DOI:
10.1039/D3FO03288D
(Paper)
Food Funct., 2024,
15, 265-283
Whole grain germinated brown rice intake modulates the gut microbiota and alleviates hypertriglyceridemia and hypercholesterolemia in high fat diet-fed mice†
Received
10th August 2023
, Accepted 13th November 2023
First published on 14th November 2023
Abstract
Hyperlipidemia is a common clinical disorder of lipid metabolism in modern society and is considered to be one of the major risk factors leading to cardiovascular-related diseases. Germinated brown rice (GBR) is a typical whole grain food. The lipid-lowering effect of GBR has received increasing attention, but its mechanism of action is not fully understood. The gut microbiota has been proposed as a novel target for the treatment of hyperlipidemia. The aim of this study was to investigate the effects of GBR on the gut microbiota and lipid metabolism in high-fat diet (HFD)-fed C57BL/6J mice. The effect of GBR on hyperlipidemia was evaluated by measuring blood lipid levels and by pathological examination. The gut microbiota was detected by 16S rRNA sequencing, and the protein and mRNA expression levels involved in cholesterol metabolism were detected by western blotting and RT-qPCR to find potential correlations. The results showed that GBR supplementation could effectively reduce the levels of TC, TG, LDL-C and HDL-C in the serum and alleviate the excessive accumulation of fat droplets caused by HFD. Moreover, GBR intervention improved HFD-fed gut microbiota disorder via increasing the diversity of the gut microbiota, reducing the Firmicutes/Bacteroidetes ratio, and improving gut barrier damage. In addition, GBR could inhibit endogenous cholesterol synthesis and promote cholesterol transport and excretion. These findings suggest that GBR may be a competitive candidate for the development of functional foods to prevent abnormal lipid metabolism.
1 Introduction
Lipid metabolism disorders caused by excessive accumulation of body fat, especially hyperlipidemia, are among the major risk factors for cardiovascular-related diseases and have become a serious public health problem worldwide.1,2 Hyperlipidemia is characterized by high levels of total cholesterol (TC), triglycerides (TG), low-density lipoprotein cholesterol (LDL-C) and/or low levels of high-density lipoprotein cholesterol (HDL-C). It can increase intravascular blood viscosity and cause blood rheological changes.3,4 Many clinical and animal studies have shown that lipid accumulation due to hepatic steatosis plays a central role in the progression and pathogenesis of hyperlipidemia. Therefore, inhibiting hepatic steatosis by decreasing adipogenesis in liver tissue is an important part of alleviating hyperlipidemia. Since the absorption of cholesterol in the gut can transport chylomicron cholesterol to the liver, this absorption pathway has been considered an intervention target for drug treatment of hyperlipidemia. Therefore, lipid homeostasis can be maintained by reducing the absorption of cholesterol by the intestine and increasing the excretion of cholesterol. With the westernization of diet, a high fat diet is considered to be one of the most crucial factors in the frequent occurrence of hyperlipidemia worldwide. Humans are in the midst of a global epidemic of hyperlipidemia due to increased caloric intake in the diet and a lack of exercise.5–7 Although traditional lipid-lowering drugs can temporarily reduce lipid levels, they often produce adverse effects, such as liver dysfunction and gastrointestinal reactions. Therefore, it is critical to develop nondrug strategies such as nutrition interventions for the prevention and treatment of hyperlipidemia.
Accumulating evidence suggests that the gut microbiota is related to host lipid homeostasis and chronic metabolic diseases.8,9 The gut microbiota and its metabolites are involved in lipid and energy metabolism, immune regulation and oxidative stress in the host and could be potential new targets for hyperlipidemia.10 Over the past few decades, whole grain diets have been receiving pronounced attention. For example, the emergence of “germinate” whole grain products has significantly increased in the food and beverage market.11 Whole grain rice is rich in dietary fiber and various bioactive compounds that can not only inhibit starch digestion and prevent the rapid rise of blood glucose levels, but also regulate glucose and lipid metabolism.12 Germinated brown rice (GBR), notably, is a whole grain food with many phytochemicals and health benefits. The contents of γ-aminobutyric acid (GABA), phytosterol esters, γ-oryzanol, and antioxidants such as vitamin E, phenolic compounds, and other bioactive compounds generally increase during brown rice germination, representing a great alternative for the alleviation of hyperlipidemia.13–15 Moreover, GBR is widely recommended as a nutritional food choice compared with brown rice and refined white rice.16–18 Emerging literature confirms that ingestion of GBR is effective at protecting against cell proliferation and apoptosis and may provide important nutrients to exert health benefits.19 Meanwhile, GBR contains more functional phytochemicals than brown rice, which may endow GBR with excellent lipid-lowering activity.20–22 Furthermore, GBR could affect genetic programming in offspring rats to improve insulin sensitivity and alleviate HFD-fed metabolic disorders.23 Previous experiments in our laboratory showed that oryzanol (100 mg kg−1) administration can regulate the expression of genes in lipid metabolism, inflammatory responses, and improve obesity in HFD-fed mice.24 In addition, the supplementation of octacosanol reduced dyslipidemia in HFD-fed mice by regulating signaling pathways related to lipid metabolism.25 A recent study showed that GBR supplementation could attenuate hyperglycemia and insulin resistance in high fat diet-fed mice, meanwhile, GBR could also modulate the alterations of the gut microbiota.26 This suggests that GBR may ameliorate hyperglycemia by regulating the gut microbiota. However, the molecular mechanism is not clear. In addition, it is not clear if GBR can regulate cholesterol metabolism. Whether the regulation of cholesterol metabolism by GBR is also associated with the alteration of the gut microbiota needs further investigation.
Therefore, the objectives of this study were to: (1) investigate the effect of GBR on the phenotype, biochemical parameters, and inflammation in mice with HFD-fed hyperlipidemia, (2) to study the regulation of lipid metabolism by GBR, focusing on the reverse transport of cholesterol, and (3) to explore the detailed mechanism by which GBR alleviates hyperlipidemia via the gut microbiota. This study may provide a valuable basis for the prevention of hyperlipidemia and related metabolic diseases by whole grain GBR and promote its comprehensive utilization.
2 Materials and methods
2.1 Materials and reagents
GBR and refined rice were purchased from Jiangsu Haiao Biotechnology Co., Ltd and Nantong Fuzhikang Rice Industry Co., Ltd, respectively, and both of them were made from Oryza sativa L. subsp. japonica (Nanjing 9108). The ingredients are shown in Table S1.† Serum biochemical indices were detected using kits obtained from the Nanjing Jiancheng Institute of Biological Engineering (Nanjing, China). The bicinchoninic acid (BCA) protein assay kit was purchased from Elabscience Biotechnology Co., Ltd (Wuhan, China). The TRIzol reagent was provided by Tiangen Biotechnology Co., Ltd (Beijing, China). Real time-quantitative polymerase chain reaction (RT-qPCR) primer sequences were obtained from GeneCreate Biological Engineering Co., Ltd (Wuhan, China). Antibodies including β-actin (#58169), IL-6 (#12912), IL-1β (#12703), PCSK9 (#85813), HMGCR (#50081), anti-mouse (#58802), and anti-rabbit (#5151) were purchased from Cell Signaling Technology; antibodies including ZO-1 (sc-33725), claudin-1 (sc-166338), LDLR (sc-18823) and SREBP-2 (sc-271616) were purchased from Santa Cruz Biotechnology. CYP7A1 (K007769P) was purchased from Solarbio Technology Co., Ltd (Beijing, China). Analytical grade chemicals were purchased from Sinopharm Group Chemical Reagent Co., Ltd (Shanghai, China).
2.2 Animal experiments
Thirty healthy C57BL/6J male mice (7 weeks old, SPF class, weight 20–22 g) were obtained from Hunan Sleck Jingda Laboratory Animal Co., Ltd (Changsha, China). Prior to the experiment, all mice were placed in sterilized animal cages under standard conditions (12 h light/dark cycle; ambient temperature: 23 ± 2 °C; humidity: 50 ± 5%) with free access to commercial standard solid food and water. After one week of adaptive feeding, mice were randomly divided into three dietary groups. (1) normal diet (Con group, 4.46 kcal g−1), fed with a normal basal diet plus refined white rice, (2) high fat diet (HFD group, 5.21 kcal g−1), fed with a high fat diet plus refined white rice, and (3) HFD + GBR diet (HFD + GBR group, 5.21 kcal g−1), fed with a high fat diet plus GBR. The drinking water in the cages was changed every other day of the week. Food intake and body weight were checked twice weekly. The diets were all custom-made by Hunan Sleck Jingda Experimental Animal Co., Ltd (Changsha, China), with the specific composition shown in Table S2.† An oral glucose tolerance test (OGTT) was also performed during the last 7 days of treatment. Mice were fasted for 12 h and given 2 g per kg body weight of oral glucose administration. Blood samples were then collected from the tail vein at intervals of 0, 30, 60, 90 and 120 minutes. Glucose levels in blood samples from the tail vein were measured using a One touch glucose meter from Laifuscom Medical Equipment Co., Ltd (Shanghai, China). Glucose tolerance was expressed as the area under the glucose curve (AUC).
Prior to the execution of the mice, blood samples were collected from their orbital veins, placed in small tubes containing sodium heparin, and centrifuged at 3000 rpm for 15 minutes at 4 °C. The serum obtained was stored at −80 °C before further measurements. After the mice were sacrificed by cervical dislocation, the liver, spleen and adipose tissue of the epididymis were removed by dissection. The intestinal contents of each mouse were collected and temporarily placed in sterile vials for gut microbiota analysis. The liver and adipose tissues of mice were fixed with 10% paraformaldehyde for histopathological examination and observation. All experimental samples were temporarily frozen in liquid nitrogen and then transferred to a −80 °C refrigerator for storage for further analysis. All animals used in this study were cared for in accordance with the Ethics Committee of the Hunan Laboratory Animal Center (Ethical review number: IACUC-2021(5)069) and the review and approval of the National Laboratory Animal Welfare Guidelines (Ministry of Science and Technology of the People's Republic of China, 2006 No. 119).
2.3 Biochemical analysis of serum and the liver
Based on the standards and solutions given by the manufacturer, serum and liver biochemical parameters (including TC, TG, LDL-C, HDL-C, alanine transaminase (ALT), and aspartate aminotransferase (AST)) were determined following the instructions of commercialized kits provided by the Nanjing Jiancheng Institute of Biological Engineering (Nanjing, China). The activities of catalase (CAT), superoxide dismutase (SOD), glutathione peroxidase (GSH-Px) and malondialdehyde (MDA) were determined by the corresponding detection kit (Nanjing Jiengcheng Institute of Bioengineering, China). The data were expressed as U mg−1, U mg−1, U mg−1 and nmol mg−1 proteins of liver samples, respectively.
2.4 Histopathological observation of the liver and epididymal fat pad
To determine the effect of GBR on the histopathological changes of the liver and adipose tissue in the HFD-fed hyperlipidemia mice, hematoxylin and eosin (H&E) staining was performed. In brief, the liver and epididymal fat pad tissues were fixed with 10% (100 g L−1) formalin solution for 24 h, cut into 5 μm sections on a rotating microtome, fixed on clean slides, and dried overnight at 37 °C. Tissue sections were depowered twice in xylene for approximately 5–10 minutes, followed by dehydration in ethanol for 2–5 minutes and staining with H&E solution. Subsequently, the sections were examined using a DM6000B light microscope (Leica, Germany). Finally, the lesions of the liver and epididymal fat pad were evaluated using Case Viewer 2.4 software (3DHISTECH, Hungary).
2.5 Gut microbiota analysis
According to the manufacturer's instructions, a rapid DNA spin extraction kit (MP Biomedicals, Santa Ana, CA, USA) was used to extract total bacterial DNA from gut contents. Then, the purity and size of DNA was evaluated by 1% agarose gel electrophoresis. The V3–V4 variable region of the 16S rRNA gene extracted from the gut content was amplified on the GeneAmp 9700 PCR system (American Applied Biosystems) using primers 338F (5′-ACTCCTACGGGAGGCAGCA-3′) and 806R (5′-GGACTACHVGGGTWTCTAAT-3′). PCR analysis was performed using the following thermal cycling program: denaturation at 95 °C for 3 minutes; denaturation at 95 °C for 30 seconds, annealing at 55 °C for 30 seconds, and extension at 72 °C for 45 seconds, totaling 27 cycles; the last step is the extension at 72 °C for 10 minutes. PCR amplicons were purified using the AxyPrep DNA gel extraction kit (Axygen, USA) and quantified using the Quanti Fluor™-ST fluorometer (Promega, USA). The TruSeqTM DNA sample prep kit was used for Miseq library construction. The RDP classifier Bayesian algorithm (version 2.11) was used to cluster OTUs (operational taxonomic units), whose similarity cutoff point was greater than 97% in the 16S rRNA sequence database of SILVA and GenBank. Sequencing and bioinformatics analysis of all DNA samples were performed on the Illumina MiSeq platform (Illumina, San Diego, California, USA).
2.6 Processing of sequencing data and analysis
The online Majorbio cloud platform (https://cloud.majorbio.com) was used to analyze sequencing data. Fastp software (version 0.19.6) was used for quality control of the samples. Based on the 16S rRNA sequence, the community phylogeny survey using unobserved state reconstruction (PICRUSt) analysis was used to predict the metabolic characteristics of the bacterial community. Pathway enrichment analysis were performed by the Kyoto Encyclopedia of Genes and Genomes (KEGG) (https://www.genome.jp/kegg/pathway.html). Spearman analysis was used to calculate the correlation between the genus level and the abundance of the three groups of the gut microbiota. The presented data are expressed as the mean ± standard deviation, and p < 0.05 was considered to be a statistically significant difference.
2.7 Real time quantitative polymerase chain reaction (RT-qPCR)
According to the relative expression levels of lipid metabolism-related genes, the lipid-lowering mechanism of GBR was further explored at the mRNA level. Total RNA was extracted from the liver tissue with Transzol Up (Transgen, China) under liquid nitrogen cooling in a reaction system containing total RNA, RNase-free ddH2O, 4 μL of 4 × gDNA wiper mix and 4 μL of 5 × HiScript II qRT SuperMix II. A NanoDrop (2000 C) spectrophotometer (Thermo, USA) was used to measure the RNA concentration and its integrity was checked by standard denaturing agarose gel electrophoresis (Bio-Rad, USA). Then, high-capacity cDNA reverse transcription was performed using the GeneAmp* PCR system 9700 (Applied Biosystems, USA) at 50 °C and 85 °C for 15 min and 5 s. RT-qPCR amplification experiments were performed using the CFX96™ real-time system (Bio-Rad, USA) in a 20 μL reaction mixture containing 1 μL of reverse transcribed cDNA, 10 μL of 2× TransStart Green qPCR SuperMix UDG mixture, 0.5 μL forward primer, 0.5 μL reverse primer, and 8 μL RNase-free water. The thermal cycling conditions were as follows: pre-denaturation at 94 °C for 3 minutes, denaturation at 94 °C for 30 seconds, annealing at 60 °C for 40 seconds, extension at 72 °C for 1 minute, and 42 cycles. These steps were repeated three times for each sample. The β-actin mRNA signal was used to normalize the relative expression levels of target mRNAs. Experiments were performed three times on a single plate and the relative quantification was calculated using the 2−ΔΔCt method. The gene sequence was first searched in the NCBI database (https://www.ncbi.nlm.nih.gov/qh), and Primer 6.0 software was used to complete the design of gene primers, as shown in Table S3.†
2.8 Western blotting analysis
500 μL of RIPA cell lysis buffer (Dingguo Biotechnology, Shanghai, China) containing 100 mM phenylmethanesulfonyl fluoride (Beyotime Technology, Shanghai, China), an EDTA-free protease inhibitor cocktail (ABclonal Technology, Wuhan, China) and PhosSTOP (Sigma-Aldrich, USA) was added to the liver tissue powder after grinding using liquid nitrogen and shaken and mixed. It was kept on ice for 30 minutes to allow sufficient lysis and then centrifuged at 13
000 rpm for 15 minutes at 4 °C. The extracted proteins were then quantified in concentration using the BCA protein assay kit (Tiangen Biotechnology, Beijing, China) to ensure equal protein content in each sample and separated by sodium dodecyl sulfate-polyacrylamide gel electrophoresis (SDS-PAGE). After complete separation, proteins in the gel were transferred to Immobilon®-P polyvinylidene fluoride (PVDF) membranes (0.45 μm, Millipore, Ireland) and then blocked at room temperature with 5% albumin bovine V (Solarbio® Life Science, Beijing, China) for 1 h with gentle shaking. These membranes were incubated overnight at 4 °C with the corresponding primary antibody solution. Subsequently, the membranes were washed three times for 10 minutes each with Tris-buffered saline containing 0.1% Tween 20 (TBST, pH 7.5) and incubated with horseradish peroxidase-conjugated anti-rabbit, anti-mouse or anti-goat IgG antibodies for 1 h at 25 °C, and then visualized with the Clarity western ECL substrate (Bio-Rad). The membranes were imaged using the ChemiDoc™ XRS+ imaging system (Bio-Rad. USA) after picture acquisition. Relative protein levels were quantified using Image Lab™ software version 6.1.
2.9 Statistical analysis
All experimental data were expressed as mean ± standard deviation (S.D.). One-way analysis of variance (ANOVA) for multiple comparisons followed by Tukey's test was used to determine the significance of differences between groups with SPSS22.0 software (SPSS Inc., Chicago, USA). The correlation between two variables was determined by Spearman's correlation. The difference was considered to be statistically significant at *: p value < 0.05 and **: p value < 0.01.
3 Results
3.1 Effects of GBR supplementation on alleviating HFD-fed weight parameters and adiposity
In this study, an HFD-fed mouse model was used to investigate the potential effects of whole grain GBR supplementation on hyperlipidemia. The change curve of body weight during the 10-week period is shown in Fig. 1A. During the experimental period, the initial body weights of the three groups of mice were similar, but the final body weights were different. The HFD + GBR group showed a relative reduction in body weight of 2.24 ± 0.08 g compared to the HFD group, suggesting that the intake of whole grain GBR could reduce body weight gain in the HFD-fed mice (Fig. 1B). However, there was no significant change in food intake between the HFD group and the HFD + GBR group throughout the HFD-fed period, while a significant difference existed between the HFD group and the Con group (p < 0.05) (Fig. 1C). In addition, no significant difference was observed in energy intake between the three groups (Fig. 1D). The food efficacy ratio of the mice in the HFD group was significantly higher than that in the Con group, whereas the food efficacy ratio of the mice in the HFD + GBR group was lower than that in the HFD group, and significant differences were observed (Fig. 1E). These results showed that GBR supplementation could reduce adiposity and decrease body weight gain in the HFD-fed mice.
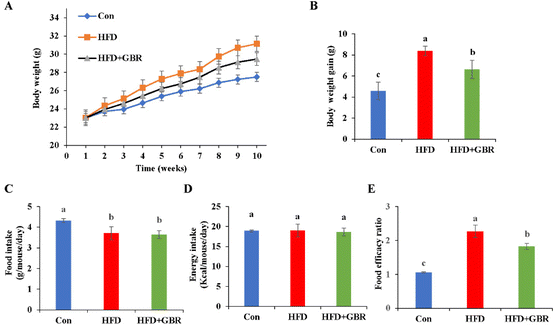 |
| Fig. 1 Effects of GBR on the body weight growth curve, body weight gain, food intake, energy intake and the food efficacy ratio of different groups. (A) Bodyweight change of mice during 10 weeks; (B) body weight gain of mice at the end of feeding; (C) food intake; (D) energy intake; and (E) food efficacy ratio; data are expressed as mean ± SD, Con: control, GBR: germinated brown rice; HFD: high fat diet; the mean value with different letters indicates significant differences (p < 0.05). n = 10. | |
3.2 Effect of GBR supplementation on alleviating HFD-fed hyperlipemia and glucose tolerance
Blood samples were collected from mice to measure serum lipid-related markers, including TC, TG, LDL-C and HDL-C. As shown in Fig. 2A–F, the serum TC and TG concentrations of the HFD group were 1.89 and 1.42 fold higher than those of the Con group. Notably, LDL-C was positively associated with the risk of cardiovascular disease and is considered to be a major causative factor in atherosclerosis. In contrast, HDL cholesterol was negatively associated with the risk of cardiovascular disease. LDL-C concentrations in the control, HFD and HFD + GBR groups were 0.33 ± 0.02 mmol L−1, 0.51 ± 0.04 mmol L−1 and 0.44 ± 0.02 mmol L−1, respectively, and HDL-C concentrations in the same groups were 1.87 ± 0.07 mmol L−1, 1.36 ± 0.14 mmol L−1, and 2.24 ± 0.16 mmol L−1, respectively. The HFD group significantly increased the LDL-C concentration, non-HDL-C concentration, and the basal risk ratio (LDL-C/HDL-C) values and decreased the concentration of HDL-C compared to the Con group. Compared with the HFD group, GBR supplementation significantly reduced LDL-C concentrations, non-HDL-C and the LDL-C/HDL-C ratio (p < 0.05) and increased HDL-C concentrations (p < 0.05) in the hyperlipidemic model mice. We also compared the atherosclerosis index (AI) of the three diets. The results showed that AI in the HFD group was significantly higher than that in the Con group or the HFD + GBR group, indicating that GBR significantly reduced the HFD-induced AI (Fig. 3G). These findings suggest that GBR significantly down-regulated serum TC, TG and LDL-C levels and improved serum cholesterol distribution and HFD-induced hyperlipidemia. Furthermore, as shown in Fig. 3H and I, blood glucose in HFD mice was significantly higher within 30 minutes after oral glucose administration, while blood glucose levels were significantly lower in the HFD + GBR treatment group than in the HFD group. After 120 minutes of oral glucose administration, the blood glucose level and AUC in the HFD group were higher than those in the Con group, and the blood glucose level in the HFD + GBR group decreased to 13.62 ± 1.11 mmol L−1, which was lower than that in the HFD group. The results indicated that whole grain GBR significantly improved the glucose tolerance of the HFD-fed mice.
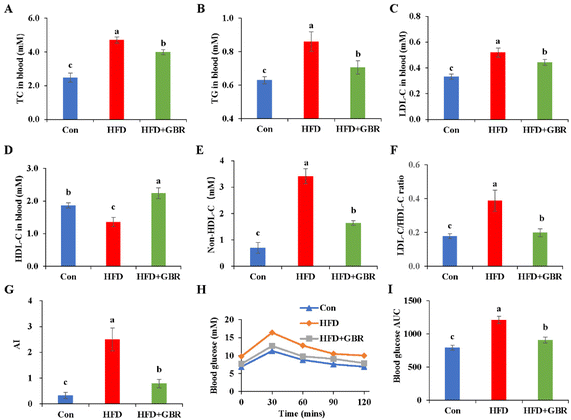 |
| Fig. 2 Effects of GBR on the serum lipid profile and blood glucose parameters of different groups. (A) Concentration of TC; (B) concentration of TG; (C) concentration of LDL-C; (D) concentration of HDL-C; (E) concentration of non-HDL-C; (F) the ratio of LDL-C/HDL-C; (G) the atherosclerosis index (AI); (H) blood glucose; and (I) area under the curve (AUC) for postprandial glucose. Non-HDL-C = TC − HDL-C; AI, atherosclerosis index = (TC − HDL-C)/HDL-C; data are expressed as mean ± SD. The mean value with different letters indicates significant differences (p < 0.05). n = 10. | |
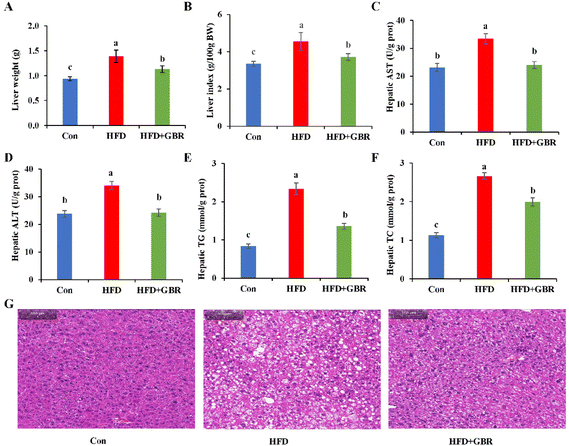 |
| Fig. 3 Effects of GBR on hepatic steatosis and liver biochemical parameters of different groups of mice. (A) Liver weight; (B) liver index; (C) hepatic AST; (D) hepatic ALT; (E) hepatic TG; (F) hepatic TC; and (G) H&E staining images of representative hepatic tissues of different groups. Data are expressed as mean ± SD. The mean value with different letters indicates significant differences (p < 0.05), n = 3. | |
3.3 Effects of GBR on regulating hepatic steatosis and injury in HFD-fed mice
After GBR intervention for 10 weeks, the liver weight of the HFD group increased by 31.79% compared with that of the Con group, while in the HFD + GBR group, these weight increases were reduced (Fig. 3A). Similarly, the liver index normalized to 100 g of body weight was significantly higher in the HFD group than in the Con group. In contrast, the liver index was significantly lower in the HFD + GBR group than in the HFD group (Fig. 3B). Liver biochemical indexes including AST, ALT, TC and TG were significantly increased in the HFD group (p < 0.05). As expected, the HFD + GBR group showed significantly decreased hepatic AST, ALT, TC and TG levels (p < 0.05) (Fig. 3C–F). The data indicated that GBR supplementation improved HFD-induced hepatic steatosis. At the end of the experimental period, the effects of GBR on liver morphology were examined. As shown in Fig. 3G, liver tissue from all mice showed no evidence of hepatitis, hemorrhage, or perivenular fibrosis. Normal hepatocytes had abundant cytoplasm, showed normal central veins, had round nuclei, and showed homogeneous staining of the cytoplasm. In the HFD group, there was typical fat vacuolation and hepatic steatosis with more lipid droplet storage between hepatocytes. As expected, the HFD + GBR group showed a significant reduction in cyclic lipid droplets in the cytoplasm after treatment and the morphology of the hepatocytes was similar to that of the Con group. In short, GBR supplementation significantly prevented high-fat diet-induced hepatic steatosis and attenuated liver fat accumulation, which can have a positive effect on hyperlipidemia.
3.4 Effects of GBR supplementation on adipose tissues in the HFD-fed mice
As shown in Fig. 4A, the epididymal fat pad weight of the HFD group increased by 58.58% compared with that of the Con group, while in the HFD + GBR group, the epididymal fat pad weight was reduced. The epididymal fat index was significantly higher in the HFD group than in the Con group. In contrast, the epididymal fat index was significantly lower in the HFD + GBR group than in the HFD group (Fig. 4B). The results of RT-qPCR analysis showed that after supplementation with GBR for 10 weeks, the gene level of leptin was increased significantly when compared with the Con group, while a significant decrease in the level of adiponectin was observed. In contrast, the HFD + GBR group had a significantly down-regulated leptin level and an up-regulated adiponectin level compared with the HFD group (p < 0.05) (Fig. 4C and D). No difference was observed in the level of resistin (Fig. 4E). Moreover, the H&E staining results of adipose tissue are shown in Fig. 4F. The morphology of adipose tissue in the HFD group was significantly larger in adipocyte diameter compared to that in the Con group. Fortunately, the GBR intervention alleviated the damage to the adipose tissue in the mice and the cells were more regularly arranged. The morphology of adipose tissue in the HFD + GBR group was closer to that in the Con group.
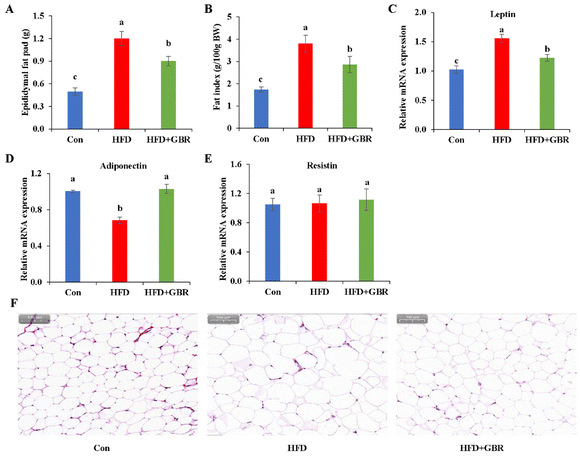 |
| Fig. 4 Effects of GBR on fat accumulation and histopathology of fat tissue in the HFD-fed mice. (A) Epididymal fat pad weight; (B) fat index; relative mRNA expression of (C) leptin, (D) adiponectin, and (E) resistin; and (F) H&E staining images of representative adipose tissues of different groups. Data are expressed as mean ± SD. The mean value with different letters indicates significant differences (p < 0.05). | |
3.5 Effects of GBR on hepatic inflammation and oxidative stress in the HFD-fed mice
Oxidative stress and inflammation are important indicators of liver damage in mice. As per our results, the HFD group significantly decreased liver SOD (p < 0.05), CAT (p < 0.05), and GSH-Px (p < 0.05) and increased the liver MDA content compared with those in the Con group. In contrast, after GBR intervention for 10 weeks, these liver oxidation markers were reversed (Fig. 5). In addition, compared with the HFD group, the HFD + GBR group significantly down-regulated the expression levels of inflammation factors and markedly up-regulated the expression levels of antioxidant genes. These results suggested that GBR intervention increased the antioxidant capacity and reduced inflammation and oxidative stress in the mouse liver.
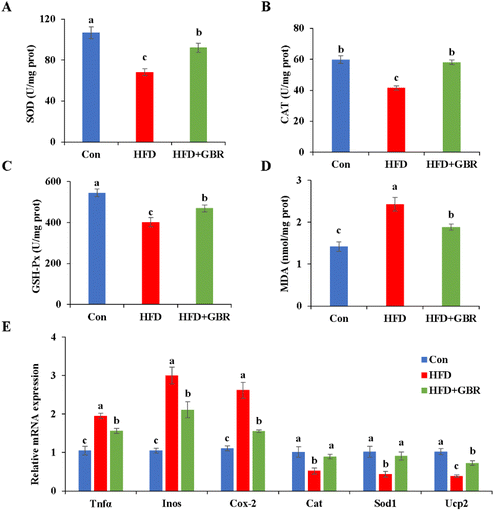 |
| Fig. 5 Effects of GBR on HFD-induced oxidative stress and inflammation in the liver of mice. (A) Hepatic SOD level; (B) hepatic CAT level; (C) hepatic GSH-Px activity; (D) hepatic MDA level; and (E) relative mRNA expression levels of genes related to inflammation and oxidative stress. Data are expressed as mean ± SD. The mean value with different letters indicates significant differences (p < 0.05), n = 6. | |
3.6 Effects of GBR on lipid and cholesterol metabolism in the HFD-fed mice
To elucidate the mechanism by which GBR supplementation ameliorates HFD-induced hyperlipidemia, we used RT-qPCR to detect liver mRNA expression levels associated with lipid metabolism. Compared with the HFD group, the HFD + GBR group significantly down-regulated the expression levels of adipogenesis genes (Acc1, Fasn, Dgat, Srebp1c, Cd36 and Pparγ) and up-regulated lipolysis genes (Atgl and Hsl) (Fig. 6A). Moreover, the genes involved in fatty acid oxidation (Pparα, Cpt1, Acox1 and Pdk4) and mitochondria-associated genes (Nrf1, Tfam, Sirt1 and Pgc1α) were significantly up-regulated in the HFD + GBR group compared with those in the HFD group (Fig. 6B and C).
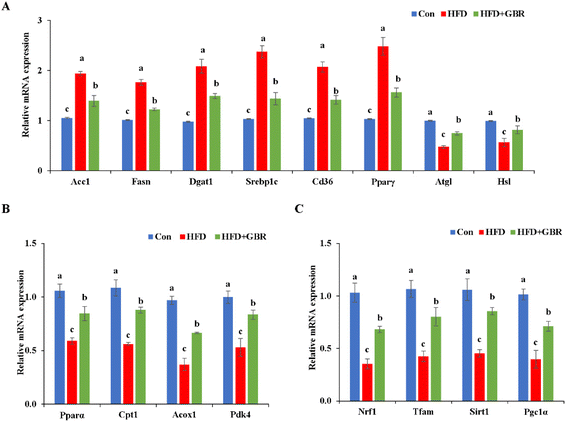 |
| Fig. 6 Effects of GBR on the expression levels of lipid metabolism-related genes in hepatic tissues. Relative mRNA expression levels of (A) genes related to lipogenesis and lipolysis, (B) genes related to fatty acid oxidation, and (C) genes related to mitochondria are shown. Data are expressed as mean ± SD. The mean value with different letters indicates significant differences (p < 0.05), n = 6. | |
Cholesterol metabolism is a complex process that requires the involvement of multiple transporter proteins and other proteins. Therefore, the expression of key genes for cholesterol absorption in the mouse liver was determined to reveal the potential mechanism by which GBR alleviates hyperlipidemia. At the protein level, compared with the Con group, the HFD group increased the protein expression levels of sterol regulatory element binding protein-2 (SREBP-2), proprotein convertase subtilisin/kexin type 9 (PCSK9) and 3-hydroxy-3-methylglutaryl coenzyme a reductase (HMGCR), and decreased the protein expression levels of the low density lipoprotein receptor (LDLR) and cytochrome P450 7A1 (CYP7A1). As expected, the HFD + GBR group reversed the HFD-induced changes in protein expression (Fig. 7A and B). At the mRNA expression level, the expression levels of Pcsk9, Hmgcr, Srebp-2 and apolipoprotein B (Apob) were significantly increased in the HFD group compared to those in the Con group, indicating the presence of impaired gut cholesterol absorption in the HFD-fed mice. Fortunately, the GBR intervention triggered a significant recovery of these genes’ expression compared to the HFD group. In addition, the expression levels of ATP binding cassette transporter a1 (Abca1), Ldlr, cyp7a1 and liver X receptor alpha (LXRα) were significantly higher in the HFD + GBR group compared to those in the HFD group, indicating that these cholesterol-related genes were affected by the HFD + GBR group (Fig. 7C). These results suggested that GBR supplementation could promote cholesterol excretion in mice by inhibiting cholesterol absorption and enhancing cholesterol transport in the small intestine, thus exerting potential anti-hyperlipidemia properties.
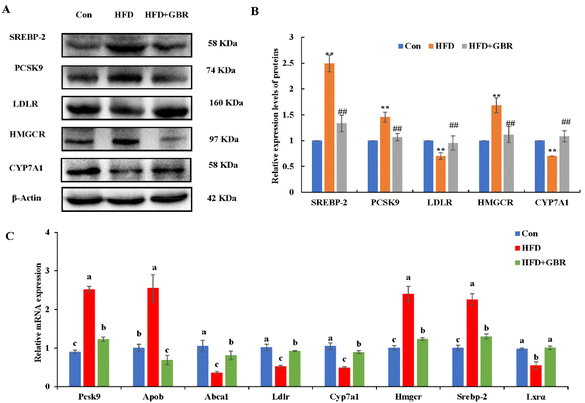 |
| Fig. 7 Effect of GBR on the expression levels of cholesterol metabolism in the hepatic tissues of mice. (A) Representative images of cholesterol metabolism-related proteins (n = 3) and (B) quantification of cholesterol metabolism-related proteins; data are expressed as mean ± SD. **p < 0.01 compared with the Con group; ##p < 0.01 compared with the HFD group; and (C) relative mRNA expression of cholesterol metabolism-associated genes examined by RT-qPCR. Data are expressed as mean ± SD. Different letters in bars indicate a significant difference (p < 0.05). | |
3.7 Effects of GBR on the diversity of the gut microbiota
To assess the significance of the gut microbiota in the regulation of hyperlipidemia in vivo, the effect of GBR on the gut microbiota was explored using 16S rRNA amplicon sequencing analysis. The number of optimized sequences was 911
630, the number of bases was 384
000
629 (bp), and the average length was 421, as shown in Table S4.† The Pan/Core curve was used to describe the changes in the total number of species and core species as the sample size increased. It can be seen from the figure that the curve tends to be smooth, indicating that the sample size met the experimental requirements (Fig. 8A and B). The rank-abundance curve was used to analyze the richness and uniformity of species. It can be seen from the figure that the curve decreased smoothly, indicating that the species diversity of the sample was higher and the amount of sequencing data was adequate (Fig. 8C). Alpha diversity allows the analysis of species diversity in a sample to assess species richness and evenness. As shown in Fig. 8D–H, the results of α-diversity analysis including the Sob, Shannon, ACE and Chao indices showed that HFD significantly reduced the diversity and richness of the intestinal microbiota in mice compared with the Con group. However, GBR supplementation produced strong improvement effects by upregulating the Sob, Shannon, ACE and Chao indices, thereby restoring microbial diversity and richness. In addition, the use of principal component analysis (PCA) to monitor the similarities and differences in the composition of the three groups gave us a preliminary understanding of the overall structure of the gut microbiota. As shown in Fig. 9A, the samples of the three groups were mainly scattered in three clusters. Samples from the HFD + GBR group were closer to the Con group than samples from the HFD group. To assess how the β-diversity of the gut microbiota changed between groups, principal coordinate analysis (PCoA) based on an unweighted Unifrac distance matrix, non-metric multidimensional scaling (NMDS), and partial least squares discriminant analysis (PLS-DA) were performed (Fig. 9B–D). In addition, after base optimization of the raw sequencing data, a high-quality sequence dataset of 384
000
629 bases was obtained. The sequence is 911
630 bases long, with an average length of 421 bases. Sequences with similarity greater than 97% were classified as one OTU, and 545 OTUs were obtained for all sequences. The Venn diagram showed 504, 433, and 485 OTUs in the Con, HFD and HFD + GBR groups, respectively, with 388 common OTUs (Fig. 9E). These results indicated that compared with the Con group, the HFD group reduced the diversity of the community, but the HFD + GBR group increased the richness of the community.
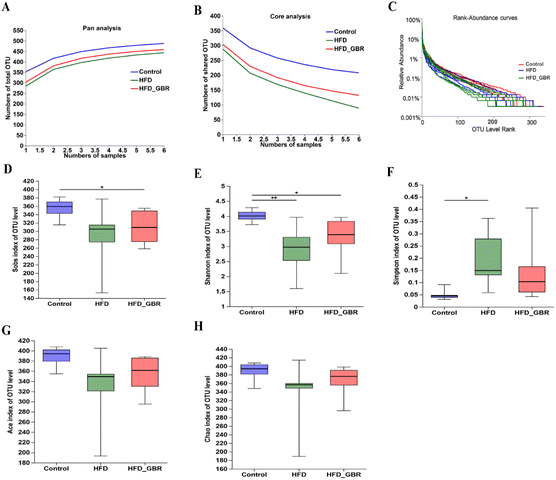 |
| Fig. 8 Effects of GBR on species analysis and the gut microbial alpha diversity of different groups. (A) Pan species analysis; (B) core species analysis; (C) rank-abundance curves; (D) Sob index; (E) Shannon index; (F) Simpson index; (G) Ace index; and (H) Chao index. The figure shows the significant difference between the two selected groups of samples, and marks the two groups with significant differences (p < 0.05 is marked as *, and p < 0.01 is marked as **). The abscissa is the group name, and the ordinate is the index average of each group, n = 6. | |
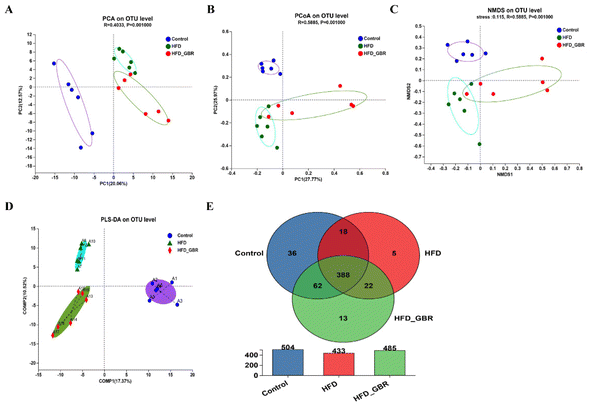 |
| Fig. 9 Effects of GBR on the gut microbial beta diversity and the Venn diagram of different groups. (A) Principal component analysis (PCA); (B) principal co-ordinate analysis (PCoA); (C) non-metric multidimensional scaling (NMDS); (D) partial least squares discriminant analysis (PLS-DA) of all groups by the weighted UniFrac distance matrix based on the OUT level; (E) Venn diagram. The Venn pie chart shows the percentage of the number of species jointly owned by the three groups to the total number of species. It can be seen from the Venn diagram that the three groups collectively have 388 species, and the HFD + GBR group has 13 unique species, n = 6. | |
3.8 Effect of GBR on the composition of the gut microbiota
To assess the overall composition of bacterial communities in different groups, particularly taxa that responded to GBR supplementation, we analyzed the degree of bacterial taxonomic similarity at the phylum, order and genus levels. At the phylum level, as seen in Fig. 10A, Firmicutes, Bacteroidetes and Desulfobacterota were the dominant phyla in all samples, accounting for over 90% of the total bacteria. Compared with the Con group, the HFD group had a higher relative abundance of the Firmicutes and Actinobacteria phyla and a lower relative abundance of the Arabidopsis and Desulfobacterota phyla. The GBR supplementation resulted in a 12.64% and 33.17% decrease in the abundance of Firmicutes and Actinobacteriota, respectively, and a 27.96% and 80.45% increase in the abundance of Bacteroidetes and Desulfobacterota, respectively (Fig. 10B–E). In addition, the Firmicutes/Bacteroidetes (F/B) ratio was higher in the HFD group (1.93 ± 0.02) than in the Con group (0.93 ± 0.05). GBR supplementation reversed the HFD-induced increase in Firmicutes and slightly increased the relative abundance of Bacteroidetes, with a significant 37.35% reduction in F/B values compared to the HFD group (Fig. 10F). The F/B ratio is an indicator associated with metabolic disorders, and GBR supplementation reversed this trend compared with the HFD group. At the order level, the abundance of Bacteroidales, Lachnospirales and Oscillospirales was significantly reduced in the HFD group compared with that in the Con group, and was somewhat alleviated by GBR supplementation. Similarly, the relative abundance of Erysipelotrichales and Peptostreptococcales in the HFD group was significantly higher than that in the Con group, and the abundance of both was reduced relative to the HFD group after GBR supplementation (Fig. 11A–F). At the genus level, Norank_F_Muribaculaceae, Lactobacillus, Lachnospiraceae_NK4A136, Faecalibaculum, and Desulfovibrio were the dominant genera in the gut microbiota of mice. Compared to the Con group, the abundance of Norank_F_Muribaculaceae, Lachnospiraceae_NK4A136_group, and Romboutsia was significantly increased in HFD mice, whereas Desulfovibrio, unclassified_f_lachnospiraceae, and unclassified_f_lachnospiraceae (Firmicutes) and Turicibacter (Turicibacteraceae) in the HFD group may have been identified as the most important biomarkers of the gut microbiota. Fortunately, the HFD + GBR group reversed the transformation induced by HFD. Among them, Norank_F_Muribaculaceae, Faecalibaculum, unclassified_f_lachnospiraceae, Norank_f_lachnospiraceae and Romboutsia became the dominant genera, which were not significantly different from the Con group (p < 0.05) (Fig. 12A–D). Furthermore, the LEfSe analysis (LDA threshold of 2) revealed that Erysipelotrichaceae and Atopobiaceae were significantly enriched in samples from the HFD group compared to the Con group. In addition to Ruminococcaceae and Enterobacteriaceae, f-norank_o_RF39 and Erysipelatoclostridiaceae were enriched in the Con group, while the HFD + GBR group samples were enriched with Staphylococcales, Rikenellaceae and Prevotellacea (Fig. S1†). These findings suggest that GBR restores HFD-induced disturbances in the gut microbiota composition of mice and promotes the proliferation of commensal bacteria. The KEGG functional predictions showed that genes primarily associated with amino acid metabolism, glucolipid metabolism, biosynthesis of other secondary metabolites, and energy metabolism, especially those related to bile acids and cholesterol, accounted for the highest abundance (Fig. S2†). In short, the results suggested that GBR supplementation could modulate the HFD-induced gut dysbiosis.
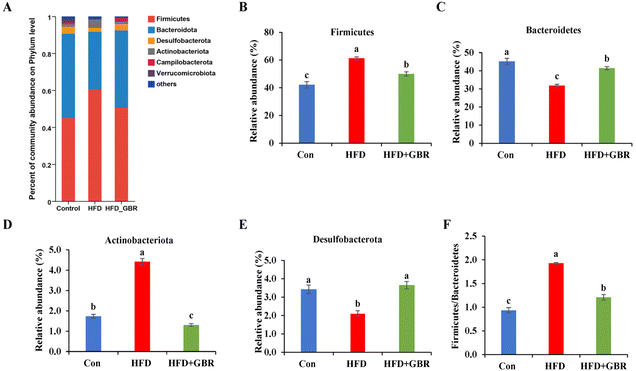 |
| Fig. 10 The effect of GBR on the community abundance at the phylum level of different groups. (A) Relative abundance at the phylum level; (B) Firmicutes abundance; (C) Bacteroidetes abundance; (D) Actinobacteriota abundance; (E) Desulfobacterota abundance; and (F) the ratio of Firmicutes to Bacteroidetes (F/B). Data are expressed as mean ± SD. Different letters in bars indicate a significant difference (p < 0.05), n = 6. | |
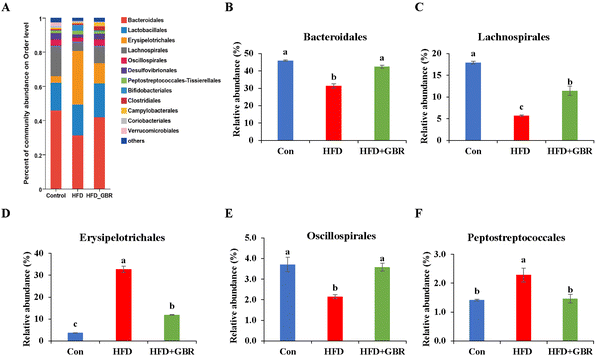 |
| Fig. 11 The effect of GBR on the community abundance at the order level of different groups. (A) Relative abundance at the order level; (B) Bacteroidales abundance; (C) Lachnospirales abundance; (D) Erysipelotrichales abundance; (E) Oscillospirales abundance; and (F) Peptostreptococcales abundance. Data are expressed as mean ± SD. Different letters in bars indicate a significant difference (p < 0.05), n = 6. | |
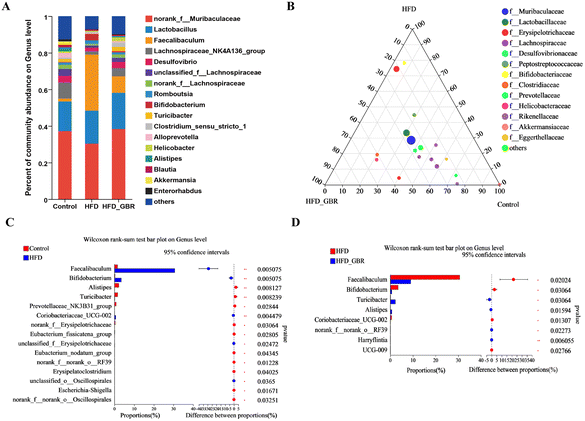 |
| Fig. 12 The effect of GBR on the community abundance at the genus level of different groups. (A) Relative abundance at the genus level; (B) ternary phase diagram; (C) Con vs. HFD at the genus level; and (D) HFD vs. HFD + GBR at the genus level, n = 6. | |
3.9 GBR alleviated gut barrier damage and inflammation
The gut barrier is an important part for maintaining intestinal homeostasis. ZO-1 and claudin-1 proteins are important proteins to maintain intestinal mucosal permeability, which can prevent harmful substances such as endotoxin from passing through the intestinal barrier into the blood circulation system. As shown in Fig. 13A, the protein expression levels of ZO-1 and claudin-1 were significantly reduced in the colon tissue of the HFD group compared with those in the Con group, indicating that HFD could cause increased gut permeability. However, the HFD + GBR group significantly increased the protein expression levels of ZO-1 and claudin-1 in the colon tissue of mice, suggesting that GBR supplements can repair the gut barrier and reduce gut permeability in the HFD-fed mice. Moreover, the HFD + GBR group significantly decreased the expression levels of IL-6 and IL-1β proteins (Fig. 13B), indicating that GBR could reduce gut inflammation in the HFD-fed mice. In addition, compared with the HFD group, the mRNA expression levels of ZO-1 and claudin-1 in colon tissue were significantly increased, while IL-6 and IL-1β mRNA expression levels were significantly decreased after 10 weeks of GBR intervention (Fig. 13C). These results indicated that GBR supplementation could protect the gut barrier and reduce intestinal inflammation in the HFD-fed mice.
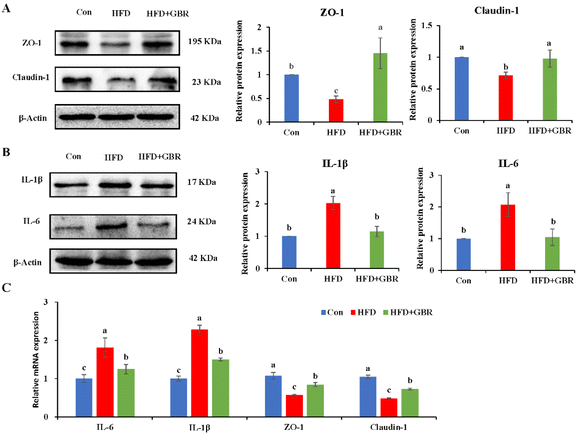 |
| Fig. 13 Effect of GBR on the gut barrier and inflammation factors of different groups. (A) Western blot analysis of the relative expression of ZO-1 and claudin-1 compared to β-actin (n = 3); (B) western blot analysis of the relative expression of IL-1β and IL-6 compared to β-actin (n = 3); and (C) relative mRNA expression of IL-6, IL-1β, ZO-1 and claudin-1 examined by RT-qPCR. Data are expressed as mean ± SD; the mean value with different letters indicates significant differences (p < 0.05). | |
3.10 Correlation between the gut microbiota and physiological indexes
Spearman's correlation analysis was used to explore the correlation between the changes of the gut microbiota, phenotypes and the biochemical indicators of mice fed with three different diets. At the family level, Atopobiaceae, Bifidobacteriaceae, Erysipelotrichaceae and Rikenellaceae were positively correlated with body weight, liver weight, spleen weight, epididymal fat pad weight, body weight gain and blood lipid levels. Monoglobaceae was negatively correlated with TG and epididymal fat pad weight, and Ruminococcaceae was negatively correlated with three organ weights and TC/LDL-C. Akkermansiaceae, Prevotellaceae and unclassified_o_Bacteroidales were negatively correlated with body weight, spleen weight and body weight gain (Fig. 14A). At the genus level, phenotypic and biochemical indices were positively correlated with Faecalibaculum and Bifidobacterium, but negatively correlated with Alistipes, Turicibacter, and Prevotellaceae_NK3B31_group in hyperlipidemic-associated mice. Furthermore, Lachnospiraceae_NK4A136_group was negatively correlated with TG and LDL-C. Lachnoclostridium was negatively correlated with TC, TG and LDL-C. Akkermansia was negatively correlated with body weight, body weight gain, and spleen weight (Fig. 14B).
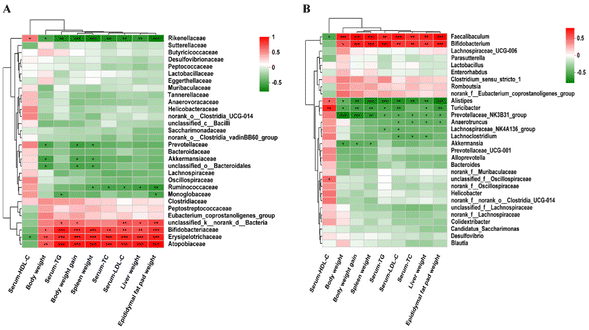 |
| Fig. 14 Spearman's correlation analysis was used to evaluate the correlations between the key bacterial phylotypes and physiological indexes (n = 10 per group). (A) Family level and (B) genus level. *p < 0.05, **p < 0.01, n = 6. | |
4 Discussions
Whole grain foods are gaining popularity as dietary supplements to prevent and slow down cardiovascular disease. Therefore, the present study aims to elucidate the mechanism by which whole grain GBR alleviates hyperlipidemia via modulating lipid metabolism and the gut microbiota in C57BL/6J mice by establishing an HFD-fed model. We found that supplementation with whole grain GBR was effective at preventing obesity after 10 weeks of intervention, as evidenced by significant reductions in body weight, body weight gain, the food efficiency ratio and serum TC, TG and LDL-C levels. Furthermore, excess weight leads to insulin resistance, which in turn promotes hyperlipidemia, creating a vicious cycle. In this study, GBR supplementation improved insulin resistance in HFD-fed mice. Histopathological examination after H&E staining showed that GBR supplementation could significantly improve fatty liver degeneration and lipid accumulation in HFD-fed mice. Moreover, after GBR intake, the increase of liver weight and epididymal fat pad weight and the ratio of liver and epididymal fat pad weight to body weight were reduced in the HFD-fed mice. Chronic low-grade inflammation and increased oxidative stress are important parameters in the pathogenesis of dyslipidemia. Our data showed that GBR supplementation could suppress inflammation by decreasing the expression levels of Tnfα, Inos and Cox-2, decrease oxidative stress by increasing the levels of anti-oxidant enzymes (SOD, CAT and GSH-Px) and reduce the hepatic MDA content. In addition, increased excretion of fecal bile acids, triglycerides, and total cholesterol appears to be essential to the body's cholesterol-lowering function. The following work requires further ESI† on metabolic biochemical indexes in feces.
As reported in a previous study, persistent hyperlipidemia may trigger liver damage to some extent.27 Our study showed that GBR could well ameliorate the HFD-induced histopathological damage in the liver of mice fed with HFD. GBR supplementation can reduce weight gain, improve dyslipidemia and reduce lipid accumulation in high-fat diet mice; it is feasible to have optimistic effects on the physiological and pathological indexes of liver tissue in mice. As a whole grain food, GBR has a significantly higher dietary fiber content than refined white rice and brown rice. This resource will stimulate interest in germinating rice and increase the production and consumption of highly nutritious, health-beneficial bran rice with pigment.28 These findings suggest that GBR supplementation may reduce HFD-induced hyperlipidemia. A study demonstrated that ferulate-rich GBR ethyl acetate extract can alleviate brain oxidative stress in HFD-fed mice.29 Our next challenge will be to examine the effect of GBR extracts (e.g., aqueous extract, alcoholic extract) on hyperlipidemia.
Accumulating evidence suggests that the gut microbiota plays an important role in the maintenance of host health, particularly in the regulation of hyperlipidemia and related metabolic disorders.30 Whole grain foods can prevent and alleviate metabolic disorders associated with the gut microbiota. In this study, we investigated the effects of three diets on high-fat-induced disorders of the gut microbiota in C57BL/6J mice. In this experiment, the diversity of the gut microbiota was analyzed using different α-diversity indices in the HFD-fed mice. Our results found that the HFD group reduced the abundance of microbial α-diversity compared to the Con group. In contrast, GBR intervention restored microbial diversity and abundance by up-regulating the Sob, Ace, Shannon and Chao 1 indices, which is consistent with the data from another report.26 From PCA analysis data, the differences in the overall structure of the gut microbiota between the HFD group and the GBR group were found.
Whole grain foods have beneficial effects in preventing or mitigating HFD-induced gut microbiota dysbiosis.31–34Firmicutes and Bacteroidetes are two major phyla in the gut that play important roles in regulating host glucose, lipid and cholesterol metabolism. Among these, Firmicutes can inhibit or eliminate the growth of Clostridium perfringens to restore gut homeostasis. In addition, some bacteria belonging to the genus Actinomyces contribute to the reduction of blood glucose and lipid levels. Bacteroidetes are among the major bacteria in the intestines of many mammals and individuals, involved in the biotransformation of bile acids and other steroids as well as in the degradation and metabolism of polysaccharides, the latter playing a key role in the development of the immune system and in gut homeostasis.35 A team from Cornell University used Bio-orthogonal Labelling Sort Sequence Spectrometry (BOSSS) to identify Bacteroidetes spp. as a specific strain that metabolizes cholesterol for the first time.36 Compared with the results of Zhao and colleagues,26 the three results were almost identical in restoring microbial diversity and abundance and increasing Bacteroidetes abundance. Besides these, we also found that RGB supplementation decreased the abundance of Firmicutes and Actinomycetes and increased the abundance of Desulphurites; meanwhile, the ratio of Firmicutes/Bacteroidetes (F/B) decreased after adding GBR. At the genus level, the HFD + GBR group reversed HFD-induced alterations of the gut microbiota. Among them, Norank_ F_ Muribaculacea, Faecalibaculum, Unclassified_ F_ Lachnospiraceae, Norank_ F_ Lachnospiraceae and Romboutsia were dominant genera. These findings suggest that GBR intervention can improve gut disorder and alleviate hyperlipidemia.
There is growing evidence for a positive correlation between the Firmicutes to Bacteroidetes (F/B) ratio and metabolic syndrome and hyperlipidemia in both human and mouse models.37 Our results showed that the F/B ratio was significantly higher in the HFD group compared to that in the Con group. The F/B ratio was significantly lower in the HFD + GBR group relative to the HFD group. These results may contribute to further understanding of the beneficial effects of whole grain GBR. The gut microbiota is involved in various physiological activities of organisms, including metabolism, nutrient absorption and production, and immune regulation.38 Compared to the Con group, Desulfovibrio, Unclassified_f_lachnospiraceae, and Unclassified_f_lachnospiraceae (Firmicutes) and Turicibacter (Turicibacteraceae) in the HFD group may have been identified as the most important biomarkers of the gut microbiota. In contrast, they were significantly inhibited by the GBR intervention. Correlation analysis based on taxonomic units showed a significant enrichment effect of GBR on Fluviicola, Roseburia, Victivalis and Lachnospira. Based on the analysis of changes in the gut microbiota of each group, GBR intervention was observed to result in a variety of metabolic functions. We analyzed the expression of genes related to the intestinal barrier. ZO-1 and claudin-1 are two key transporters involved in the regulation of intestinal fatty acid absorption. The results showed that ZO-1 and claudin-1 protein and mRNA expression increased significantly after supplementation with GBR, suggesting that GBR may improve the gut barrier. Our results showed that supplementation with GBR reduced the expression of inflammatory factors IL-6 and IL-1β in the gut, suggesting that GBR may reduce the inflammatory response in the gut. Although this work did not investigate whether SCFA was produced by beneficial symbiotic bacteria after GBR intake, nor did it explore the relationship between SCFA and cholesterol levels in mice, these works are still worthy of further investigation.
Lipid accumulation in the liver is due to an imbalance between lipid deposition and removal. The right amount of cholesterol helps animals produce bile, vitamin D and hormones in the liver and build a healthy cell wall. However, excessive intake of lipids can cause the liver to overproduce cholesterol, which will then be deposited on the blood vessel wall and form plaques to increase the risk of cardiovascular and cerebrovascular diseases. In general, the liver is the main regulator of cholesterol synthesis and metabolism. Excess cholesterol in extrahepatic tissues undergoes reverse cholesterol transport (RCT) and is transported back to the liver by high-density lipoprotein (HDL) or low-density lipoprotein molecules for metabolism and excretion.39 SREBP-2 is an important transcription factor in lipid metabolism, which participates in the regulation of fatty acid and cholesterol synthesis. CYP7A1 is the rate-limiting enzyme for cholesterol metabolism and bile acid synthesis. Non-HDL cholesterol can be transported to the liver via the hepatocyte LDL receptor LDLR, and the overexpression of CYP7A1 maintains cholesterol homeostasis through hepatic bile acid synthesis and secretion.40 A study showed that choline could prevent HFD-induced hepatic cholesterol metabolism disorders by inhibiting cholesterol accumulation and increasing bile acid synthesis through the SREBP-2/HNF-4α/CYP7A1 pathway.41 PCSK9 inhibitors are the latest drugs currently available for the treatment of hypercholesterolemia by lowering the level of LDL-C.42 HMGCR is a rate-control enzyme of the mevalonate pathway, a metabolic pathway that produces cholesterol and other isoprenoids.43 HMG-CoA reductase is the target of widely used cholesterol-lowering drugs, which are collectively referred to as statins. Our study showed that, compared with the HFD group, the expression levels of PCSK9, HMGCR and SREBP-2 through GBR intervention were down-regulated by 48.76%, 51.40% and 57.66%, respectively. These results revealed that GBR may regulate cholesterol metabolism through the SREBP-2/PCSK9/HMGCR signaling pathway in the HFD-fed mice (Fig. 15). However, we did not study the alterations of the gut microbiota and metabolic products that affect the expressions of cholesterol metabolism-related genes. They need to be studied in the future, for example, which metabolic products can affect the expressions of cholesterol metabolism-related genes and which signaling pathways and transcription factors are involved in the transcription of cholesterol metabolism-related genes.
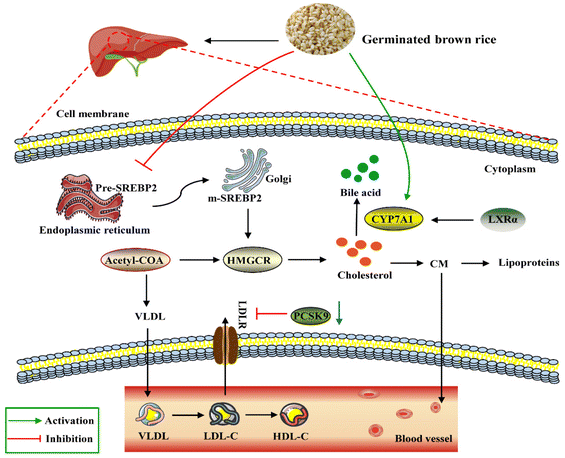 |
| Fig. 15 Schematic diagram of the molecular mechanism by which GBR regulates lipid and cholesterol metabolism in the HFD-fed mice. As shown in the figure, GBR reduces circulating TC, TG, and LDL-C levels by inhibiting SREBP2 and thus HMGCR levels. LDLR is the transcriptional target of SREBP2, and GBR enhances LDLR-mediated uptake of cholesterol from circulation by peripheral cells. Notably, GBR may also inhibit the lysosomal degradation of PCSK9-LDLR complexes mediated by PCSK9-LDLR and avoid LDL accumulation in vivo. In addition, CYP7A1 is activated by GBR to convert cholesterol into primary bile acids in the cholesterol reverse transport pathway. LXRα is the upstream regulator of CYP7A1, and GBR can promote the expression level of LXRα and regulate lipid homeostasis. | |
LXRα promoted the expression of its target gene ATP-binding cassette transporter (ABCA1).44 ABCA1 improves lipid metabolism by promoting reverse cholesterol transport, pumping excess cholesterol from peripheral cells, increasing HDL-C synthesis, and reducing TC and LDL-C levels.45 The first step in the reverse cholesterol transport process is that ABCA1 transports lipids, including phospholipids and cholesterol, to the extracellular space and then binds to the extracellular lipid receptor apolipoprotein A-I (ApoA-I) to form nascent HDL-C. Furthermore, apolipoprotein B can be used for cholesterol transport between the liver and peripheral tissues. The cholesterol-lowering mechanism of GBR may be due, at least in part, to the increased excretion of steroids and bile acids into the feces, together with enhanced CYP7A activity in the microsomal fraction of the liver. Our study suggested that supplementation with whole grain GBR significantly reduced cholesterol concentrations by regulating the expression levels of CYP7A1 and LXRα in C57BL/6J mice. The mRNA expression of LXRα and ABCA1 in the high-fat group was significantly lower than that in the normal group, suggesting that the mRNA expression levels of LXRα and ABCA1 were significantly inhibited in the hyperlipidemia state. GBR may promote cholesterol reversal in mice by up-regulating LXRα and thereby stimulating the expression of ABCa1 and CYP7A1. This suggests that the possible mechanism of supplementing germinated brown rice with cholesterol-lowering activity is mediated by increased bile acid secretion. Zhang et al. showed that crocin (CRO) can promote the up-regulation of PPARγ/LXR-α, ABCA1 and AKT phosphorylation, thereby inhibiting foam cell formation and promoting reverse cholesterol transport.46 This is consistent with our experimental results. These findings suggest that GBR may facilitate the excretion of cholesterol from the body by inhibiting cholesterol absorption in the small intestine and reducing the synthesis of cholesteryl esters through the LXRs-ABCA1 pathway, thereby achieving a reduction in hyperlipidemia. The enhanced expression promotes TC reverse transport to maintain TC homeostasis, which plays a role in the prevention and treatment of hyperlipidemia. However, the underlying mechanisms still need to be further elucidated in future studies.
Spearman's correlation analysis showed that Lachnospiraceae_NK4A136_group was negatively correlated with TG and LDL-C. Lachnoclostridium was negatively correlated with TC, TG and LDL-C. Akkermansia was negatively correlated with body weight, body weight gain, and spleen weight. Moreover, some links between lipid metabolism parameters and the gut microbiota and its metabolites have been found, but the exact relationship remains unclear. Metabolites of the gut microbiota such as secondary bile acids, TMAO and LPS have also been reported to be involved in host lipid metabolism.47,48 The PICRUSt predicted results showed that GBR supply improved energy metabolism, lipid metabolism, glycolysis and gluconeogenesis, which were closely related to metabolism (Fig. S3†). The beneficial effects of GBR on hyperlipidemia appear to be partially attributed to the synergistic action of its multiple bioactive compounds. However, further studies are needed to verify the effectiveness of GBR against hyperlipidemia in human studies and the recommended dose of GBR used in the daily diet.
5 Conclusions
Our study indicated that GBR intervention could improve hyperlipidemia in HFD-fed mice. The underlying mechanisms may involve the regulation of the composition and abundance of the gut microbiota and the expression levels of genes and proteins related to lipid metabolism in the liver and gut. Additionally, it reduces the synthesis and absorption of cholesterol and promotes the reverse transport route of cholesterol. These results support the fact that GBR can be considered a potential candidate staple food for the prevention and adjunctive treatment of hyperlipidemia. Furthermore, further studies are needed to reveal the mechanism of action of vital active ingredients in GBR to reduce hyperlipidemia and investigate the relationship between metabolites produced by GBR through the gut microbiota and lipid metabolism, which can help clinical applications.
Abbreviations
Abca1 | ATP binding cassette transporter a1 |
AI | Atherosclerotic index |
ALT | Alanine transaminase |
APob | Apolipoprotein B |
AST | Aspartate aminotransferase |
AUC | Area under the glucose curve |
CAT | Catalase |
CYP7A1 | Cytochrome P450 7A1 |
GBR | Germinated brown rice |
GSH-Px | Glutathione peroxidase |
HDL-C | High-density lipoprotein cholesterol |
HMGCR | 3-hydroxy-3-methylglutaryl coenzyme a reductase |
IL-1β | Interleukin-1β |
IL-6 | Interleukin-6 |
LDL-C | Low-density lipoprotein cholesterol |
LDLR | Low density lipoprotein receptor |
LEfSe | Linear discriminant analysis effect size |
MDA | Malondialdehyde |
PCA | Principal component analysis |
PCoA | Principal co-ordinate analysis |
PCSK9 | Proprotein convertase subtilisin/kexin type 9 |
PICRUSt | Phylogeny survey using unobserved state reconstruction |
PLS-DA | Partial least squares discriminant analysis |
RT-qPCR | Real time-quantitative polymerase chain reaction |
SOD | Superoxide dismutase |
SREBP-2 | Sterol regulatory element binding protein-2 |
TC | Total cholesterol |
TG | Triglycerides |
Conflicts of interest
The authors declare no conflict of interest.
Acknowledgements
This work was supported by the Key Projects of the State Key R&D Program, China (No. 2022YFF1100200), the Post-graduate Scientific Research Innovations Project of Hunan Province (CX20210862), and the Scientific Innovation Fund for Post-graduates of the Central South University of Forestry and Technology (CX202101027).
References
- H. Ito, K. Nakasuga, A. Ohshima, Y. Sakai, T. Maruyama, Y. Kaji, M. Harada, S. Jingu and M. Sakamoto, Excess accumulation of body fat is related to dyslipidemia in normal-weight subjects, Int. J. Obes. Relat. Metab. Disord., 2004, 28(2), 242–247 CrossRef CAS PubMed.
- J. Stewart, T. McCallin, J. Martinez, S. Chacko and S. Yusuf, Hyperlipidemia, Pediatr. Rev., 2020, 41(8), 393–402 CrossRef.
- U. J. Tietge, Hyperlipidemia and cardiovascular disease: inflammation, dyslipidemia, and atherosclerosis, Curr. Opin. Lipidol., 2014, 25(1), 94–95 CrossRef CAS PubMed.
- Ł. Bułdak, B. Marek, D. Kajdaniuk, A. Urbanek, S. Janyga, A. Bołdys, M. Basiak, M. Maligłówka and B. Okopień, Endocrine diseases as causes of secondary hyperlipidemia, Endokrynol. Pol., 2019, 70(6), 511–519 CrossRef.
- G. F. Watts, E. M. Ooi and D. C. Chan, Demystifying the management of hypertriglyceridaemia, Nat. Rev. Cardiol., 2013, 10(11), 648–661 CrossRef CAS PubMed.
- Y. Nie and F. Luo, Dietary fiber: an opportunity for a global control of hyperlipidemia, Oxid. Med. Cell. Longevity, 2021, 2021, 5542342 Search PubMed.
- F. Mainieri, S. L. Bella and F. Chiarelli, Hyperlipidemia and cardiovascular risk in children and adolescents, Biomedicines, 2023, 11(3), 809–834 CrossRef CAS PubMed.
- M. Romaní-Pérez, C. Bullich-Vilarrubias, I. López-Almela, R. Liébana-García, M. Olivares and Y. Sanz, The microbiota and the gut-brain axis in controlling food intake and energy homeostasis, Int. J. Mol. Sci., 2021, 22(11), 5830 CrossRef.
- W. Hernández-Ceballos, J. Cordova-Gallardo and N. Mendez-Sanchez, Gut microbiota in metabolic-associated fatty liver disease and in other chronic metabolic diseases, J. Clin. Transl. Hepatol., 2021, 9(2), 227–238 Search PubMed.
- W. Zhou, Y. Cheng, P. Zhu, M. I. Nasser, X. Zhang and M. Zhao, Implication of gut microbiota in cardiovascular diseases, Oxid. Med. Cell. Longevity, 2020, 2020, 5394096 Search PubMed.
- J. Pagand, P. Heirbaut, A. Pierre and B. Pareyt, The magic and challenges of sprouted grains, Cereal Foods World, 2017, 62(5), 221–226 CrossRef CAS.
- Z. Cheng, D. Qiao, S. Zhao, B. Zhang, Q. Lin and F. Xie, Whole grain rice: updated understanding of starch digestibility and the regulation of glucose and lipid metabolism, Compr. Rev. Food Sci. Food Saf., 2022, 21(4), 3244–3273 CrossRef CAS.
- C. Ren, B. Hong, X. Zheng, L. Wang, Y. Zhang, L. Guan, X. Yao, W. Huang, Y. Zhou and S. Lu, Improvement of germinated brown rice quality with autoclaving treatment, Food Sci. Nutr., 2020, 8(3), 1709–1717 CrossRef CAS.
- E. M. Oo, K. Ruamyod, L. Khowawisetsut, C. Turbpaiboon, V. Chaisuksunt, P. Uawithya, N. Pholphana, N. Rangkadilok and S. Chompoopong, Germinated brown rice attenuates cell death in vascular cognitive impaired mice and glutamate-induced toxicity in HT22 cells, J. Agric. Food Chem., 2020, 68(18), 5093–5106 CrossRef CAS.
- N. N. Wu, R. Li, Z. J. Li and B. Tan, Effect of germination in the form of paddy rice and brown rice on their phytic acid, GABA, γ-oryzanol, phenolics, flavonoids and antioxidant capacity, Food Res. Int., 2022, 159, 111603 CrossRef CAS PubMed.
- H. W. Yen, H. L. Lin, C. L. Hao, F. C. Chen, C. Y. Chen, J. H. Chen and K. P. Shen, Effects of pre-germinated brown rice treatment high-fat diet-induced metabolic
syndrome in C57BL/6J mice, Biosci., Biotechnol., Biochem., 2017, 81(5), 979–986 CrossRef CAS PubMed.
- R. Zhao, N. Ghazzawi, J. Wu, K. Le, C. Li, M. H. Moghadasian, Y. L. Siow, F. B. Apea-Bah, T. Beta, Z. Yin and G. X. Shen, Germinated brown rice attenuates atherosclerosis and vascular inflammation in low-density lipoprotein receptor-knockout mice, J. Agric. Food Chem., 2018, 66(17), 4512–4520 CrossRef CAS PubMed.
- J. C. Beaulieu, R. A. Moreau, M. J. Powell and J. M. Obando-Ulloa, Lipid profiles in preliminary germinated brown rice beverages compared to non-germinated brown and white rice beverages, Foods, 2022, 11(2), 220–236 CrossRef CAS.
- S. Petchdee, W. Laosripaiboon, N. Jarussophon and S. Kumphune, Cardio-protective effects of germinated brown rice extract against myocardial ischemia reperfusion injury, High Blood Pressure Cardiovasc. Prev., 2020, 27(3), 251–258 CrossRef CAS.
- S. M. Lim, Y. M. Goh, N. Mohtarrudin and S. P. Loh, Germinated brown rice ameliorates obesity in high-fat diet induced obese rats, BMC Complementary Altern. Med., 2016, 16, 140–151 CrossRef.
- C. L. Hao, H. L. Lin, L. Y. Ke, H. W. Yen and K. P. Shen, Pre-germinated brown rice extract ameliorates high-fat diet-induced metabolic syndrome, J. Food Biochem., 2019, 43(3), e12769 CrossRef.
- T. T. Mai, T. T. Trang and T. T. Hai, Effectiveness of germinated brown rice on metabolic syndrome: a randomized control trial in Vietnam, AIMS Public Health, 2020, 7(1), 33–43 Search PubMed.
- H. A. Adamu, M. U. Imam, O. Der-Jiun and M. Ismail, In utero exposure to germinated brown rice and its GABA extract attenuates high-fat-diet-induced insulin resistance in rat offspring, J. Nutrigenet. Nutrigenomics, 2017, 10(1–2), 19–31 CAS.
- L. Wang, Q. Lin, T. Yang, Y. Liang, Y. Nie, Y. Luo, J. Shen, X. Fu, Y. Tang and F. Luo, Oryzanol modifies high fat diet-induced obesity, liver gene expression profile, and inflammation response in mice, J. Agric. Food Chem., 2017, 65(38), 8374–8385 CrossRef CAS.
- J. Bai, T. Yang, Y. Zhou, W. Xu, S. Han, T. Guo, L. Zhu, D. Qin, Y. Luo, Z. Hu, X. Wu, F. Luo, B. Liu and Q. Lin, Octacosanol modifies obesity, expression profile and inflammation response of hepatic tissues in high-fat diet mice, Foods, 2022, 11(11), 1606–1625 CrossRef CAS PubMed.
- R. Zhao, F. Huang, C. Liu, V. Asija, L. Cao, M. Zhou, H. Gao, M. Sun, X. Weng, J. Huang, X. Liao, Z. Liu, L. Sen and G. X. Shen, Impact of germinated brown rice and brown rice on metabolism, inflammation, and gut microbiome in high fat diet-induced insulin resistant mice, J. Agric. Food Chem., 2022, 70(44), 14235–14246 CrossRef CAS.
- G. Mandraffino, C. Morace, M. S. Franzè, V. Nassisi, D. Sinicropi, M. Cinquegrani, C. Saitta, R. Scoglio, S. Marino, A. Belvedere, V. Cairo, A. Gullo, M. Scuruchi, G. Raimondo and G. Squadrito, Fatty liver as potential biomarker of atherosclerotic damage in familial combined hyperlipidemia, Biomedicines, 2022, 10(8), 1770–1784 CrossRef CAS.
- J. C. Beaulieu, S. M. Boue and P. Goufo, Health-promoting germinated rice and value-added foods: a comprehensive and systematic review of germination effects on brown rice, Crit. Rev. Food Sci. Nutr., 2022, 1–34 CrossRef PubMed.
- N. H. Azmi, N. Ismail, M. U. Imam, J. Ooi and S. N. H. Oslan, Modulation of high-fat diet-induced brain oxidative stress by ferulate-rich germinated brown rice ethyl acetate extract, Molecules, 2022, 27(15), 4907 CrossRef CAS.
- X. Jia, W. Xu, L. Zhang, X. Li, R. Wang and S. Wu, Impact of gut microbiota and microbiota-related metabolites on hyperlipidemia, Front. Cell. Infect. Microbiol., 2021, 11, 634780 CrossRef CAS PubMed.
- S. Yan, J. Chen, L. Zhu, T. Guo, D. Qin, Z. Hu, S. Han, Y. Zhou, O. D. Akan, J. Wang, F. Luo and Q. Lin, Oryzanol attenuates high fat and cholesterol diet-induced hyperlipidemia by regulating the gut microbiome and amino acid metabolism, J. Agric. Food Chem., 2022, 70(21), 6429–6443 CrossRef PubMed.
- D. Hou, Q. Zhao, L. Yousaf, B. Chen, Y. Xue and Q. Shen, A comparison between whole mung bean and decorticated mung bean: beneficial effects on the regulation of serum glucose and lipid disorders and the gut microbiota in high-fat diet and streptozotocin-induced prediabetic mice, Food Funct., 2020, 11(6), 5525–5537 RSC.
- Y. Zhang, W. Liu, D. Zhang, Y. Yang, X. Wang and L. Li, Fermented and germinated processing improved the protective effects of foxtail millet whole grain against dextran sulfate sodium-induced acute ulcerative colitis and gut microbiota dysbiosis in C57BL/6 mice, Front. Nutr., 2021, 8, 694936 CrossRef.
- Y. Meng, Q. Meng, C. Li, M. Wang, S. Li, J. Ying, H. Zheng, S. Bai, Y. Xue and Q. Shen, A comparison between partially peeled hulless barley and whole grain hulless barley: beneficial effects on the regulation of serum glucose and the gut microbiota in high-fat diet-induced obese mice, Food Funct., 2023, 14(2), 886–898 RSC.
- E. M. Brown, X. Ke, D. Hitchcock, S. Jeanfavre, J. Avila-Pacheco, T. Nakata, T. D. Arthur, N. Fornelos, C. Heim, E. A. Franzosa, N. Watson, C. Huttenhower, H. J. Haiser, G. Dillow, D. B. Graham, B. B. Finlay, A. D. Kostic, J. A. Porter, H. Vlamakis, C. B. Clish and R. J. Xavier, Bacteroides-derived sphingolipids are critical for maintaining intestinal homeostasis and symbiosis, Cell Host Microbe, 2019, 25(5), 668–680 CrossRef CAS.
- H. H. Le, M. T. Lee, K. R. Besler, J. M. C. Comrie and E. L. Johnson, Characterization of interactions of dietary cholesterol with the murine and human gut microbiome, Nat. Microbiol., 2022, 7(9), 1390–1403 CrossRef CAS PubMed.
- X. Pi, W. Teng, D. Fei, G. Zhao and W. Liu, Effects of live combined bacillus subtilis and enterococcus faecium on gut microbiota composition in C57BL/6 mice and in humans, Front. Cell. Infect. Microbiol., 2022, 12, 821662 CrossRef CAS PubMed.
- M. Moszak, M. Szulińska and P. Bogdański, You are what you eat-the relationship between diet, microbiota, and metabolic disorders-a review, Nutrients, 2020, 12(4), 1096–1126 CrossRef CAS.
- T. K. Trajkovska and S. Topuzovska, High-density lipoprotein metabolism and reverse cholesterol transport: strategies for raising HDL cholesterol, Anatolian J. Cardiol., 2017, 18(2), 149–154 Search PubMed.
- J. A. Hubacek and D. Bobkova, Role of cholesterol 7 alpha-hydroxylase (CYP7A1) in nutrigenetics and pharmacogenetics of cholesterol lowering, Mol. Diagn. Ther., 2006, 10(2), 93–100 CrossRef CAS PubMed.
- Y. Wang and Z. P. Liu, PCSK9 inhibitors: novel therapeutic strategies for lowering LDL cholesterol, Mini-Rev. Med. Chem., 2019, 19(2), 165–176 CrossRef CAS PubMed.
- H. Zheng, T. Zhao, Y. C. Xu, D. G. Zhang, Y. F. Song and X. Y. Tan, Dietary choline prevents high fat-induced disorder of hepatic cholesterol metabolism through SREBP-2/HNF-4α/CYP7A1 pathway in a freshwater teleost yellow catfish Pelteobagrus fulvidraco, Biochim. Biophys. Acta, Gene Regul. Mech., 2022, 1865(7), 194874 CrossRef CAS PubMed.
- S. Azizidoost, H. Babaahmadi-Rezaei, Z. Nazeri, M. Cheraghzadeh and A. Kheirollah, Amyloid beta increases ABCA1 and HMGCR protein expression, and cholesterol synthesis and accumulation in mice neurons and astrocytes, Biochim. Biophys. Acta, Mol. Cell Biol. Lipids, 2022, 1867(1), 159069 CrossRef CAS.
- J. Q. Wang, L. L. Li, A. Hu, G. Deng, J. Wei, Y. F. Li, Y. B. Liu, X. Y. Lu, Z. P. Qiu, X. J. Shi, X. Zhao, J. Luo and B. L. Song, Inhibition of ASGR1 decreases lipid levels by promoting cholesterol excretion, Nature, 2022, 608(7922), 413–420 CrossRef CAS PubMed.
- Y. R. Wu, X. Y. Shi, C. Y. Ma, Y. Zhang, R. X. Xu and J. J. Li, Liraglutide improves lipid metabolism by enhancing cholesterol efflux associated with ABCA1 and ERK1/2 pathway, Cardiovasc. Diabetol., 2019, 18(1), 146–158 CrossRef PubMed.
- F. Zhang, P. Liu, Z. He, L. Zhang, X. He, F. Liu and J. Qi, Crocin ameliorates atherosclerosis by promoting the reverse cholesterol transport and inhibiting the foam cell formation via regulating PPARγ/LXR-α, Cell Cycle, 2022, 21(2), 202–218 CrossRef CAS.
- M. Schoeler and R. Caesar, Dietary lipids, gut microbiota and lipid metabolism, Rev. Endocr. Metab. Disord., 2019, 20(4), 461–472 CrossRef CAS.
- X. Fang, H. Wu, X. Wang, F. Lian, M. Li, R. Miao, J. Wei and J. Tian, Modulation of gut microbiota and metabolites by berberine in treating mice with disturbances in glucose and lipid metabolism, Front. Pharmacol., 2022, 13, 870407 CrossRef CAS.
|
This journal is © The Royal Society of Chemistry 2024 |
Click here to see how this site uses Cookies. View our privacy policy here.