DOI:
10.1039/D3FO04760A
(Paper)
Food Funct., 2024,
15, 3420-3432
In vitro study of the blood–brain barrier transport of bioactives from Mediterranean foods†
Received
31st October 2023
, Accepted 11th March 2024
First published on 18th March 2024
Abstract
The Mediterranean diet (MD), characterized by olive oil, olives, fruits, vegetables, and wine intake, is associated with a reduced risk of dementia. These foods are rich in bioactives with neuroprotective and antioxidant properties, including hydroxytyrosol (HT), tyrosol (TYRS), serotonin (SER) and protocatechuic acid (PCA), a phenolic acid metabolite of anthocyanins. It remains to be established if these molecules cross the blood–brain barrier (BBB), a complex interface that strictly controls the entrance of molecules into the brain. We aimed to assess the ability of tyrosine (TYR), HT, TYRS, PCA and SER to pass through the BBB without disrupting its properties. Using Human Brain Microvascular Endothelial Cells as an in vitro model of the BBB, we assessed its integrity by transendothelial electrical resistance, paracellular permeability and immunocytochemical assays of the adherens junction protein β-catenin. The transport across the BBB was evaluated by ultra-high-performance liquid chromatography high resolution mass spectrometry. Results show that tested bioactives did not impair BBB integrity regardless of the concentration evaluated. Additionally, all of them cross the BBB, with the following percentages: HT (∼70%), TYR (∼50%), TYRS (∼30%), SER (∼30%) and PCA (∼9%). These results provide a basis for the MD neuroprotective role.
Introduction
Neurological disorders are the third most common cause of disability and premature death in the European Union (EU) and their prevalence and burden will likely increase with the progressive ageing of the European population.1 Current predictors indicate a continuous increase in dementia cases that will reach approximately 152.8 million cases in 2050.2 The two most common age-related neurodegenerative disorders are Alzheimer's disease (AD) and Parkinson's disease (PD), which affect a significant portion of the aged population. AD has been recognized as the most prevalent form of dementia, accounting for around 60–70% of total cases.3 Current estimations suggest that there has been an 81% increase in the incidence of PD since 2000.4
Diet-based interventions have been identified as a promising approach to prevent and delay the progression of neurodegenerative diseases with a substantial body of evidence and experimental support.5,6 In this regard, the Mediterranean diet (MD) has been proposed as a healthy dietary pattern with increasing evidence supporting its beneficial effects towards age-related pathologies, including neurodegenerative disorders and cognitive dysfunctions like AD and PD.7–9 This potential of MD has been mainly related to its high content in plant foods such as fruits, vegetables, and olive oil, which are sources of an array of bioactive compounds.10–12
Hydroxytyrosol (HT) and tyrosol (TYRS) are the major phenolic compounds present in virgin olive oil (VOO) (0.39–41 mg kg−1 and 0.2–32.9 mg kg−1, respectively), and table olives (14.5–3833 mg kg−1 and 0.435–353.5 mg kg−1, respectively).13 Another important source of HT and TYRS is wine (0.000071–9.6 mg L−1 and 1.1–48.3 mg L−1, respectively), with their occurrence being related to the metabolism of tyrosine (TYR) by yeast during the alcoholic fermentation.13,14 Additionally, protocatechuic acid (PCA) is the major phenolic acid formed from anthocyanins in humans.15,16 It is also present in foods at different concentrations: berries (0.62–794.13 mg per 100 g), red wine (0.21 mg per 100 mL), fruits (0.28–18.73 mg per 100 g), jams and berry jams (0.07–9.36 mg per 100 g), fruit juices (1.14–6.70 mg per 100 mL), and vegetables (0.62–10.62 mg per 100 g).17–19 On the other hand, melatonin (MEL) is an hormone synthesized from the essential amino acid tryptophan through the serotonin (SER) pathway. This hormone is involved in physiological processes such as regulation of the circadian rhythm and of the immune system and its antioxidant and neuroprotective activities have been reported.20–22 At the same time, MEL has been detected in foodstuffs including tomatoes, strawberries,23 cherries,24 apples,25 walnuts26 and pistachios,27 as well as in fermented foods, such as wine.28
In PD and AD neuronal impair is partly caused by misfolded proteins associated with oxidative stress and mitochondrial dysfunction. It has been reported that TYRS, HT and PCA inhibit the formation of α-synuclein fibrils in vitro, pointing to neuroprotective properties.29–31 During the process of aging, a decline in several precursors of MEL, including tryptophan and SER, occurs and this reduction may be linked to the occurrence of AD.32,33 In fact, SER has shown to inhibit the formation of β-amyloid (Aβ) fibrils in vitro.34 Additionally, HT and TYRS have demonstrated to be potent antioxidants at brain level. Indeed, HT ameliorates neuronal impairment by modulating mitochondrial oxidative stress in 7PA2 cells (5 μM),35 promotes a decrease of reactive oxygen species (ROS) and inhibits the monoamine oxidase (MAO) activity preserving the dopamine levels in vitro and in vivo.36–38 Besides, TYRS treatment proved to reduce ROS levels and to promote the expression of specific chaperones and antioxidant enzymes in a Caenorhabditis elegans model of PD.39In vivo studies have also demonstrated that PCA exhibits neuroprotective effects through an improvement of the endogenous antioxidant enzymatic system40,41 including certain antioxidant effects related to ROS and upregulating heme oxygenase (HO)-1 expression.42 Furthermore, in vitro studies demonstrated that SER led to a modulation of the expression of some vitagenes (genes involved in preserving cellular homeostasis under stressful conditions) against Aβ-induced toxicity.34
Despite the cumulative evidence of the neuroprotective effects of TYRS, HT, PCA and SER observed through in vitro tests, the ability to pass through the blood–brain barrier (BBB) to reach brain cells and exert their beneficial actions remain to be established. The BBB plays a key role in brain homeostasis by strictly regulating the exchanges between the periphery and the brain, preventing the access of toxic molecules to the brain, while assuring the delivery of essential molecules and the elimination of waste products toward the circulation. Endothelial cells lining the brain microvasculature constitute the basis of the BBB since they are at the interface between the brain and the periphery and have unique characteristics that account for the restricted permeability.43 These features include elaborated junctional complexes between the brain microvascular endothelial cells (BMEC), mainly formed by tight and adherens junctions (TJ and AJ, respectively).43 BMEC are considered the “front line” in the protection of the brain cells from external agents that may lead to oxidative stress, neuroinflammation, vascular reactivity, and excitotoxicity.43,44 Although some studies have shown that HT and PCA can cross the BBB45–47 there is limited knowledge of how their structure influences their brain bioavailability. D'Angelo et al.45 showed that 5 min after intravenous injection of 1.5 mg HT per kg, only 0.31% was found in the rat brain tissue. Additionally, Wu et al.46 showed that 15 min after an intravenous dose of 100 mg of HT per kg the maximum concentration reaching rat brain was 2.1 μg of HT per mL. Furthermore, it has been reported that PCA is present in rat brain following oral administration of Danshen extract.47 Despite the mentioned evidence, no information was found about TYR, TYRS, HT, PCA and SER transport across the BBB. Moreover, demonstration of these compounds’ safety profile regarding BBB integrity as they cross this interface remains to be elucidated.
The aim of the present work is to evaluate the BBB permeation of distinct bioactive compounds present in a MD (TYR, TYRS, HT, SER and PCA) at physiologically relevant concentrations, using confluent monolayers of human BMEC (HBMEC) as a simplified in vitro model of the BBB. Moreover, the BBB properties (barrier tightness, paracellular permeability and expression of an AJ protein) was also assessed to ascertain the BBB integrity in the presence of the bioactive. Our results show that TYR, TYRS, HT, SER and PCA can pass through the human in vitro BBB model to different extents without affecting the integrity of the BBB.
Experimental
Chemicals and reagents
Tyrosine (purity: ≥98%), tyrosol (≥98%), hydroxytyrosol (≥98%), serotonin (≥98%) and protocatechuic acid (≥97%), fetal bovine serum (FBS), Rosewell Park Memorial Institute (RPMI) 1640 medium, non-essential amino acids (NEAA), minimal essential medium (MEM) vitamins, sodium pyruvate, L-glutamine, antibiotic–antimycotic solution, paraformaldehyde (PFA), bovine serum albumin (BSA) and universal mounting medium (DPX), Ringer HEPES solutions and sodium fluorescein were purchased from Sigma Aldrich (St Louis, MO, USA). Thiazolyl blue tetrazolium bromide (MTT) was obtained from Alfa Aesar (Haverhill, MA, USA). Dimethyl sulfoxide (DMSO), isopropanol, hydrochloric acid, and acetonitrile (ACN) were acquired from PanReac AppliChem (Barcelona, Spain). NuSerum IV was provided by Corning Costar Corp (New York, NY, USA). Triton X-100 was purchased from VWR International (Radnor, PA, USA). β-Catenin (rabbit, #71-2700), Alexa Fluor® 555 (goat anti-rabbit, #A21428), and Hoechst 33342 were acquired from Thermo Fisher Scientific (Waltham, MA, USA).
Cell culture and treatment conditions
The human brain microvascular endothelial cells (HBMEC) line was used since it expresses junctional complexes (formed by proteins like β-catenin) that restrict the paracellular permeability of polar molecules, and caveolin-1, the main protein of caveolae, which assure transcellular transport of proteins; moreover, it expresses several influx transporters that assure the entrance of nutrients into the brain, as well as several efflux transports that assure the pumping out of molecules like xenobiotics.48,49 Accordingly, it was considered the most suitable human cell line for an in vitro BBB model concerning barrier tightness, namely for studies of BBB permeation of bioactive compounds.50 HBMEC were cultured as established in our lab.48,51 Briefly, cells were grown in RPMI supplemented with 10% (v/v) FBS, 10% (v/v) NuSerum IV, 1% (v/v) NEAA, 1% (v/v) (MEM) vitamins, 1 mM sodium pyruvate, 2 mM L-glutamine, and 1% (v/v) antibiotic–antimycotic solution (complete medium). Endothelial cell cultures were maintained at 37 °C in a humid atmosphere enriched with 5% CO2. HBMEC, used between passage 25 and 30, were seeded on rat tail collagen-I (100 μg mL−1)-coated plates or Transwell inserts (0.4 μm pore, Corning Costar Corp., New York, NY, USA). Different cell culture conditions were used according to the intended assay, as the cell growth depends on the physical support used for cells’ growth and on the intended confluency. For viability assays, HBMEC (200 μL at a density of 8 × 104 cells per mL) were seeded on 96-well plates and cultured for 48 h to achieve 90% of confluence,51 while for immunocytochemistry, cells (500 μl at a density of 5 × 104 cells per mL) were seeded on coverslips placed in 24-well plates and grown for 72 h to obtain 100% confluence. For BBB integrity and transport assays, HBMEC (500 μL at a density of 1.6 × 105 cells per mL) were seeded on semi-permeable membranes (Transwell inserts) placed in the cell culture well and forming two well-defined compartments: the apical or upper compartment, which can be considered as the “blood-side”, and the basolateral or lower compartment, which is considered the “brain side”, and the confluent HBMEC monolayer represents the BBB.48,49,51 The cells were grown for 4 days to achieve 100% of confluence and acquire junctional complexes formation and restricted permeability.
Tested compounds
Information about the used compounds is presented in Table 1, namely the structure, circulating levels, concentrations of the stock solutions and tested concentrations, which were selected based on the circulating levels. Each compound stock solution was prepared in DMSO, aliquoted and stored at −20 °C until use.
Table 1 Bioactive compounds nomenclature, abbreviation, chemical structure, circulating levels are presented, stock and tested concentration
Name |
Abbreviation |
Structure |
Circulating levels (μM) |
Stock concentrations (mM) |
Tested concentrations (μM) |
Tyrosine |
TYR |
|
60 (ref. 52) |
100 |
MTT: 0.01, 0.1, 1, 10 and 100 |
Transport and integrity: 10 and 100 |
Tyrosol |
TYRS |
|
16 026–270 000 (ref. 53) |
2000 |
MTT: 0.2, 2, 20, 200, 2000 |
7.5 (ref. 54) |
Transport and integrity: 20 and 2000 |
Hydroxytyrosol |
HT |
|
0.15–37 (ref. 30) |
100 |
MTT: 0.01, 0.1, 1, 10, 100 |
Transport and integrity: 1 and 100 |
Protocatechuic acid |
PCA |
|
0.2–2 (ref. 47 and 55) |
100 |
MTT: 0.01, 0.1, 1, 10, 100 |
0.0059–0.44 (ref. 56–58) |
Transport and integrity: 1 and 100 |
Serotonin |
SER |
|
109 (ref. 59) |
200 |
MTT: 0.02, 0.2, 2, 20, 200 |
Transport and integrity: 20 and 200 |
Cells were incubated with the bioactive compounds, diluted in RPMI, or with 0.1% DMSO in RPMI (v/v, control) and incubated at 37 °C. The incubation timepoints were assay dependent.
Cell viability assay
The effect of each compound on HBMEC viability was evaluated via an MTT assay, a colorimetric method that quantifies formazan formation in viable cells, allowing to establish a relation between the intensity of the staining and the cell metabolic activity.51 Forty-eight hours after seeding, cells were incubated with the bioactive compounds, or in their absence (control), for 4 or 24 h. Then, the media were removed and RPMI containing 0.5 mg mL−1 of MTT was added to each well and the plates incubated for 3 h at 37 °C. The supernatant was removed and the formed formazan crystals in viable cells were solubilized with 0.04 N HCl in isopropanol solution. Absorbance values were measured in a microplate reader (Varioskan LUX Multimode Microplate reader, Thermo Fisher Scientific) at 595 nm, and results presented as percentage relatively to control. Solution of Triton X-100 at 1% was used as positive control to validate the methodology.
Blood–brain barrier integrity
HBMEC monolayers integrity was ensured by transendothelial electrical resistance (TEER) measurement, sodium fluorescein (Na–F) paracellular permeability assessment, and the AJ protein β-catenin labelling, as widely accepted BBB integrity parameters.48,49,51,60
Transendothelial electrical resistance
For TEER evaluation, HBMEC in complete medium were seeded on Transwell inserts as described in section Cell culture conditions for 4 days, changing the culture medium at 2 days. The endothelial monolayers were incubated with each compound or with 0.1% DMSO in RPMI (control), added to the upper compartment of the bichamber system. RPMI with 0.1% of DMSO was added to the lower compartment of all conditions, and a 4 h incubation was performed. TEER readings were made using an EndOhmTM chamber coupled to a resistance meter (EVOMX; World Precision Instruments, Inc., USA). The TEER readings were collected before (0 h) and after (4 h) the addition of the bioactive compounds (or DMSO, control). TEER values for the tested conditions (with/without the compounds) were obtained after deducting the values of empty inserts (without cells and compounds) and multiplying by the area of the insert (1.12 cm2). The results were expressed as Ω cm2.61 The average of the TEER values in controls was considered as 100% and the tested conditions were presented as percentage of variation from control. After the last readings, the media from both compartments were collected and used for the transport studies described below, and then the empty inserts were used for the following permeability assay.
Sodium fluorescein paracellular permeability
To evaluate the paracellular permeability of the HBMEC monolayers after exposure to the bioactive compounds, or to 0.1% DMSO in RPMI (control), a permeability assay was conducted using Na–F (molecular weight: 376 Da), as usual in our lab.48,61 Briefly, after TEER measurement, the media were collected and the inserts were washed twice with Ringer–HEPES solution (150 mM NaCl, 5.2 mM KCl, 2.2 mM CaCl2·H2O, 0.2 mM MgSO4·6H2O, 2.8 mM D-glucose, 5 mM Hepes, 6 mM NaHCO3 at pH 7.4) in both compartments. Then, the inserts were transferred to new 12-well plates containing 1.5 mL of Ringer–Hepes solution, and 10 μg mL−1 of Na–F in Ringer–Hepes was added to the upper compartments (0.5 mL) and incubated at 37 °C, protected from light. The inserts were transferred to new wells containing Ringer–Hepes solution at 20, 40 and 60 min. After these times, the inserts were removed and the solutions from the lower compartments were collected and the fluorescein levels were determined using a multimode microplate reader (Varioskan LUX, Thermo Fisher Scientific; excitation: 460 nm and emission: 515 nm). The Na–F flux across the cell-free inserts and the control HBMEC monolayers were also measured. The transendothelial permeability coefficient (Pe) was calculated as described by Deli et al.62 In short, the transport was expressed as microliter of tracer diffusing from upper to lower compartments (eqn (1)): |  | (1) |
Then, average volume cleared was plotted versus time, and permeability × surface area product value for endothelial monolayer (PSe) was calculated by the following formula (eqn (2)):
| PSendothelial−1 = PStime-plotted−1 − PSinsert−1 | (2) |
Finally, PSe was divided by the surface area of the insert (1.12 cm2), giving the endothelial permeability coefficient (Pe in cm s−1). Then, the value obtained for the control was considered 100% and each of the tested compounds/concentrations was expressed as percentage of the control (inserts without extract).
Immunocytochemistry
The BBB integrity was assessed by immunocytochemistry analysis of the AJ protein, β-catenin.51 For that, the cells were seeded as mentioned before, reaching confluence in 72 h. The compounds were then added to cells and incubation was performed for 4 h. Afterwards, the culture media were removed, and the cells were fixed with 4% (w/v) PFA solution for 20 min at room temperature and then washed three times with phosphate-buffered saline (PBS) to proceed for immunocytochemistry. Briefly, cells were permeabilized with 0.3% Triton X-100 for 5 min and blocked with 3% BSA, for 60 min at room temperature. Then, cells were probed with primary antibody for β-catenin (1
:
100), overnight at 4 °C. Thereafter, cells were incubated with the secondary antibody (Alexa Fluor® 555, 1
:
500) for 60 min at room temperature. Both primary and secondary antibodies were diluted in blocking solution. Nuclei were counterstained with Hoechst 33342 dye diluted in PBS (20 μM), for 10 min at room temperature. Between incubations, cells were washed three times with PBS. Methanol-dehydrated cells were then mounted on microscopy slides with DPX, properly dried, and stored at 4 °C until image acquisition. To validate the specificity of labelling, negative controls without primary antibodies were performed. Representative images were acquired at the Faculty of Sciences of the University of Lisbon's Microscopy Facility, a node of the Portuguese Platform for BioImaging, reference PPBI-POCI-01-0145-FEDER-022122, using an Olympus BX60 microscope equipped with Olympus U-RFL-T Mercury lamp and Hamamatsu Orca R2 cooled monochromatic CCD camera, with 40× oil objective.
Transport assays
The percentage of transport of each bioactive compound through the HBMEC monolayer was determined after the media deproteinization from both compartments. Determinations were performed by ultra-high-performance liquid chromatography high resolution mass spectrometry (UHPLC-HRMS/MS) as described below.
Sample deproteinization
To precipitate proteins, 2.5 mL of ACN/1 mL of sample was added dropwise, and samples were vortexed before centrifugation at 3200g for 15 min. Then, the supernatants were collected and dried by using a vacuum concentrator (HyperVAC-LITE, GYOZEN, Korea) at 30 °C and 25g for 24 h. Samples were dissolved in H2O-ACN 10% and immediately analysed by UHPLC-HRMS/MS.
UHPLC-HRMS/MS parameters
The analysis was performed in a Thermo Scientific liquid chromatography system consisting of a binary UHPLC Dionex Ultimate 3000 RS, connected to a quadrupole orbitrap Qexactive hybrid mass spectrometer (Thermo Fisher Scientific, Bremen, Germany), equipped with a heated electrospray ionization probe (HESI-II). The column used was a ZORBAX SB-C18 (2.1 × 100 mm, 1.8 μm particle size) with a guard column (2.1 × 5 mm, 1.8 μm particle size). Both were purchased from Agilent Technologies (Waldbronn, Germany). The separation was performed using column temperature of 40 °C, a flow rate of 0.5 mL min−1, and an injection volume of 5 μL. Chromatographic conditions for each compound are shown in Table S1.† Each bioactive compound was injected in duplicate. Results were processed with the Tracefinder software (Thermo Scientific).
Statistical analysis
Results were analyzed using GraphPad Prism® 6.0 (GraphPad Software, San Diego, CA, USA) and are expressed as mean ± SD. The results represent the average of at least three independent experiments, with three technical triplicates in the case of the viability assay. Data normality was tested with D'Agostino Pearson test. One-way ANOVA was used since all results follow a normal distribution.
Results
Bioactive compounds present no toxicity to HBMEC
The major objective of this assay is to ascertain if the bioactive compounds (TYR, TYRS, HT, SER and PCA), at physiologically relevant concentrations, can cross the BBB without causing damage to the brain microvascular endothelium. So, an initial viability assay by the MTT test was performed in HBMEC cultures (Fig. 1) to determine the safe concentrations to use in the following experiments, as well as the timepoint for compounds incubation (4 or 24 h, Fig. 1A or B, respectively).
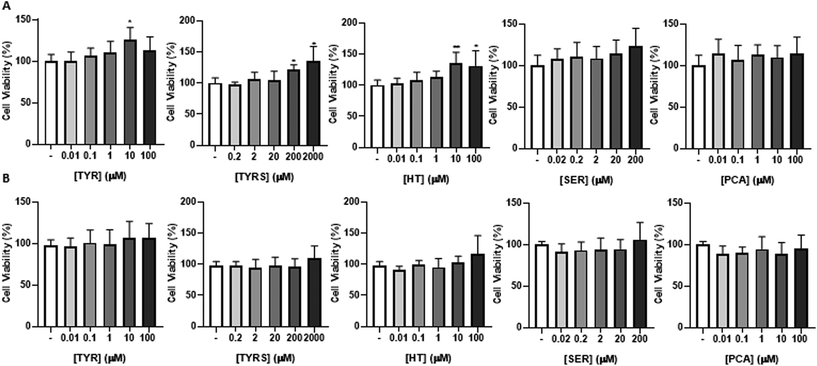 |
| Fig. 1 Human brain microvascular endothelial cells (HBMEC) viability upon exposure to the bioactive compounds. HBMEC were treated with the indicated concentrations of tyrosine (TYR), tyrosol (TYRS), hydroxytyrosol (HT), serotonin (SER) and protocatechuic acid (PCA) diluted in RPMI, or with 0.1% DMSO in RPMI (negative control), for 4 h (A) or 24 h (B). Cell viability was assessed by the MTT assay, and the values are presented as percentage relatively to control. No toxicity was observed for any compound at the tested concentrations and timepoints. All values are represented as mean ± SD of three independent experiments performed in triplicate. Statistical analysis was performed by One-way ANOVA and the significance are shown as *p < 0.05, **p < 0.01 vs. control. | |
Importantly, the bioactive compounds were tested according to the physiological levels in plasma, detailed in Table 1,30,47,52–59 and results were expressed as fold change from control (cells incubated in the absence of the compounds). In parallel, a positive control was performed by treatment of HBMEC with Triton X-100 at 1%, which revealed that formazan is not formed (absorbance close to 0), indicating a drastic loss of cell viability. In contrast, no toxic effect was observed for each compound at all concentrations and tested timepoints. Based on these results, all the compounds were selected for the next studies to assess their capability to cross the BBB. In addition, two concentrations of each compound were selected based on their proximity to the circulation levels of each one of them (Table 1), as well as a timepoint of 4 h.
The bioactive compounds display no BBB integrity impairment
We next wanted to ensure that the bioactive compounds at safe concentrations (Fig. 1) do not perturb the integrity of the endothelial monolayer. For that, the widely used BBB integrity markers, TEER, Na–F paracellular permeability, and β-catenin expression, were evaluated (Fig. 2A) using a well-validated two chamber system as an in vitro model of the BBB,48 in which the tested compounds (or media; control) were separately added to the “blood compartment” of the bichamber system (Fig. 2A).
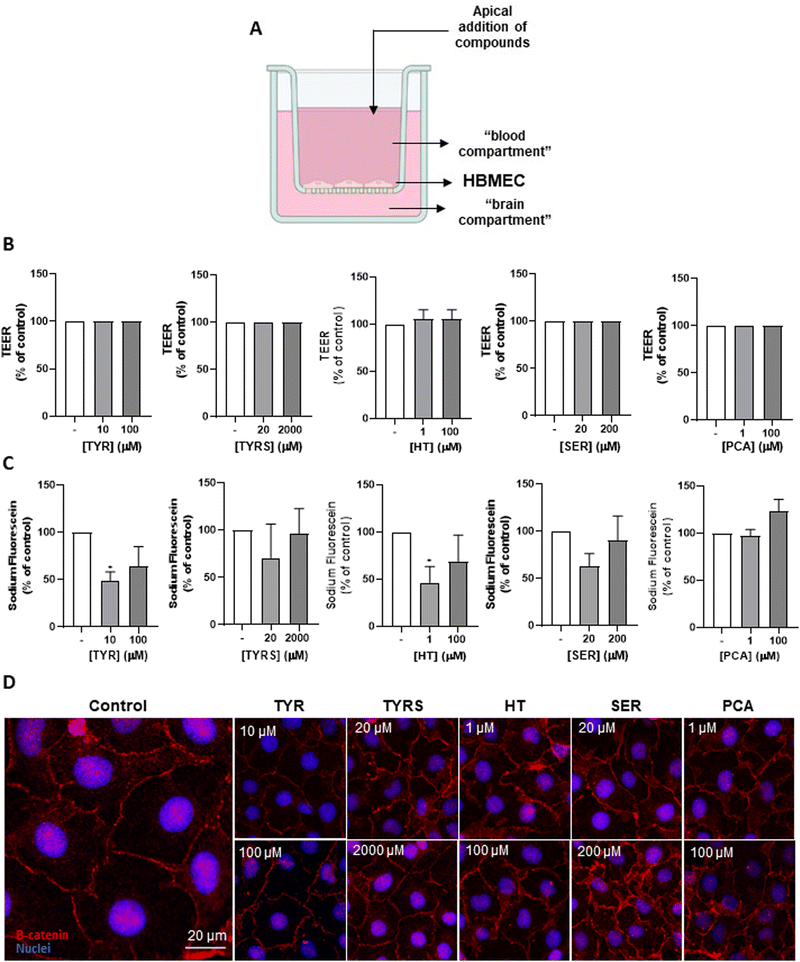 |
| Fig. 2 Effect of bioactive compounds in blood–brain barrier (BBB) integrity. Human brain microvascular endothelial cells (HBMEC) were seeded onto semi-permeable membranes. At confluence, they were treated with the indicated concentrations of tyrosine (TYR), tyrosol (TYRS), hydroxytyrosol (HT), serotonin (SER) and protocatechuic acid (PCA) diluted in RPMI, or with 0.1% DMSO in RPMI (negative control), for 4 h. Schematic experimental design used to assess compounds transport across the BBB (A). Alterations in BBB properties were evaluated by transendothelial electrical resistance (TEER) measurements (B), paracellular permeability to sodium fluorescein evaluation (C) and immunocytochemical analysis of β-catenin (red) (D). Nuclei were counterstained with Hoechst33342 dye (blue). All the parameters revealed no endothelial impairment by the exposure of the bioactive compounds. Results are expressed as mean ± SD of three independent experiments. Statistical analysis was performed by One-way ANOVA and the significances are shown as *p < 0.05 vs. control. | |
Measurement of TEER revealed that all the compounds at the studied concentrations do not induce alterations relative to the control condition (Fig. 2B). The measurement of Na–F flux demonstrated that TYRS, SER and PCA did not affect the permeability of the endothelial monolayer at both tested concentrations, while TYR and HT at 1 and 10 μM, respectively, even induced a significant decrease compared to control (p < 0.05), which indicates a less permeable or tighter barrier (Fig. 2C).
The immunocytochemical analysis of the AJ protein β-catenin following exposure to the bioactive compounds showed a junctional protein expression like the control and a well-defined location at the membrane level (Fig. 2D), reinforcing the results obtained by the two previous parameters. Together, these results suggest that the exposure of the BBB endothelium to the bioactive compounds does not cause harmful changes in the BBB integrity.
Bioactive compounds are transported across the BBB endothelium
To investigate the endothelial transport of the bioactive compounds, they were added to the upper compartment of the bichamber system and their putative transport through the BBB endothelium was assessed after 4 h incubation. The presence of each compound in the media from both chambers (“blood” and “brain” compartments) was quantified by UHPLC-HRMS/MS. Our results revealed that different concentrations of each compound were detected in the upper and lower compartments, suggesting a differential transport of the molecules (Fig. 3). The sum of the concentrations in the apical and basal compartments did not match the concentration initially added, probably due to potential metabolization as reported in literature.49 The endothelial transport of TYR, TYRS and HT was similar regardless of the concentration used (Fig. 3A). However, in the case of SER and PCA the endothelial transport significantly increased with the concentrations (Fig. 3B and C, respectively). HT proved to be the bioactive compound with the highest ability to cross the BBB (∼70%). It was followed by TYR (∼50%), TYRS (∼30%), and by SER (∼30%) and PCA (9%), at their highest concentration. The incubations with the lowest concentrations of SER and PCA resulted in modest SER transport percentage (4%) (Fig. 3B) and a negligible (0.08%) transport for PCA (Fig. 3C). Considering that the study of BBB integrity showed no disruption by exposure to the bioactive compounds, we can conclude that their detection in the “brain” compartment is due to permeation across the BBB endothelium and not to loss of BBB integrity.
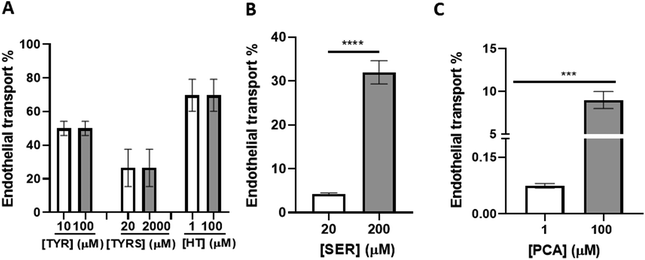 |
| Fig. 3 Transport efficiency of bioactive compounds across the blood–brain barrier (BBB) endothelium. Human brain microvascular endothelial cells (HBMEC) were seeded onto semi-permeable membranes. At confluence, they were treated for 4 h with the indicated concentrations of tyrosine (TYR), tyrosol (TYRS), hydroxytyrosol (HT) (A), serotonin (SER) (B) and protocatechuic acid (PCA) (C). The upper and lower compartments media were collected, and the endothelial transport was evaluated by UHPLC-HRMS/MS. The transport percentage (%) was determined by the ratio of the lower compartment concentration and the sum of the concentration in both upper and lower compartments. All values are mean ± SD of three independent experiments. Statistical analysis was performed by One-way ANOVA. The significances are shown as ****p < 0.0001 and ***p < 0.001 between concentrations. | |
Discussion
There is ample evidence that polyphenolic and indolic compounds present in the diet, as well as their metabolites, exhibit neuroprotective potential.29,30,63–65 Recently, studies with human subjects reported 0.25 μM of TYRS and 1.42 μM of HT recovered in urine after a dietary ingestion of a dose of 40 g of Extra Virgin Olive Oil.66 Gallardo-Fernández et al.67 estimated daily dietary intake of HT 17.25 mg per HT provided by two spoons (40 g) of virgin extra olive oil, 2 glasses of wine (6.2 mg per HT) and 7 olives, 20 g (11 mg per HT), characteristic mediterranean foods. Furthermore, the bioavailability data reported for HT is 44%68 approximately and if we consider the dilution in 5 L of plasma, the estimated circulating HT from the diet can be up to 10.69 μM which is in the range of our study.
PCA is present in many foods as described in the introduction section and has been determined in human plasma in dietetic interventions with different foods. For instance, Castello et al.56 found 0.0059 μM PCA in plasma after dietetic intake of grape pomace. Feliciano et al.57 found 0.11 μM after the consumption of cranberry juice. Sawicki et al.58 reported plasmatic concentrations of 0.24, 0.22, 0.44 μM (after de intake of muffins made with white flour, barley, and oats respectively). Additionally, it is a metabolite of anthocyanins and can be detected in plasma after the consumption of food extracts as aronia.69 Therefore, the estimation of the overall circulating levels is more complex than for TYRS and HT which is restricted to a reduced number of foods. But given reported values in literature, it is reasonable to assume that consuming a varied diet including whole grains, vegetables, and fruits, it can be reached 1 μM, as used in our study.
Once in circulation, their distribution to target tissues such as the brain relies on their ability to cross the BBB, one of the most controlled barriers in the organism. This fact, allied to the poor absorption, rapid metabolism, and elimination, may limit the bioavailability, and consequently their neuroprotective efficacy.70,71 However, it is not yet completely clear if these compounds can cross the BBB or how their structure may influence brain bioavailability. Our previous studies demonstrated that differences in brain endothelial transport could be related to the degree of chemical modification in gallic acid and vanillic acid, where methylation combined with sulfation enhanced the transport of bioavailable polyphenols metabolites.49 A crucial aspect to consider when studying the brain permeation of a dietary compound is the concentration found in circulation following dietary consumption. So, in the present study, we used each compound at concentrations within the range of those previously reported in circulation, this way assuring the physiological relevance of our findings. Another crucial aspect is to assure the safety of the tested compounds in the experimental setup. Exposure of HBMEC to TYR, TYRS, HT, SER or PCA, in the physiologically relevant concentrations did not affect cell viability, demonstrating the absence of toxicity to the BBB endothelium.
Despite the complexity of the BBB in vivo, where endothelial cells are entreated by a thick basement membrane and by pericytes and astrocytes end-feet, most BBB in vitro models rely on the use of BMEC, considered to form the anatomic basis of the BBB.43 Particularly, the cell line here used, HBMEC, has been employed in multiple studies of BBB permeation of natural compounds and of drug candidates,6,72,73 which attests its scientific recognition and value as a simplified in vitro model of the BBB to predict the potential BBB transport of bioactives.
Upon exposure to the compounds no HBMEC impairment was observed based on TEER and paracellular permeability to Na–F, revealing that the integrity of the barrier is not compromised. This was reinforced by the immunostaining of β-catenin, which revealed no changes in the expression of the AJ protein by exposure to the compounds. Collectively, these results attest that the BBB endothelial integrity is maintained upon exposure to each of the MD components, therefore providing a basis for the reliability of the BBB permeation studies. Furthermore, HT (1 μM) reduced the BBB permeability, indicating that it induces the tightness of the barrier. This result is consistent with a previous in vivo study that suggests pretreatment with dietary VOO, in which HT is present, may reduce BBB permeability.74 Based on TEER results, Mursaleen et al.75 observed that the integrity of the BBB is not affected by HT (10–20 μM). Our work corroborates these findings using additionally Na–F assay and β-catenin expression analysis. Interestingly, we recently reported an increase of HBMEC barrier tightness upon exposure to compounds found in chicory,73 indicating that a tightness of the BBB may be common to different components of the MD.
Certain insults can disrupt the BBB. This is the case of chronic stress in rats in which the tight junction (TJ) proteins zonula occludens (ZO)-1 and claudin-5 can be reduced, as determined by ELISA.76 Consequently, permeability measured by Evans blue increased, promoting the brain uptake of HT which thereafter can exert its beneficial effects. Regarding PCA, it promoted TJ expression in vascular endothelial cells after intracerebral hemorrhage.77 All in all, our results are aligned with the literature up to date concerning integrity, as these compounds do not affect it or even decrease permeability by increasing TJ proteins. After exposure of HBMEC luminal surface to TYR, TYRS, HT, PCA and SER, in the bichamber system, we have detected by means of UHPLC-HRMS/MS the presence of each of the compounds in the basal compartment (“brain side”), suggesting that these compounds are able to cross the BBB endothelium towards the brain. These results point to the brain bioavailability of molecules with known neuroprotective features. TYR, TYRS, HT were shown to cross the barrier to different extents, consistent with previous in vivo studies indicating that TYRS and HT can cross the BBB.45,46 After de administration to rats of 50 mg kg−1 salidroside (a tyrosol glucoside), TYR was detected in the cerebral tissue. These results suggest a metabolization of the glucoside yielding TYR which eventually would pass through BBB.78 TYR (10 and 100 μM) showed 50% transendothelial transport through the BBB endothelium, TYRS (20 and 2000 μM) showed 30%, and HT (1 and 100 μM) showed 70%, for both concentrations tested of each compound. Similarly, Mursaleen et al.75 obtained the same passing percentage for HT at 10 and 20 μM, even when they tested a shorter incubation time (1 hour). Hence, we can hypothesize that they may pass through the BBB by passive transport.
The apparent permeability assessed by predicted logP values for HT is higher than that of TYRS (0.85–1.19 and 0.13–0.89, respectively).79 In line with this, the present endothelial transport results showed a greater ability to cross the BBB endothelium for HT (∼70%) than for TYRS (∼30%). Figueira et al.65 speculated that intermolecular hydrogen bonds could influence the BBB permeation of the polyphenols. Thus, the ortho-diphenolic substitution present in HT may account for the higher ability to cross the barrier than a single hydroxyl substitution, as in TYRS. Indeed, the most efficient hydrogen-donor systems as the one of the dihydroxy functionality allows the molecules to cross the barrier more freely.80 On the other hand, previous studies have shown that HT transport occurs via a passive diffusion mechanism and is bidirectional in Caco-2 cells.81
The transendothelial transport of SER (20 and 200 μM) showed different percentages depending on the concentration (4 and 30%, respectively). In the case of PCA (1 and 100 μM) we also obtained different percentages (0.08 and 9%, respectively). PCA has been detected in the brain of rats fed with Danshen extract despite PCA was not present in the initial food, but it is considered a metabolite.47 Lexchin et al.82 reported that doses up to 0.435 mg kg−1 SER do not cross the BBB in the rat. According to these authors, the level at which SER passes the BBB was found to be at least 0.863 mg kg−1. This marked dose-dependent effect of SER agrees with our results. More recently, Sharma et al.83 verified that increasing 5-hydroxytryptophan, a SER precursor, the amount of SER increased in brain. Additionally, Brust et al.84 demonstrated that SER transporter mRNA is present in the brain vascular endothelial cells. All in all, it is conceivable that SER transporters presents in the BBB, which could be involved in its crossing.85
Figueira et al.65 proposed that the quercetin detected in the brain may result from the degradation of conjugated forms, which are transported across the BBB, and quercetin alone may not be able to be transported. Accordingly, a very low efficiency of free quercetin transport (around 8% after 18 h incubation) was reported.86 Furthermore, using HBMEC monolayers as a BBB in vitro model, as presently, we previously showed that the BBB endothelium is rapidly crossed by epigallocatechin gallate (EGCG) and more slowly by cyanidin-3-glucoside (C3G), but not by quercetin.60 In the same way, the efficiency of transport of PCA (100 μM) is also very low and negligible for PCA at 1 μM. The fact that we detected a low concentration of PCA and SER in the basal compartment could be attributed to several factors such as: a low uptake at the apical membrane, a reduced transport through the basolateral membrane, or a high metabolism/degradation. We can hypothesize that such metabolism may occur at the BBB level, but we cannot confirm this from our UHPLC-HRMS/MS data.
Conclusions
Our work illustrates how bioaccessible TYR, TYRS, HT, SER and PCA, at physiological concentrations, may cross the BBB endothelium, a key step for their access to brain cells and exert their neuroprotective effects. From the studied compounds, HT presents the highest ability to cross the BBB (70%) follow by TYR (50%), TYRS (30%), SER (30%) and PCA (9%) without disrupting the integrity of the BBB. However, further studies are needed to reveal how these compounds pass through the BBB, which would expand the current knowledge on the permeation of these compounds through the BBB.
Author contributions
Conceptualization: A.R.G., M.A.B., M.C.G.P., A.M.T.; methodology: A.R.G. and M.G.F.; formal analysis: A.R.G. and M.G.F.; investigation: A.R.G. and M.G.-F.; writing—original draft preparation: A.R.G. and M.G.-F.; writing—review and editing: A.R.G., M.A.B., R.H.O., M.C.G.-P. and A.M.T.; supervision: M.A.B., M.C.G.P. and A.M.T; project administration: M.A.B., M.C.G.P and A.M.T.; funding acquisition: M.A.B., M.C.G.P. and A.M.T. All authors have read and agreed to the published version of the manuscript.
Conflicts of interest
There are no conflicts to declare.
Acknowledgements
We acknowledge the Microscopy Facility of the Faculty of Sciences of the University of Lisbon, and UHPLC-HRMS/MS services (CITIUS) of the University of Seville for the equipment.
This research was funded by Grant MICINN PID2019-108722RB-C32 funded by MCIN/AEI/10.13039/501100011033, Ministerio de Ciencia, Innovación y Universidades; Grant AGL 2016-77505-C3-2-R funded by MICIU/AEI/10.13039/501100011033 y por FSE invierte en tu futuro, Ministerio de Ciencia, Innovación y Universidades for the predoctoral fellowship (M. G. F.’ FPI contract), R. H. O’ postdoctoral contract (Grant IJC2020-045695-I funded by MCIN/AEI/10.13039/501100011033, European Union NextGeneration EU/PRTR), and by the Portuguese Foundation for Science and Technology (UIDB/04138/2020 and UIDP/04138/2020).
References
- G. Deuschl, E. Beghi, F. Fazekas, T. Varga, K. A. Christoforidi, E. Sipido, C. L. Basseti, T. Vos and V. L. Feigin, The burden of neurological diseases in Europe: an analysis for the Global Burden of Disease Study, 2017, Lancet Public Health, 2020, 5, 551–567 CrossRef PubMed.
- E. Nichols, J. D. Steinmetz, S. E. Vollset, K. Fukutaki, J. Chalek, F. Abd-Allah, A. Abdoli, A. Abualhasan, E. Abu-Gharbieh, T. T. Akram and H. Al Hamad, Estimation of the global prevalence of dementia in 2019 and forecasted prevalence in 2050: an analysis for the Global Burden of Disease Study 2019, Lancet Public Health, 2022, 7, 105–125 CrossRef PubMed.
-
World Health Organization. A report about dementia. https://www.who.int/news-room/fact-sheets/detail/dementia (20, September, 2022).
-
World Health Organization. A report about dementia. https://www.who.int/news-room/fact-sheets/detail/parkinson-disease (16, May, 2023).
- I. Figueira, A. Fernandes, A. M. Djordjevic, A. Lopez-Contreras, C. M. Henriques, C. Selman, E. Ferreiro, E. S. Gonos, J. L. Trejo, J. Misra and L. J. Rasmussen, Interventions for age-related dise ases: Shifting the paradigm, Mech. Ageing Dev., 2016, 160, 69–92 CrossRef PubMed.
- D. Angelino, D. Carregosa, C. Domenech-Coca, M. Savi, I. Figueira, N. Brindani, S. Jang, S. Lakshman, A. Molokin, J. F. Urban Jr. and C. D. Davis, 5-(Hydroxyphenyl)-γ-Valerolactone-Sulfate, a Key Microbial Metabolite of Flavan-3-ols, Is Able to Reach the Brain: Evidence from Different in Silico, In Vitro and In Vivo Experimental Models, Nutrients, 2019, 11, 2678 CrossRef CAS PubMed.
- P. Agarwal, Y. Wang, N. T. Aggarwal, L. J. Cherian, B. D. James, K. Dhana, S. E. Leurgans, D. A. Bennett and J. A. Schneider, MIND and Mediterranean diet association with Alzheimer's disease pathology, Alzheimers Dement., 2021, 17, e055679 CrossRef CAS.
- A. Metcalfe-Roach, A. C. Yu, E. Golz, M. Cirstea, K. Sundvick, D. Kliger, L. H. Foulger and S. Appel-Cresswell, MIND and Mediterranean diets associated with later onset of Parkinson's disease, Mov. Disord., 2021, 36, 977–984 CrossRef CAS PubMed.
- F. Armeli, A. Bonucci, E. Maggi, A. Pinto and R. Businaro, Mediterranean diet and neurodegenerative diseases: the neglected role of nutrition in the modulation of the endocannabinoid system, Biomolecules, 2021, 11, 790 CrossRef CAS PubMed.
- S. Davinelli, A. Trichopoulou, G. Corbi, I. De Vivo and G. Scapagnini, The potential nutrigeroprotective role of Mediterranean diet and its functional components on telomere length dynamics, Ageing Res. Rev., 2019, 49, 1–10 CrossRef CAS PubMed.
- C. Angeloni, M. Malaguti, M. C. Barbalace and S. Hrelia, Bioactivity of olive oil phenols in neuroprotection, Int. J. Mol. Sci., 2017, 18, 2230 CrossRef PubMed.
- I. Figueira, R. Menezes, D. Macedo, I. Costa and C. Nunes dos Santos, Polyphenols beyond barriers: a glimpse into the brain, Curr. Neuropharmacol., 2017, 15, 562–594 CrossRef CAS PubMed.
- M. Gallardo-Fernández, M. Gonzalez-Ramirez, A. B. Cerezo, A. M. Troncoso and M. C. Garcia-Parrilla, Hydroxytyrosol in Foods: Analysis, Food Sources, EU Dietary Intake, and Potential Uses, Foods, 2022, 11, 2355 CrossRef PubMed.
- M. A. Álvarez-Fernández, E. Fernández-Cruz, E. Cantos-Villar, A. M. Tronco so and M. C. García-Parrilla, Determination of hydroxytyrosol produced by winemaking yeasts during alcoholic fermentation using a validated UHPLC–HRMS method, Food Chem., 2018, 242, 345–351 CrossRef PubMed.
- P. Vitaglione, G. Donnarumma, A. Napolitano, F. Galvano, A. Gallo, L. Scalfi and V. Fogliano, Protocatechuic acid is the major human metabolite of cyanidin-glucosides, J. Nutr., 2007, 137, 2043–2048 CrossRef CAS PubMed.
- R. C. Pimpão, T. Dew, M. E. Figueira, G. J. McDougall, D. Stewart, R. B. Ferreira, C. N. Santos and G. Williamson, Urinary metabolite profiling identifies novel colonic metabolites and conjugates of phenolics in healthy volunteers, Mol. Nutr. Food Res., 2014, 58, 1414–1425 CrossRef PubMed.
- V. Neveu, J. Perez-Jiménez, F. Vos, V. Crespy, L. du Chaffaut, L. Mennen, C. Knox, R. Eisner, J. Cruz, D. Wishart and A. Scalbert, Phenol-Explorer: an online comprehensive database on polyphenol contents in foods, Database, 2010, 2010 DOI:10.1093/database/bap024.
- J. A. Rothwell, M. Urpi-Sarda, M. Boto-Ordoñez, C. Knox, R. Llorach, R. Eisner, J. Cruz, V. Neveu, D. Wishart, C. Manach and C. Andres-Lacueva, Phenol-Explorer 2.0: a major update of the Phenol-Explorer database integrating data on polyphenol metabolism and pharmacokinetics in humans and experimental animals, Database, 2012, 2012 DOI:10.1093/database/bas031.
- J. A. Rothwell, J. Pérez-Jiménez, V. Neveu, A. Medina-Ramon, N. M’Hiri, P. Garcia Lobato, C. Manach, K. Knox, R. Eisner, D. Wishart and A. Scalbert, Phenol-Explorer 3.0: a major update of the Phenol-Explorer database to incorporate data on the effects of food processing on polyphenol content, Database, 2013, 2013 DOI:10.1093/database/bat070.
- J. L. Bermudez-Gonzalez, D. Sanchez-Quintero, L. Proaño-Bernal, R. Santana-Apreza, M. A. Jimenez-Chavarria, J. A. Luna-Alvarez-Amezquita, J. I. Straface, A. M. Perez-Partida, J. Berarducci, J. I. Armenta-Moreno and K. J. Garza-Cruz, Role of the Antioxidant Activity of Melatonin in Myocardial Ischemia-Reperfusion Injury, Antioxidants, 2022, 11, 627 CrossRef CAS PubMed.
- J. Florido, C. Rodriguez-Santana, L. Martinez-Ruiz, A. López-Rodríguez, D. Acuña-Castroviejo, I. Rusanova and G. Escames, Understanding the mechanism of action of melatonin, which induces ROS production in cancer cells, Antioxidants, 2022, 11, 1621 CrossRef CAS PubMed.
- A. K. Verma, S. Singh, G. Garg and S. I. Rizvi, Melatonin exerts neuroprotection in a chronodisrupted rat model through reduction in oxidative stress and modulation of autophagy, Chronobiol. Int., 2022, 39, 45–56 CrossRef CAS PubMed.
- M. Stürtz, A. B. Cerezo, E. Cantos-Villar and M. C. Garcia-Parrilla, Determination of the melatonin content of different varieties of tomatoes (Lycopersicon esculentum) and strawberries (Fragaria ananassa), Food Chem., 2011, 127, 1329–1334 CrossRef PubMed.
- Y. Zhao, D. X. Tan, Q. Lei, H. Chen, L. Wang, Q. T. Li, Y. Gao and J. Kong, Melatonin and its potential biological functions in the fruits of sweet cherry, J. Pineal Res., 2013, 55, 79–88 CrossRef CAS PubMed.
- Q. Lei, L. Wang, D. X. Tan, Y. Zhao, X. D. Zheng, H. Chen, Q. T. Li, B. X. Zuo and J. Kong, Identification of genes for melatonin synthetic enzymes in ‘R ed F uji'apple (M alus domestica B orkh. cv. R ed) and their expression and melatonin production during fruit development, J. Pineal Res., 2013, 55, 443–451 CrossRef CAS PubMed.
- R. J. Reiter, L. C. Manchester and D. X. Tan, Melatonin in walnuts: influence on levels of melatonin and total antioxidant capacity of blood, Nutrition, 2005, 21, 920–924 CrossRef CAS PubMed.
- E. Oladi, M. Mohamadi, T. Shamspur and A. Mostafavi, Spectrofluorimetric determination of melatonin in kernels of four different Pistacia
varieties after ultrasound-assisted solid–liquid extraction, Spectrochim. Acta, Part A, 2014, 132, 326–329 CrossRef CAS PubMed.
- M. I. Rodriguez-Naranjo, A. Gil-Izquierdo, A. M. Troncoso, E. Cantos and M. C. Garcia-Parrilla, Melatonin: a new bioactive compound in wine, J. Food Compos. Anal., 2011, 24, 603–608 CrossRef CAS.
- R. Hornedo-Ortega, M. A. Alvarez-Fernandez, A. B. Cerezo, T. Richard, A. M. Troncoso and M. C. Garcia-Parrilla, Protocatechuic acid: inhibition of fibril formation, destabilization of preformed fibrils of amyloid-β and α-synuclein, and neuroprotection, J. Agric. Food Chem., 2016, 64, 7722–7732 CrossRef CAS PubMed.
- R. Hornedo-Ortega, A. B. Cerezo, A. M. Troncoso and M. C. Garcia-Parrilla, Protective effects of hydroxytyrosol against α-synuclein toxicity on PC12 cells and fibril formation, Food Chem. Toxicol., 2018, 120, 41–49 CrossRef CAS PubMed.
- M. Gallardo-Fernández, R. Hornedo-Ortega, A. B. Cerezo, A. M. Troncoso and M. C. García-Parrilla, Melatonin, protocatechuic acid and hydroxytyrosol effects on vitagenes system against alpha-synuclein toxicity, Food Chem. Toxicol., 2019, 134, 110817 CrossRef PubMed.
- J. N. Zhou, R. Y. Liu, W. Kamphorst, M. A. Hofman and D. F. Swaab, Early neuropathological Alzheimer's changes in aged individuals are accompanied by decreased cerebrospinal fluid melatonin levels, J. Pineal Res., 2003, 35, 125–130 CrossRef CAS PubMed.
- J. Greilberger, D. Fuchs, F. Leblhuber, M. Greilberger, R. Wintersteiger and E. Tafeit, Carbonyl proteins as a clinical marker in Alzheimer's disease and its relation to tryptophan degradation and immune activation, Clin. Lab., 2010, 56, 441 CAS.
- R. Hornedo-Ortega, G. Da Costa, A. B. Cerezo, A. M. Troncoso, T. Richard and M. C. Garcia-Parrilla, In Vitro Effects of Serotonin, Melatonin, and Other Related Indole Compounds on Amyloid–β Kinetics and Neuroprotection, Mol. Nutr. Food Res., 2018, 62, 1700383 CrossRef PubMed.
- F. Visioli, M. Rodríguez-Pérez, Ó. Gómez-Torres, C. Pintado-Losa and E. Burgos-Ramos, Hydroxytyrosol improves mitochondrial energetics of a cellular model of Alzheimer's disease, Nutr. Neurosci., 2022, 25, 990–1000 CrossRef CAS PubMed.
- D. S. Goldstein, Y. Jinsmaa, P. Sullivan, C. Holmes, I. J. Kopin and Y. Sharabi, 3,4-Dihydroxyphenylethanol (Hydroxytyrosol) Mitigates the Increase in Spontaneous Oxidation of Dopamine During Monoamine Oxidase Inhibition in PC12 Cells, Neurochem. Res., 2016, 41, 2173–2178 CrossRef CAS PubMed.
- G. A. Perez-Barron, S. Montes, M. Rubio-Osornio, J. G. Avila-Acevedo, S. Garcia-Jimenez, L. C. Rios and A. Monroy-Noyola, Hydroxytyrosol inhibits MAO isoforms and prevents neurotoxicity inducible by MPP+ invivo, Front. Biosci., 2020, 1, 25–37 Search PubMed.
- G. Perez-Barron, S. Montes, Y. Aguirre-Vidal, M. Santiago, E. Gallardo, J. L. Espartero, C. Ríos and A. Monroy-Noyola, Antioxidant Effect of Hydroxytyrosol, Hydroxytyrosol Acetate and Nitrohydroxytyrosol in a Rat MPP+ Model of Parkinson's Disease, Neurochem. Res., 2021, 46, 2923–2935 CrossRef CAS PubMed.
- J. C. Garcia-Moreno, M. Porta de la Riva, E. Martínez-Lara, E. Siles and A. Cañuelo, Tyrosol, a simple phenol from EVOO, targets multiple pathogenic mechanisms of neurodegeneration in a C. elegans model of Parkinson's disease, Neurobiol. Aging, 2019, 82, 60–68 CrossRef CAS PubMed.
- S. Guan, X. L. Zhang, D. Ge, T. Q. Liu, X. H. Ma and Z. F. Cui, Protocatechuic acid promotes the neuronal differentiation and facilitates survival of phenotypes differentiated from cultured neural stem and progenitor cells, Eur. J. Pharmacol., 2011, 67, 471–478 CrossRef PubMed.
- V. N. Thakare, V. D. Dhakane and B. M. Patel, Attenuation of acute restraint stress-induced depressive like behavior and hippocampal alterations with protocatechuic acid treatment in mice, Metab. Brain Dis., 2016, 32, 401–413 CrossRef PubMed.
- Z. Xi, X. Chen, C. Xu, B. Wang, Z. Zhong, Q. Sun, Y. Sun and L. Bian, Protocatechuic acid attenuates brain edema and blood-brain barrier disruption after intracerebral hemorrhage in mice by promoting Nrf2/HO-1 pathway, Neuroreport, 2020, 31, 1274–1282 CrossRef CAS PubMed.
- F. L. Cardoso, D. Brites and M. A. Brito, Looking at the blood–brain barrier: molecular anatomy and possible investigation approaches, Brain Res., 2010, 64, 328–363 CrossRef CAS PubMed.
- N. J. Abbott, L. Rönnbäck and E. Hansson, Astrocyte–endothelial interactions at the blood–brain barrier, Nat. Rev. Neurosci., 2006, 7, 41–53 CrossRef CAS PubMed.
- S. D'Angelo, C. Manna, V. Migliardi, O. Mazzoni, P. Morrica, G. Capasso, G. Pontoni, P. Galletti and V. Zappia, Pharmacokinetics and metabolism of hydroxytyrosol, a natural antioxidant from olive oil, Drug Metab. Dispos., 2001, 29, 1492–1498 Search PubMed.
- Y. T. Wu, L. C. Lin and T. H. Tsai, Measurement of free hydroxytyrosol in microdialysates from blood and brain of anesthetized rats by liquid chromatography with fluorescence detection, J. Chromatogr. A, 2009, 1216, 3501–3507 CrossRef CAS PubMed.
- Y. J. Zhang, L. Wu, Q. L. Zhang, J. Li, F. X. Yin and Y. Yuan, Pharmacokinetics of phenolic compounds of Danshen extract in rat blood and brain by microdialysis sampling, J. Ethnopharmacol., 2011, 136, 129–136 CrossRef CAS PubMed.
- I. Palmela, H. Sasaki, F. L. Cardoso, M. Moutinho, K. S. Kim, D. Brites and M. A. Brito, Time-dependent dual effects of high levels of unconjugated bilirubin on the human blood-brain barrier lining, Front. Cell. Neurosci., 2012, 6, 22 CAS.
- I. Figueira, G. Garcia, R. C. Pimpão, A. P. Terrasso, I. Costa, A. F. Almeida, L. Tavares, T. F. Pais, P. Pinto, M. R. Ventura and A. Filipe, Polyphenols journey through blood-brain barrier towards neuronal protection, Sci. Rep., 2017, 7, 1–16 CrossRef CAS PubMed.
- D. E. Eigenmann, E. A. Jähne, M. Smieško, M. Hamburger and M. Oufir, Validation of an immortalized human (hBMEC) in vitro blood-brain barrier model, Anal. Bioanal. Chem., 2016, 408, 2095–2107 CrossRef CAS PubMed.
- J. Godinho-Pereira, M. D. Lopes, A. R. Garcia, H. M. Botelho, R. Malhó, I. Figueira and M. A. A. Brito, Drug Screening Reveals Minocycline Hydrochloride as a Therapeutic Option to Prevent Breast Cancer Cells Extravasation across the Blood–Brain Barrier, Biomedicines, 2022, 10, 1988 CrossRef CAS PubMed.
- S. Letellier, J. P. Garnier, J. Spy and B. Bousquet, Determination of the l-DOPA/l-tyrosine ratio in human plasma by high-performance liquid chromatography: Usefulness as a marker in metastatic malignant melanoma, J. Chromatogr. B: Biomed. Sci. Appl., 1997, 696, 9–17 CrossRef CAS PubMed.
- C. Grizis, J. Atta-Politou and M. A. Koupparis, Simultaneous determination of oleuropein and tyrosol in plasma using high performance liquid chromatography with UV detection, J. Liq. Chromatogr. Relat. Technol., 2003, 26, 599–616 CrossRef CAS.
- A. Boronat, J. Mateus, N. Soldevila-Domenech, M. Guerra, J. Rodríguez-Morató, C. Varon, D. Muñoz, F. Barbosa, J. C. Morales, A. Gaedigk, K. Langohr, M. I. Covas, C. Pérez-Mañá, M. Fitó, R. F. Tyndale and R. de la Torre, Cardiovascular benefits of tyrosol and its endogenous conversion into hydroxytyrosol in humans. A randomized, controlled trial, Free Radicals Biol. Med., 2019, 143, 471–481 CrossRef CAS PubMed.
- C. Czank, A. Cassidy, Q. Zhang, D. J. Morrison, T. Preston, P. A. Kroon, N. P. Botting and C. D. Kay, Human metabolism and elimination of the anthocyanin, cyanidin-3-glucoside: a 13C-tracer study, Am. J. Clin. Nutr., 2013, 97, 995–1003 CrossRef CAS PubMed.
- F. Castello, G. Costabile, L. Bresciani, M. Tassotti, D. Naviglio, D. Luongo, P. Ciciola, M. Vitale, C. Vetrani, G. Galaverna, F. Brighenti, R. Giacco, D. Del Rio and P. Mena, Bioavailability and pharmacokinetic profile of grape pomace phenolic compounds in humans, Arch. Biochem. Biophys., 2018, 646, 1–9 CrossRef CAS PubMed.
- R. P. Feliciano, A. Boeres, L. Massacessi, G. Istas, M. R. Ventura, C. N. Dos Santos, C. Heiss and A. Rodriguez-Mateos, Identification and quantification of novel cranberry-derived plasma and urinary (poly) phenols, Arch. Biochem. Biophys., 2016, 599, 31–41 CrossRef CAS PubMed.
- C. M. Sawicki, D. L. McKay, N. M. McKeown, G. Dallal, C. Y. O. Chen and J. B. Blumberg, Phytochemical pharmacokinetics and bioactivity of oat and barley flour: a randomized crossover trial, Nutrients, 2016, 8, 813 CrossRef PubMed.
- M. S. Lee, F. C. Cheng, H. Z. Yeh, T. Y. Liou and J. H. Liu, Determination of plasma serotonin and 5-hydroxyindoleacetic acid in healthy subjects and cancer patients, Clin. Chem., 2000, 46, 422–423 CrossRef CAS.
- L. Pogačnik, K. Pirc, I. Palmela, M. Skrt, K. S. Kim, D. Brites, M. A. Brito, N. P. Ulrih and R. F. Silva, Potential for brain accessibility and analysis of stability of selected flavonoids in relation to neuroprotection in vitro, Brain Res., 2016, 1651, 17–26 CrossRef PubMed.
- J. D. Sánchez-Martínez, A. R. Garcia, G. Alvarez-Rivera, A. Valdés and M. A. Brito, Cifuentes, A. In Vitro Study of the Blood–Brain Barrier Transport of Natural Compounds Recovered from Agrifood By-Products and Microalgae, Int. J. Mol. Sci., 2022, 24, 533 CrossRef PubMed.
- M. A. Deli, C. S. Ábrahám, Y. Kataoka and M. Niwa, Permeability Studies on In Vitro Blood–Brain Barrier Models: Physiology, Pathology, and Pharmacology, Cell. Mol. Neurobiol., 2005, 25, 59–127 CrossRef PubMed.
- Y. Bu, S. Rho, J. Kim, M. Y. Kim, D. H. Lee, S. Y. Kim, H. Choi and H. Kim, Neuroprotective effect of tyrosol on transient focal cerebral ischemia in rats, Neurosci. Lett., 2007, 414, 218–221 CrossRef CAS PubMed.
- C. St-Laurent Thibault, M. Arseneault, F. Longpre and C. Ramassamy, Tyrosol and hydroxytyrosol two main components of olive oil, protect N2a cells against amyloid-β-induced toxicity. Involvement of the NF- κB signaling, Curr. Alzheimer Res., 2011, 8, 543–551 CrossRef CAS PubMed.
- I. Figueira, L. Tavares, C. Jardim, I. Costa, A. P. Terrasso, A. F. Almeida, C. Govers, J. J. Mes, R. Gardner, J. D. Becker, G. J. McDougall, D. Stewart, A. Filipe, K. S. Kim, D. Brites, C. Brito, M. A. Brito and C. N. Santos, Blood–brain barrier transport and neuroprotective potential of blackberry-digested polyphenols: an in vitro study, Eur. J. Nutr., 2019, 58, 113–130 CrossRef CAS PubMed.
- D. Luque-Córdoba, C. A. Ledesma-Escobar and F. Priego-Capote, Qualitative and quantitative determination of phenols and their metabolites in urine by in-syringe solid-phase extraction and LC–MS/MS analysis for evaluation of virgin olive oil metabolism, Talanta, 2024, 266, 125029 CrossRef PubMed.
- M. Gallardo-Fernández, R. Hornedo-Ortega, I. M. Alonso-Bellido, J. A. Rodríguez-Gómez, A. M. Troncoso, M. C. García-Parrilla, J. L. Venero, A. M. Espinosa-Oliva and R. M. de Pablos, Hydroxytyrosol decreases LPS-and α-synuclein-induced microglial activation in vitro, Antioxidants, 2019, 9, 36 CrossRef PubMed.
- M. González-Santiago, J. Fonollá and E. Lopez-Huertas, Human absorption of a supplement containing purified hydroxytyrosol, a natural antioxidant from olive oil, and evidence for its transient association with low-density lipoproteins, Pharmacol. Res., 2010, 61, 364–370 CrossRef PubMed.
- L. Xie, T. Vance, B. Kim, S. G. Lee, C. Caceres, Y. Wang and B. W. Bolling, Aronia berry polyphenol consumption reduces plasma total and low-density lipoprotein cholesterol in former smokers without lowering biomarkers of inflammation and oxidative stress: A randomized controlled trial, Nutr. Res., 2017, 37, 67–77 CrossRef CAS PubMed.
- W. Fang, R. Zhang, L. Sha, P. Lv, E. Shang, D. Han, J. Wei, X. Geng, Q. Yang and Y. Li, Platelet activating factor induces transient blood–brain barrier opening to facilitate edaravone penetration into the brain, J. Neurochem., 2014, 128, 662–671 CrossRef CAS PubMed.
- Y. Chen, Y. Zhang, J. Tang, F. Liu, Q. Hu, C. Luo, J. Tang, H. Feng and J. H. Zhang, Norrin protected blood–brain barrier via frizzled-4/β-catenin pathway after subarachnoid hemorrhage in rats, Stroke, 2015, 46, 529–536 CrossRef CAS PubMed.
- C. Franco, S. Kausar, M. F. Silva, R. C. Guedes, A. O. Falcao and M. A. Brito, Multi-Targeting Approach in Glioblastoma Using Computer-Assisted Drug Discovery Tools to Overcome the Blood–Brain Barrier and Target EGFR/PI3Kp110β Signaling, Cancers, 2022, 14, 3506 CrossRef CAS PubMed.
- M. Á. Ávila-Gálvez, D. Marques, I. Figueira, K. Cankar, D. Bosch, M. A. Brito and C. N. Dos Santos, Costunolide and parthenolide: Novel blood-brain barrier permeable sesquiterpene lactones to improve barrier tightness, Biomed. Pharmacother., 2023, 167, 115413 CrossRef PubMed.
- F. Mohagheghi, M. R. Bigdeli, B. Rasoulian, A. A. Zeinanloo and A. Khoshbaten, Dietary virgin olive oil reduces blood brain barrier permeability, brain edema, and brain injury in rats subjected to ischemia-reperfusion, Sci. World J., 2010, 10, 1180–1191 CrossRef CAS PubMed.
- L. Mursaleen, B. Noble, S. Somavarapu and M. G. Zariwala, Micellar nanocarriers of hydroxytyrosol are protective against parkinson's related oxidative stress in an in vitro hcmec/d3-sh-sy5y co-culture system, Antioxidants, 2021, 10, 887 CrossRef CAS PubMed.
- L. Fan, Y. Peng and X. Li, Brain regional pharmacokinetics of hydroxytyrosol and its molecular mechanism against depression assessed by multi-omics approaches, Phytomedicine, 2023, 112, 154712 CrossRef CAS PubMed.
- Z. Xi, X. Chen, C. Xu, B. Wang, Z. Zhong, Q. Sun, Y. Sun and L. Bian, Protocatechuic acid attenuates brain edema and blood-brain barrier disruption after intracerebral hemorrhage in mice by promoting Nrf2/HO-1 pathway, NeuroReport, 2020, 31, 1274–1282 CrossRef CAS PubMed.
- F. Fan, L. Yang, R. Li, X. Zou, N. Li, X. Meng, Y. Zhang and X. Wang, Salidroside as a potential neuroprotective agent for ischemic stroke: a review of sources, pharmacokinetics, mechanism and safety, Biomed. Pharmacother., 2020, 129, 110458 CrossRef CAS PubMed.
- K. Radić, B. J. Dukovski and D. Vitali Čepo, Influence of Pomace Matrix and Cyclodextrin Encapsulation on Olive Pomace Polyphenols’ Bioaccessibility and Intestinal Permeability, Nutrients, 2020, 12, 669 CrossRef PubMed.
- R. S. Rogaie, Z. Nosratollah, B. Abolfazl, E. Akram, A. Abolfazl and R. T. Mustafa, Studies of the relationship between structure and antioxidant activity in interesting systems, including tyrosol, hydroxytyrosol derivatives indicated by quantum chemical calculations, Sci. Rep., 2013, 2, 13–18 Search PubMed.
- C. Manna, P. Galletti, G. Maisto, V. Cucciolla, S. D'Angelo and V. Zappia, Transport mechanism and metabolism of olive oil hydroxytyrosol in Caco-2 cells, FEBS Lett., 2000, 470, 341–344 CrossRef CAS PubMed.
- J. L. Lexchin, K. D. Cude-Simpson and H. C. Stancer, Brain and blood indole metabolites after peripheral administration of14C-5-HT
in rat, Neurochem. Res., 1977, 2, 39–50 CrossRef CAS PubMed.
- A. Sharma, M. A. Smith, D. F. Muresanu, P. K. Dey and H. S. Sharma, 5-Hydroxytryptophan: a precursor of serotonin influences regional blood-brain barrier breakdown, cerebral blood flow, brain edema formation, and neuropathology, Int. Rev. Neurobiol., 2019, 146, 1–44 CrossRef CAS PubMed.
- P. Brust, A. Friedrich, I. A. Krizbai, R. Bergmann, F. Roux, V. Ganapathy and B. Johannsen, Functional expression of the serotonin transporter in immortalized rat brain microvessel endothelial cells, J. Neurochem., 2000, 74, 1241–1248 CrossRef CAS PubMed.
- S. Ohtsuki, New aspects of the blood–brain barrier transporters; its physiological roles in the central nervous system, Biol. Pharm. Bull., 2004, 27, 1489–1496 CrossRef CAS PubMed.
- A. Faria, M. Meireles, I. Fernandes, C. Santos-Buelga, S. Gonzalez-Manzano, M. Dueñas, V. de Freitas, N. Mateus and C. Calhau, Flavonoid metabolites transport across a human BBB model, Food Chem., 2014, 149, 190–196 CrossRef CAS PubMed.
Footnote |
† Electronic supplementary information (ESI) available: Supplementary data: Table S1. Chromatographic conditions for each compound under study. See DOI: https://doi.org/10.1039/d3fo04760a |
|
This journal is © The Royal Society of Chemistry 2024 |