DOI:
10.1039/D3FO04106A
(Paper)
Food Funct., 2024,
15, 704-715
Dietary methionine supplementation during the estrous cycle improves follicular development and estrogen synthesis in rats†
Received
6th October 2023
, Accepted 21st November 2023
First published on 18th December 2023
Abstract
The follicle is an important unit for the synthesis of steroid hormones and the oocyte development and maturation in mammals. However, the effect of methionine supply on follicle development and its regulatory mechanism are still unclear. In the present study, we found that dietary methionine supplementation during the estrous cycle significantly increased the number of embryo implantation sites, as well as serum contents of a variety of amino acids and methionine metabolic enzymes in rats. Additionally, methionine supplementation markedly enhanced the expression of rat ovarian neutral amino acid transporters, DNA methyltransferases (DNMTs), and cystathionine gamma-lyase (CSE); meanwhile, it significantly increased the ovarian concentrations of the metabolite S-adenosylmethionine (SAM) and glutathione (GSH). In vitro data showed that methionine supply promotes rat follicle development through enhancing the expression of critical gene growth differentiation factor 9 and bone morphogenetic protein 15. Furthermore, methionine enhanced the relative protein and mRNA expression of critical genes related to estrogen synthesis, ultimately increasing estrogen synthesis in primary ovarian granulosa cells. Taken together, our results suggested that methionine promoted follicular growth and estrogen synthesis in rats during the estrus cycle, which improved embryo implantation during early pregnancy. These findings provided a potential nutritional strategy to improve the reproductive performance of animals.
1. Introduction
The ovary is an important organ for maintaining fertility and overall health in motherhood; its function mainly depends on the maintenance and normal development of follicles.1 During the estrus stage, adequate amino acid nutrition in the follicular fluid is critical for follicular development as amino acids are required for protein synthesis and activation of cellular functions;2 they work with other growth factors and carbohydrates to ensure proliferation and hormone supply of wall granulosa cells during follicular development, which can also provide sufficient conditions for oocyte growth and maturation and ovulation through the transport and processing of cumulus granulosa cells.3–6 Studies have shown that the lack of essential amino acids leads to smaller follicles and poor oocytes,7,8 which results in atresia and reduces the number of oocytes expelled. Therefore, it is important to ensure the supply of amino acids to regulate the development of follicles during estrus and improve the reproductive performance of mammals.
Methionine is an essential amino acid for mammals. It must be supplied in diets to maintain transmethylation and transsulfuration metabolism toward providing enough methyl and sulfhydryl groups, like S-adenosylmethionine (SAM) and glutathione (GSH), among which SAM is the main methyl donor, and the antioxidant GSH plays an important role in alleviating follicular atresia.9 At present, a large number of studies have shown that methionine is essential for embryonic development during pregnancy.10–14 Only a few studies have shown that the addition of methionine to cumulus–oocyte complexes can enhance oocyte protein synthesis and show higher developmental capacity.15,16 Little is known about the function of methionine in hormone synthesis in granulosa cells. Therefore, the regulation and molecular mechanisms of methionine in follicular development and hormone synthesis during estrus still need to be studied.
In this study, we determined whether nutritional regulation by methionine supplementation during the estrous cycle could promote follicular growth and maturation, improve the ovarian steroidogenesis function, and ultimately improve early embryo implantation in rats.
2. Materials and methods
2.1. Animals
All procedures involving animals were approved by the Animal Subjects Committee of China Agricultural University and adhered to the Regulations for the Administration of Affairs Concerning Experimental Animals of the State Council of the People's Republic of China (No. SYXK(
) 2015-0028). Rats were caged in a specific pathogen-free animal room under a controlled environment (temperature set at 25 °C and a lighting schedule of 12 hours light/12 hours dark). Pregnancy was induced by co-caging overnight, and the presence of spermatozoa in the vaginal smear was defined as day 1 of pregnancy.
The effects of dietary methionine supplementation during the estrous cycle on embryo implantation of rats were evaluated. A total of 200 virgin rats (Sprague Dawley rats, body weight 217.31 ± 15.64 g) (Beijing Huafukang Bioscience Co., China) were randomly divided into five groups. After a 7-day adaptation period, the rats were fed with a control diet (according to AIN93, containing 0.4% methionine) and methionine (CJ International Trading Co., Ltd, Shanghai 201107, China) supplemented diets (containing 0.6%, 0.9%, 1.07% and 1.37% methionine) during one estrous cycle, respectively (the nutrient composition of the two diets is shown in Table S1†). Pregnancy was induced by co-caging overnight, and the presence of spermatozoa in the vaginal smear was defined as day 1 of pregnancy. Pregnant rats were fed a normal diet (according to AIN 93, containing 0.4% methionine) until day 7 of pregnancy; then, all rats were anaesthetized by an intraperitoneal injection of 2.0% sodium pentobarbital (30 mg kg−1). Uterine horns were quickly exposed, and the number of implantation sites was recorded. On day 7 of pregnancy, the implantation sites were large and could be counted without magnification. The average daily feed intake and daily weight gain during estrus and pregnancy were also recorded. The optimal supplemental amount of methionine in the diet of rats during the estrous cycle was evaluated using a quadratic curve.
According to the predicted result by the above quadratic curve evaluation, we further evaluated the impacts of the optimal methionine addition amount on rat embryo implantation. A total of 100 mature SD rats (192.16 ± 7.34 g) (Beijing Huafukang Bioscience Co., Ltd, China) were randomly divided into two groups. After a 3-day adaptation, they were fed with a normal diet (0.4% methionine) or optimal methionine diet (0.83% methionine) during one estrous cycle (Table S2†). The next trial procedure was the same as described above. The number of embryo implantation sites was recorded, as well as the average daily feed intake and the average daily weight gain during estrus and early pregnancy.
To further clarify the potential mechanism through which methionine influences oocyte development, a total of 50 mature rats were randomly divided into the following two dietary groups: control diet (0.4% methionine) and 0.83% methionine diet. Dietary treatment was performed for one estrous cycle accurately as before, and 15 rats from each group were randomly selected to be sacrificed. Blood samples were collected from the abdominal vein, stored at room temperature for 2 hours and then centrifuged at 3500g for 10 minutes. The serum was stored at −20 °C until analysis. Ovaries were collected and frozen in liquid nitrogen and stored at −80 °C.
2.2. The staging of the estrous cycle
The estrous cycle in rat averages 4 days and is generally divided into four stages: proestrus, estrus, metestrus and diestrus. Estrous cycle stages were evaluated as described previously.17 Briefly, vaginal epithelial cell samples of rats were collected by vaginal swabbing at 8:00 am daily, and cytomorphological assessment was rapidly performed using optical microscopy. Estrus is characterized by the presence of predominantly anucleated keratinized epithelial cells.
2.3. Primary ovarian granulosa cell culture and treatment
Rat ovarian granulosa cells (rGCs) were collected from 21-day-old female rats injected with 0.1 mL of serum gonadotrophinum for injection (NSHF, batch number: 21106) for 40–44 hours. The ovaries were removed under sterile conditions and washed in PBS enriched with 2% tertiary antibody (penicillin–streptomycin–gentamicin solution, Solarbio, Cat. No.: P1410) three times. Then, these ovaries were placed in DMEM-basic medium (Gibco, Cat. No.: C11995500BT) supplemented with 10% FBS (Sigma-Aldrich, Cat. No.: 12103C) and 4% tertiary antibody on a 37 °C constant-temperature table. A fine needle insulin sterile syringe was used to puncture the follicles on the surface of the ovary, followed by collection of the liquid and centrifugation at 1000 rpm for 5 min to get the rGCs. The cells were resuspended and seeded onto 6-well plates using the aforementioned medium and incubated at 37 °C with 5% CO2. After allowing the cells to adhere for 6 hours, the culture medium was changed, decreasing the tertiary antibody to 2%, and then the culture medium was changed again after 24 hours. After 72 hours, the cells were treated with several doses of methionine (Met 0, 1, 2, and 5 mM) (Sigma-Aldrich) in DMEM-basic medium with 2% tertiary antibody for 24 h in 6-well cell plates. The supernatant was collected for the estrogen and progesterone assay. Then, the cells were collected after washing twice with pre-cooled PBS for RT-PCR and Western blot assay.
2.4. Follicle culture and treatment
Neonatal 12-day-old female rats were sacrificed by cervical dislocation and the ovaries were aseptically removed and briefly incubated in L15 Leibovitz (Gibco, Cat. No.: 11415064) supplemented with 1% FBS and 0.5% penicillin–streptomycin liquid (Solarbio, Cat. No.: P7630). Mechanical dissection of the ovaries was done using fine insulin needles. Follicles were individually encapsulated in 5 μL droplets of sodium alginate-diluted PBS for use, which was made with 0.5 g of sodium alginate (Sigma-Aldrich, Cat. No.: 9005-38-3) and 0.25 g of activated carbon (Sigma-Aldrich, Cat. No.: 7440-44-0) powdered in 50 mL of double distilled water, and stirred overnight. Then, the activated carbon was removed through a 0.22 μM filter before use. The encapsulated follicles were cross-linked in CaCl2 (Sigma-Aldrich, Cat. No.: C7902) solution for 2 min and the gel droplets were transferred into a half αMEM (Gibco, Cat. No.: 32571036) and half Ham's F-12 Nutrient Mixture (Gibco, Cat. No.: 11765054), followed by adding 300 μL of FBS. and placing in an incubator to balance for 30 min. The follicular diameter at the beginning of culture was 140–160 μm. The follicles were individually cultured in wells of 96-well plates (Nuclon, EW-01930-10) containing 100 μL of αMEM supplemented with the same volume of Ham's F-12 Nutrient Mixture, 1% ITS (100×) (Sigma-Aldrich, Cat. No.: I3146), 1 mg mL−1 fetuin (Sigma-Aldrich, Cat. No.: F2379), 3 mg mL−1 BSA (Sigma-Aldrich, Cat. No.: 9048-46-8), and 100 IU mL−1 FSH (Sioux Biochemical, no. 915) with 1 mM, 2 mM, and 5 mM methionine (Met1, Met2, Met5) or without (the control, Met0) methionine (Sigma-Aldrich) in humidified air with 5% CO2 at 37 °C, and half of the medium was changed every 2 days. Photographs were taken to record the state and the diameter of the follicles.
2.5. ELISAs
The serum and ovarian concentrations of enzymes and metabolites involved in the methionine metabolism pathway, DNA methyltransferases (DNMTs), cystathionine beta-synthase (CBS), cystathionine gamma-lyase (CSE) and methionine synthase (MS) were determined using commercially available ELISA Kits (Beijing Dongge Boye Biotechnology Co., Ltd). All samples were analyzed in duplicate in a single assay.
The GSH and SAM concentrations in ovary tissues were measured using a commercially available ELISA Kit (Beijing Dongge Boye Biotechnology Co., Ltd). All samples were analyzed in duplicate in a single assay.
2.6. Chemical analysis
The amino acid content in the diet was determined as follows. Except for sulfur-containing amino acids and tryptophan, the other 15 amino acids in the diet were determined using the German Sykam amino acid analyzer. The specific steps are as follows: weigh about 60–70 mg of the sample and place it at the bottom of the culture tube (the sample should not stick on the bottle wall); add 10 mL of 6 mol L−1 hydrochloric acid solution (add 2 mL for shaking first, fully soak it and then add 8 mL, flushed down along the wall), charge with nitrogen at 0.3 m3 h−1 for about 15 s and seal; hydrolyze at 110 °C for 24 h in an oven, take out, cool, shake, and filter with quantitative filter paper into an ampere tube to avoid contamination between samples; take 0.2 mL of the hydrolyzate into an evaporation tube and evaporate to dryness on an evaporation dryer; add 3 mL of sample diluent, stand for 5 min and then pass it through a 0.2 μm filter membrane, and bottle it for detection on a machine. The sulfur-containing amino acids in the diet were determined as follows: the sample was oxidized with performic acid for 16 h at 4 °C, hydrolyzed with hydrochloric acid for 24 h and analyzed using an amino acid automatic analyzer. The tryptophan content in the diet was determined as follows: the sample was hydrolyzed with 4 mol L−1 sodium hydroxide for 22 h at 110 °C and analyzed by high performance liquid chromatography.
The analysis of serum amino acids was performed by reversed-phase high-performance liquid chromatography (HPLC) as described previously.10 In brief, the amino acids were derivatized into fluorescent products using an automated reaction of 25 μL of the sample with an equal volume of o-phthaldialdehyde (OPA) containing 2 μL mL−1 2-mercaptoethanol. The flow rate through the column was 1.4 mL min−1, and the column temperature was maintained at 25 °C. The two solvents required to generate the elution gradient were methanol and sodium acetate used in 1
:
4 and 4
:
1 (v/v) ratios.
Estradiol (E2), progesterone (P4), and prostaglandin E2 (PGE2) in serum were determined by radioimmunoassays with the E2-17β (HY-10029) RIA Kit, P4 (HY-031) RIA Kit and PGE2 (HY-10047) RIA Kit (Sino-UK Bio) according to the manufacturer's protocols.
2.7. Real-time PCR analysis
Total RNA was extracted from ovaries and rCGs using NucleoZOL reagent (Macherey-Nagel) according to the manufacturer's protocols. Total RNA quality and quantity were determined using a NanoDrop system (ND-1000; Thermo Scientific). Reverse transcription was conducted using the PrimerScript™ RT Reagent Kit (TaKaRa). The specific primers employed for real-time PCR were designed using Primer Premier 6.0 and Oligo 7.0 software, and the sequences of these primers are shown in Table S3†. cDNA was amplified to quantify gene expression via real-time PCR using gene-specific primers and SYBR Green (TaKaRa). Experiments were conducted in duplicate for each sample, and the 2 − ΔΔCt method was used to calculate relative gene expression in different samples, with β-actin as the reference gene.
2.8. Western blot analysis
Cells were washed twice with pre-cooled PBS before being lysed in protease inhibitor (huaxingbio, HX1863) and RIPA buffer (Beyotime, Cat. No.: P0013B) for 10 min. The total protein sample concentration was determined using the Bicinchoninic Acid Kit (Sigma-Aldrich, Cat. No.: BCA1). In this study, 30 μg of each total protein sample was used for western blotting. Then the samples were loaded onto 12% separation gel and 5% spacer gel for SDS-PAGE (sodium dodecyl sulfate polyacrylamide gel electrophoresis). Proteins separated on the gel were transferred to a polyvinylidene fluoride (PVDF, Millipore, IPFL00010) membrane and the membrane was incubated in 5% skim milk powder at room temperature for one hour, followed by overnight incubation with primary antibodies at 4 °C. After three washes with TBS-T (Solarbio, Cat. No.: 1082), the membranes were incubated with anti-horseradish peroxidase (HRP)-labeled sheep anti-rabbit IgG or sheep anti-mouse IgG (1
:
5000, huaxingbio, Cat. No.: HX2032; 1
:
5000, huaxingbio, Cat. No.: HX2031) for 2 h at room temperature.
Protein bands were visualized using an enhanced chemiluminescence detection system (Applen Technologies Inc., P1010). Western blot images were processed using ImageJ software (Wayne Rasband, USA). B-actin was used as the loading control in this study, and the primary antibodies used in this experiment were as follows: rabbit anti-STAR (1
:
1000; CUSABIO, Cat. No.: CSB-PA022798LA01HU), rabbit anti-HSD3b1 (1
:
1000; Bioss, Cat. No.: bs-3906R), rabbit anti-CYP11A1 (1
:
1000; CUSABIO, Cat. No.: CSB-PA006389LA01HU), rabbit anti-CYP19A1 (1
:
1000; CUSABIO, Cat. No.: CSB-PA001927), rabbit anti-GDF9 (1
:
1000; CUSABIO, Cat. No.: CSB-PA005411), rabbit anti-SNAT4 (1
:
1000; CUSABIO, Cat. No.: CSB-PA021619GA01HU), rabbit anti-FSHR(1
:
1000; CUSABIO, Cat. No.: CSB-PA924165), rabbit anti-LHCGR (1
:
1000; Proteintech, Cat. No.: 19968-1-AP), and mouse anti-β-actin (1
:
5000; Immunoway, Cat. No.: YM3028).
2.9. Statistical analysis
Two-tailed Student's t-tests were used for single comparisons and analysis of variance (ANOVA) with LSD tests was used for multiple comparisons. The effect of dietary methionine content on implantation sites was analyzed using linear and quadratic polynomial contrasts. Statistical analysis was performed using SPSS, and graphics were generated with GraphPad Prism 6 software. Data are presented as the mean ± SEM unless stated otherwise. P-Values below 0.05 were considered significant.
3. Results
3.1. Dietary methionine supplementation during the estrous cycle improved embryo implantation in rats
Methionine supplementation during the estrous cycle significantly increased the number of implanted embryos (P < 0.05) (Table 1). Compared with the control group, dietary 0.9% methionine significantly increased the number of implanted embryos (13.63 ± 0.31 versus 14.91 ± 0.36). However, the number of implantation sites gradually reduced when the dietary methionine content was 1.07% or 1.37% (14.21 ± 0.41 and 13.12 ± 0.47) compared with that in the 0.9% methionine group. In addition, dietary methionine supplementation had no effect on maternal feed intake and body weight gain. By quadratic curve analysis, the optimal dietary methionine level at estrus was 0.83% (Fig. 1). Furthermore, it was confirmed that dietary 0.83% methionine significantly increased the number of embryo implantation sites (P < 0.01) (Table 2).
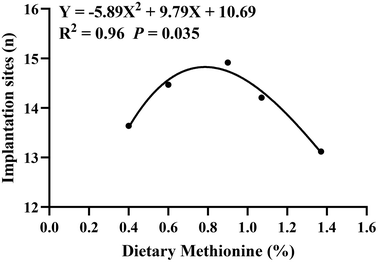 |
| Fig. 1 Effect of dietary methionine supplementation during the estrous cycle on the number of rat embryos implanted. The quadratic curve Y = −5.89X2 + 9.79X + 10.69 was obtained by fitting the dietary methionine content as the horizontal axis and the number of embryos implanted on the seventh day of gestation as the vertical axis. When X = 0.83 (the optimal methionine content), Y had the maximum value of 14.76. The numbers of samples were 22 (0.4% Met), 32 (0.6% Met), 23 (0.9% Met), 29 (1.07% Met) and 25 (1.37% Met), respectively. | |
Table 1 Effect of different dietary methionine supplementation during the estrous cycle on embryo implantation sites of rats
Items |
Control |
Level of dietary methionine (%) |
P value |
0.6% |
0.9% |
1.07% |
1.37% |
Values are means ± SEM. ADFI, average daily feed intake; BW, body weight; ADG, average daily gain. Different superscript lowercase letters within each group indicate significant difference (P < 0.05). Control group: rats were fed with the basal diet for one estrous cycle; treatment group: rats were fed with diets supplemented with different doses of methionine in the basal diet during one estrous cycle. After fertilization, all rats were fed with the same normal diet from day 1 to day 7 in pregnancy. |
Sample (n) |
22 |
32 |
23 |
29 |
25 |
|
Estrous cycle ADFI/BW (g kg−1 d−1) |
77.11 ± 0.78 |
77.03 ± 0.92 |
77.67 ± 0.46 |
78.63 ± 0.84 |
76.62 ± 0.78 |
0.41 |
Estrous cycle ADG (g d−1) |
2.82 ± 0.15 |
3.05 ± 0.16 |
2.76 ± 0.14 |
3.29 ± 0.18 |
2.84 ± 0.18 |
0.14 |
Gestation ADFI/BW (g kg−1 d−1) |
71.02 ± 2.54 |
69.71 ± 1.68 |
70.23 ± 2.59 |
70.42 ± 2.71 |
67.80 ± 3.21 |
0.94 |
Gestation body weight gain (g per 7 day) |
17.67 ± 1.29 |
16.03 ± 1.28 |
17.08 ± 1.58 |
15.67 ± 1.16 |
15.68 ± 1.49 |
0.79 |
Embryo implantation site number (n) |
13.63 ± 0.31ac |
14.47 ± 0.44ab |
14.91 ± 0.36b |
14.21 ± 0.41ab |
13.12 ± 0.47c |
0.035 |
Table 2 Effect of dietary methionine supplementation during the estrous cycle on embryo implantation sites of rats
Items |
0.4% Met |
0.83% Met |
P value |
Values are means ± SEM; 0.4% Met: rats fed a diet containing 0.4% methionine for one estrous cycle; 0.83% Met: rats fed a diet containing 0.83% methionine for one estrous cycle. After pregnancy, all rats were fed the same normal diet from day 1 to day 7 of pregnancy. |
Sample (n) |
39 |
46 |
|
Estrous cycle ADFI/BW (g kg−1 d−1) |
71.25 ± 0.85 |
73.14 ± 0.56 |
0.14 |
Estrous cycle ADG (g d−1) |
2.71 ± 0.28 |
2.87 ± 0.21 |
0.45 |
Gestation ADFI/BW (g kg−1 d−1) |
77.10 ± 1.89 |
72.94 ± 2.07 |
0.27 |
Gestation body weight gain (g per 7 day) |
28.11 ± 2.05 |
25.04 ± 2.26 |
0.51 |
Embryo implantation site number (n) |
12.69 ± 0.26 |
14.15 ± 0.20 |
<0.01 |
3.2. Dietary methionine supplementation alters serum amino acid and hormone compositions and enhances maternal methionine metabolism
Dietary methionine supplementation markedly increased serum concentrations of lysine (Lys), glycine (Gly), serine (Ser), taurine (Tau), alanine (Ala), tryptophan (Trp), proline (Pro) and glutamate (Glu) (Fig. 2A). The main serum methionine metabolic enzymes including DNMTs, CBS and CSE significantly increased after maternal methionine intake (Fig. 2B). To further understand the metabolism of methionine in the ovary, we next detected the ovarian methionine metabolic enzymes and metabolites. As expected, the ovarian DNMT and CSE concentrations also significantly increased (Fig. 2C) and the contents of methionine metabolites SAM and GSH increased (Fig. 3D and E), but they have no changes in serum (Fig. S1†).
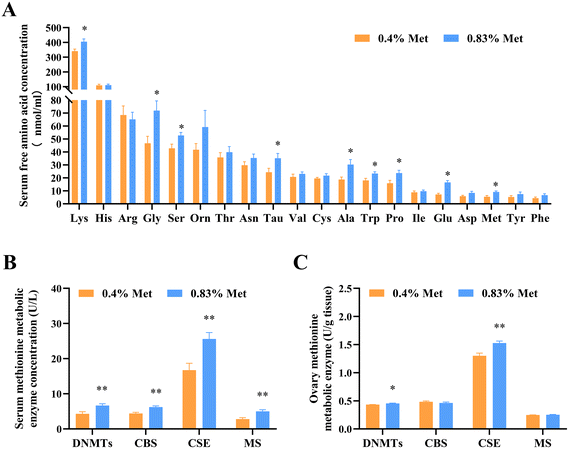 |
| Fig. 2 Feeding a 0.83% methionine diet changed the contents of serum amino acids and the key enzymes involved in the methionine metabolism pathway of rats at estrus. (A) Serum amino acid concentrations in rats fed with 0.4% and 0.83% methionine diets at estrus. (B) Contents of serum methionine metabolic enzymes at estrus. (C) Contents of ovarian methionine metabolic enzymes. ns, P > 0.05; *P < 0.05; **P < 0.0. n = 10. Abbreviations: DNMTs, DNA methyltransferases; CBS, cystathionine beta-synthase; CSE, cystathionine gamma-lyase; and MS, methionine synthase. | |
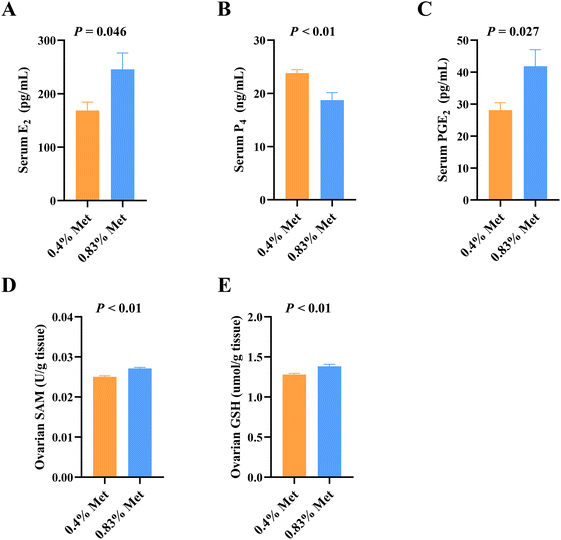 |
| Fig. 3 Methionine levels influenced E2, P4, PGE2, SAM and GSH in serum and ovaries. Serum concentration of (A) E2, (B) P4, and (C) PGE2 in rats during estrus; concentration of ovarian (D) SAM and (E) GSH on estrus in rats. Abbreviations: E2, estradiol; P4, progesterone; PGE2, prostaglandin; SAM, S-adenosylmethionine; and GSH, glutathione. n = 10. | |
In addition, we also detected changes in reproductive hormones related to follicular development in serum. After being fed 0.83% methionine diet, the serum E2 (Fig. 3A) and PGE2 (Fig. 3C) concentrations significantly increased in rats during estrus, while the content of P4 decreased (Fig. 3B).
3.3. Methionine influenced the mRNA expression of amino acid transporters and related methionine metabolic enzymes and levels of metabolites in ovaries
The utilization of amino acids by tissues in vivo is dependent on specific transporters. As expected, the mRNA expression of ovarian methionine transporters including SNAT4, LAT1, LAT2, B0AT and ASC1 significantly increased in rats fed with the 0.83% methionine diet (Fig. 4A).
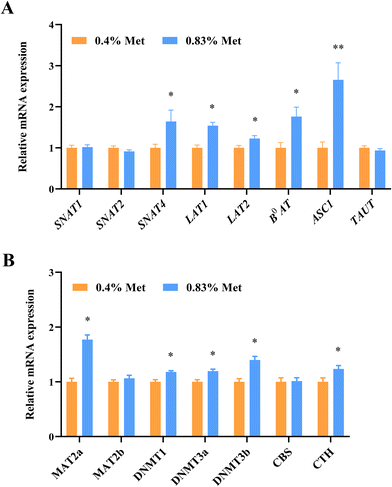 |
| Fig. 4 Dietary methionine supplementation enhanced methionine metabolism in the rat ovary. (A) Relative mRNA expression of the methionine transporter gene in ovaries at estrus. (B) Relative mRNA expression of key enzymes involved in methionine metabolism in ovarian tissues at estrus. ns, P > 0.05; *P < 0.05; **P < 0.01. n = 20. Abbreviations: SNAT1, sodium-coupled neutral amino acid transporter 1; SNAT2, sodium-coupled neutral amino acid transporter 2; SNAT4, sodium-coupled neutral amino acid transporter 4; LAT1, L amino acid transporter 1; LAT2, L amino acid transporter 2; B0AT1, B0 amino acid transporter 1; ASCT1, ASC amino acid transporter 1; TAT1, T amino acid transporter 1; MAT2a, methionine adenosyltransferase 2a; MAT2b, methionine adenosyltransferase 2b; DNMT1, DNA methyltransferases 1; DNMT3a, DNA methyltransferases 3a; DNMT3b, DNA methyltransferases 3b; CBS, cystathionine beta-synthase; and CTH, cystathionine gamma-lyase. | |
To further confirm the relationship between methionine metabolic enzymes and gene expression in ovaries, we examined the changes in gene expression levels of related metabolic enzymes. Consistent with the changes in metabolic enzymes, the relative mRNA expression of DNMT1, DNMT3A, DNMT3B and CTH significantly increased in ovarian tissues of rats fed with the 0.83% methionine diet (Fig. 4B). Surprisingly, the MAT2a expression up-regulated (Fig. 4B).
3.4. Methionine improved ovarian follicle development and estrogen synthesis in primary rGCs
The mRNA expression of critical genes influencing follicular development, including growth-promoting differentiation factor 9 (GDF9) and bone morphogenetic protein 15 (BMP15), significantly enhanced in the ovaries of rats fed with the 0.83% methionine diet (Fig. 5A). Additionally, compared with the control group, 3D culture of rat preantral follicles showed that 1 mM and 2 mM methionine effectively promoted follicle development and increased the follicle diameter and the ratio of antral follicles (Fig. 5B and C). But notably, methionine supplementation at 2 mM and 5 mM had a negative effect on embryonic development compared to 1 mM.
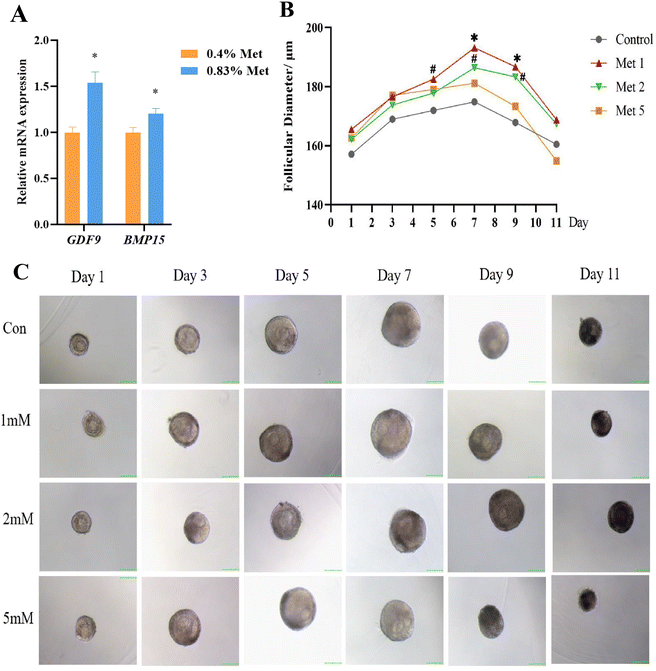 |
| Fig. 5 Methionine-promoted follicle development in vitro. (A) The relative mRNA expression of GDF9 and BMP15 in rat ovaries by feeding with 0.83% and 0.4% methionine diets. n = 20. (B) Growth of follicle diameter in response to methionine treatment at different concentrations. Control: basal medium without methionine addition; Met 1: basal medium supplemented with 1 mM methionine; Met 2: basal medium supplemented with 2 mM methionine; Met 5: basal medium supplemented with 5 mM methionine. Unit: μm. n = 23 (C) Representative images of follicles treated with different concentrations of methionine (basal medium with or without 1 mM\2 mM\5 mM methionine) on days 1, 3, 5, 7, 9 and 11 (scale bar: 100 μm, 10×). *P < 0.05, # 0.05 < P < 0.10. Abbreviations: GDF9, growth differentiation factor 9 and BMP15, bone morphogenetic protein 15. | |
To further elucidate the effects of methionine on follicular development, primary rGCs were isolated and cultured in vitro. The results showed that 2 mM and 5 mM methionine supplementation in the medium markedly increased the relative mRNA expression of neutral amino acid transporter SNAT4, as well as the critical genes for estrogen synthesis, including STAR, CYP11A1, CYP19A1 and HSD3b1, in the primary culture of rGCs (Fig. 6A–D). Meanwhile, methionine also promoted the expression of FSHR and LHCGR in rGCs (Fig. 6B–D), making the response of granulosa cells to FSH and LH more sensitive. Furthermore, the E2 level in the culture medium significantly increased in primary rGCs (Fig. 6E).
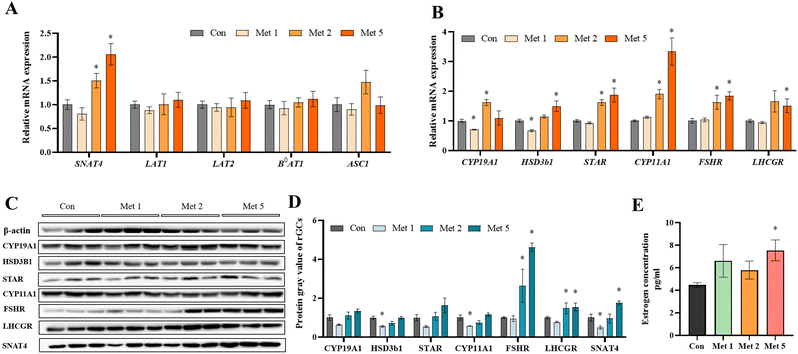 |
| Fig. 6 Estrogen synthesis of rGCs under methionine supplementation. (A) The relative mRNA expression of the methionine transporter in response to methionine treatment at 1 mM, 2 mM, and 5 mM. (B) The relative mRNA expression of various hormone receptors and related estrogen synthesis. (C and D) Western blotting analysis for CYP11A1, CYP19A1, HSD3b1, STAR, FSHR, LHCGR, and SNAT4 in rGCs after methionine supplementation. (E) Estrogen levels of rGCs treated with 1 mM, 2 mM, and 5 mM methionine. Estrogen: pg ml−1. Compared with the control group, *P < 0.05. Abbreviations: SNAT4, sodium-coupled neutral amino acid transporter 4; LAT1, L amino acid transporter 1; LAT2, L amino acid transporter 2; B0AT1, B0 amino acid transporter 1; ASCT1, ASC amino acid transporter 1; CYP19A1, cytochrome P450 family 19 subfamily A member 1; HSD3b1, hydroxy-delta-5-steroid dehydrogenase, 3 beta- and steroid delta-isomerase 1; STAR, steroidogenic acute regulatory protein; CYP11A1, cytochrome P450 family 11 subfamily A member 1; FSHR, follicle-stimulating hormone receptor; LHCGR, luteinizing hormone choriogonadotropin receptor. | |
4. Discussion
The maternal nutritional status affects ovarian and follicular functions, which in turn have profound effects on oocyte and offspring growth and development.18–20 Interestingly, changes in maternal dietary intake will affect the ovulation and oocyte development competence and ultimately have a direct impact on female reproductive outcomes.21–24 Here, we confirmed that 0.83% methionine supplementation during the estrous cycle contributed to follicular development and ovarian estrogen synthesis, which in turn improved embryo implantation during early pregnancy.
Methionine is an essential amino acid in mammals and has many important biological functions, including protein synthesis, methylation reactions, redox maintenance, polyamine synthesis and coupling to folate metabolism.9 Our study found that dietary methionine supplementation during the estrous cycle increased the contents of serum glycine, serine, proline and taurine. Amino acids serve as substrates for the synthesis of proteins, nucleotides and GSH and also play important roles as energy substrates, pH and osmolarity regulators, etc.25,26 It is well known that amino acids in the follicular fluid are derived from the blood circulation,27,28 and their content is affected by changes in blood amino acid composition.29 In a previous study, it was observed that a large amount of glycine is accumulated during oocyte maturation, which serves to regulate the oocyte volume and osmolarity and continues to participate in the regulation of volume changes during early embryonic development.30 Glycine, serine, and proline can alleviate oxidative stress and improve oocyte quality and post-fertilization development ability by promoting the methionine cycle and glutathione synthesis.31–34 Taurine is abundant in mammalian ovaries,35 and an increase in taurine content can promote oocyte maturation and improve oocyte quality.36
Methionine is metabolized through two main pathways, transmethylation and transsulfuration pathways. Methionine participates in DNA methylation in the form of SAM, through transmethylation reactions, which is critical for genome stability and susceptible to nutritional and environmental influences.37 In our study, dietary methionine supplementation increased the contents of SAM and methyltransferases and up-regulated the mRNA expression of DNMT1, DNMT3A and DNMT3B in ovarian tissue. SAM is the only intracellular methyl donor that participates in intracellular methylation processes,38 and the deficiency of a methyl donor leads to reduced methylation levels in oocytes.39,40 Alterations in DNA methylation profiles can alter gene expression profiles, leading to different phenotypes and potentially increased/decreased productivity and disease risk.41,42 Moreover, the absence of methyltransferase leads to abnormal embryonic development and death43 because the methylation of oocytes is gradually acquired during maturation, which is very important for the precise establishment of epigenetics (imprinted genes) in oocytes.44,45 In addition to the transmethylation pathway, methionine can be used to synthesize GSH via the transsulfuration pathway. Our data demonstrated that methionine significantly increased the ovarian GSH content, CSE content and mRNA expression. Ovarian oxidative stress is one of the main factors that reduce oocyte quality,46 while the addition of antioxidants can effectively protect cells from reactive oxygen species and help to improve pregnancy outcomes.47,48 Glutathione is a major intracellular antioxidant that is essential for follicular development and oocyte maturation.49 The lack of glutathione results in reduced oocyte developmental capacity, whereas higher levels of intracellular GSH contribute to improved oocyte quality and early embryonic development.50,51 These data indicated that methionine improved oocyte development by promoting methylation progression and GSH synthesis.
GDF9 and BMP15 are the growth factors secreted by oocytes and are closely associated with oocyte development and maturation.52–56 Our study confirmed that adequate maternal dietary methionine supply during the estrous cycle significantly increased ovarian GDF9 and BMP15 expression. Knockout of GDF9 and BMP15 impairs mammalian follicle and oocyte development and reduces ovulation and fertilization rates.57,58 In contrast, increased GDF9 and BMP15 expression appears to contribute to follicular development and granulosa cell proliferation, increasing the ovulation number and the oocyte fertilization rate.59–63 Furthermore, our in vitro data demonstrated that the addition of 1 mM and 2 mM methionine enhanced follicle diameter increase. This may be responsible for the ultimate increase in the number of implantation sites because oocytes obtained from large follicles had a better developmental capacity.64,65 Notably, consistent with previous studies,10,66 although methionine supplementation was beneficial for the reproductive performance of rats, the use of high doses of methionine negatively affected embryo implantation and follicular development, as demonstrated in our results.
Ovarian steroidogenesis is very important for the development and maturation of follicles and oocytes.1 Our in vivo study showed that methionine supplementation increased the serum levels of E2 and PGE2 and decreased the serum P4 level during estrus. Similarly, an increase in plasma estradiol has been reported to increase the number of ovulations and embryos produced,67,68 but the increase of progesterone concentration inhibits dominant follicle development and ovulation.69 PGE2 promotes cumulus expansion and participates in meiosis and ovulation during oocyte development.70 Increasing PGE2 concentrations can promote the cumulus expansion and oocyte maturation rates in IVM.71 Our in vitro study showed similar results. STAR, CYP11A1, and CYP19A1 are the critical genes for ovarian steroidogenesis.72 Our data showed that methionine supplementation increased the expression of STAR, CYP11A1 and CYP19A1 in rGCs and promoted estradiol synthesis. Simultaneously, it also increased the expression of the follicle stimulating hormone receptor (FSHR) and the luteinizing hormone receptor (LHR) in granulosa cells. These signaling molecules further regulated the expression of many genes involved in the expansion of the cumulus–oocyte complex, steroidogenesis, and immune cell-like functions, which are key events for successful oocyte release and ovulation.73
5. Conclusion
In conclusion, our study revealed that methionine improved ovarian estrogen synthesis and follicle development through modulating the related critical gene expression, ultimately promoting early embryo implantation. Improving our understanding of this field has high clinical and productive significance in natural and assisted reproduction.
Compliance with ethics requirements
All experiments involving animals were conducted in accordance with the ethical policies and procedures approved by the Chinese Guidelines for Animal Welfare and by the Institutional Animal Care and Use Committee of China Agricultural University (CAU20161110-2).
Author contributions
The authors’ contributions were as follows: conceptualization, S. C., F. W. and X. Z.; methodology, G. Y., F. C., F. W. and Q. Y.; formal analysis, investigation and data curation, G. Y., S. L., J. Z. and S. Z.; writing – original draft preparation, G. Y. and S. L.; writing – review and editing, F. C. and S. Z.; supervision, S. C. and S. Z.; funding acquisition, S. C. and X. Z. All authors have read and approved the final version of the manuscript.
Conflicts of interest
There are no conflicts to declare.
Acknowledgements
This work was supported by the National Natural Science Foundation of China (32172747), the China Postdoctoral Science Foundation (2023M733800) and the Beijing Innovation Consortium of Livestock Research System (BAIC05-2023).
References
- A. N. Hirshfield, Development of follicles in the mammalian ovary, Int. Rev. Cytol., 1991, 124, 43–101 CrossRef CAS PubMed.
- A. S. Ambekar, R. S. Nirujogi, S. M. Srikanth, S. Chavan, D. S. Kelkar, I. Hinduja, K. Zaveri, T. K. Prasad, H. Harsha and A. Pandey, Proteomic analysis of human follicular fluid: a new perspective towards understanding folliculogenesis, J. Proteomics, 2013, 87, 68–77 CrossRef CAS PubMed.
- R. Edwards, Follicular fluid, Reproduction, 1974, 37(1), 189–219 CrossRef CAS PubMed.
- M. Beg, D. Bergfelt, K. Kot, M. Wiltbank and O. Ginther, Follicular-fluid factors and granulosa-cell gene expression associated with follicle deviation in cattle, Biol. Reprod., 2001, 64(2), 432–441 CrossRef CAS PubMed.
- Z. L. Clark, D. A. Heath, A. R. O'Connell, J. L. Juengel, K. P. McNatty and J. L. Pitman, The follicular microenvironment in low (++) and high (I+ B+) ovulation rate ewes, Reproduction, 2020, 159(5), 585–599 CAS.
- J. Dupont, R. Scaramuzzi and M. Reverchon, The effect of nutrition and metabolic status on the development of follicles, oocytes and embryos in ruminants, Animal, 2014, 8(7), 1031–1044 CrossRef CAS PubMed.
- N. G. Costermans, K. J. Teerds, A. Middelkoop, B. A. Roelen, E. J. Schoevers, H. T. van Tol, B. Laurenssen, R. E. Koopmanschap, Y. Zhao and M. Blokland, Consequences of negative energy balance on follicular development and oocyte quality in primiparous sows, Biol. Reprod., 2020, 102(2), 388–398 CrossRef CAS PubMed.
- Y. Zhang, L. Liu, T.-L. Yin, J. Yang and C.-L. Xiong, Follicular metabolic changes and effects on oocyte quality in polycystic ovary syndrome patients, Oncotarget, 2017, 8(46), 80472 CrossRef PubMed.
- C. Freitas, A. C. Neto, L. Matos, E. Silva, Â. Ribeiro, J. L. Silva-Carvalho and H. Almeida, Follicular Fluid redox involvement for ovarian follicle growth, J. Ovarian Res., 2017, 10(1), 1–10 CrossRef PubMed.
- S. Cai, Q. Ye, X. Zeng, G. Yang, C. Ye, M. Chen, H. Yu, Y. Wang, G. Wang, S. Huang, S. Quan, X. Zeng and S. Qiao, CBS and MAT2A improve methionine-mediated DNA synthesis through SAMTOR/mTORC1/S6K1/CAD pathway during embryo implantation, Cell Proliferation, 2021, 54(1), e12950 CrossRef CAS PubMed.
- L. J. Van Winkle and R. Ryznar, One-carbon metabolism regulates embryonic stem cell fate through epigenetic DNA and histone modifications: Implications for transgenerational metabolic disorders in adults, Front. Cell Dev. Biol., 2019, 7, 300 CrossRef PubMed.
- D. S. Froese, B. Fowler and M. R. Baumgartner, Vitamin B12, folate, and the methionine remethylation cycle—biochemistry, pathways, and regulation, J. Inherited Metab. Dis., 2019, 42(4), 673–685 CrossRef CAS PubMed.
- S. Tang, Y. Fang, G. Huang, X. Xu, E. Padilla-Banks, W. Fan, Q. Xu, S. M. Sanderson, J. F. Foley and S. Dowdy, Methionine metabolism is essential for SIRT 1−regulated mouse embryonic stem cell maintenance and embryonic development, EMBO J., 2017, 36(21), 3175–3193 CrossRef CAS PubMed.
- S. Ikeda, M. Sugimoto and S. Kume, Importance of methionine metabolism in morula-to-blastocyst transition in bovine preimplantation embryos, J. Reprod. Dev., 2012, 58(1), 91–97 CrossRef CAS PubMed.
- S. Saini, V. Sharma, S. Ansari, A. Kumar, A. Thakur, H. Malik, S. Kumar and D. Malakar, Folate supplementation during oocyte maturation positively impacts the folate-methionine metabolism in pre-implantation embryos, Theriogenology, 2022, 182, 63–70 CrossRef CAS PubMed.
- S. Ikeda, T. Namekawa, M. Sugimoto and S. Kume, i., Expression of methylation pathway enzymes in bovine oocytes and preimplantation embryos, J. Exp. Zool., Part A, 2010, 313(3), 129–136 CrossRef PubMed.
- M. C. Cora, L. Kooistra and G. Travlos, Vaginal Cytology of the Laboratory Rat and Mouse: Review and Criteria for the Staging of the Estrous Cycle Using Stained Vaginal Smears, Toxicol. Pathol., 2015, 43(6), 776–793 CrossRef CAS PubMed.
- M. L. Sutton-McDowall, R. B. Gilchrist and J. G. Thompson, The pivotal role of glucose metabolism in determining oocyte developmental competence, Reproduction, 2010, 139(4), 685–695 CAS.
- Q. Wang, M. M. Chi, T. Schedl and K. H. Moley, An intercellular pathway for glucose transport into mouse oocytes, Am. J. Physiol.: Endocrinol. Metab., 2012, 302(12), E1511–E1518 CrossRef CAS PubMed.
- P. J. McKeegan and R. G. Sturmey, The role of fatty acids in oocyte and early embryo development, Reprod., Fertil. Dev., 2011, 24, 59–67 CrossRef CAS PubMed.
- S. Landau, A. Bor and H. Leibovich,
et al., The effect of ruminal starch degradability in the diet of Booroola crossbred ewes on induced ovulation rate and prolificacy, Anim. Reprod. Sci., 1995, 38(1–2), 97–108 CrossRef.
- E. M. Ferguson, C. J. Ashworth, S. A. Edwards, N. Hawkins, N. Hepburn and M. G. Hunter, Effect of different nutritional regimens before ovulation on plasma concentrations of metabolic and reproductive hormones and oocyte maturation in gilts, Reproduction, 2003, 126(1), 61–71 CAS.
- E. M. Ferguson, J. Slevin, M. G. Hunter, S. A. Edwards and C. J. Ashworth, Beneficial effects of a high fibre diet on oocyte maturity and embryo survival in gilts, Reproduction, 2007, 133(2), 433–439 CAS.
- L. F. Pisani, S. Antonini, P. Pocar, S. Ferrari, T. A. Brevini, S. M. Rhind and F. Gandolfi, Effects of pre-mating nutrition on mRNA levels of developmentally relevant genes in sheep oocytes and granulosa cells, Reproduction, 2008, 136(3), 303–312 CAS.
- R. Dumollard, M. Duchen and J. Carroll, The role of mitochondrial function in the oocyte and embryo, Curr. Top. Dev. Biol., 2007, 77, 21–49 CrossRef CAS PubMed.
- R. G. Sturmey, D. R. Brison and H. J. Leese, Symposium: innovative techniques in human embryo viability assessment. Assessing embryo viability by measurement of amino acid turnover, Reprod. BioMed. Online, 2008, 17(4), 486–496 CrossRef CAS PubMed.
- M. L. Hennet and C. M. Combelles, The antral follicle: a microenvironment for oocyte differentiation, Int. J. Dev. Biol., 2012, 56(10–12), 819–831 CrossRef CAS PubMed.
- A. M. Pelland, H. E. Corbett and J. M. Baltz, Amino Acid transport mechanisms in mouse oocytes during growth and meiotic maturation, Biol. Reprod., 2009, 81(6), 1041–1054 CrossRef CAS PubMed.
- M. Józwik, M. Józwik, C. Teng and F. C. Battaglia, Amino acid, ammonia and urea concentrations in human pre-ovulatory ovarian follicular fluid, Hum. Reprod., 2006, 21(11), 2776–2782 CrossRef PubMed.
- J. M. Baltz and C. Zhou, Cell volume regulation in mammalian oocytes and preimplantation embryos, Mol. Reprod. Dev., 2012, 79(12), 821–831 CrossRef CAS PubMed.
- L. Gao, C. Zhang, Y. Zheng, D. Wu, X. Chen, H. Lan, X. Zheng, H. Wu and S. Li, Glycine regulates lipid peroxidation promoting porcine oocyte maturation and early embryonic development, J. Anim. Sci., 2023, 101, skac425 CrossRef PubMed.
- H. Zhang, T. McClatchie and J. M. Baltz, l-Serine transport in growing and maturing mouse oocytes, J. Cell. Physiol., 2020, 235(11), 8585–8600 CrossRef CAS PubMed.
- S. Li, Q. Guo, Y. M. Wang, Z. Y. Li, J. D. Kang, X. J. Yin and X. Zheng, Glycine treatment enhances developmental potential
of porcine oocytes and early embryos by inhibiting apoptosis, J. Anim. Sci., 2018, 96(6), 2427–2437 CrossRef PubMed.
- N. Liu, X. Si, Y. Ji, Q. Yang, J. Bai, Y. He, H. Jia, Z. Song, J. Chen, L. Yang, S. Zeng, Y. Yang and Z. Wu, l-Proline improves the cytoplasmic maturation of mouse oocyte by regulating glutathione-related redox homeostasis, Theriogenology, 2023, 195, 159–167 CrossRef CAS PubMed.
- M. V. Lobo, F. J. Alonso, A. Latorre and R. M. del Río, Immunohistochemical localization of taurine in the rat ovary, oviduct, and uterus, J. Histochem. Cytochem., 2001, 49(9), 1133–1142 CrossRef CAS PubMed.
- T. Mu, Y. Feng, Y. Che, Q. Lv, J. Hu, Q. Yang and J. Yang, Taurine Promotes In-vitro Follicle Development, Oocyte Maturation, Fertilization and Cleavage of rats, Adv. Exp. Med. Biol., 2019, 1155, 197–203 CrossRef CAS PubMed.
- H. Meng, Y. Cao, J. Qin, X. Song, Q. Zhang, Y. Shi and L. Cao, DNA methylation, its mediators and genome integrity, Int. J. Biol. Sci., 2015, 11(5), 604–617 CrossRef CAS PubMed.
- J. Halušková, B. Holečková and J. Staničová, DNA methylation studies in cattle, J. Appl. Genet., 2021, 62(1), 121–136 CrossRef PubMed.
- E. Anckaert, S. Romero, T. Adriaenssens and J. Smitz, Effects of low methyl donor levels in culture medium during mouse follicle culture on oocyte imprinting establishment, Biol. Reprod., 2010, 83(3), 377–386 CrossRef CAS PubMed.
- Y. H. Zhao, J. J. Wang, P. P. Zhang, H. S. Hao, Y. W. Pang, H. Y. Wang, W. H. Du, S. J. Zhao, W. M. Ruan, H. Y. Zou, T. Hao, H. B. Zhu and X. M. Zhao, Oocyte IVM or vitrification significantly impairs DNA methylation patterns in blastocysts as analysed by single-cell whole-genome methylation sequencing, Reprod., Fertil. Dev., 2020, 32(7), 676–689 CrossRef CAS PubMed.
- S. W. Choi and S. Friso, Epigenetics: A New Bridge between Nutrition and Health, Adv. Nutr., 2010, 1(1), 8–16 CrossRef CAS PubMed.
- H. Jang and C. Serra, Nutrition, epigenetics, and diseases, Clin. Nutr. Res., 2014, 3(1), 1–8 CrossRef PubMed.
- J. Barau, A. Teissandier, N. Zamudio, S. Roy, V. Nalesso, Y. Hérault, F. Guillou and D. Bourc'his, The DNA methyltransferase DNMT3C protects male germ cells from transposon activity, Science, 2016, 354(6314), 909–912 CrossRef CAS PubMed.
- S. A. Smallwood, S. Tomizawa, F. Krueger, N. Ruf, N. Carli, A. Segonds-Pichon, S. Sato, K. Hata, S. R. Andrews and G. Kelsey, Dynamic CpG island methylation landscape in oocytes and preimplantation embryos, Nat. Genet., 2011, 43(8), 811–814 CrossRef CAS PubMed.
- H. Kobayashi, T. Sakurai, M. Imai, N. Takahashi, A. Fukuda, O. Yayoi, S. Sato, K. Nakabayashi, K. Hata, Y. Sotomaru, Y. Suzuki and T. Kono, Contribution of intragenic DNA methylation in mouse gametic DNA methylomes to establish oocyte-specific heritable marks, PLoS Genet., 2012, 8(1), e1002440 CrossRef CAS PubMed.
- A. K. Pandey, A. Gupta, M. Tiwari, S. Prasad, A. N. Pandey, P. K. Yadav, A. Sharma, K. Sahu, S. Asrafuzzaman, D. T. Vengayil, T. G. Shrivastav and S. K. Chaube, Impact of stress on female reproductive health disorders: Possible beneficial effects of shatavari (Asparagus racemosus), Biomed. Pharmacother., 2018, 103, 46–49 CrossRef PubMed.
- N. A. Rocha-Frigoni, B. C. Leão, P. C. Dall'Acqua and G. Z. Mingoti, Improving the cytoplasmic maturation of bovine oocytes matured in vitro with intracellular and/or extracellular antioxidants is not associated with increased rates of embryo development, Theriogenology, 2016, 86(8), 1897–1905 CrossRef CAS PubMed.
- M. Kere, C. Siriboon, N. W. Lo, N. T. Nguyen and J. C. Ju, Ascorbic acid improves the developmental competence of porcine oocytes after parthenogenetic activation and somatic cell nuclear transplantation, J. Reprod. Dev., 2013, 59(1), 78–84 CrossRef CAS PubMed.
- S. Matoba, S. Nakamuta, K. Miura, M. Hirose, H. Shiura, T. Kohda, N. Nakamuta and A. Ogura, Paternal knockout of Slc38a4/SNAT4 causes placental hypoplasia associated with intrauterine growth restriction in mice, Proc. Natl. Acad. Sci. U. S. A., 2019, 116(42), 21047–21053 CrossRef CAS PubMed.
- K. F. Malott, S. Reshel, L. Ortiz and U. Luderer, Glutathione deficiency decreases lipid droplet stores and increases reactive oxygen species in mouse oocytes†, Biol. Reprod., 2022, 106(6), 1218–1231 CrossRef PubMed.
- D. G. de Matos and C. C. Furnus, The importance of having high glutathione (GSH) level after bovine in vitro maturation on embryo development effect of beta-mercaptoethanol, cysteine and cystine, Theriogenology, 2000, 53(3), 761–771 CrossRef CAS PubMed.
- J. R. Silva, R. van den Hurk, H. T. van Tol, B. A. Roelen and J. R. Figueiredo, Expression of growth differentiation factor 9 (GDF9), bone morphogenetic protein 15 (BMP15), and BMP receptors in the ovaries of goats, Mol. Reprod. Dev., 2005, 70(1), 11–19 CrossRef CAS PubMed.
- J. L. Crawford and K. P. McNatty, The ratio of growth differentiation factor 9: bone morphogenetic protein 15 mRNA expression is tightly co-regulated and differs between species over a wide range of ovulation rates, Mol. Cell. Endocrinol., 2012, 348(1), 339–343 CrossRef PubMed.
- C. J. McIntosh, S. Lawrence, P. Smith, J. L. Juengel and K. P. McNatty, Active immunization against the proregions of GDF9 or BMP15 alters ovulation rate and litter size in mice, Reproduction, 2012, 143(2), 195–201 CAS.
- S. Kahraman, C. P. Çetinkaya, M. Çetinkaya, M. A. Tüfekçi, C. G. Ekmekçi and M. Montag, Is there a correlation between follicle size and gene expression in cumulus cells and is gene expression an indicator of embryo development?, Reprod. Biol. Endocrinol., 2018, 16(1), 69 CrossRef PubMed.
- Y. Li, R. Q. Li, S. B. Ou, N. F. Zhang, L. Ren, L. N. Wei, Q. X. Zhang and D. Z. Yang, Increased GDF9 and BMP15 mRNA levels in cumulus granulosa cells correlate with oocyte maturation, fertilization, and embryo quality in humans, Reprod. Biol. Endocrinol., 2014, 12, 81 CrossRef PubMed.
- P. R. Chen, K. Uh, K. Monarch, L. D. Spate, E. D. Reese, R. S. Prather and K. Lee, Inactivation of growth differentiation factor 9 blocks folliculogenesis in pigs†, Biol. Reprod., 2023, 108(4), 611–618 CrossRef PubMed.
- C. Yan, P. Wang, J. DeMayo, F. J. DeMayo, J. A. Elvin, C. Carino, S. V. Prasad, S. S. Skinner, B. S. Dunbar, J. L. Dube, A. J. Celeste and M. M. Matzuk, Synergistic roles of bone morphogenetic protein 15 and growth differentiation factor 9 in ovarian function, Mol. Endocrinol., 2001, 15(6), 854–866 CrossRef CAS PubMed.
- L. Zhao, X. Du, K. Huang, T. Zhang, Z. Teng, W. Niu, C. Wang and G. Xia, Rac1 modulates the formation of primordial follicles by facilitating STAT3-directed Jagged1, GDF9 and BMP15 transcription in mice, Sci. Rep., 2016, 6, 23972 CrossRef CAS PubMed.
- T. H. Huang, F. R. Chen, Y. N. Zhang, S. Q. Chen, F. Y. Long, J. J. Wei, K. Zhang, J. Z. Zeng, Q. Y. Zhu, J. Li-Ling and Y. Gong, Decreased GDF9 and BMP15 in follicle fluid and granulosa cells and outcomes of IVF-ET among young patients with low prognosis, J. Assist. Reprod. Genet., 2023, 40(3), 567–576 CrossRef PubMed.
- K. P. McNatty, P. Smith, L. G. Moore, K. Reader, S. Lun, J. P. Hanrahan, N. P. Groome, M. Laitinen, O. Ritvos and J. L. Juengel, Oocyte-expressed genes affecting ovulation rate, Mol. Cell. Endocrinol., 2005, 234(1–2), 57–66 CrossRef CAS PubMed.
- R. Morikawa, J. Lee and T. Miyano, Effects of oocyte-derived growth factors on the growth of porcine oocytes and oocyte-cumulus cell complexes in vitro, J. Reprod. Dev., 2021, 67(4), 273–281 CrossRef CAS PubMed.
- T. Shimizu, Y. Miyahayashi, M. Yokoo, Y. Hoshino, H. Sasada and E. Sato, Molecular cloning of porcine growth differentiation factor 9 (GDF-9) cDNA and its role in early folliculogenesis: direct ovarian injection of GDF-9 gene fragments promotes early folliculogenesis, Reproduction, 2004, 128(5), 537–543 CAS.
- A. S. Lequarre, C. Vigneron, F. Ribaucour, P. Holm, I. Donnay, R. Dalbiès-Tran, H. Callesen and P. Mermillod, Influence of antral follicle size on oocyte characteristics and embryo development in the bovine, Theriogenology, 2005, 63(3), 841–859 CrossRef PubMed.
- B. S. Shapiro, M. A. Rasouli, K. Verma, A. Raman, F. C. Garner, M. Aguirre, L. Kaye and C. Bedient, The effect of ovarian follicle size on oocyte and embryology outcomes, Fertil. Steril., 2022, 117(6), 1170–1176 CrossRef PubMed.
- V. Cavalcante-Silva, J. Ribeiro da Silva Vallim, L. Fernandes, A. C. de Oliveira and V. D'Almeida, Maternal methionine supplementation in mice affects long-term body weight and locomotor activity of adult female offspring, Br. J. Nutr., 2022, 127(8), 1143–1152 CrossRef CAS PubMed.
- K. Bianco, N. G. Mahutte, A. Arici, D. Sakkas and H. S. Taylor, Effect of estradiol on oocyte development, Int. J. Gynaecol. Obstet., 2009, 104(3), 230–232 CrossRef CAS PubMed.
- J. E. Peña, P. L. Chang, L. K. Chan, K. Zeitoun, M. H. Thornton 2nd and M. V. Sauer, Supraphysiological estradiol levels do not affect oocyte and embryo quality in oocyte donation cycles, Hum. Reprod., 2002, 17(1), 83–87 CrossRef PubMed.
- G. P. Adams, R. L. Matteri and O. J. Ginther, Effect of progesterone on ovarian follicles, emergence of follicular waves and circulating follicle-stimulating hormone in heifers, J. Reprod. Fertil., 1992, 96(2), 627–640 CrossRef CAS PubMed.
- J. D. Niringiyumukiza, H. Cai and W. Xiang, Prostaglandin E2 involvement in mammalian female fertility: ovulation, fertilization, embryo development and early implantation, Reprod. Biol. Endocrinol., 2018, 16(1), 43 CrossRef PubMed.
- D. Boruszewska, I. Kowalczyk-Zieba, K. Suwik, J. Staszkiewicz-Chodor, J. Jaworska, K. Lukaszuk and I. Woclawek-Potocka, Prostaglandin E2 affects in vitro maturation of bovine oocytes, Reprod. Biol. Endocrinol., 2020, 18(1), 40 CrossRef CAS PubMed.
- H. A. Lavoie and S. R. King, Transcriptional regulation of steroidogenic genes: STARD1, CYP11A1 and HSD3B, Exp. Biol. Med., 2009, 234(8), 880–907 CrossRef CAS PubMed.
- P. Kumar and S. F. Sait, Luteinizing hormone and its dilemma in ovulation induction, J. Hum. Reprod. Sci., 2011, 4(1), 2–7 CrossRef CAS PubMed.
|
This journal is © The Royal Society of Chemistry 2024 |
Click here to see how this site uses Cookies. View our privacy policy here.