DOI:
10.1039/D3FO03319H
(Paper)
Food Funct., 2024,
15, 295-309
Isoquercitrin alleviates lipopolysaccharide-induced intestinal mucosal barrier damage in mice by regulating TLR4/MyD88/NF-κB signaling pathway and intestinal flora†
Received
11th August 2023
, Accepted 3rd December 2023
First published on 6th December 2023
Abstract
Intestinal mucosal barrier damage is closely associated with the development of several intestinal inflammatory diseases. Isoquercitrin (IQ) is a natural flavonoid compound derived from plants, which exhibits high antioxidant and anti-inflammatory activity with minimal side effects in humans. Therefore, it shows great potential for preventing and treating intestinal mucosal barrier damage. This study aims to investigate the ameliorative effect and mechanism of IQ on lipopolysaccharide (LPS)-induced intestinal mucosal barrier damage in mice. The mice were treated with IQ for 7 days and then injected with LPS to induce intestinal mucosal barrier damage. The results revealed that IQ treatment alleviated LPS-induced intestinal mucosal barrier damage in mice, which can be evidenced by the improvements in intestinal morphology and the promotion of expression in intestinal tight junctions (ZO-1, Claudin-1, and Occludin), as well as MUC2 mucin. IQ also attenuated intestinal inflammatory responses by inhibiting the TLR4/MyD88/NF-κB signaling pathway and reducing the expression and plasma levels of IL-6, IL-1β, and TNF-α. Furthermore, IQ significantly increased the relative abundance of beneficial bacteria, including Dubosiella, Akkermansia muciniphila and Faecalibaculum rodentium, while suppressing the growth of harmful bacteria such as Mucispirillum schaedleri in the intestinal flora of mice. Consequently, IQ can alleviate the LPS-induced intestinal mucosal barrier damage in mice by inhibiting the TLR4/MyD88/NF-κB signaling pathway and modulating the intestinal flora.
1 Introduction
The intestinal mucosal barrier is one of the internal barriers with the largest contact area between the organism and the external environment, which mainly consists of immune cells in the lamina propria, intestinal epithelial cells (IECs), intestinal tight junctions (TJs), mucus layer, and intestinal commensal microbiota.1,2 The intestinal mucosal barrier can protect the organism against harmful microorganisms and their metabolites, exogenous toxins, and some pathogens, thus helping to maintain the health of the organism. Damage to the intestinal mucosal barrier can cause low-grade inflammation in the intestine, which in turn may lead to the development of a variety of intestinal inflammatory diseases, such as inflammatory bowel disease (IBD), celiac disease, and irritable bowel syndrome (IBS).3,4 In recent years, intestinal inflammatory diseases have developed into a global challenge, causing significant disruption to the lives of patients.5,6 In addition, with the increasing prevalence, intestinal inflammatory diseases have brought a huge burden to international healthcare systems.7 Drug therapy is a common treatment for intestinal inflammation, such as corticosteroids, aminosalicylates, and immunosuppressive drugs.8–10 However, long-term use of these medicines can cause various side effects, such as osteoporosis, insulin resistance, and a few adverse gastrointestinal reactions.11,12 Therefore, it is necessary to find a treatment with better safety for intestinal mucosal injury.
Notably, many natural phytochemicals derived from plants present the advantage of low toxicity, and some of them have been confirmed to have favorable protective effects against intestinal mucosal injury.13,14 Isoquercitrin (IQ) is a natural flavonoid compound, which is widely found in plants and their products.15 Studies have found that IQ has many biological functions, such as antioxidant, anti-inflammatory, and immune regulation.16–22 In addition, as a 3-O-glucoside derivative of quercetin, IQ shows high bioavailability in vivo.23 IQ has been widely used as an antioxidant in the food industry in Japan and the United States and was granted Generally Recognized as Safe (GRAS) status by FEMA and FDA in 2005 and 2007, respectively.24,25 Lots of flavonoids have been demonstrated to have good ameliorative effects on intestinal mucosal barrier damage.14,26 Therefore, IQ is expected to play an important role as a food supplement in the improvement of intestinal mucosal barrier damage in terms of affordability, beneficial biological activity, and few side effects.
There is a large intestinal micro-ecosystem of trillions of microorganisms in the intestine, which can regulate the health and disease of the organism.27 Intestinal microbial communities and their internal environment homeostasis are central elements in maintaining the function of the intestinal mucosa barrier.28 The intestinal microbiota is beneficial to human health as evidenced by facilitating the digestion and absorption of food, reducing the growth of pathogenic bacteria, and maintaining the integrity of the intestinal mucosal barrier.29 The intestinal microbiota can produce many important metabolites, such as short-chain fatty acids (SCFAs), which are mainly composed of acetic acid, propionic acid, and butyric acid.30 Importantly, the SCFAs play a crucial role in maintaining human intestinal and metabolic health, including helping to ensure the integrity of IECs, regulating intestinal immunity, and improving intestinal inflammation responses.31,32 Therefore, the intestinal microbiota can serve as a crucial target in terms of intestinal mucosal barrier protection by regulating its composition or metabolism.
LPS is one of the most effective inducers of the immune system.33–35 It has been shown that LPS can induce inflammatory responses in the intestinal mucosa.36–38 The TLR4/MyD88/NF-κB signaling pathway is critical in the regulation of inflammatory responses.39 Upon binding of LPS to the Toll-like 4 receptor (TLR4) on the surface of immune cells, the signaling bridging protein myeloid differentiation factor 88 (MyD88) is recruited, and subsequently, MyD88 binds to the cytoplasmic structural domain of TLR4 and releases downstream nuclear factor-κB (NF-κB), leading to the overexpression of some pro-inflammatory cytokines, triggering inflammation, and causing apoptosis of IECs.40–45 Finally, the intestinal mucosal barrier was disrupted. Many researchers have reported that inhibition of the TLR4/MyD88/NF-κB signaling pathway can downregulate the levels of pro-inflammatory cytokines, thereby reducing intestinal inflammatory responses.46–48
However, there is no relevant study to investigate the ameliorative effect of IQ on LPS-induced intestinal mucosal barrier damage from the perspectives of intestinal flora and the TLR4/MyD88/NF-κB signaling pathway. In this study, we investigated the role of IQ in the protection against intestinal mucosal barrier damage by gavaging mice with different doses of IQ and constructing a mouse model of LPS-induced intestinal mucosal barrier damage. Subsequently, the changes in the TLR4/MyD88/NF-κB signaling pathway and the composition of intestinal flora were investigated to explore the possible mechanisms. This study can provide new perspectives and methods for the prevention and treatment of intestinal mucosal barrier damage, as well as more theoretical references for the research and development of IQ-related healthcare products and pharmaceuticals.
2 Materials and methods
2.1 Materials and animals
IQ (purity ≥98%) was purchased from Vicky Biotechnology Co., Ltd (Sichuan, China). LPS (O55:B5, #L2880) was purchased from Sigma-Aldrich Corp. (St Louis, MO, USA). A total of 40 C57BL/6 male mice (6 weeks, BW: 20 ± 2 g) were purchased from Guangdong Medical Laboratory Animal Center (production license number: SCXK (Guangdong) 2022-0002) (Guangzhou, China). All animal procedures were conducted in accordance with the regulations of the Animal Center of China and were approved by the Ethics Committee of Huateng Biomedical Technology Co., Ltd (ethical lot number: HT-401-ABM03) (Guangzhou, China). Mice were acclimatized for one week before the start of the formal experiment. During the experiment, a temperature of 23 ± 3 °C, humidity of 40–70%, and a schedule of 12 h each in light and dark were used. The mice were allowed to drink freely and provided with standard feed. And the mice were housed in 8 cages, with 5 mice per cage.
2.2 Experimental protocols of animals
General experimental procedures for mice are shown in Fig. 1A. After one week of acclimatization, the mice were randomly divided into four treatment groups, namely Control, Model, IQ-low dose (IQ-L, 50 mg per kg BW), and IQ-high dose (IQ-H, 100 mg per kg BW). During the treatment period, the mice in the Control and Model groups were administrated with 0.1 mL per 10 g BW of normal saline (NS) by oral gavage, while the mice in IQ-treated groups were gavaged with 0.1 mL 10 g BW of IQ mixture with low and high doses described above once daily for 7 days. On the 7th day, 2 h after the gavage of NS or IQ, mice in the Model and IQ-treated groups were intraperitoneally injected with 2.5 mg per kg BW of LPS (0.1 mL 10 g BW) and the mice in the Control group were intraperitoneally injected with NS of 0.1 mL 10 g BW. 6 h later, mice were anesthetized with 0.3% sodium pentobarbital. Blood was taken from the retro-orbital and immediately collected in purple anticoagulated blood collection tubes containing EDTA-K2 (Tianai Medical Equipment Co., Ltd, Shandong, China). Then mice were sacrificed by cervical dislocation. The plasma was obtained by centrifuging the collected blood for 10 min at 4 °C with a speed of 3500 rpm min−1 after standing at room temperature for 30 min. The cecum contents were collected for 16s rRNA sequencing. The duodenum, ileum, and colon tissues were collected for intestinal morphology observation and quantitative polymerase chain reaction (Q-PCR) analysis.
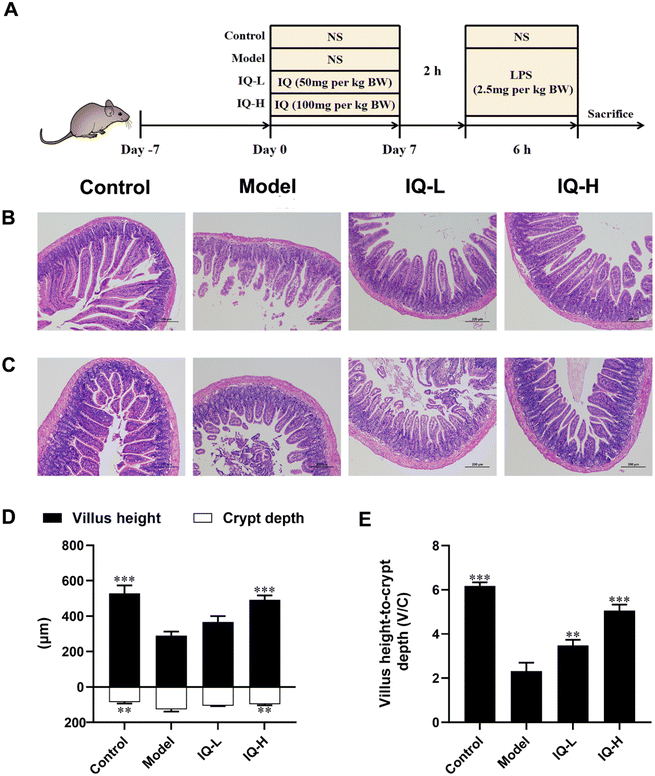 |
| Fig. 1 IQ treatment alleviates damage induced by LPS in small intestine morphology. General experimental procedures for mice (A). Photomicrographs of the duodenum (B) and ileum (C) for the different treatments by H-E staining (magnification: 100×, scale bar: 200 μm). Villus height and crypt depth (D) were measured by Image J software. The villus height to crypt depth ratio (V/C) (E). All the data were expressed as mean ± SD, and the number of biological replicates was three (n = 3). * p < 0.05, ** p < 0.01, and *** p < 0.001 vs. the Model group. | |
2.3 Small intestinal morphology analysis
Duodenum and ileum tissues (1–2 cm) were fixed in 4% paraformaldehyde and then embedded in paraffin. Hematoxylin and eosin were used successively to stain paraffin sections of 5 μm thickness. The intestinal morphology was observed by a microscope (Nikon Eclipse Ci-E, Tokyo, Japan) and captured by a digital microscope camera (Nikon DS-L4, Tokyo, Japan) at a magnification of 100. The villus height and crypt depth were measured by Image J software (National Institutes of Health, Bethesda, MD, USA).
2.4 Enzyme-linked immunosorbent assay (ELISA)
The levels of IL-6, IL-1β and TNF-α in plasma were measured by ELISA kits according to the manufacturer's instructions (CUSABIO, Wuhan, China). The main steps are as follows. Firstly, reagents, samples, and standards were prepared as instructed. Secondly, standards or samples were added and incubated for 2 hours at 37 °C. Thirdly, biotin-antibody (1×) was added and incubated for 1 hour at 37 °C. Subsequently, HRP-avidin (1×) was added and incubated for 1 hour at 37 °C. Then TMB substrate was added and incubated for 20 min at 37 °C in darkness. Finally, Stop Solution was added to each well, and the absorbance measurement was taken at 490 nm using a microplate reader (Multiskan 60, Thermo Fisher Scientific, Waltham, MA, USA).
2.5 Q-PCR analysis
The total RNA of mice ileum and colon tissues was extracted with trizol reagent (Ambion, Austin, TX, USA). The concentration and quality of RNA were determined by an ultra-micro spectrophotometer (Bio-Rad Epoch, Hercules, CA, USA) according to the relative absorbance at 260 nm (A260) and 280 nm (A280). The RNA reversed transcribed to cDNA by PreScript III RT Promix kit (Inzan Biotechnology Co., Ltd, Guangzhou, China). The reverse transcription was performed as follows: first step reaction of 2 min at 42 °C and second step reaction of 5 min at 25 °C, 15 min at 50 °C, and 2 min at 85 °C with 1000 ng total RNA. A CFX96™ Real-Time System (Bio-Rad, Hercules, CA, USA) was employed for quantification analysis, and the analysis was in a 20 μL of mixture including 10 μL of 2 × Robust SYBR Green qPCR Promix (without ROX) (Inzan Biotechnology, Guangzhou, China), 0.5 μL each of forward and reverse primer (10 μM), 2 μL of cDNA template, and 7 μL of nuclease-free water. The PCR reaction procedures were set as follows: one cycle of 10 min at 95 °C, 40 cycles of 5 s at 95 °C, and 20 s at 60 °C. The gene expression levels were analyzed via the 2−ΔΔCT method and β-actin was set as an internal reference gene. The primer sequences are shown in ESI Table S1.†
2.5 16s rRNA sequencing for intestinal flora analysis
The cecum contents of mice were sent to Kidio Biotechnology Co., Ltd (Guangzhou, China) for 16s rRNA gene sequencing. The total microbial DNA of the samples was extracted by HiPure Stool NDA kits (Magen, Guangzhou, China) according to the manufacturer's protocols. The purity and integrity of DNA were assessed by a micro-spectrophotometer (NanoDrop 2000, Thermo Fisher Scientific, Waltham, MA, USA) and 1.2% agarose gels, respectively. The specific primers (341F: CCTACGGGNGGCWGCAG, 806R: GGACTACHVGGGTWTCTAAT) tagged with a barcode were used to amplify the bacterial 16S rRNA variable region of V3–V4. PCR reactions were performed using Phusion High Fidelity PCR Master Mix (New England Biolabs, Inc., Ipswich, MA, USA). The amplicons were extracted from 2% agarose gels and purified using the AxyPrep DNA Gel Extraction kit (Axygen Biosciences, Union City, CA, USA), and quantified using Qubitn3.0 Fluorometer (Thermo Fisher Scientific, USA) and ABI StepOnePlus Real-Time PCR System (Life Technologies, Foster City, USA). The quantified amplicons were pooled in equimolar and paired-end sequenced (PE250) on the Illumina MiSeq platform according to the standard protocols. The raw reads were deposited into the NCBI Sequence Read Archive (SRA) database and further filtered (including removing reads containing more than 10% of unknown nucleotides and less than 50% of bases with quality (Q-value) > 20) using FASTP (version 0.18.0). Paired-end clean reads were merged as raw tags using FLASH (version 1.2.11). Then noisy sequences of raw tags were filtered under specific filtering conditions to obtain high-quality clean tags. The clean tags were clustered into operational taxonomic units (OTUs) of 97% similarity using the UPARSE (version 9.2.64) pipeline. All chimeric tags were removed using the UCHIME algorithm and finally, effective tags were obtained for further analysis. The representative OTU sequences were classified into organisms by a naive Bayesian model using an RDP classifier (version 2.2) based on the SILVA database (version 138.1) with a confidence threshold value of 0.8. Shannon, Simpson, and Goods coverage indices were calculated in QIIME1 (version 1.9.1). OTU rarefaction curves were plotted in the R project ggplot2 package (version 2.2.1). Non-metric multidimensional scaling (NMDS) of bray–Curtis distance and Anosim (analysis of similarities) test were generated in R projects Vegan package (version 2.5.3) and plotted in R project ggplot2 package. Linear discriminant analysis (LDA) effect size (LEfSe) was used to identify key intestinal microbiota with significant differences between groups, where LDA > 3.
2.6 Statistical analysis
The experimental data were presented as means ± SD and analyzed by one-way analysis of variance (ANOVA) with SPSS Statistics 26.0 (SPSS Inc., Chicago, USA). Differences between groups were analyzed by the Tukey test for post hoc comparison with a significant level of P < 0.05.
3 Results and discussion
3.1 IQ alleviated LPS-induced intestinal barrier damage
Many studies have reported that LPS treatment can cause damage to the intestinal mucosa and disrupt the integrity of the intestinal mucosal barrier.41,49 In this work, LPS (2.5 mg per kg BW) was used to induce intestinal mucosal barrier damage. As shown in Fig. 1B–F, LPS treatment disturbed the arrangement and integrity of the villi, which was accompanied by decreased villus height and the ratio of villus height to crypt depth (V/C) (P < 0.001), as well as increased crypt depth (P < 0.01). These results suggested that extensive damage to the intestinal mucosal barrier was induced by LPS in mice. In previous studies, intestinal mucosal barrier damage was usually accompanied by villi atrophy, crypt hyperplasia, and reduced V/C, making the intestinal morphology disrupted and affecting intestinal mucosal barrier function, which was consistent with the phenomena observed in this study.50–53
Intestinal morphology can be used to assess intestinal function and health status.54 Villi are important structures of the intestinal mucosa, and intestinal nutrient absorption would be facilitated if villi height increases.55 The mice treated with IQ showed higher villus height in the duodenum than that in the Model group (Fig. 1D). Moreover, the crypt depth and V/C are crucial indicators for intestinal development and nutrient absorption in the intestine.56,57Fig. 1D revealed that IQ treatment reduced the crypt depth in the duodenum, suggesting IQ promoted cell maturation and secretion. The mice treated with IQ also showed improvement of V/C in the duodenum, which demonstrated that IQ elevated the number and nutrient absorption capacity of IECs (Fig. 1E). The above results indicate that IQ plays an important role in the protection of intestinal morphology under pathological conditions induced by LPS.
TJs are protein complexes that connect the neighboring IECs to the endothelial cells58 and mainly consist of three transmembrane proteins (including Occludin, Claudins, and adhesion molecule proteins (JAMs)) and cytoplasmic scaffolding proteins59 (including zonula occludens (ZO) 1–3 anchored to the actin cytoskeleton). Furthermore, TJs are important targets for pathogens to translocate into the organism via IECs. The defective intestinal TJs barrier might be attributable to the pathogenesis of intestinal inflammation-related diseases.60 In addition, MUC2 is a highly crucial mucin in intestinal tissues that plays an essential role in protecting the intestinal mucosal barrier and maintaining the homeostasis of the intestinal flora.61 Therefore, it is important to maintain the intestinal mucosal barrier function by regulating the levels of TJs and MUC2 mucin.62 In the present study, LPS treatment significantly reduced the mRNA expression of TJs (ZO-1, Occludin, and Claudin-1) and MUC2 in the ileum (P < 0.05), suggesting that LPS administration damaged the intestinal mucosal barrier in mice (Fig. 2), which was consistent with the results obtained in previous studies. For instance, it was reported that LPS stimulation reduced the expression of ZO-1 and Claudin-1 proteins, and then disrupted the intestinal barrier in C57BL/6 mice.63 Furthermore, Bhatia et al.64 found that LPS treatment damaged the intestinal mucosal barrier in mice and decreased the expression of MUC2 in the ileum. Thus, the increase in the expression of TJs and mucins helps to restore the integrity and function of the intestinal mucosal barrier. Occludin is a major component of TJs and interacts with other TJs such as ZO-1 to protect the intestinal mucosal barrier.65 Noticeably, ZO-1 is the first TJ identified to be located in the cytoplasmic periphery, which is essential for the repairment of the intestinal mucosal barrier.66 Claudin-1 also occupies an important position in TJs, which can influence the expression of a variety of proteins, including those related to cell adhesion and actin cytoskeletal remodeling.67 Noteworthily, numerous studies have shown that flavonoids can improve intestinal mucosal barrier damage by upregulating the expression of TJs and MUC2 mucin.68,69 Thus, as a natural flavonoid compound, IQ is expected to protect the integrity of the intestinal mucosal barrier by enhancing the expression of TJs and mucins. In the present study, IQ treatment significantly increased the mRNA expression of ZO-1, Occludin, Claudin-1, and MUC2 in the ileum, revealing that IQ improved intestinal mucosal barrier damage induced by LPS (Fig. 2). From the above results, it can be seen that IQ can enhance the function and integrity of the intestinal mucosal barrier in mice by restoring the expression of TJs and MUC2 in the intestinal tissues of mice.
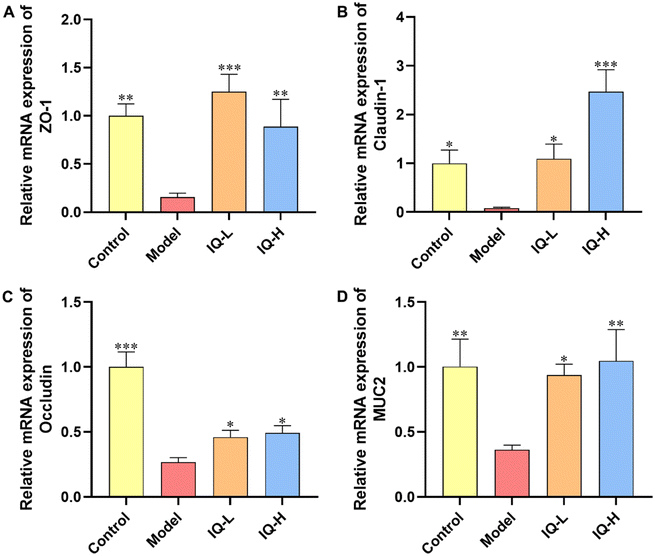 |
| Fig. 2 IQ treatment alleviates the damage of the intestinal mucosal barrier in ileum tissues induced by LPS. Relative mRNA expression levels of intestinal tight junctions, ZO-1 (A), Claudin-1 (B), Occludin (C), and MUC2 (D) in Control, Model, IQ-L, and IQ-H groups for ileum. All the data were expressed as mean ± SD, and the number of biological replicates was three (n = 3). * p < 0.05, ** p < 0.01 and *** p < 0.001 vs. the Model group. | |
3.2 IQ attenuated intestinal inflammatory responses by inhibiting the activation of TLR4/MyD88/NF-κB signaling pathway
To further investigate the regulatory mechanism of IQ improving intestinal mucosal barrier damage induced by LPS in mice, the mRNA expression of key factors of the TLR4/MyD88/NF-κB pathway was measured. The levels of pro-inflammatory factors were also determined to assess the inflammatory status of the intestinal mucosa. LPS is a component of the Gram-negative bacteria cell wall, which can activate the innate immune response of the body.70,71 As shown in Fig. S1,† upon binding of LPS to TLR4, MyD88 undergoes signal transduction and NF-κB is activated, thereby increasing the expression of downstream pro-inflammatory cytokines, allowing increased risk of intestinal mucosal barrier permeability and bacterial translocation.72 In this study, LPS treatment increased the mRNA expression of TLR4, MyD88, and NF-κB p65 in the ileum tissues of mice (P < 0.01), which demonstrated that the TLR4/MyD88/NF-κB signaling pathway was activated (Fig. 3A–C). In addition, LPS treatment increased the expression of pro-inflammatory cytokine mRNA in colonic tissue and the levels of pro-inflammatory cytokines in the plasma, including IL-6, IL-1β, and TNF-α (Fig. 3D–I), suggesting that the intestinal mucosa of mice was in an inflammatory state after LPS treatment. Qu et al.73 also found that inhibition of the TLR4/NF-κB signaling pathway reduced the levels of IL-1β, IL-6, and TNF-α while improving the integrity of intestinal TJs and alleviating colonic mucosal inflammation in mice, which protected the intestinal mucosal barrier. Moreover, it was reported that inhibition of the TLR4/NF-κB signaling pathway alleviated the inflammatory response induced by gut microbial-derived LPS in vivo, which resulted in the protection of the intestinal mucosal barrier.74 Similarly, Peng et al.75 also reported that the downregulation of the NF-κB signaling pathway improved intestinal mucosal barrier dysfunction of ulcerative colitis in mice, and decreased the expression of IL-6, IL-1β, and TNF-α to alleviate intestinal mucosal inflammation in mice. Therefore, the TLR4/MyD88/NF-κB signaling pathway is an important target to alleviate intestinal inflammation and improve intestinal mucosal barrier function. Researchers have found that IQ is effective in suppressing inflammatory responses by modulating the NF-κB pathway. For instance, Li et al.76 observed that IQ inhibited the NF-κB signaling pathway in activated human basophilic KU812 cells, thereby suppressing the production of histamine, as well as IL-6, IL-8, IL-1β, and TNF-α. Ma et al.77 found that IQ ameliorated myocardial infarction in rats by modulating the TLR4/NF-κB signaling pathway and alleviating cardiac inflammation. However, studies on IQ ameliorating LPS-induced inflammatory responses in the intestinal mucosa by modulating the TLR4/MyD88/NF-κB pathway have not been reported. In the present study, IQ treatment decreased the mRNA expression of TLR4, MyD88, and NF-κB p65 in mice ileal tissues (Fig. 3A–C, P < 0.01), revealing that the activation of this signaling pathway was blocked. Furthermore, mice in IQ-treated groups showed reduced colon mRNA expression and plasma levels of IL-6, IL-1β, and TNF-α (Fig. 3D–I), demonstrating that IQ treatment attenuated the intestinal mucosal inflammatory responses in mice. Based on the above results, it is clear that the protective effect of IQ on the intestinal mucosal barrier might be related to the relief of the LPS-induced intestinal mucosal inflammation, which was achieved via inhibiting the activation of TLR4/MyD88/NF-κB signaling pathway and reducing the levels of IL-6, IL-1β, and TNF-α.
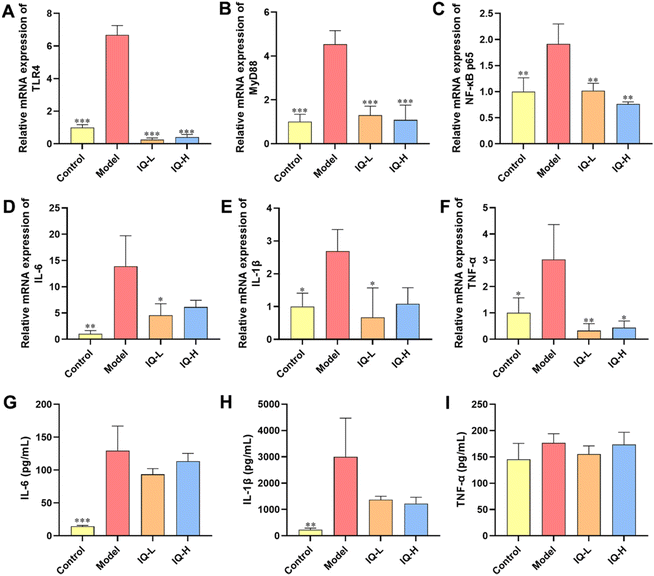 |
| Fig. 3 IQ treatment inhibits the activation of the TLR4/MyD88/NF-κB signaling pathway and slows down intestinal inflammation to improve intestinal mucosal barrier damage induced by LPS. Relative mRNA expression of TLR4 (A), MyD88 (B), and NF-κB p65 (C) for ileum, as well as IL-6 (D), IL-1β (E), and TNF-α (F) for the colon. Plasma levels of IL-6 (G), IL-1β (H), and TNF-α (I). All the data were expressed as mean ± SD, and the number of biological replicates was three (n = 3). * p < 0.05, ** p < 0.01, and *** p < 0.001 vs. the Model group. | |
3.3 IQ modulated intestinal flora in mice
As the largest symbiotic ecosystem of the organism, the intestinal microbiota is involved in regulating the integrity and function of the intestinal mucosal barrier.78–80 Therefore, to investigate the role of intestinal flora in the prevention of intestinal mucosal barrier damage, 16s rRNA sequencing was employed to analyze the composition of the gut microbiota in mice.
3.3.1 IQ altered the diversity and composition of intestinal flora in mice.
The average number of OTUs in each group obtained by partitioning all sequences based on a 97% similarity ranged from 886 to 1074 (Table 1). As shown in Fig. 4E, the sequencing coverage of each group was >99%, indicating the sequencing results represented the real situation in samples (Fig. 4E and Table 1). The ACE and Shannon curves tended to be flat (Fig. 4A and B), revealing the number of samples was sufficient.81 The Shannon and Simpson indices were associated with the richness and diversity of the intestinal microbial community, with larger index values suggesting greater richness and diversity.82 As shown in Fig. 4C, D and Table 1, LPS treatment decreased the Shannon index (5.56 ± 0.38 vs. 6.31 ± 0.28, P < 0.05) and Simpson index (0.91 ± 0.03 vs. 0.95 ± 0.01, P > 0.05) of the intestinal flora compared to the Control group, which indicate that LPS reduced the richness and diversity of mice intestinal microbiota. Compared to the Model group, the mice in the IQ-treated groups showed a slightly increase in both the Shannon index (5.99 ± 0.37 vs. 5.56 ± 0.38, 5.88 ± 0.22 vs. 5.56 ± 0.38) and Simpson index (0.95 ± 0.02 vs. 0.91 ± 0.03, 0.95 ± 0.01 vs. 0.91 ± 0.03) of intestinal microbiota, although it was not statistically significant. The above results suggest that IQ may improve the richness and diversity of intestinal microbiota to some extent.
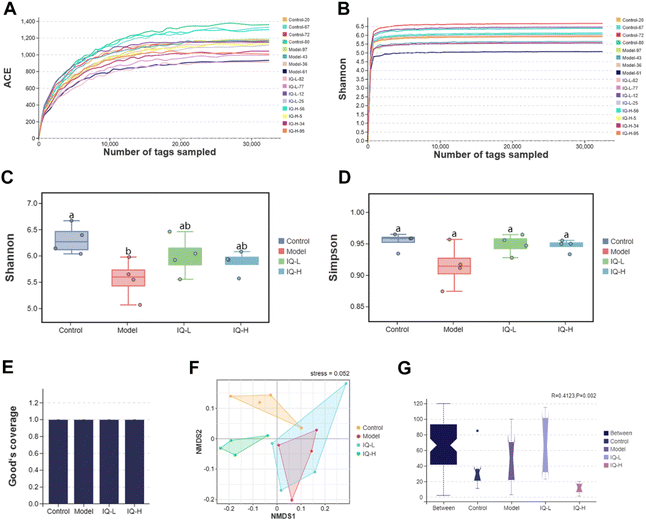 |
| Fig. 4 IQ modifies the microbial diversity and structure. The Shannon (A) and Simpson (B) rarefaction curves. The Shannon (C), Simpson (D), and Good's coverage (E) indices. NMDS analysis based on bray–Curtis distance (F). Anosim analysis (G). The Anosim analysis is a non-parametric test that could be used to determine whether the between-group or within-group distance is significantly different. R > 0 means there is a difference in the intestinal microbiota between groups, and P is used to determine whether the difference is significant. Shannon, Simpson, and Good's coverage indices were analyzed by the Tukey-HSD test, and the bray–Curtis distance was analyzed using the Kruskal–Wallis H test, with a significant level of p < 0.05. The number of biological replicates was four (n = 4). Different letters on the bars mean statistical difference with p < 0.05. | |
Table 1 Alpha diversity in different groups
|
Control (n = 4) |
Model (n = 4) |
IQ-L (n = 4) |
IQ-H (n = 4) |
Tukey-HSD test, * p < 0.05 vs. the model group. |
Label |
0.97 |
0.97 |
0.97 |
0.97 |
Observed Species |
1073.75 ± 87.81 |
929.25 ± 115.60 |
886.25 ± 101.76 |
941.50 ± 108.55 |
Shannon |
6.31 ± 0.28* |
5.56 ± 0.38 |
5.99 ± 0.37 |
5.88 ± 0.22 |
Simpson |
0.95 ± 0.01 |
0.91 ± 0.03 |
0.95 ± 0.01 |
095 ± 0.02 |
Coverage (%) |
99.51 ± 0.07 |
99.51 ± 0.07 |
99.52 ± 0.08 |
99.48 ± 0.06 |
The effect of IQ on the structure and composition of the intestinal flora in LPS-induced intestinal mucosal inflammation mice was also investigated in this work. Fig. 4F showed that the stress value of the NMDs analysis was 0.052 (<0.1), indicating a good dimensionality reduction and an accurate reflection of the real data distribution. Meanwhile, the distribution of samples in the four groups could be distinguished, suggesting that there were differences in the intestinal flora between groups. In the Anosim analysis, R > 0 means there is a difference in the intestinal microbiota between groups, and P < 0.05 means the difference is significant. As shown in Fig. 4G, R-value, and P-value were 0.412 (>0) and 0.002 (<0.05) respectively, indicating a significant difference in the microbial composition of the four groups. The mutual and unique OTUs of each group were expressed by the Venn plot in Fig. 5C. 478 OTUs were mutual by all groups, with the Control group having the largest count of unique OTUs (304). Meanwhile, 193, 183, and 211 unique OTUs were observed respectively in the Model, IQ-L, and IQ-H groups. These results agreed with the richness and diversity indices shown in Fig. 3C, D and Table 1.
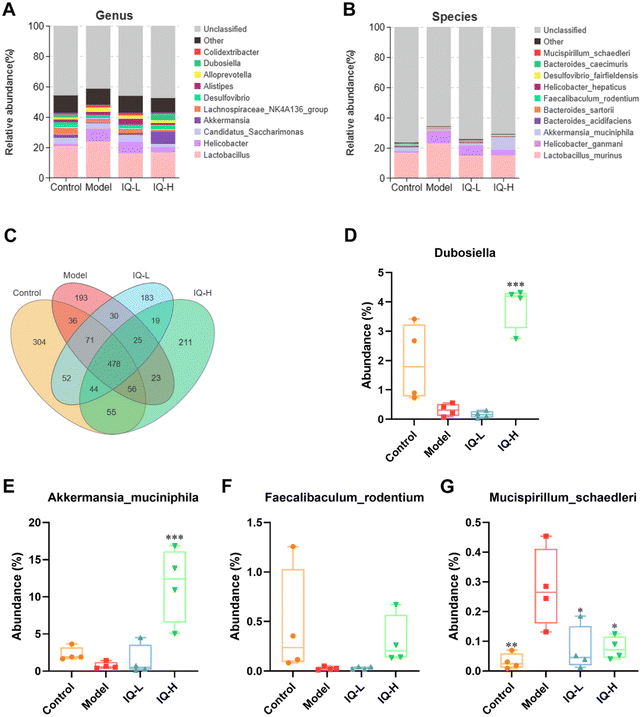 |
| Fig. 5 IQ treatment alters the intestinal microbiota composition. Intestinal microbiota composition at genus (A) and species (B) levels. Venn plot (C) that illustrated the observed OTUs counts in samples. The major intestinal microbial communities at genus (D) and species (E–G) levels. The data were expressed as mean ± SD, and analyzed by the Tukey-HSD test, and the number of biological replicates was four (n = 4). * p < 0.05, ** p < 0.01, and *** p < 0.001 vs. the Model group. | |
On this basis, the intestinal microbial communities at the genus and species levels were investigated in this work. Fig. 5A showed the relative abundance of the intestinal microbial communities at the genus level, and ten major genera were identified in cecum contents microbiota, namely Lactobacillus, Alloprevotella, Alistipes, Akkermansia, Helicobacter, Dubosiella, Desulforibrio, Candidatus Saccharimon, Lachnospiraceae NKaA136 group, and Colidextribacter. Notably, from Fig. 5D and Table 2, it can be observed that the average relative abundance of Dubosiella was lower in the Model group compared to the Control group, although the difference was not statistically significant. However, IQ (100 mg per kg BW) treatment significantly increased the relative abundance of Dubosiella (3.87 ± 0.75 vs. 0.32 ± 0.21, P < 0.001) in the intestinal microbial community in comparison with that in the Model group. Dubosiella has been reported to be an important microbial group producing butyrate.83 Butyrate is known to be an important energy substance for IECs, which can regulate the proliferation and differentiation of IECs, and slow down the intestinal inflammatory responses.84,85 Therefore, Dubosiella can protect the intestinal mucosal barrier by enhancing the homeostasis of IECs and increasing the production of butyrate.
Table 2 Relative abundance of some bacteria in each group
|
Control |
Model |
IQ-L |
IQ-H |
Tukey-HSD test, * p < 0.05, ** p < 0.01, *** p < 0.001 vs. the model group. |
Dubosiella (%) |
1.93 ± 1.33 |
0.32 ± 0.21 |
0.16 ± 0.12 |
3.87 ± 0.75*** |
Akkermansia muciniphila (%) |
2.29 ± 0.92 |
0.75 ± 0.47 |
1.42 ± 2.10 |
11.69 ± 5.04*** |
Faecalibaculum rodentium (%) |
0.38 ± 0.55 |
0.03 ± 0.02 |
0.03 ± 0.01 |
0.30 ± 0.25 |
Mucispirillum schaedleri (%) |
0.03 ± 0.03** |
0.28 ± 0.13 |
0.07 ± 0.08* |
0.08 ± 0.04* |
To further explore the effect of IQ on gut microbiota, we analyzed it at the species level. Fig. 4B showed the relative abundance of the intestinal microbiota at the species level, and ten major species were identified in cecum contents microbiota, namely Lactobacillus murinus, Bacteroides acidifaciens, Bacteroides sartorii, Faecalibaculum rodentium (F. rodentium), Akkermansia muciniphila (A. muciniphila), Desulfovibrio fairfieldensis, Helicobacter hepaticus, Bacteroides caecimuris, Helicobacter ganmani, and Mucispirillum schaedleri (M. schaedleri). The results of Fig. 5F–H and Table 2 revealed that the mice in the Model group showed a decrease in the relative abundance of F. rodentium and A. muciniphila in the intestinal tract, while an increase in the relative abundance of M. schaedleri (P < 0.01) in comparison to the Control group. As shown in Fig. 5F and Table 2, 100 mg per kg BW of IQ treatment increased the relative abundance of F. rodentium in mice intestinal microbial community, as compared with that in the Model group (0.30 ± 0.25 vs. 0.03 ± 0.02). F. rodentium, a potentially beneficial bacterium belonging to Eyrsipelotrichaceae, has been reported to be one of the major producers of butyrate and is presumed to serve as a substitute for Bifidobacterium which is a producer of SCFAs.86–88F. rodentium can also maintain the homeostasis of IECs by regulating related signaling pathways.89 Thus, F. rodentium can protect the intestinal mucosal barrier by modulating IECs and increasing the production of SCFAs. Moreover, the results of Fig. 5E and Table 2 demonstrated that IQ treatment increased the relative abundance of A. muciniphila in comparison with that in the Model group (1.42 ± 0.12 vs. 0.75 ± 0.47, 11.69 ± 5.04 vs. 0.75 ± 0.47 (P < 0.001), respectively). A. muciniphila is reported to be a probiotic that colonizes on the mucus layer of the intestinal mucosa and can obtain energy by decomposing mucins. A. muciniphila can also reduce intestinal inflammation and thus protect the intestinal mucosal barrier.90 In addition, A. muciniphila can produce a variety of SCFAs, such as acetate, propionate, and butyrate, which contribute to the decomposition of mucin.91,92 It is reported that propionate could protect the intestinal mucosal barrier by upregulating the expression of TJs while inhibiting the activation of the AKT signaling pathway.93 Thus, A. muciniphila can protect the intestinal mucosal barrier by increasing the decomposition of mucins and the production of SCFAs. Furthermore, the results shown in Fig. 5G and Table 2 revealed that IQ decreased the relative abundance of M. schaedleri compared with that of the Model group (0.07 ± 0.08 vs. 0.28 ± 0.13, 0.08 ± 0.04 vs. 0.28 ± 0.13, respectively, P < 0.05). M. schaedleri is reported to colonize the intestinal mucus layer.94 And M. schaedleri is of low abundance and high pathogenic potential, which can induce intestinal inflammation.95,96 Based on the above results, it can be known that IQ could increase the relative abundance of SCFAs-producing bacteria and decrease the relative abundance of harmful bacteria M. schaedleri to ameliorate the intestinal mucosal barrier damage. Regrettably, the correlation between these beneficial bacteria and SCFAs in this study needs to be explored in subsequent studies. In summary, IQ could relieve the disturbance of intestinal flora composition caused by LPS in mice.
3.3.2 IQ altered biomarkers in the intestinal flora of mice.
To further investigate the influence induced by LPS and IQ treatment on the intestinal microbial community of mice, LEfSe analysis was performed to identify the bacterial taxa that were significantly different among all the groups. It was found that 10, 15, 4, and 14 bacterial taxa were enriched in the Control, Model, IQ-L, and IQ-H groups, respectively (Fig. 6). Lachnospiraceae, Lachnospirales, and Lachnospiraceae_NK4A136_group were enriched in the Control group (LDA > 4). Helicobacteraceae, Helicobacter, Campilobacterota, Campylobacterales, and Campylobacteria were enriched in the Model group (LDA > 4). In addition, Verrucomicrobiota, Verrucomicrobiales, Verrucomicrobiales, Akkermansiaceae, Akkermansia, and A. muciniphila were enriched in the IQ-H group (LDA > 4). In summary, LPS and IQ treatment altered biomarkers in mice intestinal microbiota.
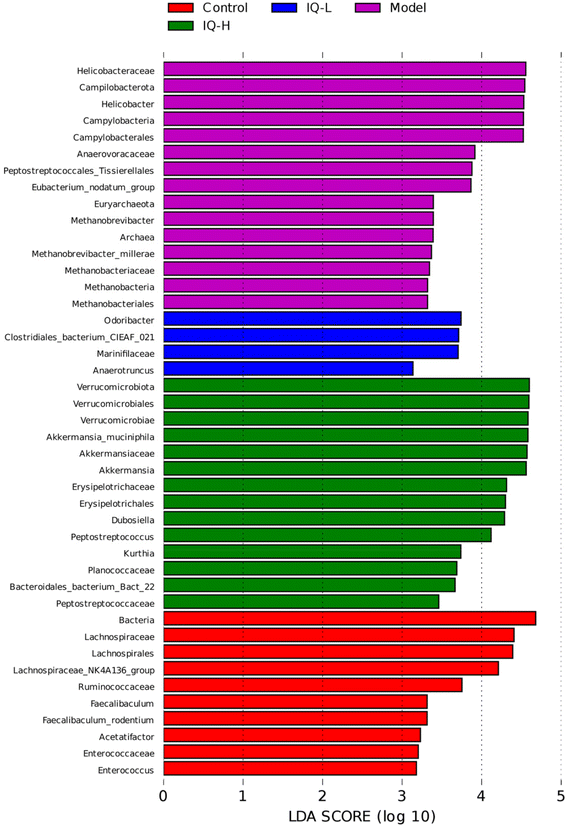 |
| Fig. 6 Analysis of key microbial communities of the four groups shown by LEfSe, and Wilcoxon and Kruskal Wallis tests were used to screen for intestinal microbiota with between-group differences in LEfSe analysis, with a significant level of p < 0.05. The larger the LDA scores, suggests a greater significant difference. | |
Taken together, IQ treatment in mice can alter the diversity and composition of intestinal flora, increasing the relative abundance of beneficial bacteria and decreasing the relative abundance of harmful bacteria, thereby improving the intestinal micro-ecological environment to protect the intestinal mucosal barrier.
4 Conclusion
IQ can alleviate LPS-induced intestinal mucosal barrier damage in mice by restoring intestinal morphology and enhancing the integrity of the intestinal mucosal barrier. A possible mechanism that IQ protects the intestinal mucosal barrier is summarized in Fig. 7. IQ can reduce intestinal mucosal inflammation by blocking the activation of the TLR4/MyD88/NF-κB signaling pathway to down-regulate the expression and content of pro-inflammatory cytokines, thereby improving intestinal mucosal barrier damage in mice. On the other hand, IQ can also regulate the structure and composition of intestinal flora in mice, promote the growth of beneficial bacteria, and inhibit the reproduction of harmful bacteria, thus improving the intestinal micro-ecological environment, which is conducive to the maintenance of the intestinal mucosal barrier health.
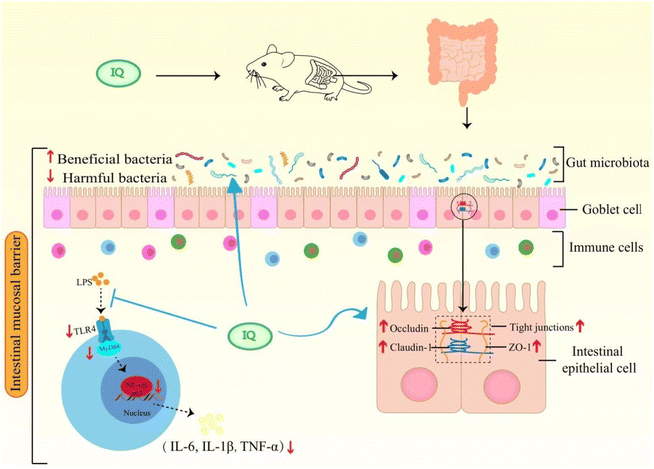 |
| Fig. 7 Possible mechanism of IQ alleviating LPS-induced intestinal mucosal barrier damage in mice. | |
Author contributions
E. Tang: conceptualization, methodology, software, validation, investigation, formal analysis, data curation, writing – original draft; T. Hu: methodology, formal analysis, investigation, data curation; Z. Jiang: formal analysis, investigation, data curation; X. Shen: investigation, data curation; H. Lin: investigation, data curation; H. Xian: investigation, data curation; X. Wu: conceptualization, funding acquisition, resources, supervision, writing – review & editing.
Conflicts of interest
This work has no conflict of interest.
Acknowledgements
The present research was supported by Guangdong Basic and Applied Basic Research Foundation (Grant No. 2021A1515110159), the Project of Educational Commission of Guangdong Province (2017KQNCX166), the Science and Technology Program of Guangzhou (202201011355), Discipline Construction Project of Guangzhou Medical University (2023) and Student Innovation Ability Improvement Program of Guangzhou Medical University (2022).
References
- Y. Dong, H. Fan, Z. Zhang, F. Jiang, M. Li, H. Zhou, W. Guo, Z. Zhang, Z. Kang, Y. Gui, Z. Shou, J. Li, R. Zhu, Y. Fu, A. Sarapultsev, H. Wang, S. Luo, G. Zhang and D. Hu, Berberine ameliorates DSS-induced intestinal mucosal barrier dysfunction through microbiota-dependence and Wnt/β-catenin pathway, Int. J. Biol. Sci., 2022, 18, 1381–1397 CrossRef CAS PubMed
.
- M. W. Rohr, C. A. Narasimhulu, T. A. Rudeski-Rohr and S. Parthasarathy, Negative Effects of a High-Fat Diet on Intestinal Permeability: A Review, Adv. Nutr., 2020, 11, 77–91 CrossRef PubMed
.
- G. Barbara, M. R. Barbaro, D. Fuschi, M. Palombo, F. Falangone, C. Cremon, G. Marasco and V. Stanghellini, Inflammatory and Microbiota-Related Regulation of the Intestinal Epithelial Barrier, Front. Nutr., 2021, 8, 718356 CrossRef PubMed
.
- J. Martel, S. H. Chang, Y. F. Ko, T. L. Hwang, J. D. Young and D. M. Ojcius, Gut barrier disruption and chronic disease, Trends Endocrinol. Metab., 2022, 33, 247–265 CrossRef CAS PubMed
.
- A. M. Kocot, E. Jarocka-Cyrta and N. Drabińska, Overview of the Importance of Biotics in Gut Barrier Integrity, Int. J. Mol. Sci., 2022, 23, 2896 CrossRef CAS PubMed
.
- T. H. Bisgaard, K. H. Allin, L. Keefer, A. N. Ananthakrishnan and T. Jess, Depression and anxiety in inflammatory bowel disease: epidemiology, mechanisms and treatment, Nat. Rev. Gastroenterol. Hepatol., 2022, 19, 717–726 CrossRef PubMed
.
- M. Jain and J. Venkataraman, Inflammatory bowel disease: An Indian perspective, Indian J. Med. Res., 2021, 153, 421–430 CrossRef CAS PubMed
.
- J. B. Rice, A. G. White, L. M. Scarpati, G. Wan and W. W. Nelson, Long-term Systemic Corticosteroid Exposure: A Systematic Literature Review, Clin. Ther., 2017, 39, 2216–2229 CrossRef CAS PubMed
.
- C. Le Berre, G. Roda, M. Nedeljkovic Protic, S. Danese and L. Peyrin-Biroulet, Modern use of 5-aminosalicylic acid compounds for ulcerative colitis, Expert Opin. Biol. Ther., 2020, 20, 363–378 CrossRef CAS PubMed
.
- F. Yasmin, H. Najeeb, S. Shaikh, M. Hasanain, U. Naeem, A. Moeed, T. Koritala, S. Hasan and S. Surani, Novel drug delivery systems for inflammatory bowel disease, World J. Gastroenterol., 2022, 28, 1922–1933 CrossRef CAS PubMed
.
- A. L. Buchman, Side effects of corticosteroid therapy, J. Clin. Gastroenterol., 2001, 33, 289–294 CrossRef CAS PubMed
.
- C. Beaupere, A. Liboz, B. Fève, B. Blondeau and G. Guillemain, Molecular Mechanisms of Glucocorticoid-Induced Insulin Resistance, Int. J. Mol. Sci., 2021, 22, 623 CrossRef CAS PubMed
.
- R. Wang, T. Chen, Q. Wang, X. M. Yuan, Z. L. Duan, Z. Y. Feng, Y. Ding, F. Bu, G. P. Shi and Y. G. Chen, Total Flavone of Abelmoschus manihot Ameliorates Stress-Induced Microbial Alterations Drive Intestinal Barrier Injury in DSS Colitis, Drug Des. Devellvery Ther., 2021, 15, 2999–3016 CrossRef PubMed
.
- J. Zhang, F. Liang, Z. Chen, Y. Chen, J. Yuan, Q. Xiong, S. Hou, S. Huang, C. Liu and J. Liang, Vitexin Protects against Dextran Sodium Sulfate-Induced Colitis in Mice and Its Potential Mechanisms, J. Agric. Food Chem., 2022, 70, 12041–12054 CrossRef CAS PubMed
.
- M. Mbikay and M. Chrétien, Isoquercetin as an Anti-Covid-19 Medication: A Potential to Realize, Front. Pharmacol., 2022, 13, 830205 CrossRef CAS PubMed
.
- V. C. Mata and J. Goldberg, Morin and isoquercitrin protect against ischemic neuronal injury by modulating signaling pathways and stimulating mitochondrial biogenesis, Nutr. Neurosci., 2022, 26, 796–806 CrossRef PubMed
.
- V. Carmona, S. Martín-Aragón, J. Goldberg, D. Schubert and P. Bermejo-Bescós, Several targets involved in Alzheimer's disease amyloidogenesis are affected by morin and isoquercitrin, Nutr. Neurosci., 2020, 23, 575–590 CrossRef CAS PubMed
.
- M. Manzoor, M. Muroi, N. Ogawa, H. Kobayashi, H. Nishimura, D. Chen, O. B. Fasina, J. Wang, H. Osada, M. Yoshida, L. Xiang and J. Qi, Isoquercitrin from Apocynum venetum L. produces an anti-obesity effect on obese mice by targeting C-1-tetrahydrofolate synthase, carbonyl reductase, and glutathione S-transferase P and modification of the AMPK/SREBP-1c/FAS/CD36 signaling pathway in mice in vivo, Food Funct., 2022, 13, 10923–10936 RSC
.
- E. H. Lee, H. J. Park, H. Y. Jung, I. K. Kang, B. O. Kim and Y. J. Cho, Isoquercitrin isolated from newly bred Green ball apple peel in lipopolysaccharide-stimulated macrophage regulates NF-κB inflammatory pathways and cytokines, 3 Biotech, 2022, 12, 100 CrossRef PubMed
.
- H. J. Park, H. N. Kim, C. Y. Kim, M. D. Seo and S. H. Baek, Synergistic Protection by Isoquercitrin and Quercetin against Glutamate-Induced Oxidative Cell Death in HT22 Cells via Activating Nrf2 and HO-1 Signaling Pathway: Neuroprotective Principles and Mechanisms of Dendropanax morbifera Leaves, Antioxidants, 2021, 10, 554 CrossRef CAS PubMed
.
- Y. Cao, L. Xie, K. Liu, Y. Liang, X. Dai, X. Wang, J. Lu, X. Zhang and X. Li, The antihypertensive potential of flavonoids from Chinese Herbal Medicine: A review, Pharmacol. Res., 2021, 174, 105919 CrossRef CAS PubMed
.
- A. Nile, S. H. Nile, J. Shin, G. Park and J. W. Oh, Quercetin-3-Glucoside Extracted from Apple Pomace Induces Cell Cycle Arrest and Apoptosis by Increasing Intracellular ROS Levels, Int. J. Mol. Sci., 2021, 22, 10749 CrossRef CAS PubMed
.
- K. Valentová, J. Vrba, M. Bancířová, J. Ulrichová and V. Křen, Isoquercitrin: pharmacology, toxicology, and metabolism, Food Chem. Toxicol., 2014, 68, 267–282 CrossRef PubMed
.
- D. Mahapatra, D. A. Donahue, A. Nyska, S. M. Hayashi, M. Koyanagi and R. R. Maronpot, alpha-Glycosyl Isoquercitrin (AGIQ) and its lack of carcinogenicity in rasH2 mice, Food Chem. Toxicol., 2021, 151, 112103 CrossRef CAS PubMed
.
- C. A. Hobbs, M. Koyanagi, C. Swartz, J. Davis, S. Kasamoto, R. Maronpot, L. Recio and S. M. Hayashi, Comprehensive evaluation of the flavonol anti-oxidants, alpha-glycosyl isoquercitrin and isoquercitrin, for genotoxic potential, Food Chem. Toxicol., 2018, 113, 218–227 CrossRef CAS PubMed
.
- J. Zhang, X. Xu, N. Li, L. Cao, Y. Sun, J. Wang, S. He, J. Si and D. Qing, Licoflavone B, an isoprene flavonoid derived from licorice residue, relieves dextran sodium sulfate-induced ulcerative colitis by rebuilding the gut barrier and regulating intestinal microflora, Eur. J. Pharmacol., 2022, 916, 174730 CrossRef CAS PubMed
.
- E. Rinninella, P. Raoul, M. Cintoni, F. Franceschi, G. A. D. Miggiano, A. Gasbarrini and M. C. Mele, What is the Healthy Gut Microbiota Composition? A Changing Ecosystem across Age, Environment, Diet, and Diseases, Microorganisms, 2019, 7, 14 CrossRef CAS PubMed
.
- C. A. Philips and P. Augustine, Gut Barrier and Microbiota in Cirrhosis, J. Clin. Exp. Hepatol., 2022, 12, 625–638 CrossRef CAS PubMed
.
- J. Żółkiewicz, A. Marzec, M. Ruszczyński and W. Feleszko, Postbiotics-A Step Beyond Pre- and Probiotics, Nutrients, 2020, 12, 2189 CrossRef PubMed
.
- M. Yang, Y. Yang, Q. He, P. Zhu, M. Liu, J. Xu and M. Zhao, Intestinal Microbiota-A Promising Target for Antiviral Therapy?, Front. Immunol., 2021, 12, 676232 CrossRef CAS PubMed
.
- E. E. Blaak, E. E. Canfora, S. Theis, G. Frost, A. K. Groen, G. Mithieux, A. Nauta, K. Scott, B. Stahl, J. van Harsselaar, R. van Tol, E. E. Vaughan and K. Verbeke, Short chain fatty acids in human gut and metabolic health, Benef. Microbes, 2020, 11, 411–455 CrossRef CAS PubMed
.
- W. Yang, T. Yu, X. Huang, A. J. Bilotta, L. Xu, Y. Lu, J. Sun, F. Pan, J. Zhou, W. Zhang, S. Yao, C. L. Maynard, N. Singh, S. M. Dann, Z. Liu and Y. Cong, Intestinal microbiota-derived short-chain fatty acids regulation of immune cell IL-22 production and gut immunity, Nat. Commun., 2020, 11, 4457 CrossRef CAS PubMed
.
- C. R. Raetz and C. Whitfield, Lipopolysaccharide endotoxins, Annu. Rev. Biochem., 2002, 71, 635–700 CrossRef CAS PubMed
.
- R. Jerala, Structural biology of the LPS recognition, Int. J. Med. Microbiol., 2007, 297, 353–363 CrossRef CAS PubMed
.
- A. Ciesielska, M. Matyjek and K. Kwiatkowska, TLR4 and CD14 trafficking and its influence on LPS-induced pro-inflammatory signaling, Cell. Mol. Life Sci., 2021, 78, 1233–1261 CrossRef CAS PubMed
.
- L. Zhao, Q. Xie, S. Etareri Evivie, D. Liu, J. Dong, L. Ping, F. Liu, B. Li and G. Huo, Bifidobacterium dentium N8 with potential probiotic characteristics prevents LPS-induced intestinal barrier injury by alleviating the inflammatory response and regulating the tight junction in Caco-2 cell monolayers, Food Funct., 2021, 12, 7171–7184 RSC
.
- Y. Bian, Y. Dong, J. Sun, M. Sun, Q. Hou, Y. Lai and B. Zhang, Protective Effect of Kaempferol on LPS-Induced Inflammation and Barrier Dysfunction in a Coculture Model of Intestinal Epithelial Cells and Intestinal Microvascular Endothelial Cells, J. Agric. Food Chem., 2020, 68, 160–167 CrossRef CAS PubMed
.
- G. Wang, W. Sun, X. Pei, Y. Jin, H. Wang, W. Tao, Z. Xiao, L. Liu and M. Wang, Galactooligosaccharide pretreatment alleviates damage of the intestinal barrier and inflammatory responses in LPS-challenged mice, Food Funct., 2021, 12, 1569–1579 RSC
.
- Y. Li, S. Yang, J. Lun, J. Gao, X. Gao, Z. Gong, Y. Wan, X. He and H. Cao, Inhibitory Effects of the Lactobacillus rhamnosus GG Effector Protein HM0539 on Inflammatory Response Through the TLR4/MyD88/NF-κB Axis, Front. Immunol., 2020, 11, 551449 CrossRef CAS PubMed
.
- Y. C. Lu, W. C. Yeh and P. S. Ohashi, LPS/TLR4 signal transduction pathway, Cytokine, 2008, 42, 145–151 CrossRef CAS PubMed
.
- Y. Cui, Q. Wang, R. Sun, L. Guo, M. Wang, J. Jia, C. Xu and R. Wu, Astragalus membranaceus (Fisch.) Bunge repairs intestinal mucosal injury induced by LPS in mice, BMC Complement Altern. Med., 2018, 18, 230 CrossRef PubMed
.
- J. Xu, C. Lu, Z. Liu, P. Zhang, H. Guo and T. Wang, Schizandrin B protects LPS-induced sepsis via TLR4/NF-κB/MyD88 signaling pathway, Am. J. Transl. Res., 2018, 10, 1155–1163 CAS
.
- H. Y. Huang, Z. J. Zhang, C. B. Cao, N. Wang, F. F. Liu, J. Q. Peng, X. J. Ren and J. Qian, The TLR4/NF-κB signaling pathway mediates the growth of colon cancer, Eur. Rev. Med. Pharmacol. Sci., 2014, 18, 3834–3843 Search PubMed
.
- M. Xie, L. Zhang, L. Li, M. Fan and L. Hou, MiR-339 attenuates LPS-induced intestinal epithelial cells inflammatory responses and apoptosis by targeting TLR4, Genes Genomics, 2020, 42, 1097–1105 CrossRef CAS PubMed
.
- Y. Duan, Y. Wang, J. Zhang, Q. Liu and X. Ding, Morphologic, digestive enzymes and immunological responses of intestine from Litopenaeus vannamei after lipopolysaccharide injection, J. Invertebr. Pathol., 2018, 153, 186–194 CrossRef CAS PubMed
.
- Y. Gao, T. Han, C. Han, H. Sun, X. Yang, D. Zhang and X. Ni, Propofol Regulates the TLR4/NF-κB Pathway Through miRNA-155 to Protect Colorectal Cancer Intestinal Barrier, Inflammation, 2021, 44, 2078–2090 CrossRef CAS PubMed
.
- B. Sun, K. Xing, C. Qi, K. Yan and Y. Xu, Down-regulation of miR-215 attenuates lipopolysaccharide-induced inflammatory injury in CCD-18co cells by targeting GDF11 through the TLR4/NF-kB and JNK/p38 signaling pathways, Histol. Histopathol., 2020, 35, 1473–1481 CAS
.
- X. Zhao, B. Dong, M. Friesen, S. Liu, C. Zhu and C. Yang, Capsaicin Attenuates Lipopolysaccharide-Induced Inflammation and Barrier Dysfunction in Intestinal Porcine Epithelial Cell Line-J2, Front. Physiol., 2021, 12, 715469 CrossRef PubMed
.
- X. Dou, Z. Ma, D. Yan, N. Gao, Z. Li, Y. Li, X. Feng, L. Meng and A. Shan, Sodium butyrate alleviates intestinal injury and microbial flora disturbance induced by lipopolysaccharides in rats, Food Funct., 2022, 13, 1360–1369 RSC
.
- X. Wang, K. Xiao, C. Yu, L. Wang, T. Liang, H. Zhu, X. Xu and Y. Liu, Xylooligosaccharide attenuates lipopolysaccharide-induced intestinal injury in piglets via suppressing inflammation and modulating cecal microbial communities, Anim. Nutr., 2021, 7, 609–620 CrossRef CAS PubMed
.
- X. Chen, Q. Kong, X. Zhao, C. Zhao, P. Hao, I. Irshad, H. Lei, M. F. Kulyar, Z. A. Bhutta, H. Ashfaq, Q. Sha, K. Li and Y. Wu, Sodium acetate/sodium butyrate alleviates lipopolysaccharide-induced diarrhea in mice via regulating the gut microbiota, inflammatory cytokines, antioxidant levels, and NLRP3/Caspase-1 signaling, Front. Microbiol., 2022, 13, 1036042 CrossRef PubMed
.
- Y. Zhang, T. Mu, H. Jia, Y. Yang and Z. Wu, Protective effects of glycine against lipopolysaccharide-induced intestinal apoptosis and inflammation, Amino Acids, 2022, 54, 353–364 CrossRef CAS PubMed
.
- M. M. Vargas, R. Artigiani Neto and V. L. Sdepanian, Quantitative histology as a diagnostic tool for celiac disease in children and adolescents, Ann. Diagn. Pathol., 2022, 61, 152031 CrossRef PubMed
.
- S. F. Liao and M. Nyachoti, Using probiotics to improve swine gut health and nutrient utilization, Anim. Nutr., 2017, 3, 331–343 CrossRef PubMed
.
- L. T. Chwen, H. L. Foo, N. T. Thanh and D. W. Choe, Growth performance, plasma Fatty acids, villous height and crypt depth of preweaning piglets fed with medium chain triacylglycerol, Asian-Australas. J. Anim. Sci., 2013, 26, 700–704 CrossRef CAS PubMed
.
- Y. N. Min, H. L. Yang, Y. X. Xu and Y. P. Gao, Effects of dietary supplementation of synbiotics on growth performance, intestinal morphology, sIgA content and antioxidant capacities of broilers, J. Anim. Physiol. Anim. Nutr., 2016, 100, 1073–1080 CrossRef CAS PubMed
.
- W. Cheng, J. Lu, W. Lin, X. Wei, H. Li, X. Zhao, A. Jiang and J. Yuan, Effects of a galacto-oligosaccharide-rich diet on fecal microbiota and metabolite profiles in mice, Food Funct., 2018, 9, 1612–1620 RSC
.
- T. Paradis, H. Bègue, L. Basmaciyan, F. Dalle and F. Bon, Tight Junctions as a Key for Pathogens Invasion in Intestinal Epithelial Cells, Int. J. Mol. Sci., 2021, 22, 2506 CrossRef CAS PubMed
.
- C. Sturgeon and A. Fasano, Zonulin, a regulator of epithelial and endothelial barrier functions, and its involvement in chronic inflammatory diseases, Tissue Barriers, 2016, 4, e1251384 CrossRef PubMed
.
- L. W. Kaminsky, R. Al-Sadi and T. Y. Ma, IL-1β and the Intestinal Epithelial Tight Junction Barrier, Front. Immunol., 2021, 12, 767456 CrossRef CAS PubMed
.
- Y. Liu, X. Yu, J. Zhao, H. Zhang, Q. Zhai and W. Chen, The role of MUC2 mucin in intestinal homeostasis and the impact of dietary components on MUC2 expression, Int. J. Biol. Macromol., 2020, 164, 884–891 CrossRef CAS PubMed
.
- B. Allam-Ndoul, S. Castonguay-Paradis and A. Veilleux, Gut Microbiota and Intestinal Trans-Epithelial Permeability, Int. J. Mol. Sci., 2020, 21, 6402 CrossRef CAS PubMed
.
- Y. Liping, Y. U. Xinglin, Z. Chao, C. Pu and D. Xiaohua, Yajieshaba prevents lipopolysaccharide-induced intestinal barrier injuryanti-inflammatory and anti-apoptosis, J. Tradit. Chin. Med., 2022, 42, 707–714 Search PubMed
.
- R. Bhatia, S. Sharma, S. K. Bhadada, M. Bishnoi and K. K. Kondepudi, Lactic Acid Bacterial Supplementation Ameliorated the Lipopolysaccharide-Induced Gut Inflammation and Dysbiosis in Mice, Front. Microbiol., 2022, 13, 930928 CrossRef PubMed
.
- G. J. Feldman, J. M. Mullin and M. P. Ryan, Occludin: structure, function and regulation, Adv. Drug Delivery. Rev., 2005, 57, 883–917 CrossRef CAS PubMed
.
- W. T. Kuo, M. A. Odenwald, J. R. Turner and L. Zuo, Tight junction proteins occludin and ZO-1 as regulators of epithelial proliferation and survival, Ann. N. Y. Acad. Sci., 2022, 1514, 21–33 CrossRef CAS PubMed
.
- K. Takasawa, A. Takasawa, T. Akimoto, K. Magara, T. Aoyama, H. Kitajima, T. Murakami, Y. Ono, D. Kyuno, H. Suzuki and M. Osanai, Regulatory roles of claudin-1 in cell adhesion and microvilli formation, Biochem. Biophys. Res. Commun., 2021, 565, 36–42 CrossRef CAS PubMed
.
- M. Liu, B. Huang, L. Wang, Q. Lu and R. Liu, Peanut skin procyanidins ameliorate insulin resistance via modulation of gut microbiota and gut barrier in type 2 diabetic mice, J. Sci. Food Agric., 2022, 102, 5935–5947 CrossRef CAS PubMed
.
- R. Fu, L. Wang, Y. Meng, W. Xue, J. Liang, Z. Peng, J. Meng and M. Zhang, Apigenin remodels the gut microbiota to ameliorate ulcerative colitis, Front. Nutr., 2022, 9, 1062961 CrossRef PubMed
.
- A. Płóciennikowska, A. Hromada-Judycka, K. Borzęcka and K. Kwiatkowska, Co-operation of TLR4 and raft proteins in LPS-induced pro-inflammatory signaling, Cell. Mol. Life Sci., 2015, 72, 557–581 CrossRef PubMed
.
- J. Tang, L. Xu, Y. Zeng and F. Gong, Effect of gut microbiota on LPS-induced acute lung injury by regulating the TLR4/NF-kB signaling pathway, Int. Immunopharmacol., 2021, 91, 107272 CrossRef CAS PubMed
.
- H. Usuda, T. Okamoto and K. Wada, Leaky Gut: Effect of Dietary Fiber and Fats on Microbiome and Intestinal Barrier, Int. J. Mol. Sci., 2021, 22, 7613 CrossRef CAS PubMed
.
- Y. Qu, X. Li, F. Xu, S. Zhao, X. Wu, Y. Wang and J. Xie, Kaempferol Alleviates Murine Experimental Colitis by Restoring Gut Microbiota and Inhibiting the LPS-TLR4-NF-κB Axis, Front. Immunol., 2021, 12, 679897 CrossRef CAS PubMed
.
- L. Xu, Q. Huang, X. Tan, Q. Zhao, J. Wu, H. Liao, W. Ai, Y. Liu, Z. Lai and L. Fu, Patchouli alcohol ameliorates acute liver injury via inhibiting oxidative stress and gut-origin LPS leakage in rats, Int. Immunopharmacol., 2021, 98, 107897 CrossRef CAS PubMed
.
- L. Peng, X. Gao, L. Nie, J. Xie, T. Dai, C. Shi, L. Tao, Y. Wang, Y. Tian and J. Sheng, Astragalin Attenuates Dextran Sulfate Sodium (DSS)-Induced Acute Experimental Colitis by Alleviating Gut Microbiota Dysbiosis and Inhibiting NF-κB Activation in Mice, Front. Immunol., 2020, 11, 2058 CrossRef CAS PubMed
.
- L. Li, X. H. Zhang, G. R. Liu, C. Liu and Y. M. Dong, Isoquercitrin suppresses the expression of histamine and pro-inflammatory cytokines by inhibiting the activation of MAP Kinases and NF-κB in human KU812 cells, Chin. J. Nat. Med., 2016, 14, 407–412 CAS
.
- C. Ma, Y. Jiang, X. Zhang, X. Chen, Z. Liu and X. Tian, Isoquercetin ameliorates myocardial infarction through anti-inflammation and anti-apoptosis factor and regulating TLR4-NF-κB signal pathway, Mol. Med. Rep., 2018, 17, 6675–6680 CAS
.
- V. T. Pham, M. Calatayud, C. Rotsaert, N. Seifert, N. Richard, P. Van den Abbeele, M. Marzorati and R. E. Steinert, Antioxidant Vitamins and Prebiotic FOS and XOS Differentially Shift Microbiota Composition and Function and Improve Intestinal Epithelial Barrier In Vitro, Nutrients, 2021, 13, 1125 CrossRef CAS PubMed
.
- X. Liao, J. Zou, M. Wu, Y. Deng, J. Shi, Y. Hao, H. Deng and W. Liao, Hypoglycemic Effect of Nobiletin via Gut Microbiota-Metabolism Axis on Hyperglycemic Mice, Mol. Nutr. Food Res., 2023, 67, 2200289 CrossRef CAS PubMed
.
- N. Di Tommaso, A. Gasbarrini and F. R. Ponziani, Intestinal Barrier in Human Health and Disease, Int. J. Environ. Res. Public Health, 2021, 18, 12836 CrossRef CAS PubMed
.
- J. Xu, J. Song, Y. Zhang, Y. Wang, L. Yang, Y. Sha, B. Sun, N. You, X. Tian, R. Lin and Y. Wu, Jinzhi protects lipopolysaccharide-treated mice against mortality by repairing intestinal mucosal barrier damage and intestinal microecology, Biomed. Pharmacother., 2020, 123, 109749 CrossRef CAS PubMed
.
- J. Li, Y. Ma, X. Li, Y. Wang, Z. Huo, Y. Lin, J. Li, H. Yang, Z. Zhang, P. Yang and C. Zhang, Fermented Astragalus and its metabolites regulate inflammatory status and gut microbiota to repair intestinal barrier damage in dextran sulfate sodium-induced ulcerative colitis, Front. Nutr., 2022, 9, 1035912 CrossRef PubMed
.
- X. Ye, Y. Liu, J. Hu, Y. Gao, Y. Ma and D. Wen, Chlorogenic Acid-Induced Gut Microbiota Improves Metabolic Endotoxemia, Front. Endocrinol., 2021, 12, 762691 CrossRef PubMed
.
- C. Martin-Gallausiaux, L. Marinelli, H. M. Blottière, P. Larraufie and N. Lapaque, SCFA: mechanisms and functional importance in the gut, Proc. Nutr. Soc., 2021, 80, 37–49 CrossRef CAS PubMed
.
- H. Liu, J. Wang, T. He, S. Becker, G. Zhang, D. Li and X. Ma, Butyrate: A Double-Edged Sword for Health?, Adv. Nutr., 2018, 9, 21–29 CrossRef PubMed
.
- Y. Liu, W. Huang, S. Ji, J. Wang, J. Luo and B. Lu, Sophora japonica flowers and their main phytochemical, rutin, regulate chemically induced murine colitis in association with targeting the NF-κB signaling pathway and gut microbiota, Food Chem., 2022, 393, 133395 CrossRef CAS PubMed
.
- D. J. Morrison and T. Preston, Formation of short chain fatty acids by the gut microbiota and their impact on human metabolism, Gut Microbes, 2016, 7, 189–200 CrossRef PubMed
.
- M. Million, M. Maraninchi, M. Henry, F. Armougom, H. Richet, P. Carrieri, R. Valero, D. Raccah, B. Vialettes and D. Raoult, Obesity-associated gut microbiota is enriched in Lactobacillus reuteri and depleted in Bifidobacterium animalis and Methanobrevibacter smithii, Int. J. Obes., 2012, 36, 817–825 CrossRef CAS PubMed
.
- Y. G. Cao, S. Bae, J. Villarreal, M. Moy, E. Chun, M. Michaud, J. K. Lang, J. N. Glickman, L. Lobel and W. S. Garrett, Faecalibaculum rodentium remodels retinoic acid signaling to govern eosinophil-dependent intestinal epithelial homeostasis, Cell Host Microbe, 2022, 30, 1295–1310 CrossRef CAS PubMed
.
- T. Zhang, Q. Li, L. Cheng, H. Buch and F. Zhang, Akkermansia muciniphila is a promising probiotic, Microb. Biotechnol., 2019, 12, 1109–1125 CrossRef PubMed
.
- V. F. Rodrigues, J. Elias-Oliveira, S. Pereira Í, J. A. Pereira, S. C. Barbosa, M. S. G. Machado and D. Carlos, Akkermansia muciniphila and Gut Immune System: A Good Friendship That Attenuates Inflammatory Bowel Disease, Obesity, and Diabetes, Front. Immunol., 2022, 13, 934695 CrossRef CAS PubMed
.
- Q. Zhai, S. Feng, N. Arjan and W. Chen, A next generation probiotic, Akkermansia muciniphila, Crit. Rev. Food Sci. Nutr., 2019, 59, 3227–3236 CrossRef CAS PubMed
.
- T. Huang, H. Shi, Y. Xu and L. Ji, The gut microbiota metabolite propionate ameliorates intestinal epithelial barrier dysfunction-mediated Parkinson's disease via the AKT signaling pathway, NeuroReport, 2021, 32, 244–251 CrossRef CAS PubMed
.
- A. Loy, C. Pfann, M. Steinberger, B. Hanson, S. Herp, S. Brugiroux, J. C. Gomes Neto, M. V. Boekschoten, C. Schwab, T. Urich, A. E. Ramer-Tait, T. Rattei, B. Stecher and D. Berry, Lifestyle and Horizontal Gene Transfer-Mediated Evolution of Mucispirillum schaedleri, a Core Member of the Murine Gut Microbiota, mSystems, 2017, 2, e00171–e00116 CrossRef CAS PubMed
.
- S. Herp, A. C. Durai Raj, M. Salvado Silva, S. Woelfel and B. Stecher, The human symbiont Mucispirillum schaedleri: causality in health and disease, Med. Microbiol. Immunol., 2021, 210, 173–179 CrossRef PubMed
.
- R. Caruso, T. Mathes, E. C. Martens, N. Kamada, A. Nusrat, N. Inohara and G. Núñez, A specific gene-microbe interaction drives the development of Crohn's disease-like colitis in mice, Sci. Immunol., 2019, 4, S94 Search PubMed
.
|
This journal is © The Royal Society of Chemistry 2024 |