DOI:
10.1039/D4FO01637H
(Paper)
Food Funct., 2024,
15, 8104-8115
Digested casein phosphopeptides impact intestinal calcium transport in vitro†
Received
8th April 2024
, Accepted 10th July 2024
First published on 11th July 2024
Abstract
Calcium is the most abundant mineral in the human body and is involved in critical physiological and cellular processes. It is essential for the development, maintenance, and integrity of bone tissue throughout life. Identifying new natural food-grade chelating agents to improve calcium uptake is of increasing interest. Casein phosphopeptides (CPPs), highly phosphorylated peptides obtained after enzymatic hydrolysis of caseins, represent promising calcium-chelating candidates. The aim of this study was to investigate, using cell culture models, the ability of a digested milk matrix enriched in CPPs to regulate calcium transport through the intestinal barrier and elucidate the involved mechanisms. To this end, a CPP-preparation underwent in vitro static digestion and was subsequently incubated with an intestinal barrier model to monitor calcium uptake and transport. Our results demonstrated that the digested CPP preparation enhanced the trans-epithelial calcium transport via paracellular pathways and that CPPs, identified by peptidomics, crossed the intestinal barrier in the same time.
1. Introduction
Calcium is one of the essential minerals for human health, primarily due to its pivotal role in maintaining the integrity of bone tissue. However, calcium has crucial functions as a second messenger in numerous vital cellular processes, including neurotransmission, muscle contraction, blood coagulation, and hormone secretion. Therefore, a lack of calcium leads to various pathologies, mainly osteoporosis, and is also linked to other disorders, including hypertension or diabetes.1 Calcium supplementation is a subject of controversy; nevertheless, it remains commonly used in various populations, to prevent or slow the progression of osteoporosis during pregnancy or to prevent primary hypertension.2–4
Food and calcium supplements are sources of calcium for the human body, with 90% of calcium absorption occurring in the small intestine and up to 10% in the colon. The regulation of its intestinal absorption is crucial and not fully understood, particularly regarding the role of food components, which requires further investigation.5 It is known that the sequestration of minerals by different agents increases their bioavailability, allowing their stabilization and preventing their precipitation.6 There are various chelating agents, but those of food origin have undeniable advantages compared to chemical agents. In addition to their food origin, they also form more stable complexes. Metal mineral salts and multi-mineral supplements can have adverse effects on food properties or lead to digestive disorders. Organic compounds harboring chelating properties, such as bioactive peptides from the hydrolysis of dietary proteins, could be a suitable alternative. Indeed, peptides derived from egg white, soybean, tilapia, or whey proteins have been characterized as calcium-chelating agents.7–9
Dairy proteins have been widely recognized as an excellent source of bioactive peptides involved in various physiological processes.10 Among them, casein phosphopeptides (CPPs) are phosphorylated peptides derived from casein hydrolysis and are known to increase calcium bioavailability.11 We recently reviewed the identification, production and bioactivity of CPPs,12 reporting that in past years, in vitro and in vivo studies performed in intestinal cells, rats and humans have shown that CPPs increase intestinal calcium absorption.13–15 The enhanced calcium bioavailability triggered by CPPs impacts the differentiation of osteoblasts and enzyme activities, preventing bone loss by modulating the calcification of cartilage and bone.16–18 Consequently, preparations enriched in CPPs are currently available on the market and used to address calcium deficiency.12 Some CPPs have shown in vitro and in vivo to resist gastrointestinal digestion,19,20 and have even been found in human plasma after a cheese-enriched diet.21 It has been evidenced that gastrointestinal digestion has little impact on the physicochemical properties of CPPs and may even produce them through casein proteolysis.22
A study has shown that CPPs sequester calcium through specific amino acid sequences, such as three phosphoryl residues followed by two glutamic acid residues, utilizing phosphate groups. Additionally, the N-terminal region seems to be involved as well.23 The parent protein ratio (α-caseins/β-casein) de facto affects the impact of CPP mixtures on calcium bioavailability at the intestinal level.24,25
Calcium crosses the intestinal barrier (IB) through paracellular and transcellular pathways. The paracellular pathway involves passive diffusion driven by a concentration gradient, without a saturation phenomenon. This pathway includes tight junctions, intercellular complexes formed by several transmembrane proteins, including claudins, occludin and cytosolic proteins such as zonula occludens-1 (ZO-1).26 Transcellular transport requires energy and allows calcium absorption when the luminal calcium concentration is lower than in the basal compartment. This process primarily involves the transient receptor potential vanilloid subfamily member 6 (TRPV6) calcium channel and the L-type calcium channel Cav1.3 present at the apical side of the enterocyte, initiating calcium transport through the plasma membrane. Calcium is then supported at the intracellular level by binding to the calbindin-D9k protein and subsequently reaches the bloodstream by passing through the plasma membrane Ca2+ ATPase PMCA1b/ATP2B1. While only a few studies have explored the mechanisms through which CPPs can influence intestinal paracellular calcium uptake and transcellular calcium transport, evidence suggests that the interaction with TRPV6 and voltage-operated L-type calcium channels (LTCCs) may be involved. This evidence has been observed in vitro using Caco-2 and HT-29 intestinal cells.27,28
The current study explores the impact of a milk-protein hydrolysate enriched in CPPs, following simulated gastrointestinal digestion (SGID), on intracellular calcium uptake and overall calcium transport using co-cultured Caco-2 and HT29-MTX intestinal cells as IB model. Additionally, the peptide populations obtained after SGID and intestinal barrier passage experiments were identified by peptidomics.
2. Experimental
2.1. Materials
The casein hydrolysate enriched in CPPs (CPP-preparation) used in this study was supplied by Ingredia S.A. (St-Pol-sur-Ternoise, France) and was produced through an industrial process outlined as follows: micellar caseins, with a ratio of micellar caseins to whey proteins of 92
:
8, underwent enzymatic hydrolysis using food-grade trypsin and were subsequently dried through atomization employing the Mini Spray Dryer B-290 from BUCHI (Rungis, France). For the RT-qPCR assay, the primers were designed in-house and subsequently synthesized by Eurogentec (Seraing, Belgium).
2.2. Simulated gastrointestinal digestion (SGID)
The gastrointestinal digestion of the CPP-preparation was simulated in vitro using the INFOGEST harmonized protocol29 adapted for protein alone.30 Simulated fluid composition and the method are described in a previous study.31 Briefly, 2 grams of CPP-preparation solubilized in 8 mL of water were first mixed with 8 mL simulated salivary fluid for 5 minutes, at pH 7.0. Then, simulated gastric fluid containing pepsin from Sigma-Aldrich (St Louis, MO, USA, P6887, 6500 U mL−1) was added volume to volume in the reactor and incubated for 2 h, at pH 3.0. Finally, simulated intestinal fluid containing pancreatin (Sigma-Aldrich, P1750, trypsin activity 45 U mL−1) was added volume to volume in the reactor and incubated for 2 h, at pH 7.0. At the end of the experiment, the digested CPP-preparation (dCPPp) was collected, heated at 95 °C for 5 min to assure enzyme denaturation, centrifuged at 13
400g for 5 min and frozen at −20 °C until further use. For control, a blank SGID (Blk) was also performed, without any powder but with the simulated digestive fluids and enzymes, to exclude the effect of enzyme autolysis. The SGID of the CPP-preparation and Blk were performed in triplicate (n = 3).
2.3. Cell culture
Experiments were conducted using human intestinal cell lines: Caco-2 human colorectal carcinoma obtained from Sigma-Aldrich (St Louis, MO, USA), and mucus-secreting HT29-MTX human adenocarcinoma (Sigma-Aldrich). Their accession numbers in the Cellosaurus database are 86010202 and 12040401, respectively. The cells were cultured in 75 cm2 flasks (Sarstedt, Nümbrecht, Germany) at 37 °C under 5% CO2, in Dulbecco's modified Eagle's medium (DMEM) (PAN Biotech, Aidenbach, Germany) supplemented with 10% (v/v) heat-inactivated fetal bovine serum (FBS), 100 U mL−1 of penicillin and streptomycin, and 2 mM of L-glutamine, constituting the complete medium.
2.4. Intracellular calcium uptake
Caco-2 cells were plated in 96-well black plates at a density of 25
000 cells per cm2 and cultured for two weeks in complete medium at 37 °C under 5% CO2. Prior to the experiment, cells reaching confluency were starved for 24 h without FBS in the medium. On the day of the experiment, cells were washed with phosphate saline buffer (PBS). Intracellular calcium levels were assessed using the FluoForte® Calcium Assay Kit (Enzo Life Sciences, Lyon, France, ENZ-51017). The calcium-binding fluorogenic probe was incubated with the cells for 1 h at 37 °C to allow its integration into the cells. Subsequently, fluorescence was measured every second for 45 seconds, well by well, using a microplate reader Xenius spectrofluorometer equipped with automatic injectors (Safas, Monaco, Monaco) at 494 nm (excitation) and 516 nm (emission). Ten s after starting fluorescence measurement, 25 μL of dCPPp (5 mg mL−1) or Blk at the same dilution, at a final calcium concentration of 10 mM, were added. Fluorescence emission was expressed as a fold change compared to the basal fluorescence measured between 0 and 9 s. The results are presented as the difference between the fluorescence levels at 10 and 30 s and expressed as a fold change relative to the control condition. Cell viability (>95%) was verified prior the experiment for each condition using the CCK-8 assay (Tebu-Bio, France).
2.5. Intestinal barrier calcium transport
Caco-2/HT29-MTX cells were seeded at a ratio 90/10 and a density of 20
000 cells per cm2 on transwells (polyethylene terephthalate membrane with a cell growth area of 0.9 cm2 and pore size of 0.4 μm, Corning, Glendale, CA, USA). The cells were cultured for three weeks in complete medium at 37 °C and under 5% CO2. Before the experiment, cells were starved 24 h without FBS in the medium. On the day of the experiment, transepithelial electrical resistance (TEER) was measured using a Voltmeter Ohmmeter MilliCell Electrical Resistance System (Millipore, Burlington, NJ, USA) to assess the integrity of the cell monolayer. Cells were washed with PBS, and 500 μL of dCPPp or blank SGID (2 or 6 mg mL−1, at a final calcium concentration of 1.6 and 4.6 mM, respectively) were added to the apical chamber, and 1.5 mL of PBS was added to the basolateral chamber for a 2 h-incubation. At different time points, 100 μL of the basolateral medium were collected and frozen at −20 °C. Calcium concentration determination was performed following the Calcium Colorimetric Assay Kit (Sigma-Aldrich) guidelines with a Xenius spectrofluorometer (Safas). Cell viability (>95%) was verified prior the experiment for each condition using the CCK-8 assay (Tebu-Bio, France).
2.6. Tight-junction permeability measurement
The permeability of tight junctions was assessed using the lucifer yellow assay. Following a 2 h-incubation with dCPPp or blank SGID, 1 mL of lucifer yellow 100 μM and 2 mL of Hank's buffer with HEPES (HHBS) were added to apical and the basolateral chambers, respectively. At 15, 30, 45, 60 and 90 min, 100 μL of the basolateral medium were collected, and the fluorescence was measured using a Xenius spectrophotometer (Xenius, Safas) at 530 nm (excitation) and 585 nm (emission). The apparent permeability coefficient was calculated according to the following equation:
where: S is the insert membrane surface area; C0 is the lucifer yellow initial concentration in the apical chamber; and
is the lucifer yellow rate of appearance in the basolateral chamber.
2.7. Real-time quantitative polymerase chain reaction (RT-qPCR)
Caco-2/HT29-MTX cells were seeded at a ratio of 90/10 and a density of 20
000 cells per cm2 in 24-well plates and grown for two weeks in complete DMEM at 37 °C under 5% CO2. On the day of the experiment, cells were washed with PBS and incubated with dCPPp or blank SGID, at 2 or 6 mg mL−1, for either 2 or 6 h. Cell viability (>95%) was verified prior the experiment for each condition using the CCK-8 assay (Tebu-Bio, France). After incubation, the supernatant was removed, and RNA was extracted with the modified NucleoZOL (Macherey-Nagel, Düren, Germany) protocol. RNA concentration and purity were determined using the Nanodrop lite (Thermo Fisher Scientific, Waltham, MA, USA). The reverse transcription reaction was performed using the RevertAid H Minus First Strand cDNA Synthesis kit (ThermoFisher Scientific, Waltham, MA, USA). RT-qPCR was performed using the Takyon™ No Rox SYBR® MasterMix dTTP Blue (Eurogentec, Seraing, Belgium) kit on a CFX Connect Real-Time PCR detection system (Biorad, Hercules, USA) and specific primers described in Table 1.
Table 1 Primers used for qPCR experiments
Genes (H. sapiens) |
Forward primer |
Reverse primer |
HPRT1 |
GCCCTGGCGTCGTGATTAGT |
GCAAGACGTTCAGTCCTGTCC |
TRPV6 |
TGATGCGGCTCATCAGTGCCAGC |
GTAGAAGTGGCCTAGCTCCTCG |
CACNA1D |
TCCCTCATCGTAATCGGCAG |
TGCATGCCAATGACCGCATA |
ATP2B1 |
TTGTAATGGGCGACATGGCA |
TTTTTCGTAATGCATCTGTGGACC |
CLDN-2 |
TGGCCTCTCTTGGCCTCCAACTTGT |
TTGACCAGGCCTTGGAGAGCTC |
CLDN-4 |
CCACTCGGACAACTTCCCAA |
ACTTCCGTCCCTCCCCAATA |
CLDN-12 |
CTGAGAGGGAGACGCTCCAA |
GTACCTGACAGTTCCAAAACAGC |
Occludin |
CAGGGAATATCCACCTATCACTTCAG |
ATCAGCAGCAGCCATGTACTCTTCAC |
ZO-1 |
CGGTCCTCTGAGCCTGTAAG |
GGATCTACATGCGACGACAA |
2.8. Size exclusion chromatography (SEC)
The peptide molecular mass profiles of the salivary, gastric, and intestinal dCPPp (18.5 g L−1, w/v) were obtained by SEC. The samples were centrifuged at 15
000g for 15 min, and the supernatants were filtered through a 0.22 μm membrane filter before SEC. Peptides were separated using the Superdex peptide 10/300 GL column (Cytiva, Saint-Germain-en-Laye, France) and an AKTA Purifier system (GE Healthcare, Chicago, USA) under isocratic elution (69.9% ultrapure water; 30% acetonitrile (ACN); 0.1% TFA) with a flow rate of 0.5 mL min−1. The absorbance was recorded at 214 nm. The column was prior calibrated with the mass molecular standards: albumin: 60 kDa; cytochrome C: 12
400 Da; aprotinin: 6500 Da; vitamin B12: 1355 Da; glutathione: 307 Da.
2.9. Peptidomics analysis
Caco-2/HT29-MTX cells were seeded at a ratio 90/10 and a density of 20
000 cells per cm2 transwells (Corning) and grown for three weeks in complete medium at 37 °C and under 5% CO2. On the day of the experiment, cells were washed with PBS, and 500 μL of dCPPp or blank SGID (31.25 mg mL−1) were added to the apical chamber and 1.5 mL of non-supplemented DMEM to the basolateral chamber for a 2 h-incubation. CPP-preparation before SGID and apical compartment media were dissolved at 1 mg mL−1 in H2O while basolateral compartment media were concentrated 10 times by centrifugal evaporation at 40 °C (miVac Centrifugal Vacuum Concentrators, Gene Vac, Ipswich, UK). All samples were centrifuged for 10 min at 8000g and 10 μL of supernatants were analyzed by RP-HPLC-MS/MS. CPP-preparation before SGID was analyzed in triplicate, whereas apical and basolateral compartment media were analyzed from two different experiments produced from two dCPPp replicates. For each transport experiment, three wells were analyzed independently.
Peptides were chromatographically separated at 30 °C on an ACQUITY UPLC system (Waters Corporation, France) using a Halo AQ-C18 column (150 × 2.1 mm, 2.7 μm, Advanced Materials Technology, USA). The mobile phases consisted of solvent A (0.1% (v/v) formic acid/99.9% (v/v) water) and solvent B (0.1% (v/v) formic acid/99.9% (v/v) ACN). Two ACN gradients (flow rate 0.5 mL min−1) were used: (1) from 1% to 30% solvent B over 22.5 min, from 30% to 95% solvent B over 2.5 min followed by washing and equilibrating procedures with 95% and 1% solvent B for 2.5 min each, respectively; (2) 1% solvent B during 3 min, from 1% to 30% solvent B over 42 min, from 30% to 95% solvent B over 5 min followed by washing and equilibrating procedures with 95% and 1% solvent B for 5 min each, respectively. The eluate was directed into the electrospray ionization source of the qTOF Synapt G2-Si™ (Waters Corporation) previously calibrated using a sodium formate solution. MS analysis was performed in sensitivity, positive ion and data dependent analysis (DDA) modes using the proprietary MassLynx software (Waters). The source temperature was set at 150 °C and the capillary and cone voltages were set to 3000 and 60 V. MS data were collected for m/z values in the range of 50 and 2000 Da with a scan time of 0.2 s. A maximum of 10 precursor ions were chosen for MS/MS analysis with an intensity threshold of 10
000. MS/MS data of peptide ions were collected using collision induced dissociation (CID) and a scan time of 0.1 s with specified voltages ranging from 8 to 9 V and from 40 to 90 V for the lower molecular mass ions and for those with a higher molecular mass, respectively. The leucine-enkephalin ([M + H+] of 556.632) was injected in the system every 2 min for 0.5 s to follow and to correct the measure error during all the time of analyze.
Database searches were performed in the UniProtKB/Swiss-Prot database restricted to Bos Taurus (accessed May 2023, 6035 entries) via PEAKS Studio X + (Bioinformatics Solutions Inc., Waterloo, Canada). A mass tolerance of 10 ppm and an MS/MS tolerance of 0.1 Da were allowed. The data searches were performed without notifying the choice of enzyme. Variable serine-threonine- and tyrosine-phosphorylations and methionine oxidation were also considered with a AScore ≥ 8 and with a maximum of 3 post-translational modifications allowed by peptide. The relevance of protein and peptide identities was judged according to their score in the research software (False Discovery Rate (FDR) < 0.1%). For CPP-preparation before SGID and each transport experiments, the peptide identification was performed with a single query combining the three replicates.
In order to envision peptide identifications, the data were exported from PEAKS Studio 8.5 to a home-built Microsoft Excel sheet to generate heat maps giving us the amino acid occurrences in β-casein, αS1-casein, αS2-casein and κ-casein. The mass spectrometry proteomics data have been deposited to the ProteomeXchange Consortium via the PRIDE32 partner repository with the dataset identifier PXD052909.
2.10 Statistical analysis
Data were expressed as mean values with their standard deviations. Statistical analyses were carried out using GraphPad Prism (San Diego, CA, USA). A Shapiro–Wilk test was prior performed to check the normal distribution of the values. The experiments including two groups were analyzed with an unpaired t-test or a Mann–Whitney test to determine all significances compared to the control group.
3. Results
3.1. Evolution of the peptide molecular mass profile of the CPP-preparation during SGID
To observe the impact of the SGID on the molecular mass distribution of the casein-derived peptides of the CPP-preparation, the samples collected from the first three compartments of the gastrointestinal tract were subjected to SEC (Fig. 1).
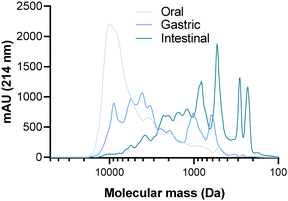 |
| Fig. 1 Peptide molecular mass (MM) profiles of the CPP-preparation in the different SGID steps. The CPP-preparation underwent a SGID and the peptide molecular mass profiles of the oral, gastric and intestinal compartment were obtained by SEC employing a Superdex peptides 10/300 GL column. The MM distribution was determined through a linear regression relationship that correlates the logarithm (log10) of known MM standard molecules and their elution volume. | |
The results initially revealed that the activity of pepsin had a notable impact on the molecular mass distribution of peptides in the CPP-preparation. Indeed, a large amount of polypeptides (>8000 Da) present in the oral compartment has disappeared in favor of smaller peptides (600 to 6000 Da), with a majority of peptides having an apparent molecular mass ranging from 1500 to 10
000 Da. Subsequently, the action of pancreatic enzymes in the intestinal compartment was extensive, leading to the emergence of low molecular mass peptides (<400 Da) and the disappearance of high molecular mass peptides. This resulted in a predominant presence of peptides with a molecular mass lower than 3000 Da.
3.2. Peptidome identification
To investigate the impact of enterocyte peptidases on the dCPPp and to identify peptides recovered after passing through the intestinal barrier, the dCPPp was incubated with the Caco-2/HT29-MTX intestinal barrier model for 2 h. Then, apical and basolateral media were recovered and analyzed by RP-HPLC-MS/MS. Peptides, and particularly CPPs, were identify in the CPP-preparation before SGID, as well as after the challenge with the intestinal barrier model in both apical and basolateral compartments (Fig. 2). As previously described,33 conventional peptidomic methods face challenges in identifying multi-phosphorylated and/or large peptides. To address this, we devised a four-phase strategy to improve the identification of CPP especially multi-phosphorylated and large peptides. However, for the simplicity of this study, we assumed that the peptides obtained after SGID are small in size. Consequently, we analyzed the samples using a conventional approach.
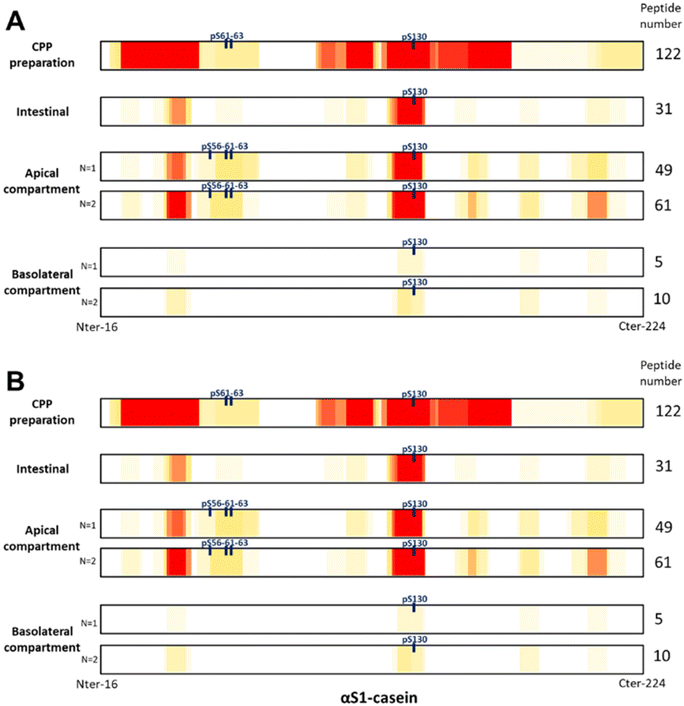 |
| Fig. 2 Heat maps obtained from peptides identified by peptidomics. Heat maps highlighting the occurrence of identified peptides along the amino acid sequences of (A) β-casein and (B) αS1-casein in CPP-preparation before SGID or in apical (before IB passage) or basolateral (after IB passage) compartments after a 2 h-incubation with the Caco-2/HT29-MTX IB model. More red color indicates more frequently detected peptides on the backbone. Identified phosphosites (pS) were represented in blue. Replicates N = 1 and N = 2 correspond to two independent experiments of transport with three replicates of dCPPp. For CPP-preparation, intestinal phase and each transport experiment, the peptide identification was performed with a single database query combining three replicates. | |
Initially, 337 peptides were identified in the CPP-preparation, including 46 CPPs and 8 phosphosites (3 phosphosites for αS1-casein, 4 phosphosites for αS2-casein and 1 phosphosite for β-casein). Phosphosites refer to phosphorylation sites on a protein or peptide, where a phosphate (HPO3) group is covalently attached to an amino acid residue constituting the protein or peptide. Subsequently, after SGID (intestinal phase), 145 peptides were identified, including 29 CPPs and 7 phosphosites (1 phosphosite for αS1-casein, 2 phosphosites for αS2-casein, 2 phosphosites for β-casein and 2 phosphosites for κ-casein). In the two independent replicates (designated as N = 1 and N = 2 in Fig. 2) of apical or basolateral compartments, 206 and 255 peptides were identified (including 36 and 41 CPPs as well as 11 phosphosites) and 23 and 38 peptides (including 2 and 1 CPPs and phosphosites), respectively. Finally, two CPPs were recovered after passing through the intestinal barrier; peptide f125-134 (EIVPNpSAEER (pSer130)) from αS1-casein and peptide f48-60 (FQpSEEQQQTEDEL (pSer50)) from β-casein. The lists of the identified peptides are available in supplemental data (ESI data 2_lists of identified peptides†).
3.3. Effect of digested CPP-preparation on calcium uptake in enterocytes and modulation of related gene expression
Calcium uptake was evaluated in differentiated Caco-2 cells. After the integration of the fluorescent probe by the cells, the dCPPp (5 mg mL−1) was injected into the cell supernatant at a final calcium concentration of 10 mM. The results obtained are quite heterogenous, and no significant difference in calcium uptake was observed between cells incubated with dCPPp and the control condition (Fig. 3A).
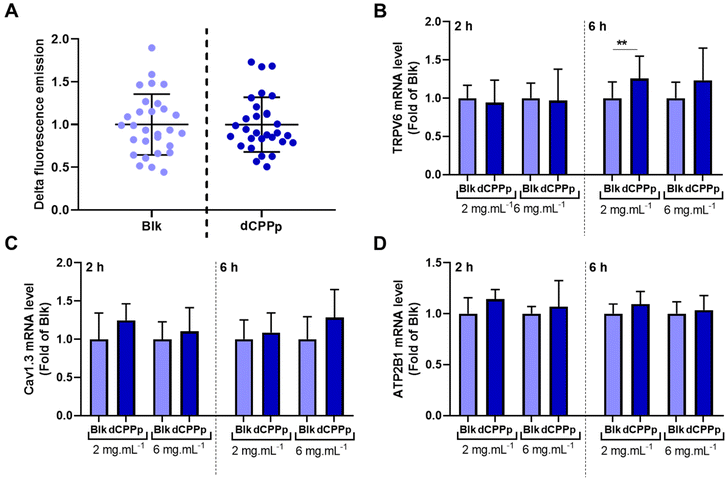 |
| Fig. 3 Effect of digested CPP-preparation on calcium uptake. (A) Caco-2 cells were incubated with the calcium-binding fluorogenic FluoForte® probe. Subsequently, 25 μL of dCPPp or Blk (SGID control condition), at a calcium final concentration of 10 mM, were added. The results are expressed as the difference of fluorescence before and after the injection and as fold as the control. Mean ± SD from three independent experiments. TRPV6 (B), Cav1.3 (C), ATP2B1 (D) mRNA relative levels normalized to HPRT1 in Caco-2/HT29-MTX coculture incubated with dCPPp (2 and 6 mg mL−1 for 2 and 6 h). The MRNA level corresponding to SGID Blk is set at 1. Mean ± SD from 4 independent experiments (N = 4) using the three SGID replicates (n = 3) (** p < 0.01, Mann–Whitney test). | |
The TRPV6 receptor and the voltage-operated L-type calcium channel (Cav1.3) present on the surface of intestinal cells are known to be involved in the intracellular calcium transport. The effect of dCPPp on TRPV6, Cav1.3 and ATP2B1 (ATPase Plasma Membrane Ca2+ Transporting 1) gene expression was therefore investigated. A Caco-2/HT29-MTX coculture was exposed to dCPPp at two different concentrations (2 and 6 mg mL−1) for 2 and 6 h. The expression of the TRPV6 gene was significantly higher compared to the control condition after a 6 h-incubation with the dCPPp at 2 mg mL−1 (Fig. 3B). However, no significant differences in the gene expression of Cav1.3 (Fig. 3C) and ATP2B1 (Fig. 3D) were observed compared to the control condition.
3.4. Effect of the digested CPP-preparation on total calcium transport and tight junction protein gene expression regulation
To investigate the effect of the dCPPp on total calcium transport across the IB model, the differentiated Caco-2/HT29-MTX cell monolayer was apically incubated with the dCPPp (2 and 6 mg mL−1). After 30 min, the basolateral medium was collected to determine calcium concentration. The dCPPp (2 mg mL−1) significantly increased total calcium transport across the IB (Fig. 4A). This effect is also observed at 6 mg mL−1 (p value = 0.0557). After 2 h-incubation, a similar trend was observed at 2 mg mL−1 (p value = 0.059), but not at 6 mg mL−1 (Fig. 4B).
 |
| Fig. 4 Effect of digested CPP-preparation on total calcium transport. Calcium concentration in the basolateral compartment was measured after 30 min (A) and 120 min (B) of incubation with dCPPp (2 and 6 mg mL−1) compared to SGID control condition (Blk). Data are expressed in mM. Mean ± SD from 4 independent experiments (N = 4) using the three SGID replicates (n = 3) (** p < 0.01, t-test). | |
Modifications in the paracellular transport, especially at the level of the tight junctions, can impact IB permeability. To assess the effect of the dCPPp on membrane integrity, we conducted experiments using Lucifer Yellow. Notably, no discernible effect was observed, as shown in ESI Fig. 1.† Total transport encompasses both intracellular and paracellular mechanisms. Paracellular transport is governed by tight junctions formed by various proteins, some of which are involved in calcium transport. To investigate this, a Caco-2/HT29-MTX coculture was incubated with dCPPp to assess the impact on the gene expression of claudins 2, 12 and 15, occludin and ZO-1. Among all the tested conditions, an increase in occludin and ZO-1 mRNA levels was observed after 6 h-incubation at 2 and 6 mg mL−1, respectively (Fig. 5).
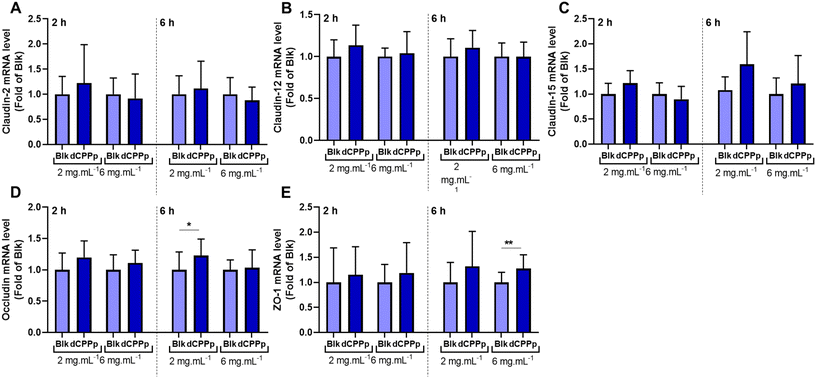 |
| Fig. 5 Effect of digested CPP-preparation on calcium paracellular transport gene expression. Claudin-2 (A), Claudin-12 (B), Claudin-15 (C), Occludin (D) and ZO-1 (E) mRNA relative levels normalized to HPRT1 in Caco-2/HT29-MTX coculture incubated with dCPPp (2 and 6 mg mL−1 for 2 and 6 h). Blk (SGID control condition) is set at 1. Mean ± SD from 4 independent experiments (N = 4) using the three SGID replicates (n = 3) (* p < 0.05, ** p < 0.01, Mann–Whitney test). | |
4. Discussion
The diverse phosphopeptides originating from milk are primarily from caseins, which are major phosphoproteins in milk. Caseins exist in several forms, including αS1-, αS2-, β-, and κ-casein. In the present study we posited that a casein hydrolysate enriched with CPPs could enhanced intestinal calcium uptake and transport due to the presence of calcium-binding peptides within CPPs. Firstly, the CPP-preparation, harboring a high calcium concentration, was digested using the harmonized INFOGEST protocol, adapted to proteins29,30 to characterize the effects of the SGID on peptide population in the different compartments in terms of molecular mass distribution (Fig. 1). A large diversity of digestive enzyme-resistant peptides was recovered in the intestinal compartment, as already described in a previous study of casein SGID.30,34 Consistent with existing literature, numerous casein-derived peptides, including CPPs, were identified following gastrointestinal digestion of dairy products. This has been demonstrated in vitro after SGID,35–37in vivo in minipigs38 or in vivo in human.20,39 In the current study, we identified 145 casein peptides, including 29 CPPs, after SGID of the CPP-preparation (Fig. 2).
The digested CPP-preparation was subsequently subjected to challenge with an IB barrier model, and the peptidomes identified and compared. Conventional peptidomic methods encounter difficulties in identifying multi-phosphorylated and/or large peptides. To address this challenge, we implemented a four-phase strategy to enhance the identification of CPPs, especially multi-phosphorylated and large peptides. However, for the simplicity of this study, we assumed that the peptides obtained after SGID are small in size. Consequently, we analyzed the samples using a conventional approach. Our findings revealed that after 2 h-incubation of the digested CPP-preparation with the Caco-2/HT29-MTX IB model, diverse peptides and CPPs originating from β-, αs1-, αs2-, and κ-caseins were identified (Fig. 2). A greater number of peptides, CPPs, and phosphorylation sites were identified compared to the intestinal phase without IB model incubation. Specifically, 11 phosphosites were identified in the apical compartment, while 7 phosphosites were identified in the intestinal compartment. Peptidases and phosphatases located in the brush border of the apical membrane can cleave and partially or totally dephosphorylate peptides, thereby facilitating their identification in a similar manner.
Chabance et al., detected casein-derived peptides in plasma of adult humans after milk or yogurt ingestion.39 Some calcium-binding peptides (both mono and multiphosphorylated CPPs) derived from αs1- and αs2-casein, such as αs1-casein (f43–52 and f43–50) and αs2-casein (f8–12, f7–12, and f6–12), alongside four non-phosphorylated peptides from the C-terminal region of β-casein (f193–209, f194–209, f200–209) (position along the lateral chain without peptide signal), were identified in plasma samples of human subject after one week of cheese consumption (100 g day−1).21 After 2 h-incubation of dCPPp with the Caco-2/HT29-MTX IB model, we identified 42 unique peptides including two CPPs: peptide f125–134 (EIVPNpSAEER (pSer130)) from αS1-casein and peptide f48–60 (FQpSEEQQQTEDEL (pSer50)) from β-casein. These two peptides have already been identified in milk or cheese digestates.40 Peptide f125–136 from αS1-casein was listed in the BIOPEP-UWM database as an anti-hypertensive peptide. Additionally, five CPPs derived from αs1-casein and β-casein, purified from a commercial CPP mixture, were found to enhance calcium transport through a Caco-2 cell monolayer.41 These five peptides include the peptide f119–134 from αS1-casein containing the peptide f125–134 and the peptide f48–63 from β-casein containing the peptide f48–60.
As previously mentioned, the physicochemical properties of CPPs directly impact their bioactivity. In the present study, we observed that the dCPPp did not affect calcium uptake (Fig. 3). Interestingly, our findings contradict previous studies that have shown that CPPs could enhance intracellular calcium uptake in both Caco-2 and HT29 cells.42–44 However, to the best of our knowledge, our study is the first to utilize a coculture of Caco-2 cells and HT29-MTX mucus-producing cells differentiated on transwells as IB model to investigate intracellular calcium intake. The presence of mucus in our model may account for these discrepant results. Nevertheless, we did observe an upregulation of the calcium channel protein TRPV6 gene expression (Fig. 3), suggesting that the intracellular transport of calcium was modulated. The involvement of the TRPV6 calcium channel in CPP-mediated calcium uptake was observed in Caco-2 cells, but not in HT-29 cells. Additionally, when TRPV6 protein expression was reduced in Caco-2 cells using siRNA, the CPP-mediated calcium uptake was diminished.45 Our results are in accordance with previous findings that CPPs mixed with CaCl2 upregulate the gene expression of TRPV6 and TRPV5 in Caco-2 cells.44
In the present study, we observed an increase in total calcium transport after a 30 min-incubation period with the dCPPp at a concentration of 2 mg mL−1 (Fig. 4). However, at 6 mg mL−1, this effect was not significant (p value = 0.057). The concentration of CPPs appears to be an important factor. Besides CPP concentration, the calcium concentration and the calcium/CPP ratio are also crucial.25,46 There is likely a saturable effect on calcium transport. Interestingly, this phenomenon does not appear to be time-dependent, as prolonged exposure to the dCPPp (120 min-incubation period) did not result in an increased transport of calcium across the IB. Although a trend was observed for the 2 mg mL−1 dose (p value = 0.059), it did not reach statistical significance. This phenomenon was already observed in vivo. In rats, the consumption of a high-casein meal led to enhanced calcium intake. However, the addition of CPPs (100 g kg−1) did not impact absorption. Moreover, at higher doses (200 to 500 g kg−1), calcium intake even decreased.47 Another study conducted on rats, utilizing lower CPP concentrations ranging from 0.7 to 3.5 g kg−1, demonstrated an increase in calcium absorption.48
In addition, several studies have demonstrated the effect of CPPs on calcium transport across the IB. However, this effect is somewhat controversial in the literature, as the increase in calcium transport varies depending on the models and protocols used. Several factors and parameters may explain these fluctuating effects. The process of digestion plays a crucial role in studying the bioactivity of CPPs. Furthermore, the composition and origin of meals can influence the digestion process, thereby impacting the physicochemical properties of CPPs released in the intestinal tract. For instance, in healthy adult humans, the consumption of bread enriched with CPPs did not result in increased calcium and zinc absorption.49 Conversely, in healthy children, single test meals consisting rice-based cereal supplemented with either 1 or 2 grams of CPPs significantly enhanced total calcium absorption. However, this effect was not observed when CPPs were added to whole-grain cereal.50
Moreover, some studies show that CPPs do not appear to impact membrane integrity and permeability, suggesting that they do not influence paracellular transport.24,51 Our results align with these findings, as we did not observe any modification of the IB integrity and permeability. However, it is known that calcium transport modification may involve proteins involved in tight junctions.52 A study examined the expression of genes encoding tight junction proteins involved in regulating calcium transport across the intestinal epithelium. It was observed that the genes encoding claudins 2, 12, and 15 were upregulated.53 In this way, it has been shown that specific claudins can create paracellular pores selective to cations, thereby facilitating calcium transport.54,55 Additionally, other proteins such as occludin, a transmembrane protein, and ZO-1, a cytosolic protein, play roles in tight junctions and may also contribute to ion transport.53 The results of our study provide, to our knowledge, the first evidence that a digested CPP-enriched mixture enhances the gene expression of occludin and ZO-1 proteins (Fig. 5), concurrent with an increase in total calcium transport. In addition to the fact that the dCPPp did not enhance the cellular uptake of calcium, this finding further supports the hypothesis of a mediated-paracellular pathway.
To conclude, this study has shown that a CPP-preparation enhances calcium transport across the intestinal epithelial membrane following simulated gastrointestinal digestion. Furthermore, we identified several CPPs capable of crossing the intestinal barrier. These results suggest that calcium is transported alongside CPPs via the paracellular pathway through interactions with transmembrane proteins or facilitated diffusion. These findings support the potential use of CPP-enriched mixtures as functional foods to improve calcium absorption.
Abbreviations
CPPs | Casein phosphopeptides |
dCPPp |
In vitro Digested CPP-preparation |
ZO-1 | Zonula occludens-1 |
TRPV6 | Transient receptor potential vanilloid subfamily member 6 |
LTCCs | L-type calcium channels |
SGID |
In vitro simulated gastrointestinal digestion |
GLP-1 | Glucagon-like peptide 1 |
IB | Intestinal Barrier |
Blk | Blank in vitro simulated gastrointestinal digestion |
TEER | Transepithelial electrical resistance |
Author contributions
MT, BD and CD contributed to investigation, formal analysis and writing – original draft. JA and AuB, contributed to project administration and supervision. AlB and RR contributed to project administration, supervision, funding acquisition and writing – review. CF contributing to supervision, formal analysis and writing – review. BC contributed to conceptualization, supervision, methodology, formal analysis, writing – review and editing. All authors have reviewed the manuscript and have given their approval for its submission.
Data availability
The main data supporting this article have been included as part of the ESI.†
Conflicts of interest
The authors declare no competing financial interest.
Acknowledgements
This research was carried out within the framework of the joint laboratory project (Allinpep) between UMRT BioEcoAgro and Ingredia, funded by the Hauts-de-France region (FEDER), as well as within the BiHauts Eco de France CPER/FEDER 2021–2027 program, which is financed by the European Union, the French State, and the Hauts-de-France Region. The authors express their gratitude to the Advanced High Throughput Technologies Platform for Biorefineries Catalysts Design, known as “REALCAT”, for their support. The REALCAT platform is funded by a French Governmental Subsidy Administered by the French National Research Agency (ANR) as part of the “Future Investments” program (ANR-11-EQPX-0037).
References
- N. Matikainen, T. Pekkarinen, E. M. Ryhänen and C. Schalin-Jäntti, Physiology of Calcium Homeostasis: An Overview, Endocrinol. Metab. Clin. North Am., 2021, 50(4), 575–590 CrossRef PubMed.
- C. Liu, X. Kuang, K. Li, X. Guo, Q. Deng and D. Li, Effects of combined calcium and vitamin D supplementation on osteoporosis in postmenopausal women: a systematic review and meta-analysis of randomized controlled trials, Food Funct., 2020, 11(12), 10817–10827 RSC.
- K. Tihtonen, P. Korhonen, J. Isojärvi, R. Ojala, U. Ashorn and P. Ashorn,
et al., Calcium supplementation during pregnancy and maternal and offspring bone health: a systematic review and meta-analysis, Ann. N. Y. Acad. Sci., 2022, 1509(1), 23–36 CrossRef PubMed.
- G. Cormick, A. P. Betran, I. B. Romero, M. S. Cormick, J. M. Belizán and A. Bardach,
et al., Effect of Calcium Fortified Foods on Health Outcomes: A Systematic Review and Meta-Analysis, Nutrients, 2021, 13(2), 316 CrossRef CAS PubMed.
-
K. Wongdee, K. Chanpaisaeng, J. Teerapornpuntakit and N. Charoenphandhu, Intestinal Calcium Absorption, in Comprehensive Physiology, John Wiley & Sons, Ltd, 2021 [cited 2024 Jan 31], pp. 2047–2073. Available from: https://onlinelibrary.wiley.com/doi/abs/10.1002/cphy.c200014 Search PubMed.
- M. E. Caetano-Silva, F. M. Netto, M. T. Bertoldo-Pacheco, A. Alegría and A. Cilla, Peptide-metal complexes: obtention and role in increasing bioavailability and decreasing the pro-oxidant effect of minerals, Crit. Rev. Food Sci. Nutr., 2021, 61(9), 1470–1489 CrossRef PubMed.
- N. Charoenphun, B. Cheirsilp, N. Sirinupong and W. Youravong, Calcium-binding peptides derived from tilapia (Oreochromis niloticus) protein hydrolysate, Eur. Food Res. Technol., 2013, 236(1), 57–63 CrossRef.
- W. Huang, Y. Lan, W. Liao, L. Lin, G. Liu and H. Xu,
et al., Preparation, characterization and biological activities of egg white peptides-calcium chelate, LWT–Food Sci. Technol., 2021, 149, 112035 CrossRef.
- Y. Lv, X. Bao, H. Liu, J. Ren and S. Guo, Purification and characterization of caclium-binding soybean protein hydrolysates by Ca2+/Fe3+immobilized metal affinity chromatography (IMAC), Food Chem., 2013, 141(3), 1645–1650 CrossRef CAS PubMed.
- N. Auestad and D. K. Layman, Dairy bioactive proteins and peptides: a narrative review, Nutr. Rev., 2021, 79(Suppl 2), 36–47 CrossRef.
- S. Sun, F. Liu, G. Liu, J. Miao, H. Xiao and J. Xiao,
et al., Effects of casein phosphopeptides on calcium absorption and metabolism bioactivity in vitro and in vivo, Food Funct., 2018, 9(10), 5220–5229 RSC.
- M. Tenenbaum, B. Deracinois, C. Dugardin, A. Matéos, A. Romelard and J. Auger,
et al., Identification, production and bioactivity of casein phosphopeptides – A review, Food Res. Int., 2022, 157, 111360 CrossRef CAS PubMed.
- S. Perego, E. Del Favero, P. De Luca, F. Dal Piaz, A. Fiorilli and L. Cantu’,
et al., Calcium bioaccessibility and uptake by human intestinal like cells following in vitro digestion of casein phosphopeptide-calcium aggregates, Food Funct., 2015, 6(6), 1796–1807 RSC.
- H. Tsuchita, T. Suzuki and T. Kuwata, The effect of casein phosphopeptides on calcium absorption from calcium-fortified milk in growing rats, Br. J. Nutr., 2001, 85(1), 5–10 CrossRef PubMed.
- R. P. Heaney, Y. Saito and H. Orimo, Effect of caseinphosphopeptide on absorbability of co-ingested calcium in normal postmenopausal women, J. Bone Miner. Metab., 1994, 12(1), 77–81 CrossRef.
- T. Matsui, H. Yano, T. Awano, T. Harumoto and Y. Saito, The influences of casein phosphopeptides on metabolism of ectopic bone induced by decalcified bone matrix implantation
in rats, J. Nutr. Sci. Vitaminol., 1994, 40(2), 137–145 CrossRef PubMed.
- G. Liu, J. Miao, S. Sun, Z. Luo, Z. Xia and B. Guo,
et al., The effect of dietary magnesium and caseinphosphopeptides on bone metabolism in rats, Food Funct., 2017, 8(12), 4487–4495 RSC.
- B. M. Donida, E. Mrak, C. Gravaghi, I. Villa, S. Cosentino and E. Zacchi,
et al., Casein phosphopeptides promote calcium uptake and modulate the differentiation pathway in human primary osteoblast-like cells, Peptides, 2009, 30(12), 2233–2241 CrossRef CAS PubMed.
- M. J. García-Nebot, A. Alegría, R. Barberá, G. Clemente and F. Romero, Addition of milk or caseinophosphopeptides to fruit beverages to improve iron bioavailability?, Food Chem., 2010, 119(1), 141–148 CrossRef.
- H. Meisel, H. Meisel, S. Fairweather-Tait, R. J. FitzGerald, R. Hartmann and C. N. Lane,
et al., Detection of caseinophosphopeptides in the distal ileostomy fluid of human subjects, Br. J. Nutr., 2003, 89(3), 351–358 CrossRef CAS PubMed.
- S. Caira, G. Pinto, P. Vitaglione, F. Dal Piaz, P. Ferranti and F. Addeo, Identification of casein peptides in plasma of subjects after a cheese-enriched diet, Food Res. Int., 2016, 84, 108–112 CrossRef CAS.
- M. J. García-Nebot, A. Alegría, R. Barberá, G. Clemente and F. Romero, Addition of milk or caseinophosphopeptides to fruit beverages to improve iron bioavailability?, Food Chem., 2010, 119(1), 141–148 CrossRef.
- A. Ferraretto, C. Gravaghi, A. Fiorilli and G. Tettamanti, Casein-derived bioactive phosphopeptides: role of phosphorylation and primary structure in promoting calcium uptake by HT-29 tumor cells, FEBS Lett., 2003, 551(1–3), 92–98 CrossRef CAS PubMed.
- Y. Cao, J. Miao, G. Liu, Z. Luo, Z. Xia and F. Liu,
et al., Bioactive Peptides Isolated from Casein Phosphopeptides Enhance Calcium and Magnesium Uptake in Caco-2 Cell Monolayers, J. Agric. Food Chem., 2017, 65(11), 2307–2314 CrossRef PubMed.
- C. Gravaghi, E. Del Favero, L. Cantu’, E. Donetti, M. Bedoni and A. Fiorilli,
et al., Casein phosphopeptide promotion of calcium uptake in HT-29 cells - relationship between biological activity and supramolecular structure, FEBS J., 2007, 274(19), 4999–5011 CrossRef PubMed.
- G. D. D. Barboza, S. Guizzardi and N. T. D. Talamoni, Molecular aspects of intestinal calcium absorption, World J. Gastroenterol., 2015, 21(23), 7142–7154 CrossRef PubMed.
- S. Perego, S. Cosentino, A. Fiorilli, G. Tettamanti and A. Ferraretto, Casein phosphopeptides modulate proliferation and apoptosis in HT-29 cell line through their interaction with voltage-operated L-type calcium channels, J. Nutr. Biochem., 2012, 23(7), 808–816 CrossRef CAS.
- S. Perego, A. Zabeo, E. Marasco, P. Giussani, A. Fiorilli and G. Tettamanti,
et al., Casein phosphopeptides modulate calcium uptake and apoptosis in Caco2 cells through their interaction with the TRPV6 calcium channel, J. Funct. Foods, 2013, 5(2), 847–857 CrossRef CAS.
- A. Brodkorb, L. Egger, M. Alminger, P. Alvito, R. Assunção and S. Ballance,
et al., INFOGEST static in vitro simulation of gastrointestinal food digestion, Nat. Protoc., 2019, 14(4), 991–1014 CrossRef CAS PubMed.
- N. Atallah, B. Deracinois, A. Boulier, A. Baniel, D. Jouan-Rimbaud Bouveresse and R. Ravallec,
et al., In Vitro Assessment of the Impact of Industrial Processes on the Gastrointestinal Digestion of Milk Protein Matrices Using the INFOGEST Protocol, Foods, 2020, 9(11), 1580 CrossRef CAS.
- C. Dugardin, L. Fleury, V. Touche, F. Ahdach, J. Lesage and M. Tenenbaum,
et al., An Exploratory Study of the Role of Dietary Proteins in the Regulation of Intestinal Glucose Absorption, Front. Nutr., 2021, 8, 769773 CrossRef.
- Y. Perez-Riverol, J. Bai, C. Bandla, D. García-Seisdedos, S. Hewapathirana and S. Kamatchinathan,
et al., The PRIDE database resources in 2022: a hub for mass spectrometry-based proteomics evidences, Nucleic Acids Res., 2022, 50(D1), D543–D552 CrossRef CAS.
- B. Deracinois, A. Matéos, A. Romelard, A. Boulier, J. Auger and A. Baniel,
et al., Partial-, Double-Enzymatic Dephosphorylation and EndoGluC Hydrolysis as an Original Approach to Enhancing Identification of Casein Phosphopeptides (CPPs) by Mass Spectrometry, Foods, 2021, 10(9), 2134 CrossRef CAS.
- L. Egger, O. Ménard, C. Delgado-Andrade, P. Alvito, R. Assunção and S. Balance,
et al., The harmonized INFOGEST in vitro digestion method: From knowledge to action, Food Res. Int., 2016, 88, 217–225 CrossRef CAS.
- E. Cruz-Huerta, M. J. García-Nebot, B. Miralles, I. Recio and L. Amigo, Caseinophosphopeptides released after tryptic hydrolysis versus simulated gastrointestinal digestion of a casein-derived by-product, Food Chem., 2015, 168, 648–655 CrossRef CAS.
- Y. Wang, R. Wang, H. Bai, S. Wang, T. Liu and X. Zhang,
et al., Casein phosphopeptide calcium chelation: preparation optimization, in vitro gastrointestinal simulated digestion, and peptide fragment exploration, J. Sci. Food Agric., 2024, 104(2), 788–796 CrossRef CAS PubMed.
- R. Portmann, P. Jiménez-Barrios, J. Jardin, L. Abbühl, D. Barile and M. Danielsen,
et al., A multi-centre peptidomics investigation of food digesta: current state of the art in mass spectrometry analysis and data visualisation, Food Res. Int., 2023, 169, 112887 CrossRef CAS PubMed.
- H. Meisel, H. Frister and E. Schlimme, Biologically active peptides in milk proteins, Z Ernährungswiss., 1989, 28(4), 267–278 CrossRef PubMed.
- B. Chabance, P. Marteau, J. C. Rambaud, D. Migliore-Samour, M. Boynard and P. Perrotin,
et al., Casein peptide release and passage to the blood in humans during digestion of milk or yogurt, Biochimie, 1998, 80(2), 155–165 CrossRef PubMed.
- F. Masotti, S. Cattaneo, M. Stuknytė and I. De Noni, Assessment of casein phosphopeptide profile in in vitro digestates of Trentingrana PDO cheese, Eur. Food Res. Technol., 2018, 244(3), 513–521 CrossRef.
- G. Liu, B. Guo, S. Sun, M. Luo, F. Liu and J. Miao,
et al., Promoting the Calcium-Uptake Bioactivity of Casein Phosphopeptides in vitro and in vivo, Front. Nutr., 2021, 8, 743791 CrossRef PubMed.
- S. Cosentino, C. Gravaghi, E. Donetti, B. M. Donida, G. Lombardi and M. Bedoni,
et al., Caseinphosphopeptide-induced calcium uptake in human intestinal cell lines HT-29 and Caco2 is correlated to cellular differentiation, J. Nutr. Biochem., 2010, 21(3), 247–254 CrossRef CAS.
- S. Perego, E. D. Favero, P. D. Luca, F. D. Piaz, A. Fiorilli and L. Cantu’,
et al., Calcium bioaccessibility and uptake by human intestinal like cells following in vitro digestion of casein phosphopeptide–calcium aggregates, Food Funct., 2015, 6(6), 1796–1807 RSC.
- H. Li, S. Duan, P. Yuan, J. Liu, X. Wang and Y. Liu,
et al., Preparation of casein phosphopeptides calcium complex and the promotion in calcium cellular uptake through transcellular transport pathway, J. Food Biochem., 2021, 45(12), e14001 CAS.
- S. Perego, S. Cosentino, A. Fiorilli, G. Tettamanti and A. Ferraretto, Casein phosphopeptides modulate proliferation and apoptosis in HT-29 cell line through their interaction with voltage-operated L-type calcium channels, J. Nutr. Biochem., 2012, 23(7), 808–816 CrossRef CAS.
- D. Erba, S. Ciappellano and G. Testolin, Effect of the ratio of casein phosphopeptides to calcium (w/w) on passive calcium transport in the distal small intestine of rats, Nutrition, 2002, 18(9), 743–746 CrossRef CAS PubMed.
- T. Bennett, A. Desmond, M. Harrington, D. McDonagh, R. FitzGerald and A. Flynn,
et al., The effect of high intakes of casein and casein phosphopeptide on calcium absorption in the rat, Br. J. Nutr., 2000, 83(6), 673–680 CrossRef CAS PubMed.
- Y. Saito, Y. S. Lee and S. Kimura, Minimum effective dose of casein phosphopeptides (CPP) for enhancement of calcium absorption in growing rats, Int. J. Vitam. Nutr. Res., 1998, 68(5), 335–340 CAS.
- M. Hansen, B. Sandström, M. Jensen and S. S. Sørensen, Effect of Casein Phosphopeptides on Zinc and Calcium Absorption from Bread Meals, J. Trace Elem. Med. Biol., 1997, 11(3), 143–149 CrossRef CAS.
- M. Hansen, B. Sandström, M. Jensen and S. S. Sørensen, Casein Phosphopeptides Improve Zinc and Calcium Absorption from Rice-Based but not from Whole-Grain Infant Cereal, J. Pediatr. Gastroenterol. Nutr., 1997, 24(1), 56 Search PubMed.
- A. Colombini, S. Perego, I. Ardoino, E. Marasco, G. Lombardi and A. Fiorilli,
et al., Evaluation of a possible direct effect by casein phosphopeptides on paracellular and vitamin D controlled transcellular calcium transport mechanisms in intestinal human HT-29 and Caco2 cell lines, Food Funct., 2013, 4(8), 1195–1203 RSC.
- C. Raveschot, B. Cudennec, F. Coutte, C. Flahaut, M. Fremont and D. Drider,
et al., Production of Bioactive Peptides by Lactobacillus Species: From Gene to Application, Front. Microbiol., 2018, 9, 2354 CrossRef PubMed.
- G. Diaz de Barboza, S. Guizzardi and N. Tolosa de Talamoni, Molecular aspects of intestinal calcium absorption, World J. Gastroenterol., 2015, 21(23), 7142–7154 CrossRef.
- H. Fujita, K. Sugimoto, S. Inatomi, T. Maeda, M. Osanai and Y. Uchiyama,
et al., Tight Junction Proteins Claudin-2 and -12 Are Critical for Vitamin D-dependent Ca2+ Absorption between Enterocytes, Mol. Biol. Cell, 2008, 19(5), 1912–1921 CrossRef PubMed.
- O. R. Colegio, C. M. Van Itallie, H. J. McCrea, C. Rahner and J. M. Anderson, Claudins create charge-selective channels in the paracellular pathway between epithelial cells, Am. J. Physiol.: Cell Physiol., 2002, 283(1), C142–C147 CrossRef PubMed.
|
This journal is © The Royal Society of Chemistry 2024 |