DOI:
10.1039/D3FO03666A
(Paper)
Food Funct., 2024,
15, 326-337
Effects of (−)-epicatechin on hepatic triglyceride metabolism†
Received
30th August 2023
, Accepted 27th November 2023
First published on 5th December 2023
Abstract
(−)-Epicatechin (EC) consumption is associated with an improvement of hyperlipemia and other metabolic changes linked to obesity and western-style diets. This work investigated the effects of EC on triglyceride (TG) metabolism both in vivo, where mice were supplemented with EC (2 and 20 mg EC per kg body weight), and in vitro, when human HepG2 hepatocytes were incubated in the presence of EC and the main EC metabolites found in human plasma. Increased hepatic TG levels were only observed after 24 weeks supplementation with EC (20 mg per kg body weight), with a preserved liver structure and absence of inflammation or oxidative stress. EC caused increased expression of diacylglycerol acyltransferases (DGAT2), key enzymes in TG synthesis, and the upregulation of PPARα, which promotes free fatty acid (FFA) oxidation. On the other hand, incubation of HepG2 cells in the presence of high concentrations of EC (1–10 μM) did not affect TG deposition nor DGAT2 expression. In summary, in mouse liver, EC upregulated mechanisms that can neutralize the potential toxicity of FFA, i.e. TG synthesis and FFA β-oxidation. Results in mouse liver and HepG2 cells stress the safety of EC in terms of TG metabolism and development of hepatopathies in doses within the limits given by a rational time and dose for human consumption.
1. Introduction
Triglycerides (TG) are the form in which organs store fatty acids.1 Liver, the major organ in the modulation of TG metabolism, synthesizes TG from newly formed fatty acids or from fatty acids of dietary origin. The final step in TG synthesis is catalyzed by the rate-limiting enzymes diacylglycerol acyltransferases (DGAT), which acylate diacylglycerol to triacylglycerol by forming covalent bonds between acyl–CoA and diacylglycerol. These enzymes have major relevance not only in TG synthesis but also in the control of tissue energy metabolism.2 While the liver normally keeps low levels of stored TG, excessive accumulation of TG can cause steatosis and non-alcoholic fatty liver disease (NAFLD). In fact, high levels of TG accumulation in the liver can increase the production of inflammatory mediators and cause oxidative stress, which in turn can lead to liver fibrosis and steatohepatitis.3 On the other hand, TG synthesis is considered a protective mechanism to prevent the hepatotoxicity of free fatty acids (FFA).4–7
Dietary flavonoids are bioactive compounds that are frequently associated with beneficial effects on dyslipidemia. Among flavonoids, the flavan-3-ol (−)-epicatechin (EC) is abundant in many fruits and vegetables including berries, cocoa, and tea. EC consumption mitigates several of the metabolic alterations associated with the consumption of western-style diets and obesity, including hyperlipidemia.8 In this regard, EC supplementation normalizes plasma lipids in rodent models of high fructose and high fat diet consumption.9,10 In rats fed a high fructose diet, EC consumption mitigated the increase in plasma and liver TG.9 Supplementation of EC in male mice for 15 weeks also prevented increases in plasma TG and FFA caused by consumption of a high fat diet.10 These protective effects were observed in all ranges of EC tested (2–20 mg per kg body weight (BW)).11 On the other hand, mice fed a control diet supplemented with 20 mg EC per kg BW for 15 weeks showed either no changes or significantly higher plasma TG concentration.10,11 In addition, mice supplemented with 20 mg EC per kg BW showed a trend for higher NAFLD activity score (NAS) compared to that of controls.11 In healthy humans, consumption of 2000 mg of cocoa flavanols and procyanidins for up to 3 months did not affect plasma TG and cholesterol levels.12
Given the limited information on the potential effects of long-term EC consumption on hepatic TG homeostasis, this study investigated the effects of EC on TG metabolism in both mouse liver and human hepatocyte (HepG2) cell culture models. Only after long-term feeding (24 weeks) of the highest amount of EC (20 mg EC per kg BW) did we observe the upregulation of DGAT2 and PPARα, and TG accumulation in the liver, without evidence of tissue inflammation or altered structure. Findings in human HepG2 hepatocytes in vitro did not show any effect of EC and its main human metabolites on DGAT2 expression and TG accumulation. The present findings stress the safety of EC in terms of TG metabolism and development of hepatopathies in doses administered within the limits of rational human consumption.
2. Materials and methods
2.1. Materials
Antibodies against monocyte chemoattractant protein-1 (MCP-1) (#2029), tumor necrosis factor-α (TNF-α) (#11948), IκB kinase-α (IKKα) (#2682), phospho (Ser176/80)-IKKα/β (p-IKKα/β) (#2697), p65 (#8242), phospho (Ser526)-p65 (p-p65) (#3033), acetyl–CoA carboxylase (ACC) (#3676), adipose triglyceride lipase (ATGL) (#2138), ubiquitin (#3936), c-Jun N-terminal kinase-2 (JNK2) (#9258), β-actin (#12620) and secondary antibodies anti-rabbit HRP-conjugated (#7074) or biotinylated (#14708) were obtained from Cell Signaling Technology (Danvers, MA, USA). Antibodies for F4/80 (sc-25830), nitric oxide synthase 2 (NOS2) (sc-649), phospho (Thr183/Tyr185)-JNK (sc-6254), transforming growth factor 1 beta (TGF1β) (sc-130348), smooth muscle actin (SMA) (sc-53015), fibronectin (sc-271098), collagen type 1 alpha 1 (COL1A1) (sc-293182), phospho (Ser 78/Ser 80)-ACCα (p-ACCα) (sc-271965), fatty acid synthase (FAS) (sc-48357), stearoyl–CoA desaturase 1 (SCD1) (sc-515875), long-chain acyl–CoA synthetase 3 (ACSL3) (sc-166374), ACSL5 (sc-365478), DGAT1 (sc-271934), DGAT2 (sc-293211), sterol-regulatory element binding protein-1C (SREBP-1c) (sc-366), carbohydrate response element binding protein (ChREBP) (sc-515922), peroxisome proliferator-activated receptor alpha (PPARα) (sc-398394), α-tubulin (sc-23948), and agarose A/G beads (sc-2003) were obtained from Santa Cruz Biotechnology (Santa Cruz, CA, USA). The antibody for NADPH oxidase 4 (NOX4) (ab216654) was acquired from Abcam, Inc. (Cambridge, MA, USA). PVDF membranes were obtained from Bio-Rad (Hercules, CA, USA). An enhanced chemiluminescence (ECL) western blotting system was obtained from Bio-Rad Laboratories (Hercules, CA, USA). All other chemicals were purchased from Sigma-Aldrich Co (St. Louis, MO, USA). EC metabolites were a kind gift of Mars Inc. (Hackettstown, NJ, USA).
2.2. Animals and animal care
All procedures were in agreement with standards for the care of laboratory animals as outlined in the NIH Guide for the Care and Use of Laboratory Animals; experimental protocols were approved before implementation by the University of California, Davis Animal Use and Care Administrative Advisory Committee. Procedures were administered under the auspices of the Animal Resource Services of the University of California, Davis.
Healthy 8-week-old male C57BL/6J mice (20–25 g) were purchased from The Jackson Laboratories (Sacramento, CA). After one week of acclimation, mice were randomly divided (15 animals per group) and fed for 24 weeks either: (i) a control diet containing approximately 10% total calories from fat (C) or (ii) the control diet supplemented with 2 (CE2) or 20 mg EC (CE20) per kg BW. The composition of the control diet has been previously described.13 Body weight and food intake were measured weekly. The EC-containing diet was prepared every two weeks to adjust the amount of EC on the basis of the body weight. In addition, all diets were replaced every two days and stored at −20 °C until use to avoid EC degradation. The amounts of EC used in the study were calculated using the Reagan-Shaw et al. scaling criteria14 to relate consumption in humans to that in mice. Based on this calculation, the human equivalent dose of EC for 2 and 20 mg EC per kg BW would be 11 and 110 mg, respectively, for a 70 kg adult. Allometric scaling is an appropriate method to compare bioactive consumption in different species.15 In humans, the equivalent of 2 mg EC per kg BW in mice can be reached by consuming a diet rich in EC,16 while 20 mg EC per kg BW in mice can be reached in humans by supplementation or consumption of selective EC-rich fruits and vegetables and derivatives.17 After 13 and 24 weeks on the dietary treatments, mice were euthanized by cervical dislocation. Blood was collected from the sub-mandibular vein into EDTA tubes, and plasma was obtained after centrifugation at 3000g for 10 min at room temperature. Livers were collected, weighed, and flash-frozen in liquid nitrogen, and then stored at −80 °C until further analysis.
2.3. HepG2 cell culture
HepG2 cells were cultured at 37 °C under an atmosphere of humidified air and 5% (v/v) CO2 in Dulbecco's modified Eagle's medium (DMEM high glucose) supplemented with 10% (v/v) fetal bovine serum (FBS) and antibiotics (100 IU ml−1 penicillin and 10 mg ml−1 streptomycin). The medium was replaced every 2 days until 80% confluency. For the experiments, confluent cells were switched to DMEM low glucose supplemented with 2% (v/v) FBS and antibiotics. Cells were then incubated in the absence or the presence of 1 and 10 μM EC, structurally related (−)-epicatechin metabolites (SREM) and 5-(4′-hydroxyphenil)-γ-valerolactones (PVL) for 1 to 72 h. SREM is a mixture that contains (−)-epicatechin-3′-β-D-glucuronide (40%), (−)-epicatechin-3′-sulfate (30%), and 3′-O-methyl-(−)-epicatechin-5-sulfate (30%), which are the most abundant EC metabolites detected in human plasma after oral EC consumption (1.8 mg EC per kg BW).18 The PVL mixture is composed of the main gut microbiota-generated EC metabolites in humans consisting of 65% and 35% of the sulfate and glucuronide PVL conjugates, respectively.19 The lowest concentration of medium used to incubate cells was based on evidence that consumption of 1.8 mg EC per kg BW, approximately 135 mg EC for a 70 kg individual, leads to a maximum plasma concentration of approximately 1 μM SREM.18
2.4. Cell viability
Cell viability was assessed by the MTT assay, based on the conversion of MTT into formazan crystals by living cells. After the corresponding treatments, HepG2 cells were combined with MTT solution (0.5 mg ml−1 in PBS) and after 45 min of incubation at 37 °C, the reaction was stopped by addition of 0.01 N HCl containing 10% (w/v) SDS. After 2.5 h of incubation, absorbance (λ570–λ690 nm) was measured using a Biotek Synergy H1 plate reader (BioTek Instruments, Winooski, VT) and values expressed as percentage of control (non-treated) cells.
2.5. Determination of liver lipids content
The determination of liver TG content was performed as previously described.20 Briefly, after extraction and saponification, liver and cell homogenates were mixed with a KOH (30% w/v)
:
ethanol (1
:
2 v
:
v) solution and evaporated overnight at 55 °C. The following day, 1 ml of 50% (v/v) ethanol was added, and samples were centrifuged at 10
000g for 5 min at room temperature. To the resulting supernatant was added 1 M MgCl2 and the mixture was incubated on ice for 10 min. After centrifugation at 10
000g for 5 min at room temperature, supernatants were collected and analyzed for TG content using the TG Color GPO/PAP AA colorimetric enzymatic triglyceride kit (Wiener Lab, Rosario, Argentina).
Liver cholesterol content was determined using the Bligh and Dyer method21 with some modifications. Briefly, a 300 μl aliquot of 10% (w/v) liver homogenate was mixed with 900 μl of a chloroform
:
methanol (2
:
1, v
:
v) solution. After centrifugation at 3000g for 10 min, the supernatant was discarded, and the lower phase was collected and evaporated for 1.5 h using an Eppendorf Vacufuge (Eppendorf, Hamburg, Germany). After evaporation, the resulting pellet was resuspended in 50 μl of methanol with 0.05% (v/v) Tween-20 solution and sonicated. 10 μl of the lipid suspension was analyzed for cholesterol content using the Cholestat enzymatic total cholesterol kit (Wiener Lab, Rosario, Argentina).
Liver FFA were extracted and measured following the manufacturer's protocol (Abcam Inc., Cambridge, MA). For the extraction, 10 mg of liver was homogenized in 200 μl of 1% (v/v) Triton X-100 in chloroform, incubated on ice for 20 min and then centrifuged at 14
000g for 5 min at room temperature. The organic phase was collected and first air dried at 50 °C and then dried under vacuum for 30 min. Dried pellets were resuspended in 200 μl of fatty acid assay buffer, and this suspension was vortexed for 5 min. The FFA concentration was determined by fluorometric assay following the manufacturer's instruction.
2.6. Metabolic measurements
Plasma levels of alanine amino-transaminase (ALT), aspartate amino-transaminase (AST), TG, and cholesterol were determined using kits from the Wiener Lab Group (Rosario, Argentina) following the manufacturer's protocols. Determination of plasma FFA was conducted using a fluorometric assay from Abcam Inc. (Cambridge, MA) following the manufacturer's guidelines.
2.7. Histological analyses
Liver samples were fixed overnight in 4% (w/v) neutralized paraformaldehyde solution in PBS. Samples were subsequently washed twice in phosphate buffered saline solution, dehydrated, embedded in paraffin, cut into sections of 5 μm thickness, and placed on glass slides for histological analysis. Hematoxylin and eosin staining was performed following standard procedures to assess liver steatosis. Hepatic histological examination was performed using the NAS described by Liang et al.22 Sections were examined using an Olympus BX51 microscope (Olympus America Inc., Center Valley, PA). Four randomly selected fields per animal were assessed and analyzed using Image J (National Institutes of Health, Bethesda, MD). The researcher was blinded to the treatment groups.
2.8. Protein extraction and western blot analysis
Livers were homogenized as previously described11 using a BeadMill24 homogenizer (Thermo Fisher Scientific Inc., Piscataway, NJ). Aliquots of total homogenates containing 30 μg of protein were denatured with Laemmli buffer, separated by reducing 7–15% polyacrylamide gel electrophoresis, and electroblotted to PVDF membranes. Colored and biotinylated molecular weight standards were run simultaneously. Membranes were blocked for 1.5 h in 5% (w/v) non-fat milk and subsequently incubated in the presence of the corresponding primary antibodies overnight at 4 °C (1
:
750 or 1
:
1000 v/v) in 5% (w/v) BSA in TBS buffer (50 mM Tris, 150 mM NaCl, pH 7.6), containing 0.1% (v/v) Tween-20. After incubation for 90 min at room temperature in the presence of the secondary antibodies (HRP conjugated) (1
:
10
000 v/v), conjugates were visualized using enhanced chemiluminescence. Images were captured using the Bio-Rad ChemiDoc Imager, and bands were quantified using Image Lab software (Bio-Rad, Hercules, CA).
2.9. Immunoprecipitation
To assess the ubiquitination status of DGAT2, tissue homogenates were prepared as described in the previous section with IP buffer (#87787), and 200 μg of proteins were incubated with 0.4 μg of the secondary antibody m-IgGκ BP-HRP (sc-516102) and 15 μl of agarose A/G beads for 30 min at 4 °C. After centrifugation at 500g for 3 min at 4 °C, 45 μl of supernatant was collected and immunoprecipitated with 3 μl of monoclonal mouse anti-DGAT2 antibody on agarose A/G beads overnight at 4 °C. After centrifugation, beads were washed three times with complete lysis buffer and protease inhibitors, the pellet was resuspended in Laemmli sample buffer and PBS and heated at 95 °C for 5 min. Then, lysates and the immunoprecipitated proteins were separated by 7–10% (w/v) SDS-PAGE followed by transfer to PVDF membranes and blotting with antibodies against DGAT2 and ubiquitin. A negative control was used to validate the accuracy of the immunoprecipitation.
2.10. RNA isolation and quantitative PCR (q-PCR)
RNA was extracted from tissues and HepG2 cells using the TRIzol reagent (Invitrogen, Carlsbad, CA) following the manufacturer's instructions. The cDNA was generated using high-capacity cDNA Reverse Transcriptase (Applied Biosystems, Grand Island, NY). Gene expression was assessed by q-PCR (iCycler, Bio-Rad) with the corresponding primers for DGAT1, DGAT2, and PPARα described in ESI Table 1.† Ct values were normalized to the β-actin housekeeping gene. Gene expression was determined using the 2−ΔΔCt method.23
2.11. Statistical analysis
Data were analyzed by one-way analysis of variance (ANOVA) using Statview 5.0 (SAS Institute Inc., Cary, NC). Fisher's least significance difference test was used to examine differences between group means. A P value <0.05 was considered statistically significant. Data are shown as means ± SE.
3. Results
3.1. Effects of 24-week EC supplementation on body weight and plasma metabolic parameters
Supplementation with 2 or 20 mg EC per kg BW for 24 weeks did not affect cumulative food intake and body weight compared to mice consuming non-supplemented diet (Table 1). Plasma lipid levels, including total cholesterol, TG, and FFA, were similar among different groups (Table 1).
Table 1 Animal outcome and metabolic parameters
Parameter |
C |
CE2 |
CE20 |
Animal outcome and metabolic parameters from mice fed for 24 weeks a control diet without (C) or with supplementation with 2 or 20 mg EC per kg BW (CE2, CE20). Values are shown as mean ± SE and are the average of 10 animals per group. Cholesterol, TG and FFA concentrations, and ALT and AST activities were measured in plasma. |
Cumulative food intake (g) |
595 ± 18 |
587 ± 12 |
631 ± 25 |
Body weight (g) |
39.8 ± 0.9 |
37.5 ± 1.0 |
40.0 ± 1.0 |
Liver weight (g) |
1.79 ± 0.13 |
1.75 ± 0.12 |
1.72 ± 0.13 |
Liver weight/BW (mg g−1) |
45.8 ± 1.8 |
45.3 ± 1.3 |
43.7 ± 3.0 |
Total cholesterol (mg dl−1) |
220 ± 8 |
211 ± 9 |
219 ± 9 |
TG (mg dl−1) |
73.1 ± 3.7 |
70.2 ± 3.5 |
73.5 ± 2.5 |
FFA (μM) |
554 ± 30 |
534 ± 52 |
589 ± 37 |
ALT (U l−1) |
30.6± 3.7 |
36.6 ± 5.2 |
37.6 ± 4.6 |
AST (U l−1) |
43.8 ± 5.3 |
48.9 ± 4.4 |
48.2 ± 6.0 |
3.2. Effects of 13- and 24-week EC supplementation on liver lipid deposition
We next evaluated the effects of long-term (24 weeks) EC supplementation on liver lipid content and parameters of liver damage. Liver weight was similar among the different dietary groups (Table 1). Plasma ALT and AST activities, indicators of liver damage, were not affected by EC supplementation (Table 1).
While liver cholesterol and FFA content was similar among all groups (Fig. 1A), liver TG content was significantly higher in mice supplemented with 20 mg EC per kg BW for 24 weeks compared to C and CE2 animals (42% and 44% higher, respectively) (p < 0.05) (Fig. 1A). On the other hand, mice supplemented with 20 mg EC per kg BW for 13 weeks did not show any increase in liver TG content compared to control mice (Fig. 1B).
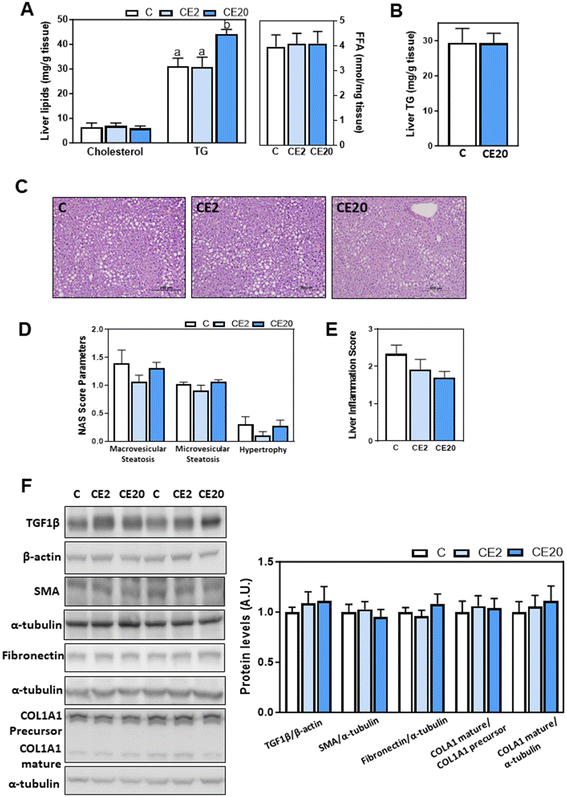 |
| Fig. 1 Effects of long-term EC supplementation on liver lipids, steatosis, and hepatic fibrosis in mice. Mice were fed different diets as described in the Materials and methods section. After 24 weeks on the corresponding diets the following parameters were measured: (A) liver cholesterol, TG, and FFA content were assessed as described in the methods; (C) images of liver hematoxylin and eosin tissue staining; (D and E) NAFLD activity score (NAS) and inflammation scores were evaluated as described in methods; and (F) proteins involved in fibrosis: TGF1β, SMA, fibronectin, and COL1A1 were assessed by western blot and values were normalized to β-actin (TGF1β) or α-tubulin levels (SMA, fibronectin, and COL1A1) as loading controls. Results for CE2 and CE20 were referred to control group values (C). Results are shown as mean ± SE of 10–13 animals per group. Values having different superscripts are significantly different (p < 0.05, one-way ANOVA test). (B) Liver TG accumulations were also measured in mice that were fed for 13 weeks the control diet without EC supplementation (C), and the control diet supplemented with 20 mg EC per kg BW (CE20) as previously described.53 Results are shown as mean ± SE of 6–7 animals per group. Values having different superscripts are significantly different (p < 0.05, one-way ANOVA test). | |
Because of the observed increase in liver TG content in mice supplemented with 20 mg EC per kg BW for 24 weeks, we next conducted a histological tissue characterization using hematoxylin and eosin staining. The NAS value represents the sum of scores for steatosis (microvesicular and macrovesicular steatosis), and hepatocyte injury (hypertrophy). Hematoxylin and eosin staining showed preserved liver structure and similar NAS for all groups (Fig. 1C–E). To assess liver fibrosis, TGF1β, SMA, fibronectin, and COL1A1 protein levels were measured by western blot (Fig. 1F). Supplementation with EC did not affect the protein levels of any of these liver fibrosis markers.
3.3. Effects of 24-week EC supplementation on parameters of liver inflammation and oxidative stress
According to the liver “two hits hypothesis”,24,25 increased liver TG synthesis and accumulation can result in an increased production of inflammatory mediators and oxidative stress. Thus, we next investigated the effects of long-term EC supplementation on redox-sensitive signaling pathways (NF-κB and JNK) and select parameters of inflammation (MCP-1, TNFα, and F4/80) and oxidative stress (NOX4 and NOS2). Supplementation with EC for 24 weeks did not result in the liver activation of the redox-sensitive signals JNK (phosphorylation in Thr183/Tyr185) and NF-κB (IKK phosphorylation in Ser176/Ser80 and p-65 phosphorylation in Ser526) (Fig. 2A). Furthermore, EC did not affect NOS2, NOX4, MCP-1, F4/80, and TNFα liver protein levels (Fig. 2B).
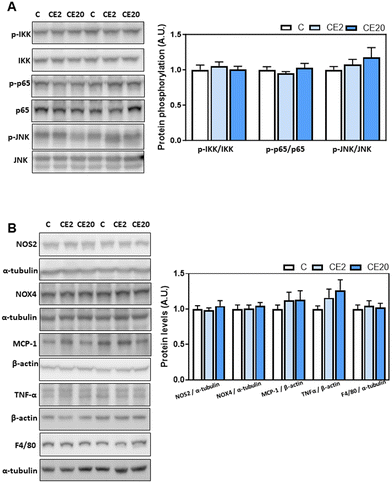 |
| Fig. 2 Effects of long-term EC supplementation on parameters of liver inflammation and oxidative stress in mice. Mice were fed different diets as described in the Materials and methods section. After 24 weeks on the corresponding diets, the following parameters were measured by western blot analysis: (A) activation of NF-κB, i.e. phosphorylation of IKK at Ser176/Ser80 and of p65 at Ser526, and of JNK, measuring its phosphorylation at Thr183/Tyr185; (B) inflammation and oxidative stress, i.e. NOS2, NOX4, MCP-1 and F4/80 protein levels. Bands were quantified and values referred to total protein levels (IKK, p65, and JNK), to α-tubulin (NOS2, NOX4, and F4/80), or to β-actin (MCP-1) levels as loading controls. Results for CE2 and CE20 were referred to control group values (C). Results are shown as mean ± SE of 8–10 animals per group. Values having different superscripts are significantly different (p < 0.05, one-way ANOVA test). | |
3.4. Effects of 24-week EC supplementation on liver enzymes involved in lipid de novo lipogenesis and lipolysis
The observed increase in deposition of TG in the liver upon supplementation with 20 mg EC per kg BW could be due to an increase in de novo lipogenesis (DNL) or decreased TG lipolysis. Thus, we next evaluated the effects of EC supplementation on the levels of proteins involved in these processes, i.e. malonyl–CoA synthesis (ACC), fatty acid synthesis (FAS), fatty acid desaturation (SCD1), fatty acid activation (ACSL3 and ACSL5), and TG synthesis (DGAT1, DGAT2) and lipolysis (ATGL). Phosphorylation of ACCα (Ser78/Ser80) and protein levels of FAS, ACSL3 and ACSL5 were not affected by EC supplementation (Fig. 3A). Regarding DNL, mice supplemented with 20 mg EC per kg BW showed increased SCD1 protein levels compared to C and CE2 mice (Fig. 3A). Furthermore, while 24-week supplementation with 2 mg EC per kg BW did not affect DGAT2 protein and mRNA levels, supplementation with 20 mg EC per kg BW led to higher levels of DGAT2 protein (84%) (Fig. 3A) and mRNA (27%) (Fig. 4A) compared to the C group. EC supplementation with 2 and 20 mg EC per kg BW did not affect DGAT1 protein levels (Fig. 3A). In alignment with no effects on liver TG content (Fig. 1B), supplementation with 20 mg EC per kg BW for a shorter period of time (13 weeks) did not affect SCD1 and DGAT2 protein levels (Fig. 3B).
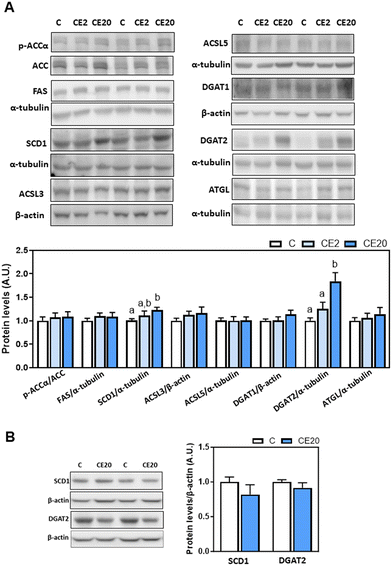 |
| Fig. 3 Effects of long-term EC supplementation on liver enzymes involved in lipid de novo lipogenesis and lipolysis in mice. (A) Mice were fed different diets as described in the Materials and methods section. After 24 weeks on the corresponding diets, the following proteins involved in de novo lipogenesis and lipolysis were measured by western blot analysis: phosphorylation of ACCα at Ser78/Ser80, and protein levels of ACC, FAS, SCD1, ACSL5, DGAT1, DGAT2, and ATGL. Bands were quantified and values referred to total protein levels (ACC), to α-tubulin (FAS, SCD1, ACSL5, DGAT2, and ATGL) or to β-actin (DGAT1) levels as loading controls. Results for CE2 and CE20 were referred to control group values (C). (B) SCD1 and DGAT2 protein levels were also measured, as described above, in mice that were fed for 13 weeks the control diet without EC supplementation (C), and the control diet supplemented with 20 mg EC per kg BW (CE20) as previously described.53 Results are shown as mean ± SE of 8–10 animals per group. Values having different superscripts are significantly different (p < 0.05, one-way ANOVA test). | |
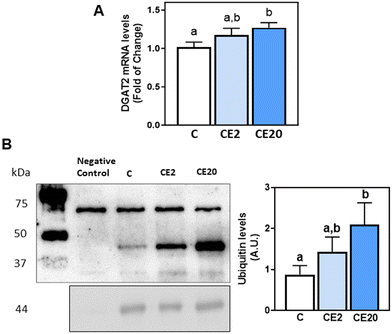 |
| Fig. 4 Effects of long-term EC supplementation on mouse liver DGAT2 mRNA levels and ubiquitination. Mice were fed different diets as described in the Materials and methods section. After 24 weeks on the corresponding diets the following parameters were measured: (A) mRNA levels of liver DGAT2 were assessed by q-PCR. Values were normalized to β-actin (housekeeping gene); (B) DGAT2 ubiquitination was measured after DGAT2 immunoprecipitation from total protein tissue fractions as described in the methods. DGAT2 and its ubiquitination levels were subsequently measured by western blot analysis. Ubiquitinated bands between 37 and 75 kDa were quantified and values referred to those of DGAT2. A sample that underwent the whole procedure of immunoprecipitation in the absence of anti-DGAT2 antibody was used as the negative control. Right panel: values for CE2 and CE20 were referred to the control group value. Results are shown as mean ± SE of 8–10 animals per group. Values having different superscripts are significantly different (p < 0.05, one-way ANOVA test). | |
3.5. Effects of 24-week EC supplementation on transcription factors involved in DNL and lipolysis
We next investigated the modulation of transcription factors that are involved in TG and fatty acid synthesis (SREBP-1c, ChREBP) and fatty acid β-oxidation (PPARα). Liver protein levels of SREBP1-c (precursor and mature forms) and ChREBP were similar among different groups, as evaluated by western blot assay (Fig. 5A). On the other hand, PPARα mRNA levels were higher in the CE20 group compared to C, while PPARα protein levels were significantly higher in CE2 compared to C mice (Fig. 5B and C).
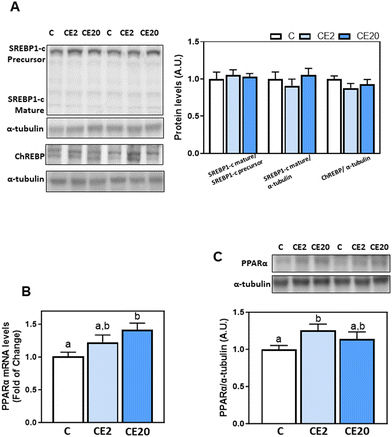 |
| Fig. 5 Effects of long-term EC supplementation on transcription factors involved in liver de novo lipogenesis and lipid β-oxidation in mice. Mice were fed different diets as described in the Materials and methods section. After 24 weeks on the corresponding diets the following parameters were measured: (A) master transcription factors involved in de novo lipogenesis regulation, SREBP1-c and ChREBP protein levels were measured by western blot analysis; (B and C) transcription factors involved in lipid β-oxidation, PPARα gene expression and protein levels were measured by q-PCR and western blot analyses, respectively. Bands were quantified and values referred to SREBP1-c precursor (SREBP1-c mature) or α-tubulin (SREBP1-c mature, ChREBP, and PPARα) (loading controls). mRNA levels of PPARα were normalized to β-actin (housekeeping gene). Results for CE2 and CE20 were referred to control group values (C). Results are shown as mean ± SE of 8–10 animals per group. Values having different superscripts are significantly different (p < 0.05, one-way ANOVA test). | |
3.6. Effects of 24-week EC supplementation on DGAT2 post-translational regulation
DGAT2 is post-translationally regulated via degradation through the endoplasmic reticulum-associated degradation (ERAD) pathway. To investigate whether the increased protein levels of DGAT2 associated with EC supplementation were mediated through an impairment of DGAT2 degradation, we next evaluated DGAT2 ubiquitination levels. DGAT2 was immunoprecipitated from liver homogenates and its ubiquitination levels were subsequently assessed by western blot assay. Supplementation with 20 mg EC per kg BW led to higher levels of DGAT2 ubiquitination compared to the C group (Fig. 4B).
3.7. Effects of EC and SREM on TG content and DGAT1 and DGAT2 mRNA levels in HepG2 cells
We next investigated the potential effects of EC and its metabolites that appear in human plasma after EC oral consumption, i.e. the main structurally related EC metabolites (SREM) (−)-epicatechin-3′-β-D-glucuronide, (−)-epicatechin-3′-sulfate, and 3′-O-methyl-(−)-epicatechin-5-sulfate at a ratio and concentration similar to that found in human plasma, and the main microbiota metabolite phenyl-γ-valerolactone (PVL), on TG content and DGAT1 and DGAT2 mRNA levels in human HepG2 cells. Human HepG2 cell cultures have demonstrated their reliability as valuable systems for studying TG metabolism in hepatocytes. We initially assessed the effects of EC, SREM, and PVL on HepG2 cell viability using the MTT assay (ESI Fig. 1A and B†). HepG2 cell incubation with 1 or 10 μM EC for 24, 48, and 72 h did not affect cell viability. Treatment of HepG2 cells for up to 72 h with 1 and 10 μM EC did not affect cell TG content (Fig. 6A). Incubation of HepG2 cells with 1 or 10 μM EC, SREM, and PVL for up to 24 h did not affect DGAT2 or DGAT1 mRNA levels (Fig. 6B, C and 7A, B).
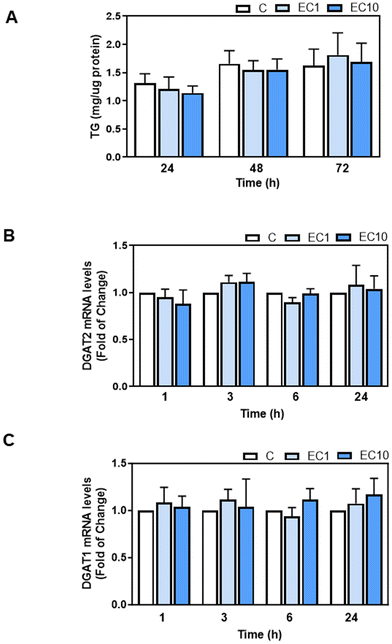 |
| Fig. 6 Effects of EC on TG content and DGAT2 and DGAT1 gene expression in HepG2 cells. HepG2 cells were incubated in the absence or the presence of 1 and 10 μM EC (EC1 and EC10, respectively) for 1–72 h as indicated in each corresponding graph. (A) TG contents were measured as described in the Materials and methods section and values were normalized to total protein concentrations. (B and C) mRNA levels of DGAT2 and DGAT1 were assessed in HepG2 cells by q-PCR. Values were normalized to β-actin (housekeeping gene). Results for EC1 and EC10 were referred to control values (C) (non-added cells). Results are shown as mean ± SE of 10 (for TG) and 3–4 (for q-PCR) independent experiments. Values having different superscripts are significantly different (p < 0.05, one-way ANOVA test). | |
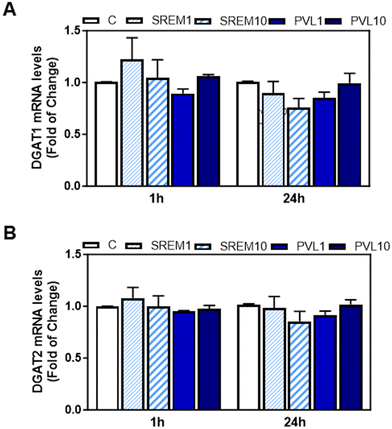 |
| Fig. 7 Effects of SREM and PVL on DGAT1 and DGAT2 gene expression in HepG2 cells. HepG2 cells were incubated in the absence or the presence of 1 and 10 μM SREM (SREM 1 and SREM10, respectively) or PVL (PVL1 and PVL10, respectively) for 1 or 24 h as described in the Materials and methods section. (A and B) mRNA levels of DGAT1 and DGAT2 were measured by q-PCR. Values were normalized to β-actin (housekeeping gene). Results for SREM and PVL were referred to control values (C) (non-added cells). Results are shown as mean ± SE of 3–4 independent experiments. Values having different superscripts are significantly different (p < 0.05, one-way ANOVA test). | |
4. Discussion
Only long-term (24 weeks) consumption of EC at the highest dose (20 mg per kg BW) resulted in the upregulation of liver DGAT2 and PPARα, and increased TG deposition. On the other hand, incubation of human HepG2 cells with high concentrations of EC and the EC human plasma metabolites did not affect cell TG levels and/or DGAT2 gene expression. While results support the safety of rational EC supplementation in humans, current results point to the need for understanding potential negative effects of long-term supplementation and pharmacological doses of polyphenols on human health.
Consumption of EC or EC-rich foods has been linked to improvements in plasma and hepatic lipids.8 Consumption of enriched bread containing 0.05% of a 1
:
1 mixture of EC and quercetin for three months significantly decreased plasma total cholesterol, LDL-cholesterol, TG, and fasting glucose levels in humans who had at least three of the risk factors for metabolic syndrome.26 Daily supplementation with 50 mg of EC for four weeks resulted in a decrease (75 mg dl−1) of plasma TG levels in subjects with hypertriglyceridemia.27 Additionally, the daily consumption of 993 mg of cocoa flavanols for 8 weeks significantly reduced circulating levels of TG, total cholesterol, and LDL-cholesterol in elderly individuals.28 In fact, a meta-analysis of randomized controlled trials found that consumption of chocolate or cocoa products significantly reduces TG levels.29 On the other hand, both acute (2 h) and chronic (4 weeks) consumption of isolated apple monomeric flavanols, primarily EC, by humans at doses of 70 mg or 140 mg, did not exhibit significant impact on blood lipids, including TG and total cholesterol.30 In mice, supplementation for 15 weeks with 20 mg EC per kg BW mitigated high fat diet-induced increases in plasma TG concentrations, but did not prevent increases in FFA, and total cholesterol.10 EC (20 mg per kg BW) also mitigated increases in plasma and liver TG concentrations caused by consumption of a high fructose diet in rats.9 Administration of 1 mg EC per kg BW twice daily for 2 weeks to male offspring of obese rats reduced their serum TG levels.31 Overall, findings show that EC consumption decreases circulating and liver TG in humans and/or rodents. In this study, we found that a longer term (24 weeks) consumption of EC (20 mg per kg BW) increased TG deposition in the liver.
EC-mediated liver TG increase was associated with elevated protein levels of DGAT2 in mouse liver, while DGAT1 was not affected. DGAT2 is responsible for catalyzing the final and limiting step in TG biosynthesis. DGAT2 possesses two transmembrane domains, and its active site is oriented towards the cytosol. This positioning suggests that DGAT2 plays a role in catalyzing the synthesis of TG specifically intended for storage within cytosolic lipid droplets.32 In contrast, DGAT1 exhibits three transmembrane domains, and its active site is situated in the lumen of the endoplasmic reticulum, which directs TG towards nascent lipoproteins.33 The regulation of DGAT2 involves not only transcriptional control but also protein stability,34 in particular degradation. Findings that EC supplementation increased DGAT2 protein ubiquitination, an indication of protein degradation, and increased DGAT2 mRNA and protein levels, suggest that EC could be modulating DGAT2 at the level of transcription, not post-translationally. Besides TG synthesis, liver TG levels are regulated by lipolysis. The central enzyme in TG degradation is ATGL. Findings that ATGL levels in the liver were not affected by EC supplementation supports an effect at the level of TG synthesis, not degradation.
Importantly, despite the increased TG deposition in the liver associated with long-term high dosage of EC supplementation in mice, there was no associated inflammation or evidence of fibrosis. In fact, studies show that TG accumulation per se is not inherently hepatotoxic and can actually serve as a defense mechanism to counterbalance excess FFA availability.4,5,35,36 Liver DGAT2, the expression of which was increased by EC supplementation, plays a crucial role in safeguarding the endoplasmic reticulum against the lipotoxic effects induced by high fat diets.37 Additionally, decreased DGAT2 expression reduces hepatic TG, which subsequently increases FFAβ-oxidation and exacerbates steatohepatitis in mice.4
In terms of mechanism of action, EC and cocoa polyphenols can regulate the expression of transcription factors involved in homeostasis of TG and FFA, including PPARγ,38 PPARα, ChREBP and SREBPs.39 PPARα is recognized for its central role in modulating FFA homeostasis in liver, heart, and brown adipose tissue,40 while also exerting anti-inflammatory properties.41 PPARα regulates FFA and TG metabolism through its direct effects on fatty acid transport and β-oxidation genes and its induction of lipolysis, respectively.40 The transcriptional regulation of fatty acid synthesis, elongation, and desaturation involves SREBP-1c and ChREBP.42 In terms of fatty acid synthesis, both ChREBP and SREBP-1c stimulate the expression of ACC and FAS.42–46 We currently observed that chronic EC supplementation did not affect SREBP-1c and ChREBP protein levels, but significantly increased PPARα gene and protein levels in mouse liver. These findings suggest potentially positive effects of EC in regulating lipogenesis and/or fatty acid β-oxidation, and subsequently attenuating the accumulation of lipids in the liver. In support of this, daily administration of 1 mg EC per kg BW47 or 10–40 mg EC per kg BW prevented the upregulation of SREBP-1c in the liver of rats fed a high fat diet.39
There are notable differences in the metabolism of EC among various species, such as those observed between mice and humans.48 There is no 3′-O-methyl-EC-7-sulfate or EC-3′-O-β-D-glucuronide appearing in mouse plasma upon EC consumption. Among the four major SREMs identified in humans, only two, EC-3′-sulfate and 3′-O-methyl-EC-5-sulfate, were found in mice. In addition, the conjugation of PVL is different in rodents and in humans.19 As an initial step in understanding a potential negative effect of EC supplementation in humans on TG homeostasis in the liver, we evaluated the effects of the main human EC metabolites on DGAT2/1 expression and/or TG accumulation. Evidence that exposure to very high levels of EC and its metabolites did not affect these parameters is relevant initial evidence suggesting that TG accumulation in the liver observed in mice does not seem to occur in an in vitro model of human hepatocytes.
Flavan-3-ols have been recognized for their numerous beneficial properties.49–51 Nevertheless, it should be noted that they can also exhibit toxicity effects when consumed as pure compounds, at high doses and during long periods of time. While the safety implications of supplementing diets with high doses of EC in humans have not been thoroughly studied, current studies have not found adverse effects. The COcoa Supplement and Multivitamin Outcomes Study (COSMOS), which is the most extensive trial to date (with a median duration of 3.6 years) investigating the effects of long-term cocoa extract supplementation on clinical events in older individuals in the United States, revealed no safety issues related to cocoa flavanol supplementation (500 mg flavanols per day, including 80 mg of EC).52 Another human study showed that consumption of up to 2000 mg per day of cocoa flavanols for a duration of 12 weeks was well tolerated.12 It should be noted that the specific amount of EC provided in that study is unknown as it primarily represented the intake of flavanol monomers. The inclusion of the evaluation of parameters of liver damage in future clinical studies involving chronic supplementation with pure EC or EC-rich foods will help to rule out any potentially damaging accumulation of TG in the liver.
In summary, chronic EC consumption promotes changes in liver lipid homeostasis. An elevation in hepatic TG content in mice was associated with an upregulation of DGAT2. While excess TG deposition may have negative consequences in the long-term, the upregulation of DGAT2 can protect the tissue from lipotoxicity by incorporating FFA into TG. Additionally, EC upregulated PPARα, which plays a major role in the regulation of fatty acid β-oxidation. In vitro experiments in human HepG2 cells did not replicate the effects of EC on TG accumulation in mouse liver. Considering the limited available literature, our study provides valuable initial insights for future research on the effects of EC on TG metabolism. Results also suggest that while consumption of EC-rich foods is safe, supplementation with large doses of pure EC for long periods of time should be avoided as potential liver toxicity should be considered.
Abbreviations
ACC | Acetyl-CoA carboxylase |
ACSL | Acyl-CoA synthetase long-chain |
ALT | Alanine amino-transaminase |
AST | Aspartate amino-transaminase |
ATGL | Adipose triglyceride lipase |
ChREBP | Carbohydrate response element binding protein |
COL1A1 | Collagen type 1 alpha 1 |
DGAT | Diacylglycerol acyltransferases |
EC | (−)-Epicatechin |
FAS | Fatty acid synthase |
PVL | Phenyl-γ-valerolactone |
IKK | IκB kinase |
JNK | cc-Jun N-terminal kinase |
MCP-1 | Monocyte chemoattractant protein-1 |
NAFLD | Non-alcoholic fatty liver disease |
NAS | NAFLD activity score |
NF-κB | Nuclear factor κB |
NOS | Nitric oxide synthase |
NOX | NADPH oxidase |
PPARα | Peroxisome proliferator-activated receptor α |
SCD1 | Stearoyl-CoA desaturase 1 |
SMA | Smooth muscle actin |
SREBP-1c | Sterol-regulatory element binding protein-1c |
SREM | Structure related (−)-epicatechin metabolites |
TG | Triglycerides |
TGF1β | Transforming growth factor 1 β |
TNF-α | Tumor necrosis factor-α |
Conflicts of interest
There are no conflicts to declare.
Acknowledgements
This work was supported by H. E. Jastro awards to ZM and grants from NIFA USDA (CA-D-NTR-7244-H and CA-D-NTR-2819-H) to PO. PO is a correspondent researcher from CONICET, Argentina. The graphical abstract was generated with BioRender.com.
References
- M. Alves-Bezerra and D. E. Cohen, Triglyceride Metabolism in the Liver, Compr. Physiol., 2017, 8, 1–8 Search PubMed.
- Y. Shi and D. Cheng, Beyond triglyceride synthesis: the dynamic functional roles of MGAT and DGAT enzymes in energy metabolism, Am. J. Physiol.: Endocrinol. Metab., 2009, 297, E10–E18 CrossRef CAS PubMed.
- E. Buzzetti, M. Pinzani and E. A. Tsochatzis, The multiple-hit pathogenesis of non-alcoholic fatty liver disease (NAFLD), Metabolism, 2016, 65, 1038–1048 CrossRef CAS PubMed.
- K. Yamaguchi, L. Yang, S. McCall, J. Huang, X. X. Yu, S. K. Pandey, S. Bhanot, B. P. Monia, Y. X. Li and A. M. Diehl, Inhibiting triglyceride synthesis improves hepatic steatosis but exacerbates liver damage and fibrosis in obese mice with nonalcoholic steatohepatitis, Hepatology, 2007, 45, 1366–1374 CrossRef CAS PubMed.
- C. Chitraju, N. Mejhert, J. T. Haas, L. G. Diaz-Ramirez, C. A. Grueter, J. E. Imbriglio, S. Pinto, S. K. Koliwad, T. C. Walther and R. V. Farese Jr., Triglyceride Synthesis by DGAT1 Protects Adipocytes from Lipid-Induced ER Stress during Lipolysis, Cell Metab., 2017, 26, 407–418 CrossRef CAS PubMed.
- J. Liu, L. Han, L. Zhu and Y. Yu, Free fatty acids, not triglycerides, are associated with non-alcoholic liver injury progression in high fat diet induced obese rats, Lipids Health Dis., 2016, 15, 27 CrossRef PubMed.
- M. Li, C. Xu, J. Shi, J. Ding, X. Wan, D. Chen, J. Gao, C. Li, J. Zhang, Y. Lin, Z. Tu, X. Kong, Y. Li and C. Yu, Fatty acids promote fatty liver disease via the dysregulation of 3-mercaptopyruvate sulfurtransferase/hydrogen sulfide pathway, Gut, 2018, 67, 2169–2180 CrossRef CAS PubMed.
- E. Cremonini, D. E. Iglesias, J. Kang, G. E. Lombardo, Z. Mostofinejad, Z. Wang, W. Zhu and P. I. Oteiza, (-)-Epicatechin and the comorbidities of obesity, Arch. Biochem. Biophys., 2020, 690, 108505 CrossRef CAS PubMed.
- A. Bettaieb, M. A. Vazquez Prieto, C. Rodriguez Lanzi, R. M. Miatello, F. G. Haj, C. G. Fraga and P. I. Oteiza, (-)-Epicatechin mitigates high-fructose-associated insulin resistance by modulating redox signaling and endoplasmic reticulum stress, Free Radicals Biol. Med., 2014, 72, 247–256 CrossRef CAS PubMed.
- E. Cremonini, A. Bettaieb, F. G. Haj, C. G. Fraga and P. I. Oteiza, (-)-Epicatechin improves insulin sensitivity in high fat diet-fed mice, Arch. Biochem. Biophys., 2016, 599, 13–21 CrossRef CAS PubMed.
- E. Cremonini, Z. Wang, A. Bettaieb, A. M. Adamo, E. Daveri, D. A. Mills, K. M. Kalanetra, F. G. Haj, S. Karakas and P. I. Oteiza, (-)-Epicatechin protects the intestinal barrier from high fat diet-induced permeabilization: Implications for steatosis and insulin resistance, Redox Biol., 2018, 14, 588–599 CrossRef CAS PubMed.
- J. I. Ottaviani, M. Balz, J. Kimball, J. L. Ensunsa, R. Fong, T. Y. Momma, C. Kwik-Uribe, H. Schroeter and C. L. Keen, Safety and efficacy of cocoa flavanol intake in healthy adults: a randomized, controlled, double-masked trial, Am. J. Clin. Nutr., 2015, 102, 1425–1435 CrossRef CAS PubMed.
- E. Cremonini, E. Daveri, A. Mastaloudis and P. I. Oteiza, (-)-Epicatechin and Anthocyanins Modulate GLP-1 Metabolism: Evidence from C57BL/6J Mice and GLUTag Cells, J. Nutr., 2021, 151, 1497–1506 CrossRef PubMed.
- S. Reagan-Shaw, M. Nihal and N. Ahmad, Dose translation from animal to human studies revisited, FASEB J., 2008, 22, 659–661 CrossRef CAS PubMed.
- R. Rucker, Allometric Scaling: Comparison of Interspecies Nutritional Relationships and Requirements, J. Nutr., 2022, 152, 2626–2627 CrossRef PubMed.
- A. Vogiatzoglou, A. A. Mulligan, M. A. Lentjes, R. N. Luben, J. P. Spencer, H. Schroeter, K. T. Khaw and G. G. Kuhnle, Flavonoid intake in European adults (18 to 64 years), PLoS One, 2015, 10, e0128132 CrossRef PubMed.
- J. M. Harnly, R. F. Doherty, G. R. Beecher, J. M. Holden, D. B. Haytowitz, S. Bhagwat and S. Gebhardt, Flavonoid content of U.S. fruits, vegetables, and nuts, J. Agric. Food Chem., 2006, 54, 9966–9977 CrossRef CAS PubMed.
- J. I. Ottaviani, T. Y. Momma, G. K. Kuhnle, C. L. Keen and H. Schroeter, Structurally related (-)-epicatechin metabolites in humans: Assessment using de novo chemically synthesized authentic standards, Free Radicals Biol. Med., 2011, 52, 1403–1412 CrossRef PubMed.
- G. Borges, J. I. Ottaviani, J. J. J. van der Hooft, H. Schroeter and A. Crozier, Absorption, metabolism, distribution and excretion of (-)-epicatechin: A review of recent findings, Mol. Aspects Med., 2018, 61, 18–30 CrossRef CAS PubMed.
- E. Daveri, E. Cremonini, A. Mastaloudis, S. N. Hester, S. M. Wood, A. L. Waterhouse, M. Anderson, C. G. Fraga and P. I. Oteiza, Cyanidin and delphinidin modulate inflammation and altered redox signaling improving insulin resistance in high fat-fed mice, Redox Biol., 2018, 18, 16–24 CrossRef CAS PubMed.
-
A. Sündermann, L. F. Eggers and D. Schwudke, in Encyclopedia of Lipidomics, ed. M. R. Wenk, Springer Netherlands, Dordrecht, 2016, pp. 1–4, DOI:10.1007/978-94-007-7864-1_88-1.
- W. Liang, A. L. Menke, A. Driessen, G. H. Koek, J. H. Lindeman, R. Stoop, L. M. Havekes, R. Kleemann and A. M. van den Hoek, Establishment of a general NAFLD scoring system for rodent models and comparison to human liver pathology, PLoS One, 2014, 9, e115922 CrossRef PubMed.
- X. Rao, X. Huang, Z. Zhou and X. Lin, An improvement of the 2⁁(-delta delta CT) method for quantitative real-time polymerase chain reaction data analysis, Biostat. Bioinf. Biomath., 2013, 3, 71–85 Search PubMed.
- Z. Wang, Z. Li, Y. Ye, L. Xie and W. Li, Oxidative Stress and Liver Cancer: Etiology and Therapeutic Targets, Oxid. Med. Cell. Longevity, 2016, 2016, 7891574 Search PubMed.
- J. K. Dowman, J. W. Tomlinson and P. N. Newsome, Pathogenesis of non-alcoholic fatty liver disease, QJM, 2010, 103, 71–83 CrossRef CAS PubMed.
- A. Leyva-Soto, R. Alejandra Chavez-Santoscoy, O. Porras, M. Hidalgo-Ledesma, A. Serrano-Medina, A. Alejandra Ramírez-Rodríguez and N. Alejandra Castillo-Martinez, Epicatechin and quercetin exhibit in vitro antioxidant effect, improve biochemical parameters related to metabolic syndrome, and decrease cellular genotoxicity in humans, Food Res. Int., 2021, 142, 110101 CrossRef CAS PubMed.
- G. Gutiérrez-Salmeán, E. Meaney, M. A. Lanaspa, C. Cicerchi, R. J. Johnson, S. Dugar, P. Taub, I. Ramírez-Sánchez, F. Villarreal, G. Schreiner and G. Ceballos, A randomized, placebo-controlled, double-blind study on the effects of (-)-epicatechin on the triglyceride/HDLc ratio and cardiometabolic profile of subjects with hypertriglyceridemia: Unique in vitro effects, Int. J. Cardiol., 2016, 223, 500–506 CrossRef PubMed.
- D. Mastroiacovo, C. Kwik-Uribe, D. Grassi, S. Necozione, A. Raffaele, L. Pistacchio, R. Righetti, R. Bocale, M. C. Lechiara, C. Marini, C. Ferri and G. Desideri, Cocoa flavanol consumption improves cognitive function, blood pressure control, and metabolic profile in elderly subjects: the Cocoa, Cognition, and Aging (CoCoA) Study–a randomized controlled trial, Am. J. Clin. Nutr., 2015, 101, 538–548 CrossRef CAS PubMed.
- T. Y. C. Tan, X. Y. Lim, J. H. H. Yeo, S. W. H. Lee and N. M. Lai, The Health Effects of Chocolate and Cocoa: A Systematic Review, Nutrients, 2021, 13, 2909 CrossRef CAS PubMed.
- W. J. Hollands, H. Tapp, M. Defernez, N. Perez Moral, M. S. Winterbone, M. Philo, A. J. Lucey, M. E. Kiely and P. A. Kroon, Lack of acute or chronic effects of epicatechin-rich and procyanidin-rich apple extracts on blood pressure and cardiometabolic biomarkers in adults with moderately elevated blood pressure: a randomized, placebo-controlled crossover trial, Am. J. Clin. Nutr., 2018, 108, 1006–1014 CrossRef PubMed.
- S. De Los Santos, L. A. Reyes-Castro, R. M. Coral-Vázquez, J. P. Méndez, M. Leal-García, E. Zambrano and P. Canto, (-)-Epicatechin reduces adiposity in male offspring of obese rats, J. Dev. Origins Health Dis., 2020, 11, 37–43 CrossRef CAS PubMed.
- T. C. Walther and R. V. Farese Jr., The life of lipid droplets, Biochim. Biophys. Acta, 2009, 1791, 459–466 CrossRef CAS PubMed.
- P. J. McFie, S. L. Stone, S. L. Banman and S. J. Stone, Topological orientation of acyl-CoA:diacylglycerol acyltransferase-1 (DGAT1) and identification of a putative active site histidine and the role of the n terminus in dimer/tetramer formation, J. Biol. Chem., 2010, 285, 37377–37387 CrossRef CAS PubMed.
- K. Choi, H. Kim, H. Kang, S. Y. Lee, S. J. Lee, S. H. Back, S. H. Lee, M. S. Kim, J. E. Lee, J. Y. Park, J. Kim, S. Kim, J. H. Song, Y. Choi, S. Lee, H. J. Lee, J. H. Kim and S. Cho, Regulation of diacylglycerol acyltransferase 2 protein stability by gp78-associated endoplasmic-reticulum-associated degradation, FEBS J., 2014, 281, 3048–3060 CrossRef CAS PubMed.
- S. K. Koliwad, R. S. Streeper, M. Monetti, I. Cornelissen, L. Chan, K. Terayama, S. Naylor, M. Rao, B. Hubbard and R. V. Farese Jr., DGAT1-dependent triacylglycerol storage by macrophages protects mice from diet-induced insulin resistance and inflammation, J. Clin. Invest., 2010, 120, 756–767 CrossRef CAS PubMed.
- J. Liu, L. Han, L. Zhu and Y. Yu, Free fatty acids, not triglycerides, are associated with non-alcoholic liver injury progression in high fat diet induced obese rats, Lipids Health Dis., 2016, 15, 27 CrossRef PubMed.
- C. Chitraju, T. C. Walther and R. V. Farese Jr., The triglyceride synthesis enzymes DGAT1 and DGAT2 have distinct and overlapping functions in adipocytes, J. Lipid Res., 2019, 60, 1112–1120 CrossRef CAS PubMed.
- F. Ali, A. Ismail, N. M. Esa and C. P. Pei, Transcriptomics expression analysis to unveil the molecular mechanisms underlying the cocoa polyphenol treatment in diet-induced obesity rats, Genomics, 2015, 105, 23–30 CrossRef CAS PubMed.
- H. Cheng, N. Xu, W. Zhao, J. Su, M. Liang, Z. Xie, X. Wu and Q. Li, (-)-Epicatechin regulates blood lipids and attenuates hepatic steatosis in rats fed high-fat diet, Mol. Nutr. Food Res., 2017, 61 Search PubMed.
- M. Pawlak, P. Lefebvre and B. Staels, Molecular mechanism of PPARα action and its impact on lipid metabolism, inflammation and fibrosis in non-alcoholic fatty liver disease, J. Hepatol., 2015, 62, 720–733 CrossRef CAS PubMed.
- N. Bougarne, B. Weyers, S. J. Desmet, J. Deckers, D. W. Ray, B. Staels and K. De Bosscher, Molecular Actions of PPARα in Lipid Metabolism and Inflammation, Endocr. Rev., 2018, 39, 760–802 CrossRef PubMed.
- J. D. Browning and J. D. Horton, Molecular mediators of hepatic steatosis and liver injury, J. Clin. Invest., 2004, 114, 147–152 CrossRef CAS PubMed.
- S. Ishii, K. Iizuka, B. C. Miller and K. Uyeda, Carbohydrate response element binding protein directly promotes lipogenic enzyme gene transcription, Proc. Natl. Acad. Sci. U. S. A., 2004, 101, 15597–15602 CrossRef CAS PubMed.
- A. K. Stoeckman, L. Ma and H. C. Towle, Mlx is the functional heteromeric partner of the carbohydrate response element-binding protein in glucose regulation of lipogenic enzyme genes, J. Biol. Chem., 2004, 279, 15662–15669 CrossRef CAS PubMed.
- K. Iizuka, R. K. Bruick, G. Liang, J. D. Horton and K. Uyeda, Deficiency of carbohydrate response element-binding protein (ChREBP) reduces lipogenesis as well as glycolysis, Proc. Natl. Acad. Sci. U. S. A., 2004, 101, 7281–7286 CrossRef CAS PubMed.
- G. Liang, J. Yang, J. D. Horton, R. E. Hammer, J. L. Goldstein and M. S. Brown, Diminished hepatic response to fasting/refeeding and liver X receptor agonists in mice with selective deficiency of sterol regulatory element-binding protein-1c, J. Biol. Chem., 2002, 277, 9520–9528 CrossRef CAS PubMed.
- G. Gutiérrez-Salmeán, P. Ortiz-Vilchis, C. M. Vacaseydel, L. Garduño-Siciliano, G. Chamorro-Cevallos, E. Meaney, S. Villafaña, F. Villarreal, G. Ceballos and I. Ramírez-Sánchez, Effects of (-)-epicatechin on a diet-induced rat model of cardiometabolic risk factors, Eur. J. Pharmacol., 2014, 728, 24–30 CrossRef PubMed.
- J. I. Ottaviani, G. Borges, T. Y. Momma, J. P. Spencer, C. L. Keen, A. Crozier and H. Schroeter, The metabolome of [2-(14)C](-)-epicatechin in humans: implications for the assessment of efficacy, safety, and mechanisms of action of polyphenolic bioactives, Sci. Rep., 2016, 6, 29034 CrossRef CAS PubMed.
- P. M. Aron and J. A. Kennedy, Flavan-3-ols: nature, occurrence and biological activity, Mol. Nutr. Food Res., 2008, 52, 79–104 CrossRef CAS PubMed.
- L. Hooper, C. Kay, A. Abdelhamid, P. A. Kroon, J. S. Cohn, E. B. Rimm and A. Cassidy, Effects of chocolate, cocoa, and flavan-3-ols on cardiovascular health: a systematic review and meta-analysis of randomized trials, Am. J. Clin. Nutr., 2012, 95, 740–751 CrossRef CAS PubMed.
- E. Márquez Campos, L. Jakobs and M. C. Simon, Antidiabetic Effects of Flavan-3-ols and Their Microbial Metabolites, Nutrients, 2020, 12, 1592 CrossRef PubMed.
- H. D. Sesso, J. E. Manson, A. K. Aragaki, P. M. Rist, L. G. Johnson, G. Friedenberg, T. Copeland, A. Clar, S. Mora, M. V. Moorthy, A. Sarkissian, W. R. Carrick and G. L. Anderson, Effect of cocoa flavanol supplementation for the prevention of cardiovascular disease events: the COcoa Supplement and Multivitamin Outcomes Study (COSMOS) randomized clinical trial, Am. J. Clin. Nutr., 2022, 115, 1490–1500 CrossRef PubMed.
- J. Kang, Z. Wang and P. I. Oteiza, (-)-Epicatechin mitigates high fat diet-induced neuroinflammation and altered behavior in mice, Food Funct., 2020, 11, 5065–5076 RSC.
|
This journal is © The Royal Society of Chemistry 2024 |