DOI:
10.1039/D4GC00846D
(Perspective)
Green Chem., 2024,
26, 4468-4476
A perspective on renewable production of amino acids from biomass through the chemocatalytic method
Received
19th February 2024
, Accepted 14th March 2024
First published on 18th March 2024
Abstract
Amino acids are an important class of fine chemicals that contain both amine groups and carboxyl groups, which are commonly used in food, medicine, and cosmetics. The conventional methods for amino acid synthesis, including the Strecker synthesis, enzymatic synthesis, and fermentation, suffer from highly toxic cyanides, non-renewable resources, enzyme instability, and high energy consumption. The development of environmentally friendly technologies for amino acid synthesis remains a formidable challenge. Biomass is the most abundant renewable resource on Earth and serves as an ideal candidate for the production of valuable chemicals and liquid fuels. This perspective highlights the potential of amino acid synthesis from biomass through the chemocatalytic method, including the catalytic conversion of biomass-derived oxygen-containing feedstocks to amino acids by introducing N-functional groups, as well as the catalytic synthesis of amino acids with chitin-derived nitrogen-containing feedstocks as starting materials. Finally, a perspective on critical challenges and future opportunities for amino acid synthesis through the chemocatalytic method is presented.
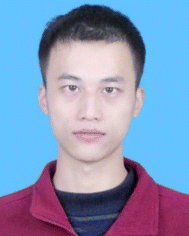 Maofeng Ding | Maofeng Ding graduated from the South China University of Technology with a Bachelor's degree in 2021. Later, he joined the research group of Prof. Xingang Li and earned a Master's degree at Tianjin University. His current research interests mainly focus on the photocatalytic conversion of biomass to high-value nitrogen-containing chemicals. |
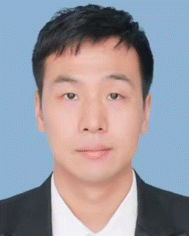 Song Song | Song Song is an Associate Professor at the School of Chemical Engineering & Technology of Tianjin University. He received his Ph.D. under the supervision of Prof. Naijia Guan and Prof. Landong Li from Nankai University in 2017. From 2017 to 2020, he worked as a research fellow in Prof. Ning Yan's group at the National University of Singapore. He joined Tianjin University as an Associate Professor in August 2020. His research interests include the thermo-/photo-catalytic conversion of biomass to valuable chemicals. |
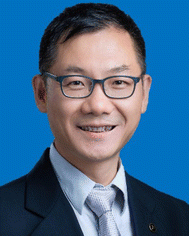 Xingang Li | Xingang Li is the Dean of the Department of Catalysis Science & Engineering and Chair Professor at Tianjin University. He received his BS (1998) and Ph.D. degrees (2003) from the University of Science & Technology of China. After finishing postdoctoral research at IRCELyon (France) and the University of Patras (Greece), in 2007 he joined the School of Chemical Engineering & Technology of Tianjin University. His research interests include energy catalysis and environmental catalysis. He has published more than 160 articles, served as the Associate Editor of “Journal of Chemical Technology & Biotechnology”, and was awarded the “Young Scientist Award” by the International Association of Catalysis Societies. |
1 Introduction
Amino acids are the building blocks of proteins, which are widely used in the food and pharmaceutical industries.1 The global market for amino acids is $26.55 billion in 2022 and is expected to reach $57.58 billion by 2032.2 This increasing demand necessitates low carbon and highly efficient technologies for amino acid synthesis to meet increasingly stringent environmental quality standards in the chemical industry. The current methods for the production of amino acids mainly include chemical and biological processes. The Strecker synthesis is a well-known chemical method for the production of α-amino acids. However, it utilizes highly toxic cyanides as nitrogen sources and non-renewable aldehydes as substrates. Moreover, an additional step for optical resolution of DL-amino acids is inevitable.3,4 The industrial production of amino acids mainly depends on the biological fermentation process, but it suffers from low efficiency, high costs, and enzyme instability, as well as high energy consumption.5,6 Given the aforementioned challenges in amino acid synthesis, it is highly desirable to develop efficient and green methods for the production of amino acids from renewable feedstocks.
Biomass is a renewable organic carbon resource, and is a potential alternative to the dwindling fossil fuel reserves. Lignocellulose is the most abundant biomass on Earth, which can be easily converted into various oxygen-containing platform molecules, such as lactic acid and furfural.7–15 Chitin is the second most abundant biopolymer with an annual production of 100 Gt, consisting of N-acetylglucosamine (NAG) and glucosamine units linked by β-1,4-glycosidic bonds.16 In the past few decades, great advances have been achieved in the catalytic conversion of biomass to valued chemicals and liquid fuels.6,17–25 However, the “functional group gap” between amino acids and biomass constituents has made the production of amino acids from biomass notoriously difficult, as amino acids simultaneously contain amine groups and carboxyl groups, which are absent in biomass constituents.
In 2018, Yan's group pioneered a novel chemocatalytic method for synthesizing α-amino acids through the amination of lignocellulose-derived α-hydroxyl acids using NH3 over Ru-based heterogeneous catalysts.11 A series of α-amino acids, including alanine, leucine, valine, aspartic acid, and phenylalanine, were efficiently obtained.11–13 Apart from α-hydroxyl acids, glycerol and furfural were also reported recently as starting substrates for preparing alanine and proline, respectively.14 Meanwhile, the production of amino acids, such as glucosaminic acid and tyrosine, from the natural nitrogen-containing polymer chitin has also been demonstrated through the chemocatalytic method.15,26
The chemocatalytic method with biomass as a renewable feedstock has great potential to improve the synthesis efficiency and reduce carbon footprint in amino acid synthesis.27–30 Currently, the number of review articles on renewable production of nitrogen-containing chemicals from biomass is vast. However, the literature lacks an overview on the production of amino acids from biomass. While this method is still in its infancy, it is highly desirable to give a comprehensive overview of amino acid synthesis, which is the subject of this perspective article. This perspective starts with a brief introduction on existing methods for amino acid synthesis. Then, we mainly focus on two categories for the production of amino acids from biomass through the chemocatalytic method (Fig. 1). The first is to catalytically convert biomass-derived oxygen-containing feedstocks to amino acids by introducing N-functional groups. The second section involves utilizing chitin-derived nitrogen-containing feedstocks as starting materials for the catalytic synthesis of amino acids. In the end, a number of challenges and opportunities are highlighted for the chemocatalytic amino acid synthesis from biomass.
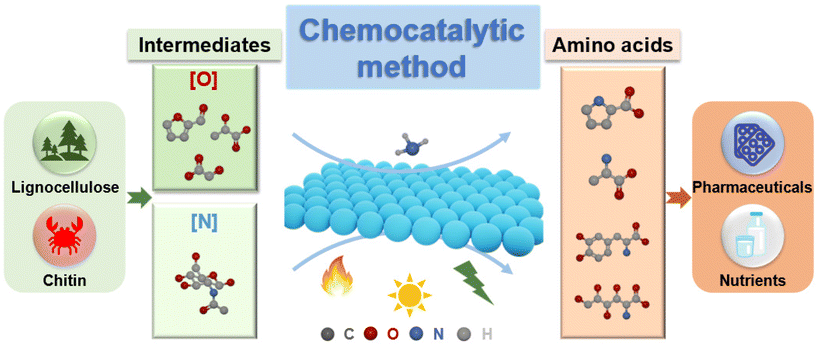 |
| Fig. 1 Production of amino acids from biomass through the chemocatalytic method. | |
2 Existing methods for amino acid synthesis
2.1 Chemical synthesis
The Strecker synthesis is the oldest known chemical synthesis of α-amino acids, as reported by Adolph Strecker in 1850 (Fig. 2).32 It begins with the condensation of NH3 to an aldehyde, forming an imine. Afterwards, the addition of a cyanide ion to an imine produces an α-aminonitrile. This is then hydrolyzed to yield racemic α-amino acid under acidic conditions. By varying the R group on the aldehyde, a series of α-amino acids are synthesized through the Strecker synthesis.33,34 To address the issue of chirality, the asymmetric Strecker synthesis has been developed.35–39 For example, Zuend et al.3 utilized a chiral amino-thiourea catalyst that was compatible with aqueous cyanide salts to achieve the asymmetric Strecker synthesis of unnatural α-amino acids. Although the Strecker synthesis has been well studied, the utilization of highly toxic cyanide sources inevitably limits its practical application.3 So far, only a limited number of amino acids have been commercially produced through this method. For example, methionine, used as feed additives in industrial livestock farming, can be produced by the Strecker synthesis using methyl mercaptan, acrolein and hydrogen cyanide. The entire process has been ongoing at Evonik Degussa in Germany for 50 years and contributed to 60% of the world's methionine capacity in 2014.40
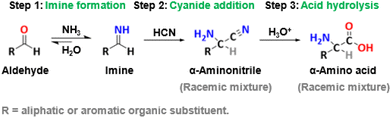 |
| Fig. 2 The Strecker synthesis of α-amino acids.3,31 | |
2.2 Biological synthesis
Biological synthesis can be divided into two categories: enzymatic synthesis and fermentation process. Enzymatic synthesis means the utilization of enzymes to facilitate the production of specific amino acids from proteins. For example, the efficient production of alanine, leucine, and glutamic acid from dried fish protein has been achieved with the combination of alcalase and neutrase enzymes.41 One notable advantage of enzymatic synthesis is the production of optically pure D- or L-amino acids at high substrate concentrations while minimizing the by-product formation.5 Nonetheless, enzymes are costly and lack stability, rendering them unsuitable for the large-scale industrial production of amino acids. To date, the commercial applications of enzymatic synthesis for the production of amino acids, such as L-aspartic acid and L-alanine, have had only limited success.42
Currently, the industrial production of amino acids mainly depends on the fermentation process (Fig. 3). Corynebacterium glutamicum (C. glutamicum) and Escherichia coli (E. coli) are two of the most commonly used bacteria in this process.43 These two bacteria have the ability to generate a wide range of amino acids, and various metabolic engineering modifications have also been implemented to enhance their efficiency in amino acid synthesis. For example, the genetically modified C. glutamicum was successfully employed for high-yield production of L-lysine and L-glutamic acid.44,45 The modified E. coli has been developed to facilitate the production of valuable aromatic amino acids such as L-tryptophan, L-phenylalanine, and L-tyrosine.46 Notably, the fermentation process exclusively produces L-form amino acids, thus obviating additional purification steps. Furthermore, it can be operated under mild conditions to prevent product degradation. However, the fermentation process necessitates sterile conditions, and suffers from high energy consumption, low production efficiency, as well as high capital and operational costs.47
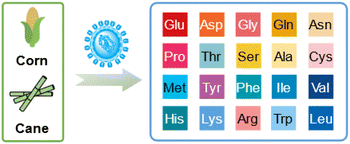 |
| Fig. 3 The fermentation process for amino acid synthesis. | |
3 Amino acid synthesis through the chemocatalytic method
3.1 Lignocellulose
3.1.1 α-Hydroxyl acids.
α-Hydroxyl acids, containing hydroxyl and carboxyl groups, are readily available from various lignocellulose constituents. For example, lactic acid was quantitatively obtained from glucose in the presence of Ba(OH)2 at room temperature.11 Heteropolyacid catalysts could afford 49% glycolic acid directly from cellulose through selective oxidation.48 In principle, it seems to be a promising strategy for the production of α-amino acids from α-hydroxyl acids, in which the key chemistry is to construct an amine group –NH2via amination with the carboxyl group –COOH remaining intact. In the following section, we will introduce the recent progress in the thermocatalytic, photocatalytic, and electrocatalytic conversion of α-hydroxyl acids to α-amino acids (Fig. 4).
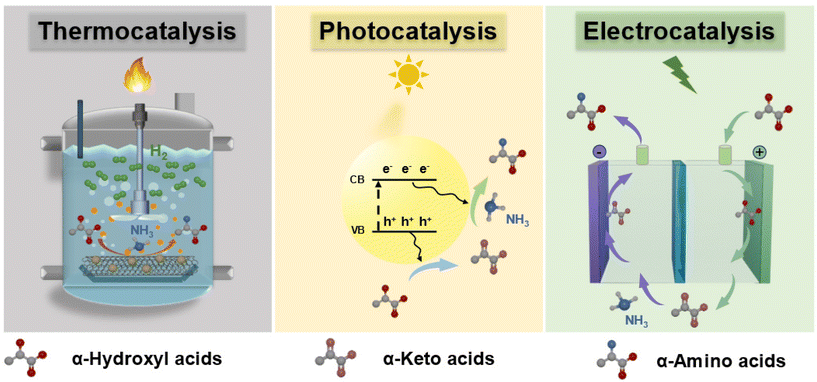 |
| Fig. 4 Thermocatalytic, photocatalytic, and electrocatalytic conversion of α-hydroxyl acids into α-amino acids. | |
Thermocatalytic system.
In 2018, Deng et al. first reported the production of α-amino acids from α-hydroxyl acids and NH3 over a thermocatalytic system.11 Using 3 wt% Ru/CNT catalyst in NH3 aqueous solution at 220 °C and 10 bar H2, alanine was efficiently obtained via the amination of lactic acid with the maximum yield of 62% (Fig. 5). Moreover, a two-step strategy was developed for the conversion of glucose to alanine in 43% yield. Afterwards, extensive research efforts were devoted to develop highly efficient thermocatalytic systems for amino acid synthesis from α-hydroxyl acids.49–52 For example, Xie et al. reported that N-doped carbon nanotube-supported Ru nanoparticles (Ru/N-CNTs) exhibited a remarkable performance in the amination of lactic acid, in which the maximum alanine yield of 70% was obtained at 180 °C and 10 bar H2 for 2 h.52 The outstanding activity of the Ru/N-CNT catalyst was mainly attributed to the highly dispersed Ru nanoparticles, the strong electronic interaction between Ru nanoparticles and N-CNTs, and the enhanced adsorption of lactic acid through basic sites. Xin et al. prepared a magnetic catalyst Ru/Ni@C by ethylene glycol reduction of metal Ru supported on encapsulated Ni@C.51 The best catalytic performance was obtained with a lactic acid conversion of 70% and an alanine yield of 64% at 200 °C and 15 bar H2 for 2 h. The formed RuO2 species, acting as Lewis acid sites, could improve the activation of the hydroxyl group of lactic acid as well as the adsorption ability of NH3, accordingly facilitating the amination of lactic acid to alanine. In addition, Wang et al. reported a one-step protocol to convert glycerol into alanine with lactic acid as an intermediate over a Ru1Ni7/MgO catalyst in the presence of NaOH.14 Alanine with a yield of 43% was obtained, which is comparable with the yield of alanine (41%) produced from microbial cultivation processes.53 The formation of Ru–Ni alloys in the Ru1Ni7/MgO catalyst promoted C–H activation in the conversion of glycerol to lactic acid and the amination of lactic acid.
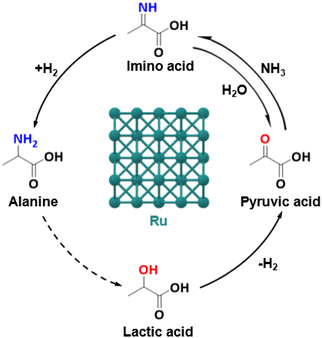 |
| Fig. 5 Thermocatalytic conversion of lactic acid and NH3 into alanine using a Ru-based catalyst.11,49,52 | |
A series of α-amino acids, including alanine, α-aminobutyric acid, valine, leucine, aspartic acid, and phenylalanine, have been successfully obtained through the thermocatalytic amination of α-hydroxyl acids. However, high reaction temperature (>180 °C) is necessary to overcome the energy barrier for the dehydrogenation of hydroxyl groups of α-hydroxyl acids, which will in turn induce the formation of side products, such as ethanol, propionic acid, and propenamide.11,49 Therefore, developing high-efficient and selective catalysts for amino acid synthesis at low temperatures is highly desirable for future research.
Photocatalytic system.
In the 1990s, attempts were made to synthesize amino acids under light irradiation. However, only commercially available semiconductors in the bulk form were investigated and their photocatalytic activities were extremely low without synthetic interest.54,55 In 2020, Song et al. prepared a series of CdS semiconductors with different morphologies and successfully applied them to the photocatalytic amino acid synthesis from α-hydroxyl acids under visible light irradiation.13 The highest alanine productivity (10.5 mmol h−1 gcat−1) was obtained over ultrathin CdS nanosheets at 50 °C and 1 bar N2, higher than that obtained in the thermocatalytic system (3.1 mmol h−1 gcat−1, 3 wt% Ru/CNT). The reaction mechanism for the photocatalytic conversion of lactic acid to alanine is shown in Fig. 6. First, the dehydrogenation of lactic acid to pyruvic acid was triggered by photogenerated holes. Then, the self-addition of pyruvic acid with NH3 to imino acid occurred. Finally, photogenerated electrons reduced imino acid to alanine.13,56 In addition, the production of H2 was regarded as a major side reaction, which would consume photogenerated electrons. Also, alanine could be further converted into alaninamide upon increasing the reaction time, resulting in low selectivity.13 Afterwards, Fu et al.56 prepared a highly fused CdS/Ti3C2 MXene Schottky junction photocatalyst, which significantly enhanced the alanine productivity (33.7 mmol g−1 h−1). The formation of the CdS/Ti3C2 Schottky junction not only enhanced the separation efficiency of photogenerated charges, but also inhibited the recombination of photoexcited electrons–holes, accordingly facilitating the photocatalytic activity.
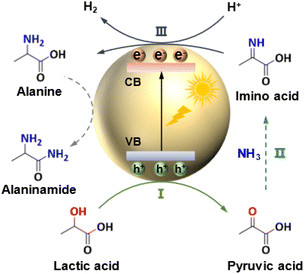 |
| Fig. 6 Photocatalytic conversion of lactic acid and NH3 into alanine.13 | |
Unlike the thermocatalytic process, photocatalysis could utilize photogenerated charge carriers to activate substrates, easily generating active radical species as key intermediates, which enables the conversion of α-hydroxyl acids to be efficiently conducted under mild conditions. Thus, amino acid synthesis through the photocatalytic system is a very green method. However, the overall yields of amino acids currently are limited (<30%) in the photocatalytic system, possibly resulting from the further photocatalytic conversion of alanine to by-products, such as alaninamide and pyruvic acid.12,13 It is highly desirable to develop highly efficient photocatalytic systems to inhibit undesired side reactions, such as H2 production.
Electrocatalytic system.
In 2022, Sun et al. reported an electrochemical strategy for synthesizing α-amino acids with high yields from α-hydroxyl acids and NH2OH or NH3.12 The electrocatalytic system mainly consists of two steps: (1) selective oxidation of α-hydroxyl acids to α-keto acids at the anode; (2) reduction of oxime (or imine) intermediates obtained from α-keto acids at the cathode. Also, a flow cell was successfully developed for the continuous conversion of α-hydroxyl acids to α-amino acids. More recently, wasted nitrogen resources (NO and NO3−) are adopted in the electrocatalytic system for synthesizing α-amino acids from α-keto acids, which are key intermediates in the amination of α-hydroxyl acids to α-amino acids. For example, Zhang et al.57 reported an electrocatalytic system for synthesizing alanine from NO and pyruvic acid. They designed a spatially decoupled two-pot electrocatalytic system that utilized flow reactors loaded with oxide-derived Ag for the production of alanine. 3.85 g of alanine with a total faradaic efficiency of 70% and a purity of >98% was obtained at a current density of 100 mA cm−2. Wang et al.58 reported the electrosynthesis of alanine with a maximum yield of 55% from pyruvic acid and NO3− catalyzed by PdCu nanobead wire (Fig. 7). The conversion of pyruvic acid and NO3− to alanine is a tandem process: NO3− was first electroreduced to the *NH2OH intermediate, which could interact with pyruvic acid to form pyruvic oxime, and then the pyruvic oxime was electroreduced to yield alanine.
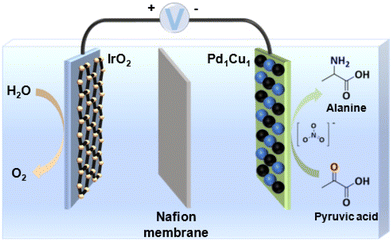 |
| Fig. 7 Electrocatalytic conversion of pyruvic acid and nitrate into alanine. Adapted and modified with permission from ref. 58. Copyright 2023 John Wiley and Sons. | |
The electrocatalytic system can separate the production of α-amino acids into two half-reactions: dehydrogenation and reductive amination, which are conducted at the anode and cathode, respectively. The main advantage of the electrocatalytic system is to prevent the further conversion of α-amino acids, which is inevitable in the reported thermocatalytic and photocatalytic systems.59–62 However, complex electrolytes, organic solvents, and a large number of organic bases are normally used in the electrocatalytic system. Also, the utilization of expensive α-keto acids as substrates is not cost-effective.
Finally, we have summarized the merits and limitations of the thermocatalytic, photocatalytic, and electrocatalytic conversion of α-hydroxyl acids to α-amino acids in Table 1.
Table 1 The merits and limitations of the thermocatalytic, photocatalytic, and electrocatalytic conversions of α-hydroxyl acids into α-amino acids
Catalytic systems |
Merits |
Limitations |
Thermocatalysis |
High efficiency |
Harsh conditions |
Large scale |
Noble metal catalysts |
Photocatalysis |
Mild conditions |
Limited yields |
Semiconductors |
Poor stability |
Electrocatalysis |
Mild conditions |
Complex electrolytes |
Continuous production |
Noble metal catalysts |
3.1.2 Furfural.
Furfural is a low-cost (1.0–1.2 € per kg) and widely available lignocellulose-derived platform molecule with an annual production of over 600 kT.63 It serves as a suitable substrate for the production of cyclic amino acids. In 2020, Song et al. reported the production of D-proline from furfural through a procedure combining chemical and biological processes (Fig. 8).64 First, a dual-layer catalytic system (Pd@S-1 + H-Beta) was designed to achieve one-step conversion of furfural to pyrrole via a tandem decarbonylation–amination reaction, obtaining an overall yield of 75% under optimal conditions. Pyrrole was then converted to DL-proline through a two-step process, involving carboxylation with CO2 and subsequent hydrogenation. After treating with E. coli, D-proline was obtained in a theoretically maximum yield of 50% with excellent ee (>99%).
 |
| Fig. 8 Chemocatalytic conversion of furfural into D-proline.64 | |
3.2 Chitin and its derivatives
Chitin contains naturally fixed nitrogen in the form of amide functionality, which renders it as an ideal resource for producing various N-containing chemicals without the addition of nitrogen sources.65 Since the concept of “shell biorefinery” was proposed by Yan et al. in 2015, various N-containing chemicals, such as amino acids, have been efficiently produced from chitin and its derivaties.16,66–73
As the monomer of chitin, NAG serves as an ideal substrate for the production of amino acids. For example, Ebitani et al. successfully achieved the aerobic oxidation of NAG to the corresponding amino acid over supported Au catalysts in water under mild conditions.74 Fukuoka et al.75 reported a two-step chemocatalytic method for the production of a commonly used amino acid in a protected form, acetylglycine, from NAG. In the first step, the catalytic conversion of NAG to N-acetyl monoethanolamine was achieved over Ru/C catalysts in NaHCO3 aqueous solution under H2 pressure.76,77 In the second step, the same combination of Ru/C- and NaHCO3-catalyzed oxidation of N-acetyl monoethanolamine to acetylglycine was achieved under an O2 atmosphere. The Ru species in Ru/C were present as small hydrous RuO2 clusters, which were identified as catalytically active sites for the oxidation of N-acetyl monoethanolamine. In addition, Dai et al. reported the tandem production of glucosaminic acid from chitosan, a partially deacetylated chitin (Fig. 9).15
 |
| Fig. 9 Chemocatalytic conversion of chitosan into glucosaminic acid. Adapted and modified with permission from ref. 15. Copyright 2019 American Chemical Society. | |
Chitin is also directly utilized for the production of amino acids. Yan and Zhou et al. reported the production of two aromatic amino acids (tyrosine and levodopa (L-DOPA)) directly from shell waste-derived chitin via an integrated biorefinery process, which involved a chemical pretreatment of chitin-containing shell waste and an enzymatic/fermentative bioprocess using metabolically engineered E. coli (Fig. 10).26 The engineered E. coli strains produced 0.91 g L−1 tyrosine or 0.41 g L−1L-DOPA from 22.5 g L−1 unpurified shell waste-derived chitin hydrolysates.
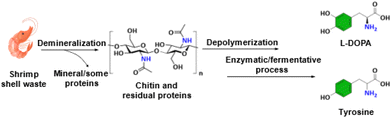 |
| Fig. 10 Conversion of shrimp shell waste-derived chitin into aromatic amino acids.26 | |
4 Conclusions and outlook
Highly efficient and green production of amino acids is of great significance in pursuit of a sustainable future. The chemocatalytic method has emerged as a promising strategy for the renewable production of amino acids from biomass, which has great potential to improve the amino acid synthesis efficiency and reduce carbon footprint. In this perspective, we introduce the significant advances that have been made in the chemocatalytic amino acid synthesis from various biomass constituents, including α-hydroxyl acids, furfural, glycerol, and chitin and its derivatives. With the remarkable progress, the chemocatalytic method will play a more important role in the field of amino acid synthesis in the future. We believe that this perspective can inspire many future studies using this method. Hence, we hope to share our perspective on the opportunities and challenges in amino acid synthesis through the chemocatalytic method.
4.1 New reaction routes
There exist many opportunities for discovering new reaction routes for producing amino acids from biomass. For example, glycine is the simplest amino acid composed of only two carbons and one amino group, which can be obtained from the oxidation of ethanolamine. Since the production of ethanolamine from cellulose has been reported,78 it has great potential to directly produce glycine from cellulose. Hemicellulose, mainly composed of 5-carbon sugars, serves as a suitable substrate for the production of 5-carbon amino acids, such as glutamic acid. Lignin, as one of the main components of lignocellulose, is the most abundant renewable aromatic source on Earth and is an ideal resource for the production of aromatic amino acids. For example, tyrosine may be synthesized from lignin with p-coumaric acid as a key building block.
Several amino acids have been successfully obtained from biomass via multi-step reactions. For example, glucosaminic acid has been obtained from chitosan via consecutive hydrolysis, deacetylation, and oxidation.15 Also, the production of proline from furfural via decarbonylation, amination, carboxylation, and hydrogenation has been demonstrated.64 However, the complex reaction procedure would inevitably induce high energy consumption, excessive carbon emissions, and expensive investment. Thus, designing novel chemocatalytic systems that enable combining the existing multiple reaction steps into a single reactor is highly desirable.
4.2 Chirality
Chirality is crucial for amino acids, especially for their application in the pharmaceutical field. However, it is still challenging to synthesize chiral amino acids through the chemocatalytic method. Developing hybrid catalytic systems seems to be a promising strategy for synthesizing chiral amino acids through the chemocatalytic method. For example, the photothermal catalytic system consisting of semiconductors as light harvesters grafted with homogeneous asymmetric catalysts has great potential to achieve the production of chiral amino acids from biomass.
The chiral resolution has been demonstrated as an effective method for separating two racemic amino acids, which includes kinetic resolution, chromatographic resolution, crystallization resolution, and membrane-based resolution.4,79 Thus, integrating this process with the chemocatalytic method can also achieve the conversion of biomass into chiral amino acids, but they have been rarely reported so far.
4.3 New catalytic systems
The key to the chemocatalytic method is the rational design and preparation of novel catalysts. Take the example of α-amino acids discussed in Fig. 4. Ru-based catalysts have been demonstrated to be effective for the thermocatalytic amination of α-hydroxyl acids to α-amino acids. Currently, the majority of existing research mainly focuses on the development of robust Ru-based catalysts via the trial-and-error method. Limited attention has been paid to explore the active sites and the related reaction mechanisms. In situ characterization studies are highly recommended to observe structural changes of catalysts under reaction conditions and provide insights into surface-adsorbed species and reaction intermediates.
CdS-based photocatalysts exhibit unique catalytic performance in the photocatalytic amination of α-hydroxyl acids to α-amino acids. However, they suffer from photocorrosion under light irradiation. Suitable strategies for improving their resistance to photocorrosion are highly desirable. Additionally, the practical application of the CdS-based photocatalytic system is impeded by the intrinsic toxicity of cadmium ions. Future research should focus on developing more robust and eco-friendly materials. For example, transition-metal (oxy)nitride photocatalysts, such as TiOxNy, which are promising materials for a wide range of redox reactions such as overall water splitting,80 may hold great potential for amino acid synthesis.
Author contributions
Xingang Li and Song Song: conceptualization, writing – review & editing, supervision, and funding acquisition. Maofeng Ding: conceptualization and writing – original draft.
Conflicts of interest
The authors declare no conflicts of interest.
Acknowledgements
We are thankful for financial support from the National Natural Science Foundation of China (Grant No. 22372115, 22102113) and the Program for Introducing Talents of Discipline to Universities of China (No. BP0618007).
References
-
N. Tonouchi and H. Ito, Amino Acid Fermentation, Springer, 2017, pp. 3–14 Search PubMed.
- Precedence Research. Amino acid market. https://www.precedenceresearch.com/amino-acids-market (accessed on 06-12-2023).
- S. J. Zuend, M. P. Coughlin, M. P. Lalonde and E. N. Jacobsen, Nature, 2009, 461, 968–970 CrossRef PubMed.
- S. Singh, B. K. Gogoi and R. L. Bezbaruah, Curr. Microbiol., 2011, 63, 94–99 CrossRef CAS PubMed.
- M. D'Este, M. Alvarado-Morales and I. Angelidaki, Biotechnol. Adv., 2018, 36, 14–25 CrossRef PubMed.
- N. Yan and Y. Wang, Chem, 2019, 5, 739–741 CAS.
- W. Schutyser, T. Renders, S. Van den Bosch, S. F. Koelewijn, G. T. Beckham and B. F. Sels, Chem. Soc. Rev., 2018, 47, 852–908 RSC.
- J. V. Vermaas, L. Petridis, J. Ralph, M. F. Crowley and G. T. Beckham, Green Chem., 2019, 21, 109–122 RSC.
- P. N. Amaniampong, Q. T. Trinh, K. De Oliveira Vigier, D. Q. Dao, N. H. Tran, Y. Wang, M. P. Sherburne and F. Jerome, J. Am. Chem. Soc., 2019, 141, 14772–14779 CrossRef CAS PubMed.
- N. M. Eagan, M. D. Kumbhalkar, J. S. Buchanan, J. A. Dumesic and G. W. Huber, Nat. Rev. Chem., 2019, 3, 223–249 CrossRef CAS.
- W. Deng, Y. Wang, S. Zhang, K. M. Gupta, M. J. Hulsey, H. Asakura, L. Liu, Y. Han, E. M. Karp, G. T. Beckham, P. J. Dyson, J. Jiang, T. Tanaka, Y. Wang and N. Yan, Proc. Natl. Acad. Sci. U. S. A., 2018, 115, 5093–5098 CrossRef CAS PubMed.
- K. Yan, M. L. Huddleston, B. A. Gerdes and Y. Sun, Green Chem., 2022, 24, 5320–5325 RSC.
- S. Song, J. Qu, P. Han, M. J. Hulsey, G. Zhang, Y. Wang, S. Wang, D. Chen, J. Lu and N. Yan, Nat. Commun., 2020, 11, 4899 CrossRef CAS PubMed.
- Y. Wang, S. Furukawa, S. Song, Q. He, H. Asakura and N. Yan, Angew. Chem., Int. Ed., 2020, 59, 2289–2293 CrossRef CAS PubMed.
- J. Dai, G. Gözaydın, C. Hu and N. Yan, ACS Sustainable Chem. Eng., 2019, 7, 12399–12407 CAS.
- H. Kobayashi, T. Sagawa and A. Fukuoka, Chem. Commun., 2023, 59, 6301–6313 RSC.
- X. Chen, S. Song, H. Li, G. Gozaydin and N. Yan, Acc. Chem. Res., 2021, 54, 1711–1722 CrossRef CAS PubMed.
- C. Zhang and F. Wang, Acc. Chem. Res., 2020, 53, 470–484 CrossRef CAS PubMed.
- Y. Jing, Y. Guo, Q. Xia, X. Liu and Y. Wang, Chem, 2019, 5, 2520–2546 CAS.
- J. V. Vermaas, M. F. Crowley and G. T. Beckham, ACS Sustainable Chem. Eng., 2020, 8, 17839–17850 CrossRef CAS.
- C. Mondelli, G. Gözaydın, N. Yan and J. Pérez-Ramírez, Chem. Soc. Rev., 2020, 49, 3764–3782 RSC.
- Y. Cui, J. He, K.-L. Yang and K. Zhou, Metab. Eng. Commun., 2020, 11, e00137 CrossRef PubMed.
- Y. Cui, J. He, K.-L. Yang and K. Zhou, J. Ind. Microbiol. Biotechnol., 2020, 47, 543–550 CrossRef CAS PubMed.
- J. Q. Bond, A. A. Upadhye, H. Olcay, G. A. Tompsett, J. Jae, R. Xing, D. M. Alonso, D. Wang, T. Zhang, R. Kumar, A. Foster, S. M. Sen, C. T. Maravelias, R. Malina, S. R. H. Barrett, R. Lobo, C. E. Wyman, J. A. Dumesic and G. W. Huber, Energy Environ. Sci., 2014, 7, 1500–1523 RSC.
- S. S. Wong, R. Shu, J. Zhang, H. Liu and N. Yan, Chem. Soc. Rev., 2020, 49, 5510–5560 RSC.
- X. Ma, G. Gozaydin, H. Yang, W. Ning, X. Han, N. Y. Poon, H. Liang, N. Yan and K. Zhou, Proc. Natl. Acad. Sci. U. S. A., 2020, 117, 7719–7728 CrossRef CAS PubMed.
- M. Pelckmans, T. Renders, S. Van de Vyver and B. F. Sels, Green Chem., 2017, 19, 5303–5331 RSC.
- Y. Wang, S. Furukawa, X. Fu and N. Yan, ACS Catal., 2019, 10, 311–335 CrossRef.
- J. Kühlborn, J. Groß and T. Opatz, Nat. Prod. Rep., 2020, 37, 380–424 RSC.
- J. He, L. Chen, S. Liu, K. Song, S. Yang and A. Riisager, Green Chem., 2020, 22, 6714–6747 RSC.
- R. E. Steiger, Org. Synth., 2003, 22, 23–23 Search PubMed.
- A. Strecker, Justus Liebigs Ann. Chem., 1850, 75, 27–45 CrossRef.
- R. J. Block, Chem. Rev., 1946, 38, 501–571 CrossRef CAS PubMed.
- S. L. Miller, J. Am. Chem. Soc., 1955, 77, 2351–2361 CrossRef CAS.
- K. Harada, Nature, 1963, 200, 1201–1201 CrossRef CAS PubMed.
- F. A. Davis, R. E. Reddy and P. S. Portonovo, Tetrahedron Lett., 1994, 35, 9351–9354 CrossRef CAS.
- H. Ishitani, S. Komiyama, Y. Hasegawa and S. Kobayashi, J. Am. Chem. Soc., 2000, 122, 762–766 CrossRef CAS.
- J. Huang and E. J. Corey, Org. Lett., 2004, 6, 5027–5029 CrossRef CAS PubMed.
- H. Yan, J. Suk Oh, J.-W. Lee and C. Eui Song, Nat. Commun., 2012, 3, 1212 CrossRef PubMed.
- T. Willke, Appl. Microbiol. Biotechnol., 2014, 98, 9893–9914 CrossRef CAS PubMed.
- V. Ramakrishnan, A. E. Ghaly, M. S. Brooks and S. M. Budge, Enzyme Eng., 2013, 2, 112 Search PubMed.
- G. Zhao, G. Gong, P. Wang, L. Wang, H. Liu and Z. Zheng, Ann. Microbiol., 2014, 64, 1615–1621 CrossRef CAS.
-
M. Ikeda, Microbial production of l-amino acids, Springer, Berlin, Heidelberg, 2003, pp. 1–35 Search PubMed.
- J. Becker, O. Zelder, S. Hafner, H. Schroder and C. Wittmann, Metab. Eng., 2011, 13, 159–168 CrossRef CAS PubMed.
- R. Aoki, M. Wada, N. Takesue, K. Tanaka and A. Yokota, Biosci. Biotechnol. Biochem., 2005, 69, 1466–1472 CrossRef CAS PubMed.
- A. Rodriguez, J. A. Martnez, N. Flores, A. Escalante, G. Gosset and F. Bolivar, Microb. Cell Fact., 2014, 13, 1–15 CrossRef PubMed.
- K. Ivanov, A. Stoimenova, D. Obreshkova and L. Saso, Biotechnol. Biotechnol. Equip., 2013, 27, 3620–3626 CrossRef CAS.
- J. Zhang, X. Liu, M. Sun, X. Ma and Y. Han, ACS Catal., 2012, 2, 1698–1702 CrossRef CAS.
- M. A. Shah, I. Khalil, S. Tallarico, T. Donckels, P. Eloy, D. P. Debecker, M. Oliverio and M. Dusselier, Dalton Trans., 2022, 51, 10773–10778 RSC.
- Y. Liu, L. Zhang, S. Feng and X. Chen, Ind. Eng. Chem. Res., 2022, 61, 10285–10293 CrossRef CAS.
- H. Xin, Z. Xiu, S. Liu, H. Wang, C. Wang, L. Ma and Q. Liu, RSC Adv., 2022, 12, 16847–16859 RSC.
- Z. Xie, B. Chen, F. Peng, M. Liu, H. Liu, G. Yang and B. Han, ChemSusChem, 2020, 13, 5683–5689 CrossRef CAS PubMed.
- M. Wada, K. Narita and A. Yokota, Appl. Microbiol. Biotechnol., 2007, 76, 819–825 CrossRef CAS PubMed.
- W. W. Dunn, Y. Aikawa and A. J. Bard, J. Am. Chem. Soc., 1981, 103, 6893–6897 CrossRef CAS.
- T. Sakata, J. Photochem., 1985, 29, 205–215 CrossRef CAS.
- Y. W. Han, L. Ye, T. J. Gong and Y. Fu, Angew. Chem., Int. Ed., 2023, 62, e202306305 CrossRef CAS PubMed.
- M. Li, Y. Wu, B.-H. Zhao, C. Cheng, J. Zhao, C. Liu and B. Zhang, Nat. Catal., 2023, 6, 906–915 CrossRef CAS.
- J. Wu, L. Xu, Z. Kong, K. Gu, Y. Lu, X. Wu, Y. Zou and S. Wang, Angew. Chem., Int. Ed., 2023, 62, e202311196 CrossRef CAS PubMed.
- E. A. Jeffery, O. Johansen and A. Meisters, Aust. J. Chem., 1978, 31, 79–84 CrossRef.
- E. A. Jeffery and A. Meisters, Aust. J. Chem., 1978, 31, 73–78 CrossRef.
- H. Duan and F. Wang, Chem. Catal., 2022, 2, 641–643 CrossRef CAS.
- X. Liu, D. C. Y. Leong and Y. Sun, Green Chem., 2020, 22, 6531–6539 RSC.
- S. Jiang, W. Ramdani, E. Muller, C. Ma, M. Pera-Titus, F. Jerome and K. De Oliveira Vigiera, ChemSusChem, 2020, 13, 1699–1704 CrossRef CAS PubMed.
- S. Song, V. Fung Kin Yuen, L. Di, Q. Sun, K. Zhou and N. Yan, Angew. Chem., Int. Ed., 2020, 59, 19846–19850 CrossRef CAS PubMed.
- K. Kurita, Mar. Biotechnol., 2006, 8, 203–226 CrossRef CAS PubMed.
- N. Yan and X. Chen, Nature, 2015, 524, 155–157 CrossRef CAS PubMed.
- H. Yang, G. Gözaydın, R. R. Nasaruddin, J. R. G. Har, X. Chen, X. Wang and N. Yan, ACS Sustainable Chem. Eng., 2019, 7, 5532–5542 CrossRef CAS.
- S. Cao, Y. Liu, L. Shi, W. Zhu and H. Wang, Green Chem., 2022, 24, 493–509 RSC.
- X. Chen, S. L. Chew, F. M. Kerton and N. Yan, Green Chem., 2014, 16, 2204–2212 RSC.
- X. Gao, X. Chen, J. Zhang, W. Guo, F. Jin and N. Yan, ACS Sustainable Chem. Eng., 2016, 4, 3912–3920 CrossRef CAS.
- X. Chen, Y. Gao, L. Wang, H. Chen and N. Yan, ChemPlusChem, 2015, 80, 1565–1572 CrossRef CAS PubMed.
- A. D. Sadiq, X. Chen, N. Yan and J. Sperry, ChemSusChem, 2018, 11, 532–535 CrossRef CAS PubMed.
- T. T. Pham, X. Chen, T. Söhnel, N. Yan and J. Sperry, Green Chem., 2020, 22, 1978–1984 RSC.
- Y. Ohmi, S. Nishimura and K. Ebitani, ChemSusChem, 2013, 6, 2259–2262 CrossRef CAS PubMed.
- K. Techikawara, H. Kobayashi and A. Fukuoka, ACS Sustainable Chem. Eng., 2018, 6, 12411–12418 CrossRef CAS.
- F. D. Bobbink, J. Zhang, Y. Pierson, X. Chen and N. Yan, Green Chem., 2015, 17, 1024–1031 RSC.
- H. Kobayashi, K. Techikawara and A. Fukuoka, Green Chem., 2017, 19, 3350–3356 RSC.
- G. Liang, A. Wang, L. Li, G. Xu, N. Yan and T. Zhang, Angew. Chem., Int. Ed., 2017, 56, 3050–3054 CrossRef CAS PubMed.
- H. L. Lee, Y. L. Hung, A. Amin, D. E. Pratama and T. Lee, Ind. Eng. Chem. Res., 2023, 62, 1946–1957 CrossRef CAS.
- B. Dong, J. Cui, Y. Qi and F. Zhang, Adv. Mater., 2021, 33, e2004697 CrossRef PubMed.
|
This journal is © The Royal Society of Chemistry 2024 |