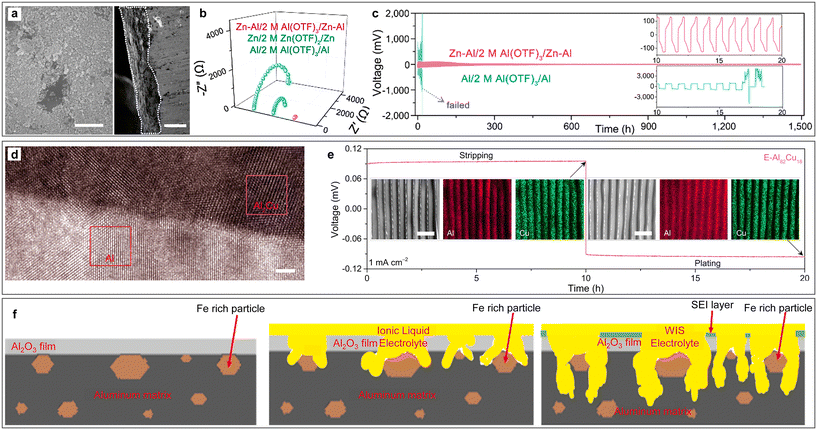 |
| Fig. 6 Aluminium alloy for ARAMBs. (a–c) Zn–Al alloy. (a) SEM images of the top view and cross section of Zn–Al alloy. Scale bars: 500 nm (left) and 30 μm (right). (b) Comparative Nyquist plots of symmetrical cells with different electrodes. (c) Comparison of long-term cycling stability for symmetric cells based on Al and Zn–Al alloy electrodes (0.2 mA cm−2). Reproduced with permission.64 Copyright 2020, AMER CHEMICAL SOC. (d and e) Eutectic Al82Cu18 alloy. (d) HRTEM image of Al/Al2Cu interfacial region. Scale bars: 2 nm. (e) Stripping/plating voltage profile of E-Al82Cu18 symmetric cells at a current density of 1 mA cm−2 in 2 M Al(OTF)3 aqueous electrolytes. Insets: SEM images and corresponding EDS mappings of Al and Cu after stripping (left) and plating (right). Scale bars: 1 μm. Reproduced with permission.66 Copyright 2022, NATURE PORTFOLIO. (f) Schematic illustration of aluminium matrix corrosion during the cycling process in the presence of Al3Fe intermetallic phase. Reproduced with permission.67 Copyright 2022, ELSEVIER. | |
Alloying elements can also be introduced through the impurities in Al metal. For example, trace iron impurities in the aluminium metal due to industrial production resulted in the precipitation of the Al3Fe intermetallic phase.67 Through SEM, it could be observed that the corrosion of the Al matrix preferentially began at the high iron content area and spread between the iron-containing intermetallic phases. The presence of Al3Fe served as a preferential site for the “water-in-salt” (WiS) electrolyte to initiate corrosion of the Al matrix, which promoted the electrolyte to break the passivation oxide barrier to access the Al bulk, and thus accelerated the electrochemical process (Fig. 6f). The above-mentioned report indicates that Al anode treatment with metallurgical techniques is crucial for the development of ARAMBs.
In conclusion, the use of aluminium alloy anodes has the merits of reducing the voltage hysteresis phenomenon in the galvanostatic test of symmetric batteries, and therefore greatly extends the cycle life of batteries. However, the use of alloy anodes usually sacrifices the discharge potential of the full cell, and thus results in a decrease in energy density. Therefore, the amount and species of alloy metal should be carefully selected.
Therefore, by designing the SEI, artificial interfacial functional layer and aluminium alloy anode, the kinetics of Al stripping process can be largely accelerated with a low overpotential in symmetric Al electrochemical cells (Table 1). However, most strategies are still not sufficiently durable given that the overpotentials inevitably increase during long cycling. Therefore, more approaches need to be developed to prolong the life of ARAMBs.
Table 1 Cyclic performance of symmetric batteries using different anode modification strategies
Electrode |
Electrolyte |
Current density (mA cm−2) |
Overpotentiala (V) |
Cycling life (h) |
Ref. |
Overpotential refers to the difference between the potential of symmetrical batteries during charging and discharging at the current density of the same row.
|
Al treated with AlCl3-[EMIm]Cl IL |
2 M Al(OTF)3 |
0.2 |
ca. 0.2 |
50 |
48
|
Al treated with AlCl3-Acm DES |
2 M Al(OTF)3 |
0.05 |
0.2 |
300 |
56
|
Al treated with AlCl3-urea ES |
2 M Al(OTF)3 |
0.1 |
<2 |
60 |
58
|
PVDF-Al |
1 M Al(OTF)3 |
0.1 |
0.5 |
100 |
59
|
Al@a–Al |
0.5 M Al2(SO4)3 |
0.05 |
0.3 |
800 |
60
|
MXene/E-Al97Ce3 |
2 M Al(OTF)3 |
0.5 |
0.05 |
1000 |
61
|
Zn–Al |
2 M Al(OTF)3 |
0.2 |
0.025 |
1000 |
64
|
E-Al82Cu18 |
2 M Al(OTF)3 |
0.5 |
0.053 |
2000 |
66
|
3 Modification of aqueous electrolytes
Aqueous electrolytes are considered suitable to realize large-scale commercial applications because of their intrinsic advantages such as high ionic conductivity, environmental friendliness, easy accessibility and low cost. As an indispensable constituent of rechargeable aluminium batteries, the electrolyte not only acts as the “ionic bridge” transporting Al3+ ions to link the cathode with the anode, but also plays crucial roles in suppressing the HER at the interface and maintaining the reactivity of the Al anode, both of which severely hinder the development and application of ARAMBs. In this section, we summarize the three main current electrolyte modification strategies for ARAMBs including highly concentrated single-salt electrolyte, highly concentrated bi-salt electrolyte, and electrolyte with additive modification. These strategies are usually combined to achieve the optimal electrochemical performance.
3.1 Highly concentrated single-salt electrolyte
The concentration of aqueous electrolytes has a decisive influence on the solvation structures, which have a great impact on the ESW, ionic conductivity and electrolyte/electrode interface. In the conventional salt concentration, the low reduction voltage of aluminium leads to the inevitable HER. In addition, the passivation film of the aluminium metal anode will rapidly be rebuilt after contacting water. With an increase in the electrolyte concentration, most water molecules will coordinate with metallic cations, and the proportion of free H2O in the electrolyte gradually decreases. Consequently, the water activity is suppressed and the ESW of the electrolyte is expanded, which will be favourable for the reversible stripping/deposition of Al3+ on the aluminium anode.
As a commonly used electrolyte salt in ARAMBs, aluminium trifluoromethanesulfonate (Al(OTF)3) was used to prepare highly concentrated “water-in-salt” electrolytes in 2019.68 The overall stable window for 5 M Al(OTF)3 aqueous electrolyte is in the range of −0.3 to 3.3 V (vs. Al/Al3+). Based on the WiS-Al(OTF)3 electrolyte, the AlxMnO2·nH2O cathode delivered a specific capacity of 467 mA h g−1 and energy density of 481 W h kg−1. In addition to transition metal oxides, the WiS strategy is also used for PBA cathodes. Zhou et al. demonstrated a high-capacity PBA-type FeFe(CN)6 cathode with double transition metal redox sites in a similar WiS-Al(OTF)3 electrolyte.69 The LSV result showed that the WiS-Al(OTF)3 electrolyte exhibited an electrochemical window of 2.65 V (Fig. 7a, cathodic side: 1.65 V vs. Ag/AgCl and anodic side: −1 V vs. Ag/AgCl). The Tafel plot of H2 evolution also showed that the overpotential of H2 in WiS-Al(OTF)3 electrolyte was higher than that in other electrolytes (Fig. 7b), which also confirmed the suppressed reactivity of water in the WiS-Al(OTF)3 electrolyte. The authors further noted that the water molecules in the WiS-Al(OTF)3 electrolyte were not sufficient for the formation of two hydrate shells with Al3+, and thus the electrolyte was dominated by loose ion pairs (LIP) and intimate ion pairs. The fitting result of the Raman spectra showed that the free anion fraction decreased to zero after the concentration of the electrolyte increased to 5 M, while the LIP fraction also increased correspondingly. Therefore, it was concluded that water activity was suppressed due to the strong cation–anion and cation–water interaction in the WiS-Al(OTF)3 electrolyte. Similarly, a potassium nickel hexacyanoferrate cathode also demonstrated stable cycling up to 500 cycles and maintained its 3D framework structure in the WiS-Al(OTF)3 electrolyte.70
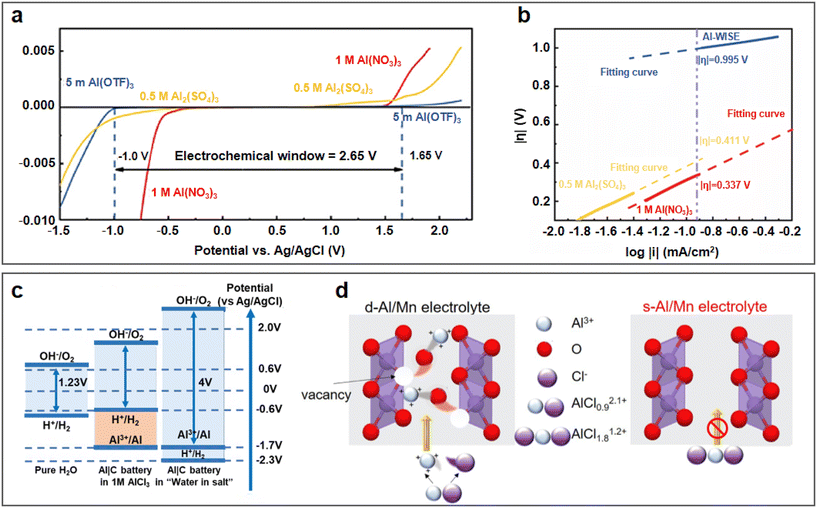 |
| Fig. 7 Highly concentrated single-salt electrolyte. (a and b) WiS-Al(OTF)3 electrolyte. (a) Linear sweep voltammetry of aqueous electrolytes on titanium mesh in a three-electrode system at 10 mV s−1 using electrolytes of 5 M Al(OTF)3, 1 M Al(NO3)3, and 0.5 M Al2(SO4)3, respectively. (b) Tafel plot of H2 evolution in different electrolytes. Reproduced with permission.69 Copyright 2019, AMER CHEMICAL SOC. (c and d) WiS-AlCl3 aqueous electrolytes. (c) Schematic illustration of an expanded ESW for WiS-AlCl3 electrolyte. Reproduced with permission.71 Copyright 2019, the ROYAL SOCIETY OF CHEMISTRY. (d) Schematic illustration of highly-concentrated electrolyte inhibiting electrochemical dissolution of MnO2. Reproduced with permission.72 Copyright 2022, ELSEVIER. | |
In addition to Al(OTF)3, AlCl3 also exhibits excellent solubility in aqueous solution, and thus has been used for the preparation of highly concentrated electrolytes. A WiS-AlCl3 electrolyte (mass ratio of AlCl3·6H2O to H2O was 12) with an ESW of greater than 4 V was used in an Al/graphite full battery.71 The CV tests showed that the HER voltage in the WiS-AlCl3 electrolyte shifted to less than −2.3 V compared to −0.6 V in the 1 M AlCl3 solution (vs. Ag/AgCl) (Fig. 7c). The lower HER voltage in the WiS-AlCl3 electrolyte allowed Al3+ ions to be reduced and deposited on the aluminium anode at about −1.7 V (vs. Ag/AgCl), which was not possible in the dilute solution. Due to the reversible stripping/deposition of the Al anode in the WiS-AlCl3 electrolyte, the Al/graphite full battery achieved a stable life of more than 1000 charge/discharge cycles with a high capacity retention of 99%, even at high current densities (500 mA g−1). When matching with the α-MnO2 cathode, a high discharge voltage of 1.9 V combined with a high discharge capacity of 285 mA h g−1 could be achieved, contributing to a high discharge energy density of 370 W h kg−1 (based on the mass of MnO2).73 The WiS-AlCl3 electrolyte not only contributed to the reversible stripping/deposition behaviour of the Al anode but also the cycling stability of the cathode. The solvation structure of the Al3+ cations in the saturated electrolyte (composed of 4.4 M AlCl3 and 1 M MnCl2 in H2O) was larger than in the dilute electrolyte (composed of 1 M AlCl3 and 1 M MnCl2 in H2O), as shown in Fig. 7d, which avoided the intercalation of solvated cations with larger volumes into the MnO2 cathode, and thereby facilitated the stability of the Al/MnO2 battery.72
3.2 Highly concentrated bi-salt electrolyte
As mentioned above, by increasing the concentration of the electrolyte, the interaction between cations and water molecules is enhanced, thus reducing the activity of water. In this case, to obtain a higher concentration of electrolyte with a lower content of free water molecules, researchers have developed highly concentrated bi-salt electrolytes, which have been applied in the field of ARAMBs.74
A water in bi-salt electrolyte containing supporting Li-salt at a high concentration (1 M Al(OTF)3 + 15 M LiOTF) with a greatly enlarged electrochemical window of up to 4.35 V was reported in 2021.75 Using an AlxMnO2 cathode, the bi-salt electrolyte enabled a discharge capacity of 160 mA h g−1 after 150 cycles with CE of ca. 95%. Similarly, a dual-salt electrolyte with 5 M Al(OTF)3 and 0.5 M LiOTF was proposed with MnO as the cathode.74 By changing the supporting Li-salt to lithium bis(trifluoromethane sulfonyl)imide (LiTFSI), the resulting bi-salt electrolyte consisting of 2 M Al(OTF)3 and 20 M LiTFSI exhibited an outstanding electrochemical window of close to 3 V.76 The linear sweep voltammetry of the Al/Mo cells clearly demonstrated the effect of LiTFSI on the electrochemical stability of the aqueous electrolyte (Fig. 8a). With an increase in LiTFSI concentration, the blue shift of the peak at 765 cm−1 in the Raman spectra indicated the formation of a considerable amount of contact ion pairs (Fig. 8b). In general, ultra-high concentrations of LiTFSI resulted in a reduction in free H2O around Al3+ (Fig. 8c), thus achieving an inhibitory effect on HER. In addition to Li salts, supporting Ca-salts, such as Ca(OTF)2, could also be added to the Al(OTF)3 aqueous solution to broaden its electrochemical window.77 AlCl3 has also been employed to develop high-performance bi-salt electrolytes. Li et al. proposed a series of AlCl3/LiTFSI aqueous electrolytes with different molar ratios and evaluated their electrochemical performance.78 The LSV curves (Fig. 8d) suggested that the HER activity in the bi-salt electrolyte with 1 M AlCl3 and 9 M LiTFSI is lower than that in aqueous solutions of other common aluminium salts. The solvation structures in the electrolyte were further investigated by molecular dynamic simulations (Fig. 8e). A portion of water molecules participated in the primary solvation sheath of cations, while others interacted with each other via hydrogen bonds. The increase in LiTFSI concentration led to an increase in the proportion of water molecules involved in the cationic solvation structures. When the concentration of LiTFSI in the electrolyte increased to 15 M, about 97% of the water molecules coordinated with cations (Fig. 8f). The significantly reduced amount of free water molecules helped to suppress the activity of HER reaction, thereby effectively improving the overall performance of the batteries.
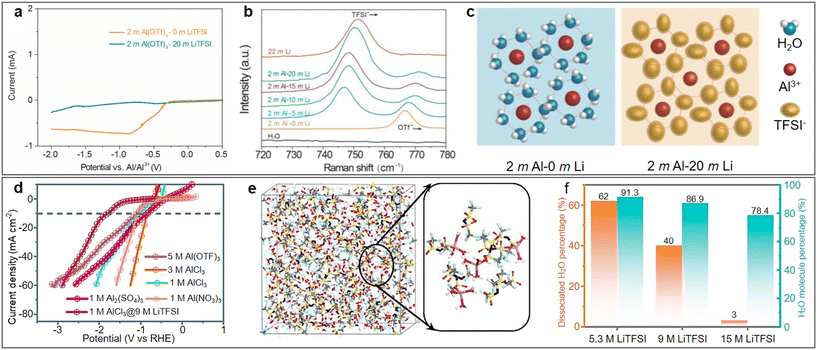 |
| Fig. 8 Highly concentrated bi-salt electrolyte. (a–c) 2 M Al(OTF)3 + 20 M LiOTF electrolyte. (a) Linear sweep voltammetry of Al/Mo cells with different electrolytes. (b) Raman spectra of the electrolytes with varying LiTFSI concentrations. (c) Schematic diagram of solvation structures in bi-salt electrolyte. Reproduced with permission.76 Copyright 2023, KEAI PUBLISHING LTD. (d–f) AlCl3 + LiTFSI electrolyte. (d) Comparison of different electrolytes in suppressing HER activity. (e) Snapshot of local structure in 0.5 M AlCl3 + 15 M LiTFSI electrolyte. (f) Proportion of dissociated H2O and total H2O in electrolytes containing different concentrations of LiTFSI. Reproduced with permission.78 Copyright 2022, the ROYAL SOCIETY OF CHEMISTRY. | |
3.3 Electrolyte additives
To achieve the optimal electrochemical performance, some electrolyte additives have been added to regulate the chemical environment (for example, pH) in combination with the high-concentration strategy.79 In aqueous Al/S batteries, it has been reported that the formation of a passivation layer on the Al anode surface could be effectively prevented by adding 0.02 M HCl as an additive to the bi-salt electrolyte (1 M Al(OTF)3 + 17 M LiTFSI).80 Similarly, the addition of 1 M H3PO4 to the WiS-Al(OTF)3 electrolyte effectively induced the Al3+ ions to be bonded to both H2PO4− and OTF− to form complex cations, Al(H2PO4−)x(OTF−)y + (H2O)n, which could mitigate the strong charge densities of the trivalent Al3+ and hinder the formation of a passivating film.81 In addition to changing the chemical environment, some electrolyte additives of soluble low-valence salts of the corresponding cathode metal have been added to inhibit the dissolution of the cathode in the electrolyte. For example, the addition of 0.1 M of Mn(OTF)2 to 2 M Al(OTF)3 electrolyte could effectively increase the discharge capacity and cycle life of TAl/MnO2 full cells, thus achieving more than 100 reversible cycles at a current density of 200 mA g−1.48
In summary, increasing the concentration of solutes in aqueous electrolytes plays a significant role in broadening their electrochemical window by regulating the solvation structure of Al3+ ions. However, the high salt concentration also increases the overall cost of the battery, and fluorinated salts such as Al(OTF)3 and LiOTF also increase the environmental concerns for subsequent recycling and treatment. In addition, the reported studies on ARAMBs rarely demonstrated the CE of the aluminium metal stripping/deposition behaviour, which is a key challenge for the further development and practical application. Therefore, it may be more feasible to further develop other aqueous electrolyte modification strategies, such as introducing more functional additives or constructing aqueous/organic mixed solvents,82–84 to achieve highly reversible electrochemical stripping/deposition behaviour in the aluminium metal by regulating the hydrogen bonding and Al3+ ion solvation structure of the electrolyte at lower electrolyte concentrations. Moreover, due to the intricate interfacial issue associated with the Al anode, a synergistic effect between the modification of the anode and electrolyte is usually required to suppress the HER process and facilitate the Al stripping process, simultaneously.
4 Design of cathode materials
ARAMBs are known as promising energy storage devices with a high energy and power density, owing to their three-electron electrochemical mechanism and the small ionic radius of Al3+. However, in practical applications, the high charge density and large hydrated ionic radius of Al3+ induce sluggish diffusion dynamics and large electrostatic forces between the host framework and Al3+, leading to the collapse of the cathode materials. Therefore, most cathodes of ARAMBs encounter the challenge of poor cycle stability and slow kinetics. In general, the current research on the cathodes of ARAMBs is still in its infancy, which mainly include vanadium-based materials, manganese-oxide materials, molybdenum-based materials, PBAs, carbon materials and organic materials.
4.1 Vanadium-based materials
Considering their merits of abundant valence states, vanadium-based materials are routinely selected for the fabrication of aqueous batteries, exhibiting a high specific capacity. In recent years, a variety of vanadium-based materials have been studied as cathodes of ARAMBs, including V2O5, VO2-B, VOPO4, FeVO4, KVO, and NH4V4O10.49,55,78,85–89 In 2019, the electrochemical performance and charge storage mechanism of an orthorhombic V2O5 cathode were first studied by Zhao et al. through experimental and theoretical analysis.49 In acidic 2 M Al(OTF)3 electrolyte (pH ≈ 2), V2O5 undergoes reversible protonation rather than Al-ion intercalation reaction, providing a specific capacity of 186 mA h g−1 (Fig. 9a and b). DFT calculation revealed the stable structure evolution after one proton uptake. Furthermore, the authors found that the intercalation/de-intercalation chemistry depends on the ion concentration and charge density of ions in the electrolyte. Bronze-type vanadium dioxide (VO2-B) with a 2D holey nanobelt morphology was also designed as a cathode of AABs.85 The large 4.984 Å × 3.281 Å tunnel allowed reversible intercalation/de-intercalation of both Al3+ and H+ (Fig. 9c), enabling a high capacity of 234 mA h g−1 at 150 mA g−1 (Fig. 9d) and a capacity retention of 77.2% after 1000 cycles at 1 A g−1. It is worth mentioning that the electrochemical performance was characterized in a three-electrode system with a high concentration of Al(OTF)3 (5 M). The robust bilayer shear structure and holey nanobelt morphology could not only relieve the lattice stress and volume variation in the discharge/charge cycles but also facilitate the electrolyte/electrode contact with shortened ion diffusion paths. In addition to intercalation-type cathodes, conversion-type V-based cathodes materials have also attracted interest from researchers. For example, the FeVO4 cathode reacts with Al3+ through a transformation from FeVO4 phase to spinel AlxVyO4 and amorphous Fe–O–Al phase, delivering an initial capacity of 350 mA h g−1 in the three-electrode system with 1 M AlCl3 electrolyte.86 However, the capacity rapidly decayed with cycling, which is attributed to the surface reaction between the electrolyte and FeVO4. The formed V2O5 is soluble in acidic electrolyte. In summary, the low operation voltage, sluggish kinetics and poor cycling stability of V-based cathodes are their major issues in AABs. Simultaneously, their cycling performance and reaction mechanism are usually affected by the pH of the electrolyte.
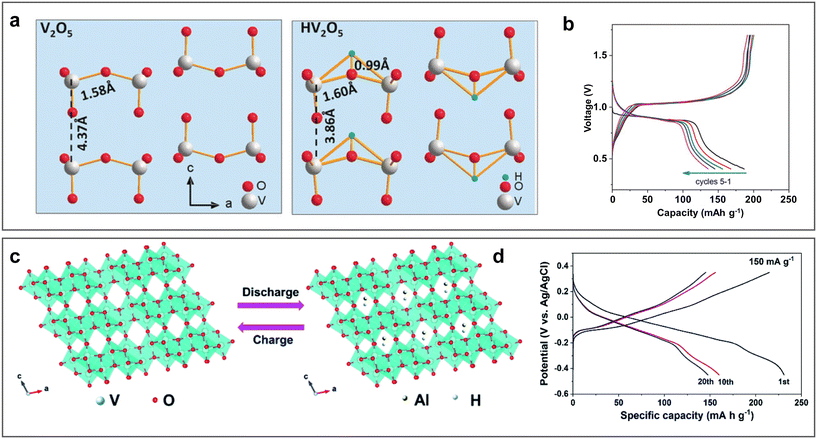 |
| Fig. 9 Vanadium-oxide cathode materials. (a and b) Orthorhombic V2O5. (a) Structure of pristine V2O5 before (left) and after (right) proton intercalation. (b) Galvanostatic charge/discharge (GCD) curves of Al/V2O5 ARAMBs at 40 mA g−1 with electrolyte of 2 M Al(OTF)3. Reproduced with permission.49 Copyright 2020, WILEY-VCH VERLAG GMBH. (c and d) Bronze-type vanadium dioxide (VO2-B). (c) Schematic diagram of H+ and Al3+ intercalation/de-intercalation in VO2-B during the discharging and charging processes. (d) GCD curves of Al/VO2-B ARAMBs at 150 mA g−1 with electrolyte of 5 M Al(OTF)3. Reproduced with permission.85 Copyright 2020, the ROYAL SOCIETY OF CHEMISTRY. | |
4.2 Manganese-oxide materials
Generally, manganese-oxide cathode materials have the advantages of low cost, high specific capacity, and high operational voltage plateau, but encounter issues such as complicated mechanism and poor stability. In 2018, α-MnO2 nanorods were first reported for ARAMBs in cooperation with an aqueous Al(OTF)3 electrolyte and IL-treated Al anode, displaying a reversible electrochemical discharge/charge process with an initial specific capacity of 380 mA h g−1 (Fig. 10b), corresponding to an energy density of about 500 W h kg−1 (based on the mass of α-MnO2).48 The transmission electron microscopy (TEM) images (Fig. 10a) unveiled the formation of a highly crystalline MnO2 core coated by amorphous low-valence Mn oxide shell after discharge. In addition, 0.1 M Mn(OTF)2 was also introduced in the electrolyte to substantially reduce the dissolution of low-valence Mn and improve the cycling stability. Some researchers believed that the pre-addition of Mn2+ electrolyte leads to a reversible dissolution/deposition process of the cathode materials. Birnessite MnO2 was found to reduce to Mn2+ in the first discharge process in Al(OTF)3 electrolyte with the pre-addition of 0.5 M MnSO4, and then amorphous AlxMn1−xO2 was generated in the following charge process.90 In the following cycles, it experienced a reversible dissolution/deposition process.
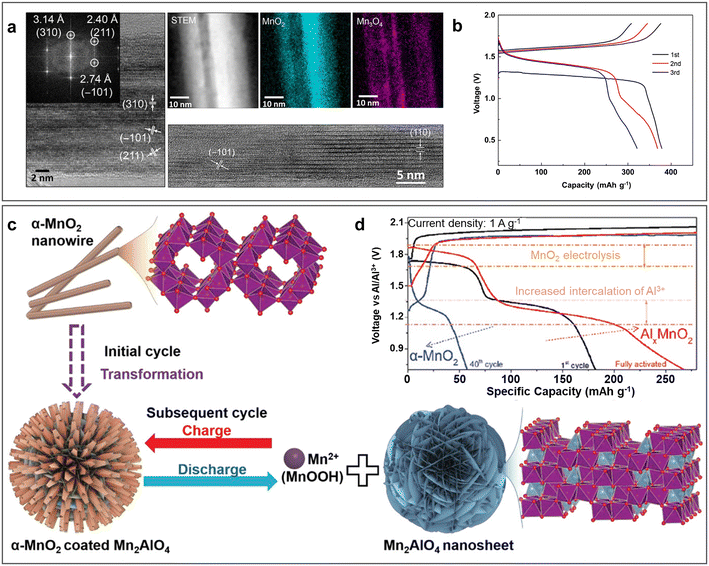 |
| Fig. 10 Manganese-oxide cathode materials. (a and b) α-MnO2 cathode. (a) HRTEM image of α-MnO2 cathode with the fast Fourier transform (FFT) inset (left) before and (right) after discharge with corresponding EELS mapping. (b) GCD curves of Al/α-MnO2 ARAMBs at 100 mA g−1 with electrolyte of 2 M Al(OTF)3. Reproduced with permission.48 Copyright 2018, AMER ASSOC ADVANCEMENT SCIENCE. (c and d) Phase transformation α-MnO2 nanowire cathode. (c) Schematic diagram of transformation from α-MnO2 nanowire to AlxMnO2. (d) GCD curves of Al/α-MnO2 ARAMBs at 1 A g−1 with WiS-AlCl3/MnSO4 electrolyte. Reproduced with permission.73 Copyright 2021, WILEY-VCH VERLAG GMBH. | |
In response to the reaction mechanism, there are some reports suggesting that the mechanisms of the first cycle are different from the following cycles. In the initial cycle, Mn oxides, such as Mn3O4, MnO, and MnO2, are converted to aluminium-containing manganese oxides (AlxMnO2), while Al is not completely extracted in subsequent cycles.64,68,73 For instance, the spinel Mn3O4 precursor transformed to the mix layered and amorphous phase of AlxMnO2·nH2O during the first charge to 1.8 V in 5 M Al(OTF)3 aqueous electrolyte.68 The experimental characterization and DFT calculation proved the mechanism that Mn2+ in the tetrahedral sites and a part of Mn3+ at the octahedral sites dissolved in the electrolyte and the Al3+ preferred to insert in the vacant sites due to its low formation energy. It exhibited a specific capacity of 467 mA h g−1 mainly from the reversible intercalation/de-intercalation of Al3+. Similarly, the MnO precursor generated AlxMnO2via topological conversion between rock-salt MnO and MnO2, where Mn2+ was partly oxidized to Mn4+ and rearranged into the MnO2 phase, while the other Mn2+ dissolved.64 In addition, α-MnO2 nanowires were reported to transform into two phases in the first cycle with the insertion/extraction of Al3+ in WiS-AlCl3/MnSO4 electrolyte, which included Mn2AlO4 nanosheets coated by the α-MnO2 nanorods (Fig. 10c).73 Then, the reversible electrolysis of MnO2 together with the deintercalation of AlCl4− and the intercalation of Al3+ into Mn2AlO4 contributed to the 1.9 V and 1.2 V discharge plateaus, respectively, in the subsequent cycles. This unique structure enabled a specific of 285 mA h g−1 at 500 mA g−1 and a high CE of over 95% after 500 cycle at 5 A g−1 (Fig. 10d).
To improve the structural stability, a spinel-structured cathode was reported. Al2/3Li1/3Mn2O4 (ALMO) was synthesized from an LiMn2O4 precursor in 1 M AlCl3/NaCl mixed electrolyte, where Al3+ inserted in the lattice and occupied some of the Li+ sites.91 The diffusion tunnel of the stable spinel structure provided an excellent rate performance and cycling stability with the reversible intercalation mechanism of Al3+. It exhibited about 145 mA h g−1 at the high current density of 1 A g−1 with 63% capacity retention after 1000 cycles in Al/AlCl3-NaCl/ALMO cells. The authors noted that the decrease in capacity mainly happened in the initial dozens of cycles due to the dissolution of the cathodes. The defect engineering and cation substitution strategy was also reported to provide an impressive cycle performance in cathodes in the field of ARAMBs. In 2023, Yang et al. synthesized cobalt-substituted Mn1−xCoxO2 2D nanosheets and obtained Mn1−x−yCox
yO2 by modulating the defects.92 According to the experimental and calculation analyses, they revealed that the disorder stacking of nanosheets and porous and crumpled structure enhanced the electrode/electrolyte contact, while the Co-substitution and Mn vacancies significantly improved the electronic conductivity, ion diffusion kinetics of the materials, and effectively inhibited the dissolution of Mn in 2 M Al(OTF)3 aqueous electrolyte. It exhibited a remarkable specific capacity of 585 mA h g−1 with an energy density of 685 W h kg−1 (based on the mass of the cathode and anode) and high cycling stability (78% over 300 cycles). Overall, the energy density of Mn-based materials is comparatively high among AABs cathodes. Currently, research is focused on inhibiting Mn dissolution and improving the structural stability. In the future, loading carbon materials and synthesizing hydrogel electrolytes may be feasible strategies to further improve the electrochemical performance.
4.3 Molybdenum-based materials
Molybdenum-based materials such as MoO3 exhibit layered structures, which have been investigated as electrode materials due to their characteristic of accommodating various ions with fast kinetics. In 2022, oxygen-deficient MoO3 nanobelts (OD-MoO3−x) were prepared via the hydrothermal approach and demonstrated the reversible H+ intercalation/de-intercalation mechanism in 1 M AlCl3 electrolyte (pH ≈ 2.4).93 The DFT calculations in combination with experimental characterization indicated the formation of monoclinic H0.6MoO3 phase after the first charge process due to the incomplete de-intercalation of H+ in the initial cycle. Therefore, the following cycles experienced the reversible phase transitions of H0.6MoO3 ↔ H1.68MoO3 ↔ H2MoO3 (Fig. 11a). Given that the expansion of the interlayer is caused by the deficiency of oxygen, it achieved an excellent rate capability (97 mA h g−1 at the high current density of 50 A g−1, Fig. 11b) with a capacity retention of 67 mA h g−1 over 10
000 cycles. The electrochemical storage mechanism of oxygen-deficient MoO3 nanosheets (D-MoO3) was further investigated by Huang et al. DFT calculation demonstrated that Al(H2O)43+ was easily absorbed on the (020) surface, while H3O+ inserted into D-MoO3 through the Grotthuss mechanism.94 Subsequently, H and OH dissociated from H3O+ formed bonds with O and Mo, respectively. In addition to MoO3, two-dimensional molybdenum dichalcogenide layered materials have also been reported, in which hexagonal MoTe2 with an interlayer spacing of 0.69 nm was investigated as an electrochemical storage material for multiple ions.95 In a three-electrode electrochemical system coupled with 1 M AlCl3 electrolyte, MoTe2 demonstrated the optimal performance with a specific capacity retention of 100 mA h g−1 at 1 A g−1 over 250 cycles (Fig. 11d). The conversion-type electrochemical mechanism, 3MoTe2 + 4Al3+ + 12e− ↔ 3Mo + 2Al2Te3, was illustrated via ex situ XRD and XPS analysis of the MoTe2 cathode before and after initial discharge and charge (Fig. 11c). However, a disadvantage of the molybdenum-based electrode materials is their low discharge potential, which limits the energy density of AABs. Nevertheless, they also enable the possibility of constructing an aqueous Al-ion battery with their use as anodes and Mn oxides as cathodes.
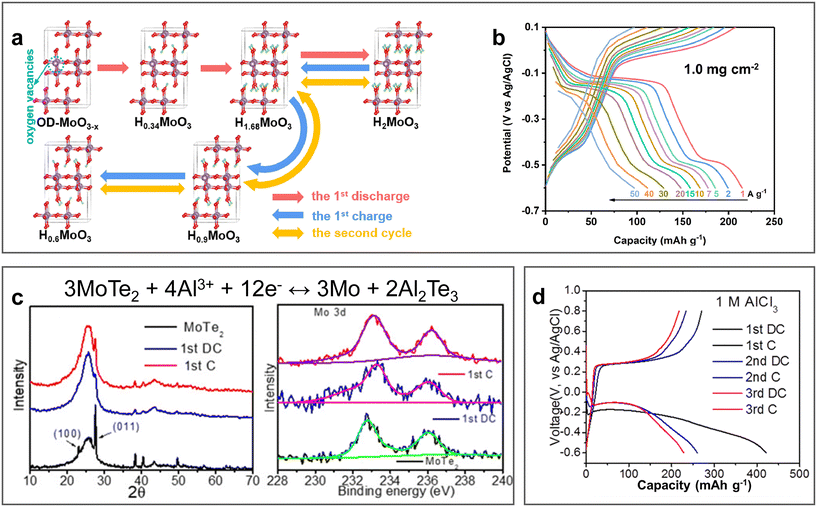 |
| Fig. 11 Molybdenum-based cathode materials. (a and b) Oxygen-deficient MoO3 cathode (OD-MoO3−x). (a) Schematic diagram of H+ intercalation/de-intercalation mechanism in OD-MoO3−x. (b) GCD curves for OD-MoO3−x cathode in 1 M AlCl3 electrolyte at different current densities. Reproduced with permission.93 Copyright 2022, ELSEVIER. (c and d) MoTe2 cathode. (c) Electrochemical mechanism, ex situ XRD pattern of MoTe2 and ex situ XPS spectra of Mo 3d before and after the initial discharge and charge. (d) GCD curves for MoTe2 cathode in 1 M AlCl3 electrolyte at 1 A g−1.95 Copyright 2023, the ROYAL SOCIETY OF CHEMISTRY. | |
4.4 Prussian blue analogues
PBAs are a class of coordination materials with a 3D open framework, which can be generally represented with the chemical formula of AxM1[M2(CN)6]y·nH2O, where A represents an alkali metal and M1 and M2 represent transition metals. Their large interstitial sites and diffusion tunnels can facilitate the intercalation/de-intercalation of Al3+.96 In 2015, Liu et al. first applied PBAs as cathodes of AABs (Fig. 12a).97 In a three-electrode electrochemical system, copper hexacyanoferrate (KCu[Fe(CN)6]·8H2O, CuHCF) prepared via the co-precipitation strategy exhibited a capacity of 46.9 mA h g−1 at 40 mA g−1 with a retention of 54.9% over 100 cycles in 0.5 M Al2(SO4)3 (Fig. 12b). Since then, several PBA electrodes have been reported for AABs, although most of them were studied in three-electrode electrochemical system. In general, PBAs have the advantages of simple synthesis, low cost, and high rate and cycling performance, whereas their disadvantage of low specific capacity and low discharge voltage still need to be improved.
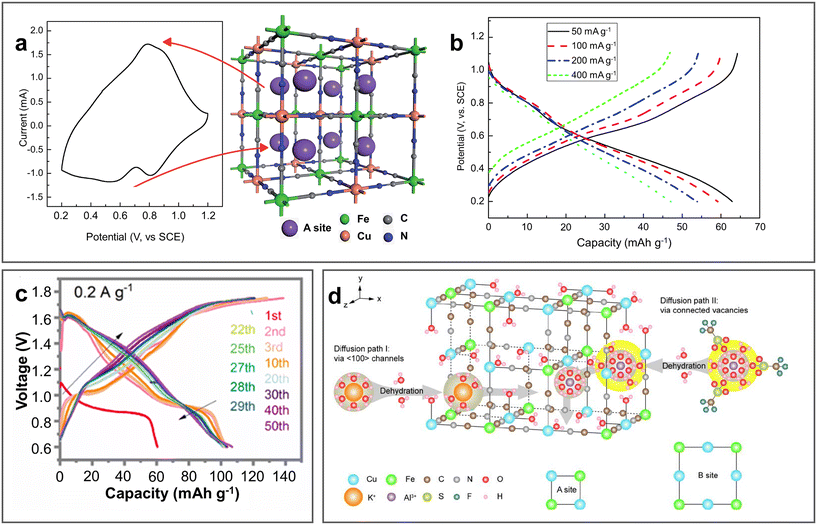 |
| Fig. 12 PBA cathode materials. (a and b) KCu[Fe(CN)6]·8H2O (CuHCF) cathode. (a) CV curve of CuHCF cathode in 0.5 M Al2(SO4)3 electrolyte with its crystal structure after Al3+ intercalation. (b) GCD curves for CuHCF in same electrolyte at various current densities. Reproduced with permission.97 Copyright 2015, the ROYAL SOCIETY OF CHEMISTRY. (c) GCD curves for Al/MnFe-PBA ARAMBs at 0.2 A g−1 with electrolyte of 1 M Al(OTF)3. Reproduced with permission.52 Copyright 2021, ELSEVIER. (d) Crystal structure of K0.03Cu[Fe(CN)6]0.65·2.7H2O (CuHCFe) with two possible diffusion channels. Reproduced with permission.98 Copyright 2023, ELSEVIER SCI LTD. | |
Vacancy modulation has been reported as an effective method to promote the rate performance and enhance the capacity through the activation of redox sites. Employing this strategy, Mn4[Fe(CN)6]2.88Δ0.29·11.8H2O (MnFe-PBA) was prepared via the co-precipitation strategy with Fe(CN)6 vacancies, which achieved a high capacity of 106.3 mA h g−1 at 0.2 A g−1 with the average discharge voltage of 1.2 V (Fig. 12c).52 Taking advantage of the two reactive metal redox sites of Fe3+/Fe2+ and Mn3+/Mn2+, MnFe-PBA showed reversible 1.2 Al3+ insertion/extraction with a change in structure between cube and monocline, and transformed to the tetragonal phase due to the Jahn–Teller effect with an increase in the content of Mn3+ after 50 cycles. Also, its cycling life could be further improved to over 200 cycles using a nano-fibrillated cellulose/polyacrylamide (NFC/PAM) gel electrolyte. As shown in Fig. 12d, K0.03Cu[Fe(CN)6]0.65·2.7H2O with two redox sites (Cu+/Cu2+, Fe3+/Fe2+) can not only provide both the (100) channels of traditional A sites and enlarged ion diffusion paths linked by Fe(CN)6 vacancies, but also shield the coulombic interactions between Al3+ and the host lattice by interstitial water.98 Consequently, it only underwent 2.5% volume change during the charge/discharge process, contributing to the outstanding rate performance (73% capacity at 50 C with average CE of 99.92%) and cycling stability (96% retention at 70 mA g−1 for over 3000 cycles) in 1 M Al(OTF)3. By comparing different aluminium salt aqueous solutions and pure acid electrolyte, the authors revealed that the type of anion instead of pH determined the electrochemical performance. Among them, OTF− exhibited the best performance (Al(OTF)3 > Al(NO3)3 > Al2(SO4)3) on account of the one order of magnitude larger diffusion coefficient of Al3+ during the faradaic processes. Morphology design is helpful to improve the electrochemical performance of PBAs. For example, the K2CoFe(CN)6 framework with highly stacked structures can decrease the length of ion transport.99 Self-assembled Fe–Co PBA with a multi-void nano-frame structure exposed plenty of active sites and relieved volumetric changes during cycling.100 In addition, preintercalation strategy was also investigated, which can effectively expand the ion diffusion channels, improve the ionic conductivity, inhibit structural changes, and enhance the cycling stability. CuHCF achieved the best performance after preintercalation in 0.1 M Al2(SO4)3·18H2O solution at 40 °C.98 It showed 1.6 times higher capacity than the original sample and 80.3% capacity retention over 5000 cycles with a high CE of nearly 100%.
The reaction mechanism and working potential of PBAs can also be affected by the electrolyte concentration. KCoFe(CN)6·5.5H2O (CoHCF) was synthesized via a simple coprecipitation method and its energy storage mechanism was investigated in saturated AlCl3 aqueous electrolyte, achieving a specific capacity of 103.5 mA h g−1.101 The experimental characterization demonstrated that CoHCF can undergo reversible Al3+ and H+ intercalation/de-intercalation together with the redox reaction of the Cl−/Cl0 pair at high potential, which was influenced by both the concentration of Al2Cl7− and the CoHCF catalyst. With an increase in the concentration of AlCl3, the discharge capacity increased in the high potential platform and reached the maximum when the concentration reached saturation. The reversible Cl−/Cl0 redox reaction promoted a high average discharge voltage of 1.65 V and high energy density of 155 W h kg−1 (based on the cathode material and Al anode), while the AlCl3 electrolyte enhance the cycling capacity (94.7% over 1200 cycles in Al/CoHCF battery).
In summary, PBAs exhibit outstanding cycling stability due to their large tunnels and small volume changes during the intercalation/de-intercalation of Al3+. However, their specific capacity needs to be improved urgently. Moreover, most of them are currently tested in the three-electrode system. Also, their electrochemical performance needs to be further investigated when matched with metal-Al anodes.
4.5 Carbon materials
Carbon materials, especially graphite-based carbon materials, are widely used as cathodes in aluminium batteries with ionic liquid electrolyte systems due to their remarkable cycling stability with high out-put voltage. In 2019, graphite was used as a cathode by Pan et al. in ARAMBs by using WiS-AlCl3 electrolyte.71 The assembled Al/WiS-AlCl3/graphite batteries displayed a high capacity retention of about 99% over 1000 cycles. Graphite was reported to exhibit a dual-ion intercalation mechanism, including the insertion of Al3+ and the extraction of AlCl4− during the discharge process, in which the voltage plateau was affected by the concentration of Cx[AlCl4] in graphite. Furthermore, a one-dimensional single-walled carbon nanotube (SWCNT) was also applied as a cathode material in WiS-AlCl3 electrolyte.102 Matching with the Al anode, SWCNT also revealed a multiple ion intercalation mechanism, realizing a capacity of 790 mA h g−1 at 5 A g−1, in which the de-intercalation of AlCl4− could provide the high discharge voltage plateau of 1.8 V and Cl− could assist the intercalation of Al3+ and H+ with an extended discharge voltage plateau at 1.2 V (Fig. 13a and b).
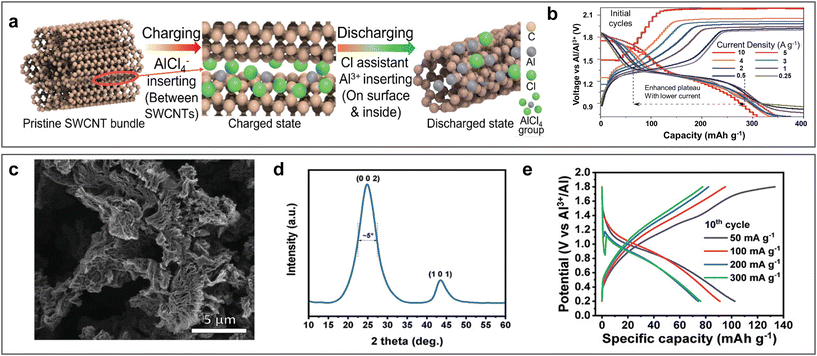 |
| Fig. 13 Carbon-based cathode materials. (a and b) Single-walled carbon nanotube cathode (SWCNT). (a) Schematic diagram of multi-ion intercalation/de-intercalation mechanism during charge/discharge process. (b) GCD for Al/SWCNT ARAMBs at various current densities with WiS-AlCl3 electrolyte. Reproduced with permission.102 Copyright 2021, WILEY-VCH VERLAG GMBH. (c and e) Reduced graphene oxide (rGO) cathode. (c) SEM image and (d) XRD of rGO. (e) GCD for Al/RGO ARAMBs at various current densities with 2 M Al(OTF)3 electrolyte. Reproduced with permission.103 Copyright 2022, WILEY. | |
In addition, two-dimensional reduced graphene oxide (rGO) synthesized by the freeze-drying method was also reported for ARAMBs with Al(OTF)3 electrolyte.103 rGO is endowed with a large interlayer spacing, small crystallite size (Fig. 13c and d) and high hydrophobicity, enabling a pseudocapacitive charge storage mechanism with an energy density of 136 W h kg−1 (based on the mass of the cathode, Fig. 13e). [Al(H2O)6]3+, as the main cation in the electrolyte, did not require desolvation during insertion and acted as a proton donor to contact with oxygen in rGO, which promoted the formation of an electrochemical double layer and stabilized the rGO structure effectively. In general, carbon materials with abundant active sites show good cycling stability. However, there are few reports on the use of carbon materials for the fabrication of ARAMBs, and their electrochemical mechanism still need to be further clarified.
4.6 Organic materials
Different from the conventional inorganic materials, where the electrochemical reaction is limited by the inner space in their crystal lattice, organic electrode materials with the merits of diverse designs, adaptable structures, and flexible frameworks have attracting increasing attention.104,105 In 2020, phenazine (PZ) was first employed as a cathode by Chen et al. for ARAMBs in 5 M Al(OTF)3 electrolyte, where a high concentration of OTF− decreased the solvation effect of H2O.106 The large conjugated system and flexible structure of PZ facilitated the intercalation/de-intercalation of large-sized ions and improved the structural stability. The C
N redox active centre was found to be reversibly coordinated with Al(OTF)2+ rather than Al3+, which could reduce the desolvation energy and coulombic interactions effectively (Fig. 14a and b). Quinone electrodes with carbonyl (C
O) as redox sites have also been investigated as cathodes for ARAMBs. By comparing a variety of quinone electrodes, Li et al. found that macrocyclic calix[4]quinone (C4Q) exhibited excellent electrochemical behaviour in terms of energy density, rate capability and low-temperature performance.53 The large cavity and sterically multi-adjacent carbonyls in C4Q made it easier for intramolecular Al(OTF)2+ coordination with small structural change, achieving a high specific capacity of 400 mA h g−1. Impressively, a pouch-type ARAMB was first assembled by using the Al/C4Q battery system, achieving a high energy density of 93 W h kg−1 with a low polarization of 42 mV (Fig. 14c–e). Benefiting from the designability of organic electrode materials, tetrachloro-1,4-benzoquinone was designed for ARAMBs.65 The inductive effect of the Cl− electron-withdrawing group increased the electrochemical activity of carbonyl, showing a specific capacity of 144 mA h g−1 through the reversible enolization of carbonyls.
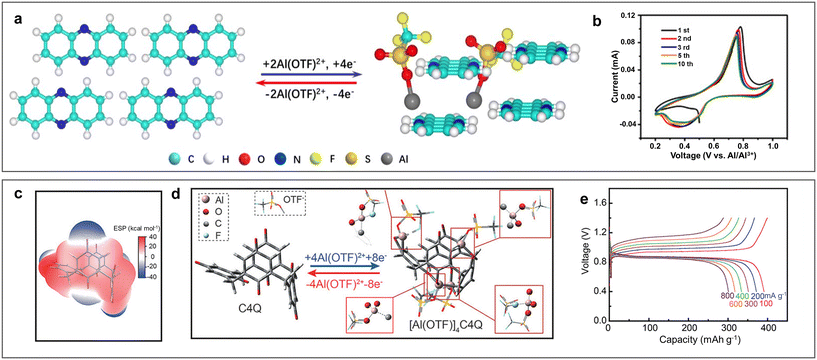 |
| Fig. 14 Organic cathode materials. (a and b) Phenazine (PZ) cathode. (a) Al(OTF)2+ storage mechanism in PZ. (b) CV curves of Al/PZ ARAMBs at 0.1 mV s−1 with electrolyte of 5 M Al(OTF)3. Reproduced with permission.106 Copyright 2021, WILEY-VCH VERLAG GMBH. (c and e) Calix[4]quinone (C4Q) cathode. (c) ESP and (d) optimized structures of C4Q before and after Al(OTF)2+ uptake. (e) GCD curves of Al/C4Q ARAMBs at various current densities with electrolyte of 1 M Al(OTF)3. Reproduced with permission.53 Copyright 2021, WILEY-VCH VERLAG GMBH. | |
Polymerization is known as an effective way to inhibit solubility and improve the electronic conductivity of organic cathodes materials. Poly(2,3-diaminophenazine) (PDAP) electropolymerized on carbon substrates exhibited high electronic conductivity and low solubility due to its long-range conjugated skeleton, as well as strong intermolecular interactions, such as hydrogen boding and π–π interaction collaboration with carbon.107 Meanwhile, PDAP is a bi-polar organic cathode with n-type (C
N) and p-type (N–H) electroactive groups, demonstrating reversible storage capability for Al3+, H+ and ClO4− with long-life stability for over 1000 cycles in Al/1 M Al(ClO4)3/PDAP cells. In addition, the nonconjugated organic electroactive material 2,2,6,6-tetramethylpiperidyl-1-oxy (TEMPO) was also investigated by in situ electrochemical characterization.108 In aqueous electrolyte, hydrolysis, ligand exchange and protonation can generate TEMPOH2+, which can result in comproportionation with TEMPO+ and allow a reversible electrochemical reaction. Poly(TEMPO methacrylate) (PTMA) provided a capacity of over 110 mA h g−1 at 4 C with a CE close to 100% and capacity retention of 77% after 800 cycles. In summary, organic cathodes have the advantage of high specific capacity, with the disadvantages of low conductivity and dissolution. Thus, combining carbon materials and polymerization is a promising strategy to improve the conductivity and resolve the dissolution problems.
In summary, the electrochemical performances of the above-mentioned types of cathodes are listed in Table 2, among which transition metal oxide materials are the most studied, carbon materials achieved the highest specific capacity, and PBAs exhibited the best cycling stability. However, it should be mentioned that the evaluation standards are not uniform, including three-electrode system and Al anodes. The cycle life is usually much higher in the three-electrode system given that the electrochemical performance is not affected by Al metal anodes. Meanwhile, the mass loading of the cathode is generally low, which needs to be further increased for practical applications.
Table 2 Electrochemical performance of ARAMBs with various cathode materials
Cathode |
Anode |
Electrolyte |
Capacity (mA h g−1, mA g−1) |
Cycling performance [retention, cycles (mA g−1)] |
Ref. |
Coin cell.
Three-electrode system.
PMMA cell.
Swagelok-type cell.
Dual electrolytic cell.
|
V2O5 |
TAl |
2 M Al(OTF)3 |
186, 20 |
65%, 50/20a |
49
|
VO2-B |
— |
5 M Al(OTF)3 |
237, 150 |
77.2%, 1000/1000b |
55
|
FeVO4 |
— |
1 M AlCl3 |
350, 60 |
10%, 20/60b |
86
|
VOPO4·2H2O |
Al |
2.5 M Al(OTF)3 |
125.4, 20 |
60%, 40/20a |
85
|
NH4V4O10 |
Zn–Al |
2 M Al(OTF)3 |
241.76, 1000 |
43%, 100/1000a |
88
|
KVO |
Al |
0.5 M AlCl3 + 12 M LiTFSI |
223, 1000 |
64.6%, 300/4000b |
78
|
α-MnO2 |
TAl |
2 M Al(OTF)3 |
380, 100 |
44%, 40/100a |
48
|
Cryptomelane MnO2 |
— |
1 M Al(NO3)3 |
109, 20 |
61.6%, 60/20b |
109
|
Bir-MnO2 |
TAl |
2 M Al(OTF)3 + 0.5 M Mn(OTF)2 |
554, 100 |
57.7%, 65/100a |
90
|
AlxMnO2·nH2O (Mn3O4) |
Al |
5 M Al(OTF)3 |
467, 30 |
58%, 50/30a |
68
|
AlxMnO2 (MnO) |
Zn–Al |
2 M Al(OTF)3 + 0.2 M Mn(OTF)2 |
460, 100 |
/a |
64
|
AlxMnO2 (α-MnO2) |
Al |
AlCl3·6H2O : MnSO4·6H2O : H2O = 4 : 1 : 1 (mass ratio) |
285, 1000 |
95%, 500/5000c |
73
|
Al2/3Li1/3Mn2O4 |
— |
1.0 M AlCl3/NaCl |
152, 100 |
67.4%, 1000/1000b |
91
|
Al |
162, 100 |
89.4%, 230/1000d |
Mn1−x−yCox yO2 |
Al |
2 M Al(OTF)3 |
585, 100 |
78%, 300/100a |
92
|
BQ-AlxMnO2 |
Zn–AL |
2 M Al(OTF)3 + 0.2 M Mn(OTF)2 |
300, 1000 |
90%, 800/1000a |
110
|
OD-MoO3−x |
— |
1 M AlCl3 |
97, 50 000 |
69.1%, 10 000/50 000b |
93
|
D-MoO3 |
— |
0.5 M Al2(SO4)3 |
167.39, 5000 |
73.6%, 1000/5000b |
94
|
MoTe2 |
— |
1 M AlCl3 |
420, 1000 |
23.8%, 250/1000b |
95
|
CuHCF |
— |
0.5 M Al2(SO4)3 |
63, 50 |
54.9%, 1000/400b |
97
|
KNHCF |
Al |
5 M Al(OTF)3 |
46.5, 20 |
52%, 500/20a |
70
|
CuHCF |
— |
1 M AlCl3 |
54.5, 1000 |
80.3%, 5000/1000b |
111
|
CuHCFe |
— |
1 M Al(OTF)3 |
69.35, 70 |
70%, 2300/350b |
98
|
K2CoFe(CN)6 |
— |
1 M Al(NO3)3 |
50, 100 |
76%, 1600/100b |
112
|
MnFe-PBA |
TAl |
1 M Al(OTF)3 |
106.3, 200 |
69.5%, 100/500a |
52
|
FF-PBA |
— |
5 M Al(OTF)3 |
97.62, 150 |
60.8%, 100/150b |
69
|
CoHCF |
Al |
Saturated AlCl3 |
103, 50 |
94.7%, 1200/250e |
101
|
Graphite |
Al |
AlCl3·6H2O : H2O = 0.8 : 1 (mass ratio) |
165, 500 |
99%, 1000/500a |
71
|
SWCNT |
Al |
AlCl3·6H2O : H2O = 10 : 1 (mass ratio) |
790/5000 |
/b |
102
|
rGO |
TAl |
2 M Al(OTF)3 |
136, 50 |
60% 90/50a |
103
|
PZ |
Al |
5 M Al(OTF)3 |
132, 50 |
76.5% 300/50a |
106
|
C4Q |
TAl |
1 M Al(OTF)3 |
400, 40 |
81%, 50/200a |
53
|
TCQ |
Zn–Al |
1 M Al(OTF)3 |
147.7, 200 |
70.7%, 200/2000a |
65
|
PDAP |
Al |
1 M Al(ClO4)3 |
321, 100 |
65%, 1000/2000a |
107
|
PTME |
Al |
1 M Al(OTF)3 |
111, 2 C |
77%, 800/4 Cd |
108
|
5 Summary and outlook
In summary, considerable efforts have been devoted to each component of ARAMBs to stabilize their electrochemical performance. To facilitate the Al stripping process and suppress the HER reaction, the intrinsic highly passivated Al surface is removed with the formation of an ionic liquid analogue-derived SEI or artificial layer. In addition, the fabrication of Al alloys can shift the potential of the anode, and thus suppress the HER process. The design of the electrolyte is also crucial to reduce the parasitic reactions and maintain long cycle stability in batteries. Building highly concentrated electrolytes and adding additives that are widely used in other aqueous batteries are also feasible for ARAMBs. Finally, due to the high charge density of the Al3+ ion, the cathode materials are mostly vanadium-based materials, manganese-oxide materials, molybdenum-based materials, PBAs, carbon materials and organic materials. The reaction can be based on the conversion, intercalation or charge compensation mechanism, where Mn-based and organic cathodes generally exhibit a higher specific capacity than other materials. However, although intriguing progress has been made, the development of ARAMBs is still in its infancy and a few issues need more attention in future studies (Fig. 15).
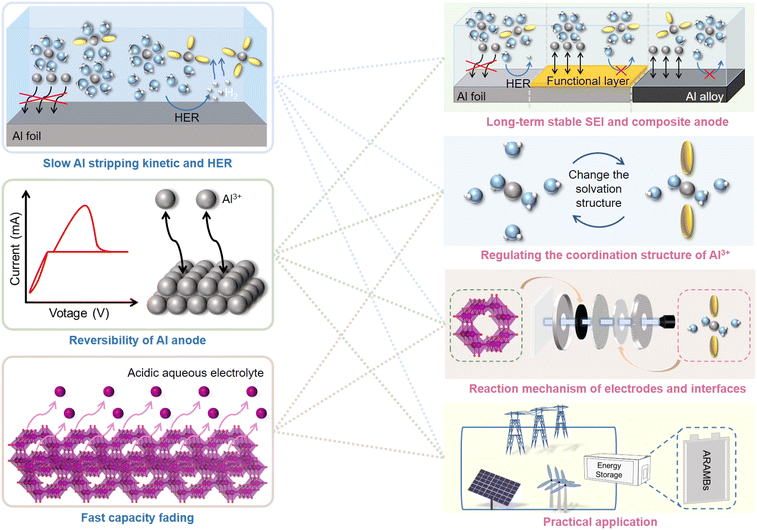 |
| Fig. 15 Summary and outlook of rechargeable aqueous aluminium metal batteries. | |
1) Simultaneously meeting the requirements of fast Al stripping kinetics and suppression of the HER process. The dense passivation film with a high band-gap on the Al anode is known to cause sluggish kinetics for Al stripping, thus leading to a low operation voltage in ARAMBs. Also, although the kinetics improves when the passivation film is removed, it also usually accelerates the generation of H2.
2) The reversibility of Al anode under aqueous electrolyte during repeated cycling. The CE of Al stripping/plating in IL-based electrolytes such as AlCl3-[EMIm]Cl has reached over 99%. However, the reversibility in aqueous electrolyte is inferior and leads to the continuous consumption of Al during cycling.
3) The fast capacity fading on the cathode side in acidic aqueous aluminium electrolyte. Given that most Al salts such as Al(OTF)3, Al2(SO4)3, and AlCl3 are known as strong acid and weak base salts, low pH makes the design of cathodes difficult. Most metal oxide cathodes are soluble during the discharge process, leading to low cycling life in batteries. Meanwhile, the high charge density of Al3+ leads to large electrostatic interaction with the lattice and high volume change, resulting in a poor kinetic performance and low reversibility.
Due to the ever-growing ratio of renewable energy in electric power generation, its intermittent characteristic accelerates the need for electrochemical energy storage systems. In this case, the emerging ARAMBs are endowed with several merits, including high abundance Al resource, high specific capacity of Al anode, cost efficiency and improved safety of aqueous electrolytes, which are promising candidates in the future smart grid if their electrochemical performance can be further enhanced. We prospect that further studies should be focused on the following aspects.
1) Developing long-term stable SEI and composite anodes. As a metal anode, the repetitive stripping/plating process during the discharge/charge process will lead to the rupture of the interphase, resulting in the formation of a highly passivated oxide interphase. Accordingly, the construction of an inorganic/polymer interphase may be a possible strategy to resolve this issue, in which inorganic compounds provide decent ionic conductivity to transport Al3+ and elastic polymers can accommodate the volume changes during the Al stripping/plating process.
2) Regulating the coordination structure of Al3+ in aqueous electrolytes. It is known that coordinated H2O is easily transferred to the anode side in the presence of an electric field and inducing the HER process. In this case, the introducing of an organic component in the electrolyte that is prone to coordination with Al3+ seems to be a possible approach to suppress the HER reaction. When the organic component moves to the anode side with Al3+, its reduction will generate a new interphase rather than H2. In addition, the coordination structure of Al3+ has a significant influence on the reversibility of electrochemical plating on the metal anode. Therefore, the development of strategies that can effectively change the coordination structure of Al3+ is the key to improving the CE of Al stripping/plating. Finally, the current strategy of increasing the concentration of salt will lead to an increase in the cost of ARAMBs. Therefore, it is also important to further develop modification strategies at low concentrations to meet cost requirements.
3) Unveiling the ambiguous reaction mechanism of electrodes and interfaces. Given that various ions are present in the aqueous electrolyte, the cathode can react with H+, Al3+, Al(OTF)22+, Al(H2O)63+, Al2Cl7−, etc. during the discharge process, making it difficult to design stable cathodes for ARAMBs. Simultaneously, the presence of various ions also makes it difficult to clearly study the mechanism of the electrochemical deposition of Al. In combination with advanced operando characterizations and theoretical calculations, we expect that the reason for different reaction mechanisms at electrodes and interfaces with various types of cathode materials and electrolytes can be illustrated, thus providing fundamental principles for the future design of ARAMBs.
4) Evaluating the electrochemical performance of ARAMBs under practical conditions. Currently, the electrochemical tests of ARAMBs are usually performed under the conditions of coin cells or even three-electrode systems, in which most side reactions are ignored. Thus, by evaluating the behaviour under practical conditions, for example, low anode to cathode capacity ratio, high cathode loading, lean electrolytes, and high overall capacity (>1 Ah), we can better demonstrate whether the designed batteries are suitable for commercial applications. Through the simultaneous progress on the anode, electrolyte, and cathode, the overall electrochemical performance of ARAMBs can be further enhanced to satisfy the requirement of future energy storage/conversion systems.
Data availability statement
No primary research results, software or code have been included and no new data were generated or analysed as part of this review.
Conflicts of interest
The authors declare no conflict of interest.
Acknowledgements
This work was supported by the National Natural Science Foundation of China (no. 52201259 and 22372083), the National Key R&D Program of China (2021YFB2500300), the Natural Science Foundation of Tianjin (no. 22JCZDJC00380), and the Young Elite Scientist Sponsorship Program by CAST.
References
- B. Dunn, H. Kamath and J. M. Tarascon, Electrical energy storage for the grid: A battery of choices, Science, 2011, 334, 928–935 CrossRef CAS PubMed.
- P. J. Heptonstall and R. J. K. Gross, A systematic review of the costs and impacts of integrating variable renewables into power grids, Nat. Energy, 2021, 6, 72–83 CrossRef.
- Z. X. Zhu, T. L. Jiang, M. Ali, Y. H. Meng, Y. Jin, Y. Cui and W. Chen, Rechargeable batteries for grid scale energy storage, Chem. Rev., 2022, 122, 16610–16751 CrossRef CAS.
- Z. Yang, J. Zhang, M. C. W. Kintner-Meyer, X. Lu, D. Choi, J. P. Lemmon and J. Liu, Electrochemical energy storage for green grid, Chem. Rev., 2011, 111, 3577–3613 CrossRef CAS.
- J. B. Goodenough, How we made the Li-ion rechargeable battery, Nat. Electron., 2018, 1, 204–204 CrossRef.
- J. W. Choi and D. Aurbach, Promise and reality of post-lithium-ion batteries with high energy densities, Nat. Rev. Mater., 2016, 1, 16013 CrossRef CAS.
- S. Xin, X. Zhang, L. Wang, H. J. Yu, X. Chang, Y. M. Zhao, Q. H. Meng, P. Xu, C. Z. Zhao, J. H. Chen, H. C. Lu, X. R. Kong, J. L. Wang, K. Chen, G. Huang, X. B. Zhang, Y. Su, Y. Xiao, S. L. Chou, S. L. Zhang, Z. P. Guo, A. B. Du, G. L. Cui, G. J. Yang, Q. Zhao, L. B. Dong, D. Zhou, F. Y. Kang, H. Hong, C. Y. Zhi, Z. Z. Yuan, X. F. Li, Y. F. Mo, Y. Z. Zhu, D. F. Yu, X. C. Lei, J. X. Zhao, J. Y. Wang, D. Su, Y. G. Guo, Q. Zhang, J. Chen and L. J. Wan, Roadmap for rechargeable batteries: Present and beyond, Sci. China: Chem., 2023, 67, 13–42 CrossRef.
- N. Yabuuchi, K. Kubota, M. Dahbi and S. Komaba, Research development on sodium-Ion batteries, Chem. Rev., 2014, 114, 11636–11682 CrossRef CAS PubMed.
- Y.-F. Zhu, Y. Xiao, S.-X. Dou, Y.-M. Kang and S.-L. Chou, Spinel/Post-spinel engineering on layered oxide cathodes for sodium-ion batteries, eScience, 2021, 1, 13–27 CrossRef.
- J. Muldoon, C. B. Bucur and T. Gregory, Quest for nonaqueous multivalent secondary batteries: Magnesium and beyond, Chem. Rev., 2014, 114, 11683–11720 CrossRef CAS PubMed.
- D. L. Chao, W. H. Zhou, F. X. Xie, C. Ye, H. Li, M. Jaroniec and S. Z. Qiao, Roadmap for advanced aqueous batteries: From design of materials to applications, Sci. Adv., 2020, 6, eaba4098 CrossRef CAS.
- Q. Zhao, W. W. Huang, Z. Q. Luo, L. J. Liu, Y. Lu, Y. X. Li, L. Li, J. Y. Hu, H. Ma and J. Chen, High-capacity aqueous zinc batteries using sustainable quinone electrodes, Sci. Adv., 2018, 4, eaao1761 CrossRef.
- L. Miao, Y. K. Lv, D. Z. Zhu, L. C. Li, L. H. Gan and M. X. Liu, Recent advances in zinc-ion hybrid energy storage: Coloring high-power capacitors with battery-level energy, Chin. Chem. Lett., 2023, 34, 107784 CrossRef CAS.
- Z. Cai, J. Wang and Y. Sun, Anode corrosion in aqueous Zn metal batteries, eScience, 2023, 3, 100093 CrossRef.
- J. Ren, H.-Y. Wu, W. Yan, P. Huang and C. Lai, Stable zinc anode by regulating the solvated shell and electrode–electrolyte interface with a sodium tartrate additive, Ind. Chem. Mater., 2024, 2, 328–339 RSC.
- Y. Y. Liu, G. J. He, H. Jiang, I. P. Parkin, P. R. Shearing and D. J. L. Brett, Cathode design for aqueous rechargeable multivalent ion batteries: Challenges and opportunities, Adv. Funct. Mater., 2021, 31, 2010445 CrossRef CAS.
- Q. Li and N. J. Bjerrum, Aluminum as anode for energy storage and conversion: A review, J. Power Sources, 2002, 110, 1–10 CrossRef CAS.
- Y. Liu, Q. Sun, W. Li, K. R. Adair, J. Li and X. Sun, A comprehensive review on recent progress in aluminum–air batteries, Green Energy Environ., 2017, 2, 246–277 CrossRef.
- D. Peramunage and S. Licht, A solid sulfur cathode for aqueous batteries, Science, 1993, 261, 1029–1032 CrossRef CAS PubMed.
- D. Peramunage, R. Dillon and S. Licht, Investigation of a novel aqueous aluminum/sulfur battery, J. Power Sources, 1993, 45, 311–323 CrossRef CAS.
- P. Ding, J. Zhang, N. Han, Y. Zhou, L. Jia and Y. Li, Simultaneous power generation and CO2 valorization by aqueous Al–CO2 batteries using nanostructured Bi2S3 as the cathode electrocatalyst, J. Mater. Chem. A, 2020, 8, 12385–12390 RSC.
- S. Zaromb, The use and behavior of aluminum anodes in alkaline primary batteries, J. Electrochem. Soc., 1962, 109, 1125 CrossRef CAS.
- N. Jayaprakash, S. K. Das and L. A. Archer, The rechargeable aluminum-ion battery, Chem. Commun., 2011, 47, 12610–12612 RSC.
- M. C. Lin, M. Gong, B. G. Lu, Y. P. Wu, D. Y. Wang, M. Y. Guan, M. Angell, C. X. Chen, J. Yang, B. J. Hwang and H. J. Dai, An ultrafast rechargeable aluminium-ion battery, Nature, 2015, 520, 324–328 CrossRef CAS.
- E. Faegh, B. Ng, D. Hayman and W. E. Mustain, Practical assessment of the performance of aluminium battery technologies, Nat. Energy, 2021, 6, 21–29 CrossRef CAS.
- G. Wang, E. Dmitrieva, B. Kohn, U. Scheler, Y. Liu, V. Tkachova, L. Yang, Y. Fu, J. Ma, P. Zhang, F. Wang, J. Ge and X. Feng, An efficient rechargeable aluminium–amine battery working under quaternization chemistry, Angew. Chem., Int. Ed., 2022, 61, e202116194 CrossRef CAS PubMed.
- D. J. Kim, D.-J. Yoo, M. T. Otley, A. Prokofjevs, C. Pezzato, M. Owczarek, S. J. Lee, J. W. Choi and J. F. Stoddart, Rechargeable aluminium organic batteries, Nat. Energy, 2019, 4, 51–59 CrossRef CAS.
- Z. Huang, X. Du, M. Ma, S. Wang, Y. Xie, Y. Meng, W. You and L. Xiong, Organic cathode materials for rechargeable aluminum-ion batteries, ChemSusChem, 2023, 16, e202202358 CrossRef CAS PubMed.
- H. Chen, H. Y. Xu, S. Y. Wang, T. Q. Huang, J. B. Xi, S. Y. Cai, F. Guo, Z. Xu, W. W. Gao and C. Gao, Ultrafast all-climate aluminum-graphene battery with quarter-million cycle life, Sci. Adv., 2017, 3, eaao7233 CrossRef PubMed.
- Z. J. Lin, M. L. Mao, C. X. Yang, Y. X. Tong, Q. H. Li, J. M. Yue, G. J. Yang, Q. H. Zhang, L. Hong, X. Q. Yu, L. Gu, Y. S. Hu, H. Li, X. J. Huang, L. M. Suo and L. Q. Chen, Amorphous anion-rich titanium polysulfides for aluminum-ion batteries, Sci. Adv., 2021, 7, eabg6314 CrossRef CAS PubMed.
- Q. Pang, J. Meng, S. Gupta, X. Hong, C. Y. Kwok, J. Zhao, Y. Jin, L. Xu, O. Karahan, Z. Wang, S. Toll, L. Mai, L. F. Nazar, M. Balasubramanian, B. Narayanan and D. R. Sadoway, Fast-charging aluminium–chalcogen batteries resistant to dendritic shorting, Nature, 2022, 608, 704–711 CrossRef CAS PubMed.
- C. Xu, T. Diemant, A. Mariani, M. E. Di Pietro, A. Mele, X. Liu and S. Passerini, Locally concentrated ionic liquid electrolytes for wide-temperature-range aluminum-sulfur batteries, Angew. Chem., Int. Ed., 2024, 63, e202318204 CrossRef CAS PubMed.
- C. Xu, M. Zarrabeitia, Y. Li, J. Biskupek, U. Kaiser, X. Liu and S. Passerini, Three-dimensional nitrogen-doped carbonaceous networks anchored with cobalt as separator modification layers for low-polarization and long-lifespan aluminum–sulfur batteries, ACS Nano, 2023, 17, 25234–25242 CrossRef CAS PubMed.
- Y. Guo, W. Wang, H. Lei, M. Wang and S. Jiao, Alternate storage of opposite charges in multisites for high-energy-density Al–MOF batteries, Adv. Mater., 2022, 34, 2110109 CrossRef CAS PubMed.
- Q. Zhao, J. X. Zheng, Y. Deng and L. Archer, Regulating the growth of aluminum electrodeposits: towards anode-free Al batteries, J. Mater. Chem. A, 2020, 8, 23231–23238 RSC.
- Y. H. Heo, J. Lee, S. Ha, J. Chan Hyun, D. H. Kang, J. Yoon, H. S. Kim, Y. Choi, Y. Chan Kang, H.-J. Jin, S. J. Kim and Y. S. Yun, 3D-structured bifunctional MXene paper electrodes for protection and activation of Al metal anodes, J. Mater. Chem. A, 2023, 11, 14380–14389 RSC.
- J. Yoon, S. Moon, S. Ha, H.-K. Lim, H.-J. Jin and Y. S. Yun, Nanoconfinement effect of nanoporous carbon electrodes for ionic liquid-based aluminum metal anode, J. Energy Chem., 2022, 74, 121–127 CrossRef CAS.
- S. Ha, J. C. Hyun, J. H. Kwak, H.-D. Lim, B. S. Youn, S. Cho, H.-J. Jin, H.-K. Lim, S. M. Lee and Y. S. Yun, Waste-induced pyrolytic carbon nanotube forest as a catalytic host electrode for high-performance aluminum metal anodes, Chem. Eng. J., 2022, 437, 135416 CrossRef CAS.
- J. Y. Shi, J. Zhang and J. Guo, Avoiding pitfalls in rechargeable aluminum batteries research, ACS Energy Lett., 2019, 4, 2124–2129 CrossRef CAS.
- X. Ma, J. Yu, Y. Hu, J. Texter and F. Yan, Ionic liquid/poly(ionic liquid)-based electrolytes for lithium batteries, Ind. Chem. Mater., 2023, 1, 39–59 RSC.
- G. A. Elia, K. Marquardt, K. Hoeppner, S. Fantini, R. Y. Lin, E. Knipping, W. Peters, J. F. Drillet, S. Passerini and R. Hahn, An overview and future perspectives of aluminum batteries, Adv. Mater., 2016, 28, 7564–7579 CrossRef CAS PubMed.
- Q. Zhao, S. Stalin and L. A. Archer, Stabilizing metal battery anodes through the design of solid electrolyte interphases, Joule, 2021, 5, 1119–1142 CrossRef CAS.
- S. Liu, J. J. Hu, N. Yan, G. Pan, G. Li and X. Gao, Aluminum storage behavior of anatase TiO2 nanotube arrays in aqueous solution for aluminum ion batteries, Energy Environ. Sci., 2012, 5, 9743–9746 RSC.
- W. H. Zuo, W. H. Zhu, D. F. Zhao, Y. F. Sun, Y. Y. Li, J. P. Liu and X. W. Lou, Bismuth oxide: A versatile high-capacity electrode material for rechargeable aqueous metal-ion batteries, Energy Environ. Sci., 2016, 9, 2881–2891 RSC.
- T. Koketsu, J. W. Ma, B. J. Morgan, M. Body, C. Legein, W. Dachraoui, M. Giannini, A. Demortière, M. Salanne, F. Dardoize, H. Groult, O. J. Borkiewicz, K. Chapman, P. Strasser and D. Dambournet, Reversible magnesium and aluminium ions insertion in cation-deficient anatase TiO, Nat. Mater., 2017, 16, 1142–1148 CrossRef CAS PubMed.
- H. Y. Ma, H. W. Chen, Y. J. Hu, B. J. Yang, J. Z. Feng, Y. T. Xu, Y. L. Sun, H. H. Cheng, C. Li, X. B. Yan and L. T. Qu, Aqueous rocking-chair aluminum-ion capacitors enabled by a self-adaptive electrochemical pore-structure remolding approach, Energy Environ. Sci., 2022, 15, 1131–1143 RSC.
- H. Yu, C. Lv, C. Yan and G. Yu, Interface engineering for aqueous aluminum metal batteries: Current progresses and future prospects, Small Methods, 2023, 2300758 Search PubMed.
- Q. Zhao, M. J. Zachman, W. I. Al Sadat, J. X. Zheng, L. F. Kourkoutis and L. Archer, Solid electrolyte interphases for high-energy aqueous aluminum electrochemical cells, Sci. Adv., 2018, 4, eaau8131 CrossRef CAS PubMed.
- Q. Zhao, L. Liu, J. Yin, J. Zheng, D. Zhang, J. Chen and L. A. Archer, Proton intercalation/de-intercalation dynamics in vanadium oxides for aqueous aluminum electrochemical cells, Angew. Chem., 2020, 59, 3048–3052 CrossRef CAS PubMed.
- X. B. Cheng, R. Zhang, C. Z. Zhao and Q. Zhang, Toward safe lithium metal anode in rechargeable batteries: A review, Chem. Rev., 2017, 117, 10403–10473 CrossRef CAS PubMed.
- S. Kumar, V. Verma, H. Arora, W. Manalastas and M. Srinivasan, Rechargeable Al-metal aqueous battery using NaMnHCF as a cathode: Investigating the role of coated-Al anode treatments for superior battery cycling performance, ACS Appl. Energy Mater., 2020, 3, 8627–8635 CrossRef CAS.
- D. Wang, H. Lv, T. Hussain, Q. Yang, G. Liang, Y. Zhao, L. Ma, Q. Li, H. Li, B. Dong, T. Kaewmaraya and C. Zhi, A manganese hexacyanoferrate framework with enlarged ion tunnels and two-species redox reaction for aqueous Al-ion batteries, Nano Energy, 2021, 84, 105945 CrossRef CAS.
- Y. Li, L. Liu, Y. Lu, R. Shi, Y. Ma, Z. Yan, K. Zhang and J. Chen, High-energy-density quinone-based electrodes with [Al(OTF)]2+ storage mechanism for rechargeable aqueous aluminum batteries, Adv. Funct. Mater., 2021, 31, 2102063 CrossRef CAS.
- J. Smajic, A. Alazmi, N. Wehbe and P. M. F. J. Costa, Electrode–electrolyte interactions in an aqueous aluminum–carbon rechargeable battery system, Nanomaterials, 2021, 11, 3235 CrossRef CAS PubMed.
- Y. Cai, S. Kumar, R. Chua, V. Verma, D. Yuan, Z. K. Kou, H. Ren, H. Arora and M. Srinivasan, Bronze-type vanadium dioxide holey nanobelts as high performing cathode material for aqueous aluminium-ion batteries, J. Mater. Chem. A, 2020, 8, 12716–12722 RSC.
- R. F. Bai, J. Yang, G. J. Li, J. Y. Luo and W. J. Tang, Rechargeable aqueous aluminum-FeFe(CN)6 battery with artificial interphase through deep eutectic solution, Energy Storage Mater., 2021, 41, 41–50 CrossRef.
- T. Dong, K. L. Ng, Y. J. Wang, O. Voznyy and G. Azimi, Solid electrolyte interphase engineering for aqueous aluminum metal batteries: A critical evaluation, Adv. Energy Mater., 2021, 11, 2100077 CrossRef CAS.
- S. Kumar, T. Salim, V. Verma, W. Manalastas and M. Srinivasan, Enabling Al-metal anodes for aqueous electrochemical cells by using low-cost eutectic mixtures as artificial protective interphase, Chem. Eng. J., 2022, 435, 134742 CrossRef CAS.
- Q. F. Hao, F. Chen, X. T. Chen, Q. H. Meng, Y. Qi and N. Li, Bi-functional poly(vinylidene difluoride) coated Al anodes for highly rechargeable aqueous Al-ion batteries, Electrochim. Acta, 2022, 421, 140495 CrossRef CAS.
- C. S. Yan, C. D. Lv, B. E. Jia, L. X. Zhong, X. Cao, X. L. Guo, H. J. Liu, W. J. Xu, D. B. Liu, L. Yang, J. W. Liu, H. H. Hng, W. Chen, L. Song, S. Z. Li, Z. Liu, Q. Y. Yan and G. H. Yu, Reversible Al metal anodes enabled by amorphization for aqueous aluminum batteries, J. Am. Chem. Soc., 2022, 144, 11444–11455 CrossRef CAS PubMed.
- Q. Ran, S. P. Zeng, M. H. Zhu, W. B. Wan, H. Meng, H. Shi, Z. Wen, X. Y. Lang and Q. Jiang, Uniformly MXene-grafted eutectic aluminum-cerium alloys as flexible and reversible anode materials for rechargeable aluminum-ion battery, Adv. Funct. Mater., 2023, 33, 2211271 CrossRef CAS.
- C. B. Breslin and W. M. Carroll, The electrochemical behaviour of aluminium activated by gallium in aqueous electrolytes, Corros. Sci., 1992, 33, 1735–1746 CrossRef CAS.
- B.-E. Jia, E. Hu, Z. Hu, J. J. Liew, Z. Hong, Y. Guo, M. Srinivasan, Q. Zhu, J. Xu, J. Chen, H. Pan and Q. Yan, Laminated tin–aluminum anodes to build practical aqueous aluminum batteries, Energy Storage Mater., 2024, 65, 103141 CrossRef.
- C. S. Yan, C. Lv, L. G. Wang, W. Cui, L. Y. Zhang, K. N. Dinh, H. T. Tan, C. Wu, T. P. Wu, Y. Ren, J. Q. Chen, Z. Liu, M. Srinivasan, X. H. Rui, Q. Y. Yan and G. H. Yu, Architecting a stable high-energy aqueous Al-ion battery, J. Am. Chem. Soc., 2020, 142, 15295–15304 CrossRef CAS PubMed.
- J. He, X. Shi, C. Wang, H. Zhang, X. Liu, Z. Yang and X. Lu, A quinone electrode with reversible phase conversion for long-life rechargeable aqueous aluminum–metal batteries, Chem. Commun., 2021, 57, 6931–6934 RSC.
- Q. Ran, H. Shi, H. Meng, S. P. Zeng, W. B. Wan, W. Zhang, Z. Wen, X. Y. Lang and Q. Jiang, Aluminum-copper alloy anode materials for high-energy aqueous aluminum batteries, Nat. Commun., 2022, 13, 576 CrossRef CAS PubMed.
- S. Arshadi Rastabi, G. Razaz, M. Hummelgård, T. Carlberg, N. Blomquist, J. Örtegren and H. Olin, Metallurgical investigation of aluminum anode behavior in water-in-salt electrolyte for aqueous aluminum batteries, J. Power Sources, 2022, 523, 231066 CrossRef CAS.
- C. Wu, S. C. Gu, Q. H. Zhang, Y. Bai, M. Li, Y. F. Yuan, H. L. Wang, X. Y. Liu, Y. X. Yuan, N. Zhu, F. Wu, H. Li, L. Gu and J. Lu, Electrochemically activated spinel manganese oxide for rechargeable aqueous aluminum battery, Nat. Commun., 2019, 10, 73 CrossRef CAS PubMed.
- A. X. Zhou, L. W. Jiang, J. M. Yue, Y. X. Tong, Q. Q. Zhang, Z. J. Lin, B. H. Liu, C. Wu, L. M. Suo, Y. S. Hu, H. Li and L. Q. Chen, Water-in-salt electrolyte promotes high-capacity FeFe(CN)6 cathode for aqueous Al-ion battery, ACS Appl. Mater. Interfaces, 2019, 11, 41356–41362 CrossRef CAS PubMed.
- Y. Gao, H. Yang, X. Wang, Y. Bai, N. Zhu, S. Guo, L. Suo, H. Li, H. Xu and C. Wu, The compensation effect mechanism of Fe–Ni mixed prussian blue analogues in aqueous rechargeable aluminum-ion batteries, ChemSusChem, 2020, 13, 732–740 CrossRef CAS PubMed.
- W. D. Pan, Y. F. Wang, Y. G. Zhang, H. Y. H. Kwok, M. Y. Wu, X. L. Zhao and D. Y. C. Leung, A low-cost and dendrite-free rechargeable aluminium-ion battery with superior performance, J. Mater. Chem. A, 2019, 7, 17420–17425 RSC.
- Y. Xu, J. L. Ma, T. L. Jiang, H. H. Ding, W. P. Wang, M. M. Wang, X. H. Zheng, J. F. Sun, Y. Yuan, M. Y. Chuai, N. Chen, Z. Y. Li, H. L. Hu and W. Chen, Tuning electrolyte solvation structures to enable stable aqueous Al/MnO2 battery, Energy Storage Mater., 2022, 47, 113–121 CrossRef.
- W. Pan, J. Mao, Y. Wang, X. Zhao, K. W. Leong, S. Luo, Y. Chen and D. Y. C. Leung, High-performance MnO2/Al battery with In Situ electrochemically reformed AlxMnO2 nanosphere cathode, Small Methods, 2021, 5, 2100491 CrossRef CAS PubMed.
- Q. Sun, L. Chai, S. Chen, W. Zhang, H. Y. Yang and Z. Li, Dual-salt mixed electrolyte for high performance aqueous aluminum batteries, ACS Appl. Mater. Interfaces, 2024, 16, 10061–10069 CrossRef CAS PubMed.
- Y. N. Gao, Y. Li, H. Y. Yang, L. M. Zheng, Y. Bai and C. Wu, Bi-salt electrolyte for aqueous rechargeable aluminum battery, J. Energy Chem., 2022, 67, 613–620 CrossRef CAS.
- W. Tang, L. Deng, L. Guo, S. Zhou, Q. Jiang and J. Luo, Reversible aqueous aluminum metal batteries enabled by a water-in-salt electrolyte, Green Energy Environ., 2024, 9, 1183–1191 CrossRef CAS.
- X. Yang, H. Gu, Q. Sun, W. Zhang and Z. Li, Synergistic co-embedding of metal ions and hydrogen protons for high stability double salt aqueous aluminum battery, Energy Storage Mater., 2023, 61, 102917 CrossRef.
- X. J. Li, Y. C. Tang, C. A. Li, H. M. Lv, H. D. Fan, W. L. Wang, T. H. Cai, Y. P. Cui, W. Xing, Z. F. Yan, C. Y. Zhi and H. F. Li, Relieving hydrogen evolution and anodic corrosion of aqueous aluminum batteries with hybrid electrolytes, J. Mater. Chem. A, 2022, 10, 4739–4748 RSC.
- Z. C. Zhao, Z. H. Zhang, T. Xu, W. B. Wang, B. F. Wang and X. B. Yu, Solvation structure regulation for highly reversible aqueous Al metal batteries, J. Am. Chem. Soc., 2024, 146, 2257–2266 CrossRef CAS PubMed.
- Z. Hu, Y. Guo, H. Jin, H. Ji and L.-J. Wan, A rechargeable aqueous aluminum–sulfur battery through acid activation in water-in-salt electrolyte, Chem. Commun., 2020, 56, 2023–2026 RSC.
- H. Lv, S. Yang, C. Li, C. Han, Y. Tang, X. Li, W. Wang, H. Li and C. Zhi, Suppressing passivation layer of Al anode in aqueous electrolytes by complexation of H2PO4− to Al3+ and an electrochromic Al ion battery, Energy Storage Mater., 2021, 39, 412–418 CrossRef.
- M. Xia, H. Fu, K. Lin, A. M. Rao, L. Cha, H. Liu, J. Zhou, C. Wang and B. Lu, Hydrogen-bond regulation in organic/aqueous hybrid electrolyte for safe and high-voltage K-ion batteries, Energy Environ. Sci., 2024, 17, 1255–1265 RSC.
- R. Tao, H. Fu, C. Gao, L. Fan, E. Xie, W. Lyu, J. Zhou and B. Lu, Tailoring interface to boost the high-performance aqueous Al ion batteries, Adv. Funct. Mater., 2023, 33, 2303072 CrossRef CAS.
- X. Ma, H. Fu, J. Shen, D. Zhang, J. Zhou, C. Tong, A. M. Rao, J. Zhou, L. Fan and B. Lu, Green ether electrolytes for sustainable high-voltage potassium ion batteries, Angew. Chem., Int. Ed., 2023, 62, e202312973 CrossRef CAS PubMed.
- Q. Pang, S. Yang, X. Yu, W. He, S. Zhang, Y. Tian, M. Xing, Y. Fu and X. Luo, Realizing reversible storage of trivalent aluminum ions using VOPO4·2H2O nanosheets as cathode material in aqueous aluminum metal batteries, J. Alloys Compd., 2021, 885, 161008 CrossRef CAS.
- S. Kumar, R. Satish, V. Verma, H. Ren, P. Kidkhunthod, W. Manalastas and M. Srinivasan, Investigating FeVO4 as a cathode material for aqueous aluminum-ion battery, J. Power Sources, 2019, 426, 151–161 CrossRef CAS.
- M. Tian, R. H. Li, C. F. Liu, D. H. Long and G. Z. Cao, Aqueous Al-ion supercapacitor with V2O5 mesoporous carbon electrodes, ACS Appl. Mater. Interfaces, 2019, 11, 15573–15580 CrossRef CAS PubMed.
- R. Liu, J. Liu, Y. Li, C. Zhang and H. Li, Aqueous aluminum-ion batteries based on layered NH4V4O10 nanosheets as cathode, Solid State Sci., 2023, 145, 107315 CrossRef CAS.
- S. Nandi, Y. Yan, X. Yuan, C. Wang, X. He, Y. Li and S. K. Das, Vanadyl ethylene glycolate: A novel organic-inorganic electrode material for rechargeable aqueous aluminum-ion battery, Solid State Ionics, 2023, 389, 116085 CrossRef CAS.
- S. He, J. Wang, X. Zhang, J. Chen, Z. Wang, T. Yang, Z. Liu, Y. Liang, B. Wang, S. Liu, L. Zhang, J. Huang, J. Huang, L. A. O'Dell and H. Yu, A High-energy aqueous aluminum-manganese battery, Adv. Funct. Mater., 2019, 29, 1905228 CrossRef CAS.
- R. C. Li, C. H. Xu, X. B. Wu, J. L. Zhang, X. Yuan, F. Wang, Q. R. Yao, M. S. Balogun, Z. G. Lu and J. Q. Deng, Aluminum-ion storage reversibility in a novel spinel Al2/3Li1/3Mn2O4 cathode for aqueous rechargeable aluminum batteries, Energy Storage Mater., 2022, 53, 514–522 CrossRef.
- J. Yang, W. Gong and F. Geng, Defect modulation in cobalt manganese oxide sheets for stable and high-energy aqueous aluminum-ion batteries, Adv. Funct. Mater., 2023, 33, 2301202 CrossRef CAS.
- W. J. Huang, K. Zhang, B. Yuan, L. C. Yang and M. Zhu, Predominant intercalation of H+ enables ultrahigh rate capability of oxygen deficient MoO3 for aqueous Al-ion batteries, Energy Storage Mater., 2022, 50, 152–160 CrossRef.
- C. Huang, Z. Jiang, F. Liu, W. Li, Q. Liang, Z. Zhao, X. Ge, K. Song, L. Zheng, X. Zhou, S. Qiao, W. Zhang and W. Zheng, Oxygen vacancies boosted hydronium intercalation: A paradigm shift in aluminum-based batteries, Angew. Chem., Int. Ed., 2024, 63, e202405592 CrossRef CAS PubMed.
- S. Nandi, Y. C. Yan, X. T. Yuan, C. Z. Wang, X. M. He, Y. Z. Li and S. K. Das, Investigation of reversible metal ion (Li+, Na+, Mg2+, Al3+) insertion in MoTe2 for rechargeable aqueous batteries, Phys. Chem. Chem. Phys., 2023, 25, 13833–13837 RSC.
- Z. Li, K. Xiang, W. Xing, W. C. Carter and Y.-M. Chiang, Reversible aluminum-ion intercalation in prussian blue analogs and demonstration of a high-power aluminum-ion asymmetric capacitor, Adv. Energy Mater., 2014, 5, 1401410 CrossRef.
- S. Liu, G. L. Pan, G. R. Li and X. P. Gao, Copper hexacyanoferrate nanoparticles as cathode material for aqueous Al-ion batteries, J. Mater. Chem. A, 2015, 3, 959–962 RSC.
- X. Li, A. Wu, C. Gao, Z. Li and S. W. Lee, Copper hexacyanoferrate as a long-life cathode for aqueous aluminum ion batteries, Mater. Today Energy, 2023, 31, 101205 CrossRef CAS.
- Y. Ru, S. Zheng, H. Xue and H. Pang, Potassium cobalt hexacyanoferrate nanocubic assemblies for high-performance aqueous aluminum ion batteries, Chem. Eng. J., 2020, 382, 122853 CrossRef CAS.
- A. Zhao, W. Chang, Y. Xiao and N. Peng, Construction of cobalt hexacyanoferrate multivoid nanoframes as efficient aqueous aluminum ion batteries, J. Alloys Compd., 2024, 976, 173101 CrossRef CAS.
- X. Yuan, X. Yuan, S. Zhang, P. Chen, X. Wu, J. Ye, L. Liu, L. Fu, T. Wang, K. I. Ozoemena and Y. Wu, An aqueous rechargeable Al-ion battery based on cobalt hexacyanoferrate and Al metal, Adv. Energy Mater., 2023, 14, 2302712 CrossRef.
- W. Pan, Y. Zhao, J. Mao, Y. Wang, X. Zhao, K. W. Leong, S. Luo, X. Liu, H. Wang, J. Xuan, S. Yang, Y. Chen and D. Y. C. Leung, High-energy SWCNT cathode for aqueous Al-ion battery boosted by multi-ion intercalation chemistry, Adv. Energy Mater., 2021, 11, 2101514 CrossRef CAS.
- J. Smajic, B. E. Hasanov, A. Alazmi, A. H. Emwas, N. Wehbe, A. Genovese, A. El Labban and P. M. F. J. Costa, Aqueous aluminum-carbon rechargeable batteries, Adv. Mater. Interfaces, 2021, 9, 2101733 CrossRef.
- B. B. Yang, Y. K. Wang, H. F. Bian, Y. L. Zhang, Y. Y. Du, H. B. Lu and D. Bin, A rocking-chair aqueous aluminum-ion battery based on an organic/inorganic electrode, Chem. Commun., 2023, 59, 8246–8249 RSC.
- J. Su, M. Zhang, H. Tian, M. Han, Z. Sun, K. Du, F. Cui, J. Li, W. Huang and Y. Hu, Synergistic π-conjugation organic cathode for ultra-stable aqueous aluminum batteries, Small, 2024 DOI:10.1002/smll.202312086.
- J. C. Chen, Q. N. Zhu, L. Jiang, R. Y. Liu, Y. Yang, M. Y. Tang, J. W. Wang, H. Wang and L. Guo, Rechargeable aqueous aluminum organic batteries, Angew. Chem., Int. Ed., 2021, 60, 5794–5799 CrossRef CAS PubMed.
- W. Wang, S. Zhang, L. Zhang, R. Wang, Q. Ma, H. Li, J. Hao, T. Zhou, J. Mao and C. Zhang, Electropolymerized bipolar poly(2,3-diaminophenazine) cathode for high-performance aqueous Al-ion batteries with an extended temperature range of −20 to 45 °C, Adv. Mater., 2024, 36, 2400642 CrossRef CAS PubMed.
- S. Jiang, Y. Xie, Y. Xie, L.-J. Yu, X. Yan, F.-G. Zhao, C. J. Mudugamuwa, M. L. Coote, Z. Jia and K. Zhang, Lewis acid-induced reversible disproportionation of TEMPO enables aqueous aluminum radical batteries, J. Am. Chem. Soc., 2023, 145, 14519–14528 CrossRef CAS PubMed.
- J. Joseph, J. Nerkar, C. Tang, A. Du, A. P. O'Mullane and K. Ostrikov, Reversible intercalation of multivalent Al3+ ions into potassium-rich cryptomelane nanowires for aqueous rechargeable Al-ion batteries, ChemSusChem, 2019, 12, 3753–3760 CrossRef CAS PubMed.
- H. Meng, Q. Ran, M. H. Zhu, Q. Z. Zhao, G. F. Han, T. H. Wang, Z. Wen, X. Y. Lang and Q. Jiang, Benzoquinone-lubricated intercalation in manganese oxide for high-capacity and high-rate aqueous aluminum-ion battery, Small, 2024, 20, 2310722 CrossRef CAS PubMed.
- J. Zheng, D. W. Li, Z. M. Feng, Y. Wang and T. Sun, Preintercalated copper hexacyanoferrate as a long-time cycle cathode material for aqueous aluminum-ion batteries, ACS Sustainable Chem. Eng., 2023, 11, 6280–6291 CrossRef CAS.
- Y. Ru, S. S. Zheng, H. G. Xue and H. Pang, Potassium cobalt hexacyanoferrate nanocubic assemblies for high-performance aqueous aluminum ion batteries, Chem. Eng. J., 2020, 382, 122853 CrossRef CAS.
Footnote |
† These authors contributed equally to this work. |
|
This journal is © Institute of Process Engineering of CAS 2025 |
Click here to see how this site uses Cookies. View our privacy policy here.