DOI:
10.1039/D4FB00164H
(Review Article)
Sustainable Food Technol., 2024,
2, 1592-1609
Future production of yeast biomass for sustainable proteins: a critical review
Received
31st May 2024
, Accepted 22nd August 2024
First published on 2nd September 2024
Abstract
Yeast biomass has untapped potential as a sustainable source of nutritional protein. To realise this, current production capacity and the demand for yeast-based products in food applications must be increased. This review explores the possibility of increasing yeast supply using low-cost and sustainable substrates such as lignocellulosic sugars, starch hydrolysates and waste lactose, and by utilising the waste yeast biomass that will be generated from future recombinant protein production. Candidate yeast strains and processes for producing biomass from these substrates are reviewed in relation to production efficiency and product functionality. The opportunity to lower production costs and control yeast cell properties using continuous cultivation is highlighted. Current knowledge of how yeast diversity, metabolism and physiology are influenced by growth conditions is brought together to understand how yeast biomass can be produced with desirable functional properties. In particular, this review provides insights into how the variety and adaptability of yeast can make it possible to adjust attributes such as protein and cell wall composition through strain selection and controlled production. Major gaps are identified as targets for future research, in particular understanding the functional properties of non-Saccharomyces yeast biomass that could be produced from lignocellulosic sugars, lactose and precision fermentation. Specific, controlled studies of yeast biomass functionality in relation to species and growth are now needed to help expand the scale of production and associated environmental benefits of nutritional yeast.
Sustainability spotlight
This article aims to enable expanded production of yeast biomass as a sustainable source of nutritional protein. The review focussed on addressing key limits to current yeast supply by outlining paths to utilisation of low-cost and sustainable feedstocks and efficient production processes. Achieving this at large scale would contribute to three UN SDGs: 2: zero hunger: increasing the world's supply of nutritional protein in a sustainable and economic manner will help alleviate hunger. 12: responsible consumption and production: ensuring more sustainable production of nutritional proteins while better utilising wastes. 15: life on land: freeing up land currently used to inefficiently produce protein will help reduce pressures leading to deforestation.
|
1. Introduction
1.1 Yeast as a sustainable source of protein
The growing global demand for protein is straining key resources such as land, water and phosphorous, and is significantly contributing to greenhouse gas (GHG) emissions.1 While plant-based proteins can displace more resource- and GHG-intensive animal-derived proteins, microbial protein production from organisms such as yeast, can be even more resource-efficient.2,3 Microbial biomass is produced in fermenters, which enables complete utilisation of nitrogen and phosphorus while eliminating run-off pollution.4 Further, microbes have significant inherent advantages over plants and animals as a source of protein, including very short generation times, the ability to manipulate metabolism and composition, and the possibility of continuous production independent of climatic factors. Although heterotrophically grown microbes such as yeast require an organic carbon source, this can involve utilisation of low-value by-products or wastes5 such as molasses and lactose, sugars obtained from high-yielding crops such as sugarcane, or sugars derived from second-generation feedstocks such as agricultural wastes or lignocellulosic crops such as switchgrass.6 Thus, utilising microbial protein production methods can provide major reductions in land, water and GHG footprints compared to animal protein production.2,4,6 The potential of microbial protein production for food (often referred to a single cell protein, SCP) has been recognised for almost a century, with the term ‘single cell protein’ (SCP) first coined at Massachusetts Institute of Technology in 1966.7–10 However, production and utilisation of microbial proteins must be dramatically increased to realise the potential environmental benefits. Further, large-scale production of yeast biomass should preferably utilise second generation feedstocks,11 leveraging the experience and achievements from industrial scale bioethanol production.12
Yeast is a particularly promising source of microbial protein. Yeasts such as Saccharomyces have been domesticated for millennia, studied in detail, and are robust and fast-growing in a variety of industrial applications.13 Traditionally, the catalytic power of yeast has been used to leaven bread14 and produce ethanol and flavours in beer and wine.15 More recently, the ability of recombinant yeast to express foreign proteins is being developed to produce specific food-related products such as proteins and vitamins, using so-called ‘precision fermentation’.16 As will be discussed below, a variety of yeast species can be used for food ingredient production, including S. cerevisiae (Baker's yeast and ale yeast), Saccharomyces pastorianus (lager yeast),17,18Kluyveromyces and Candida (lactose-fermenting yeasts), Komagataella phaffii (methyltrophic yeast)19 and Yarrowia (oleaginous yeast). The focus of this review is on future sustainable production of yeast biomass as a source of nutritional proteins for food applications20 such as extruded food products,21,22 processed meats23 and mayonnaise.24 This includes both purpose-grown yeast and yeast biomass as a byproduct from precision fermentation.
1.2 Yeast proteins as food ingredients
Yeast are a rich source of proteins, which typically represent 40–60% of the cell biomass.20 As a food ingredient, yeast proteins comprise the entire proteome of the organism including a multitude of enzymes and structural proteins. This is contrast to most plant proteins used as food ingredients, which typically comprise a small number of highly abundant storage proteins (e.g. glycinin and β-conglycinin in soybeans,25 globulin, glutelin, albumin and prolamin in chickpeas26 and glutenins and gliadins in wheat27). The limited structural diversity with different plant protein ingredients makes them relatively straightforward to understand in terms of food functionality and nutritional characteristics such as amino acid profile. In comparison, the diversity of proteins within yeast cells coupled with variability of protein profiles as a function of both species and growth conditions makes it much more challenging to characterise and predict their behaviour as food ingredients. It also provides an opportunity to tailor the properties of the produced yeast proteins through species selection and process design.
Currently, yeast serves as the raw material for so-called yeast extracts,28 which are derived from autolysed yeast cells and used as ingredients for a range of food applications including savoury spreads, soups, snacks, sauces and prepared meals.29 As food ingredients, the hydrolysed yeast proteins, peptides and amino acids have desirable taste attributes such as umami and kokumi, participate in Maillard reactions and contribute to the nutritional value of the products.30 Proteins from yeast such as S. cerevisiae and Pichia sp. are generally considered to not pose concerns in terms of allergenicity or toxicity.31 Further, the proteins in S. cerevisiae contain high amounts of essential amino acids.32,33 The content and profile of essential amino acids in S. cerevisiae, Yarrowia lipolytica and Candida utilis compared favourably against wheat, egg, cow milk and FAO requirements.20 While the high content of nucleic acids in yeast would limit safe dietary intake, there are established methods involving heat, enzyme or alkali treatments to breakdown or extract the RNA.34 Increasing global consumption of yeast biomass to accrue meaningful environmental benefits, will rely on both reducing the cost of production to commodity levels and expanding the range of applications for yeast products. Developing yeast products with desirable attributes will be key, and will require careful control of yeast biology during large-scale production and bioprocessing to optimise the properties of yeast cells for specific food applications and products.
The functionality of yeast biomass as a food ingredient for various formulations such as baked goods, snacks and processed meat has been recently reviewed,35 and includes properties such as solubility, flavour enhancement, nutritional supplementation, foaming and emulsification. While these reviews provide a good overview of the relevant functional properties of relevance and the recent literature, it is notable that the information was not discussed in relation to different yeast species or growth conditions.35 Overall, research into food functionality of yeast is still in its infancy, with studies by food scientists starting to emerge, but mostly on generic ‘yeast proteins’. For example, a recent study investigating the foaming and emulsifying properties of yeast protein did not mention the species of yeast, the growth conditions, nor any characterisation information of the proteins.36 Further, the digestibility of one yeast protein concentrate powder has recently been studied, however the species and growth conditions of the yeast were not detailed.37 Further, there appears to be a lack of comparative analyses of the functional properties between or within different yeasts or of different individual or classes of yeast proteins from within the proteome. Although not specifically targeted to nutrition, one early studied compared the amino acid profiles of eight yeast species including Saccharomyces cerevisiae, Candida utilis, Kluyveromyces fragilis, finding some broad similarities but also variability.38 As discussed below, the protein and amino acid profiles of different yeasts grown in different ways on different media will be highly variable and to some extent able to be manipulated. Producing yeast biomass with desirable properties will require understanding how yeast proteomes are influenced by controllable production parameters, in the context of scalable and economic feedstocks and processes. There have been major recent advances in our understanding of yeast metabolism39–41 that can now be applied to controlling the yeast proteome as a raw material for food applications grown on substrates sourced from sustainable feedstocks.
2. Future sources of edible yeast biomass
Current production of potentially edible protein from baker's and brewer's yeasts is a little under 1 M tonne per year (assuming 1.7 Mt per year of yeast containing ∼50% protein42). This is equivalent to ∼0.3% of current global protein demand (260–290 Mt per year).1 Approximately 2/3 of this yeast is baker's yeast, and 1/3 is brewer's spent yeast (BSY) that is produced as a by-product from alcoholic beverage production.28,43 However, only a small proportion of this yeast biomass is converted into food ingredients such as yeast extracts. Most baker's yeast is used in baking applications, while the majority of BSY is used as an animal feed.42 Yeast biomass is also generated in wine (i.e. lees)44 and spirit making (i.e. spent yeast from distilleries), but these processes produce relatively low amounts of biomass. Further, yeast biomass is a byproduct of industrial bioethanol production, however these processes are not operated under food-grade conditions, limiting the potential of the yeast biomass as a source of human nutritional protein.
Future expansion of yeast food products will require major new sources of yeast. While it is possible to increase the use of available BSY for yeast-based food ingredients, as a by-product, brewer's yeast production is ultimately limited by the demand for beer. There is much greater scope to expand the production of baker's yeast as a food ingredient. This would require construction of new dedicated fermentation facilities to the increase production capacity of baker's yeast biomass for food applications. Production of baker's yeast has traditionally used molasses as the carbon source, however the sustainability and price of molasses is becoming a limiting factor.45,46 Baker's yeast can also be produced using hydrolysed starch from crops such as cassava47 or rice,48 which can greatly expand the amount of available substrate.
To further expand future yeast protein production, it is increasingly being acknowledged that it is preferable to use second generation feedstock materials that don't compete with food production and that production of protein should be considered over fuels.11 In particular, yeasts that are able to utilise pentose sugars (natively or via genetic modification) could be produced from lignocellulosic sugars, and spent yeast resulting from production of recombinant food proteins could be utilised. These there is also potential to produce other edible yeasts such as Kluyveromyces using the lactose in dairy waste streams such as whey, or oil-bearing yeasts such as Yarrowia lipolytica. These opportunities represent a range of new yeast production processes that will differ from each other in many respects, including the species/strain of yeast, growth medium composition, reactor mode and operational conditions (Table 1). In this section, the key distinguishing features of the different yeast production options will be critically reviewed. This provides the practical context for the subsequent analysis of the effects of controllable yeast production parameters on biomass properties.
Table 1 Comparative summary of future options for expanding the production of yeast biomass as a food ingredient
|
Starch hydrolysates |
Lignocellulosic sugars |
Precision fermentation yeast |
Lactose-fermenting |
Yeast |
Genus/Species |
S. cerevisiae or others |
Various, including Saccharomyces (recombinant), Komagataella, and Yarrowia |
S. cerevisiae or Komagataella phaffii (formerly Pichia pastoris) |
Kluyveromyces or Candida |
GMO |
|
No |
Maybe |
Yes |
No |
Reactor mode |
|
Fed-batch or continuous |
Batch (non-Crabtree yeast), fed-batch (Crabtree yeast) or continuous (Crabtree or non-Crabtree yeast) |
Fed-batch |
Batch (non-Crabtree yeast), fed-batch (Crabtree yeast) or continuous (Crabtree or non-Crabtree yeast) |
Harvest growth state |
|
Decelerating growth (batch/fed-batch); controlled μ = D for continuous |
Decelerating growth (batch/fed-batch); controlled μ = D for continuous |
Decelerating growth |
Decelerating growth (batch/fed-batch); controlled μ = D for continuous |
Feedstock |
Source |
Corn, wheat, tubers, rice |
Grasses, forestry, food crop residues |
Sugarcane/beet (molasses), various (methanol and other) |
Dairy |
Sustainability |
Up-grading of food supply (edible sugar to protein) |
Increased food supply (non-edible sugar to protein) |
Increased food supply (waste by-product to protein) |
Increased food supply (waste by-product to protein) |
Growth medium |
C-source |
Molasses (sucrose); or starch hydrolysates (glucose, maltose) |
Lignocellulosic sugars including glucose, xylose, mannose, galactose and arabinose |
Various, including molasses and methanol |
Lactose from whey |
N-source |
Urea or ammonia/um |
Urea or ammonia/um |
Various |
OrgN in whey |
Phenolics and inhibitors |
Molasses phenolics (e.g. caffeic acid, ferulic acid, gallic acid)49 |
Various including furfural and HMF |
Minimal |
Not significant |
Extracellular |
Temperature (°C) |
∼30 |
Various |
Various |
∼30 |
Environment |
Dissolved O2 |
Aerobic |
Aerobic |
Aerobic |
Aerobic |
pH |
∼4.5 |
4–6 |
4–6 |
4–5 (ref. 50) |
Future scope |
Manipulable conditions to tailor yeast biomass |
Yes |
Yes |
No |
Yes |
Potential scale of production |
Very large |
Very large |
Large |
Moderate |
2.1 Expanded production of baker's yeast
As an active ingredient for commercial and domestic baking applications, the major aim of conventional processes is to produce high yields of active Baker's yeast from low-cost medium ingredients.51,52 This has led to a tightly defined and well-established production process. Future expanded production of baker's yeast biomass for nutritional proteins can be expected to follow a similar process, aimed at maximising biomass yield. To achieve a high biomass yield baker's yeast is produced using aerobic fed-batch fermentation. The yeast must be grown under aerobic conditions to enable more efficient aerobic respiration to occur in place of fermentation. When grown aerobically, the biomass yield can reach up to 0.5 g g−1 compared to around 0.1 g g−1 under anaerobic conditions under which much of the substrate carbon is converted to ethanol instead of new cell matter.53 To be aerobic, active aeration is required to maintain the oxygen level above a critical dissolved oxygen concentration, the value of which varies in response to adaptive yeast metabolism.54
Aerobic growth is a necessary but not sufficient requirement for avoiding ethanol production by baker's yeast. To grow rapidly when high concentrations of glucose are present, some yeast including S. cerevisiae invoke a form of overflow metabolism, referred to as the Crabtree effect, to generate energy via aerobic fermentation rather than the more efficient respiration.55,56 This occurs when the concentration of glucose in the medium exceeds a critical value, which has been reported to be as low as 40 and 50 mg L−1.53 This means that batch cultivation of Saccharomyces, even under aerobic conditions, will result in the formation of ethanol as high sugar concentrations are present in the initial growth media. Therefore, to avoid the Crabtree effect and resulting ethanol production, fed-batch cultivation is used in which low concentrations of glucose are maintained by controlled feeding of substrate during the cultivation to provide the necessary flow of carbon.57–59 Industrial fed-batch production of baker's yeast is performed in large bubble column bioreactors (>100 m3)53 operated at temperatures between 28–30 °C.60 The reactors are typically seeded with about 20 g L−1 of yeast, which increases to a final concentration of 55–60 g L−1.53 The growth medium is typically cane or beet molasses, which contains sucrose that is rapidly hydrolysed to glucose and fructose by the yeast's invertase, with ammonia fed as a nitrogen source.53 Very large-scale production of yeast biomass will require cheap and sustainable sources of fermentable sugars that extend beyond the limited supply of molasses. Starch-based feedstocks that can be hydrolysed to glucose would appear compatible with current molasses-based production processes involving S. cerevisiae. In contrast to production of biofuels from these crops that presents a food versus fuel issue, production of yeast biomass represents an up-grading of food supply. While new facilities dedicated to the production of baker's yeast biomass for food applications from starch-based feedstock could use a similar fed-batch cultivation process, there is also the scope to increase efficiency using aerobic continuous cultivation operated to maximise productivity while avoiding the Crabtree effect, as discussed in Section 3.2.
2.2 Yeast production from lignocellulosic biomass
While producing yeast protein from edible sugars can be considered an upgrade in food supply, it would be preferably to increase protein supply using non-edible materials i.e. second generation feedstocks.11 In particular, it is possible to grow yeast on highly abundant lignocellulosic materials, which have been intensely studied as a feedstock for bioethanol production.61 Importantly, the high abundance of lignocellulosic biomass means there is essentially no limit to the amount of biomass that could be produced from this resource. Further, lignocellulosic material can be sourced as wastes from current crop production, or produced at high-efficiency on marginal lands.6 While lignocellulosic must undergo pretreatment using methods such as acid and enzyme hydrolysis to release the fermentable sugars, these processes are well established and have been proven at scale in a range of countries using various feedstocks.12 In addition, biorefinery pathways have been established to produce multiple products in addition to the fermentable sugars, for example from the lignin component, which can improve process economics.12,62 In contrast to bioethanol production, growing yeast biomass as a protein source requires aerobic conditions to maximise biomass yield. Further, for yeast subject to the Crabtree effect, fed-batch or continuous cultivation will be required to avoid high sugar concentrations in the growth medium. An additional consideration for yeast biomass production from lignocellulose is the need to supplement the carbohydrate-rich hydrolysates with a balanced supply of an economical nitrogen source.
A specific challenge for yeast production from lignocellulosic materials, is that the hydrolysates contain a mix of sugars including glucose, xylose, mannose, arabinose and galactose.63 While many yeasts including Candida utilis can grow aerobically on xylose64 and others such as Scheffersomyces stipitis (formerly Pichia stipitis) can even grow anaerobically,63S. cerevisiae is unable to utilise xylose and arabinose necessitating genetic modification for growth on these sugars.65,66 The resulting yeast biomass would therefore be considered a genetically modified food, which could limit its application in some jurisdictions. Theoretically any yeast that can grow on xylose67 could be produced, although due consideration would be needed for the use of any yeast strains that have not yet approved for human consumption.
Another challenge is that lignocellulosic material must be hydrolysed to release the fermentable sugars. In addition to increasing the cost, thermochemical pretreatment used to facilitate enzymatic of hydrolysis of lignocellulose produces toxic byproducts such as furfural and hydroxymethyl furfural (HMF).68 As furfural is toxic to yeast, there is active research into metabolic engineering of furfural-tolerant yeast.69 The potential presence of furfural and HMF in the edible yeast biomass should also be considered. As products of the Maillard reaction, furfural and HMF are inevitably present in many foods, with considerable research being undertaken into concerns for the potential toxicity of these chemicals.70 Although warranting verification, it should be possible to ensure sufficiently low concentrations of these compounds remain in the yeast following washing of the harvested biomass.
Despite these challenges, there are many recent examples of yeast being successfully grown on different biological wastes. For example, Candida utilis was produced as SCP from ammonia-pretreated and enzymatically hydrolysed spent brewers grain71 and from orange peel residues.72Candida maltose was grown on volatile fatty acids produced from acidogenic bacterial fermentation of coffee grinds processed by alkaline pretreatment and subjected to enzyme hydrolysis.73 Five different species of yeast (Candida utilis, Candida tropicalis, Saccharomyces cerevisiae, Saccharomycopsis fibuligera, and Geotrichum candidum) were grown separately and in combination on the sugars contained starch processing wastewater.74 It has also been demonstrated that nitrogen-rich biogas slurry from anaerobic digestors could be used to grow Debaryomyces hansenii, a yeast able to tolerate the high alkali/saline conditions.75 Beyond the yeast strains mentioned so far, oil-bearing yeast such as Yarrowia76 could be grown as a source of both protein and nutritional oils. Importantly, Yarrowia is commonly found in food such as cheese, yoghurt, kefir, and soy sauce66 and has been concluded to be a safe organism.77Yarrowia lipolytica biomass has been produced by direct conversion of food waste and by indirect conversion of volatile fatty acids produced during initial anaerobic bacterial fermentation.78Yarrowia lipolytica and another oleaginous yeast Rhodosporidium toruloides have been cultivated on cocoa fatty acid distillates.79
While the use of waste feedstocks can increase the extent and sustainability of yeast protein production, it must also be economic. A recent technoeconomic analysis of the production of single-cell protein organisms including Candida utilis from steam explosion pretreated and enzymatically hydrolysed wheat straw has been conducted.80 Encouragingly, it was concluded from the analysis that yeast protein production from lignocellulosic biomass had potential to be both technically and economically feasible.80 The promise of producing yeast protein from lignocellulose is further supported by the increasing number of commercial plants producing ethanol,12 a much lower value product than protein, from lignocellulosic feedstocks. Overall, there appears to be vast potential for future production of yeast biomass from a variety of waste feedstocks. Nonetheless, there is much work to be done performing more technoeconomic assessments and life-cycle analyses of yeast biomass production from different feedstocks to provide confidence to establish an industry. This should occur in parallel with the establishment of yeast protein and biomass as bulk food ingredient, which will help to define the market value of the products, a key component in meaningful technoeconomic assessments.
2.3 Yeast as a by-product of recombinant food proteins (‘precision fermentation’)
As mentioned above, there is growing interest in expanding the production of specific food ingredients such as milk proteins and leghemoglobin using genetically modified microorganisms including yeast such as S. cerevisiae and Komagataella phaffii (formerly Pichia pastoris) using so-called ‘precision fermentation’.3,16,81,82 In this case the residual yeast biomass is a byproduct that is produced under food-grade conditions. As such, expansion of this industry could result in a significant new source of yeast biomass for food production. If production of animal-free milk proteins expressed by recombinant yeast is scaled to the extent that it replaces even a small fraction of global milk production, it could result in large amounts of residual yeast biomass. For example, if recombinant yeast were used to produce the equivalent of 1% of global milk protein supply, and assuming a 40
:
1 ratio of biomass-to-recombinant protein,83 >5 Mt per year of yeast biomass would be produced. While recombinant proteins expressed by yeast have been approved for food applications in some jurisdictions such as the USA, it does not appear that the yeast itself has been approved for human consumption and the implications of the recombinant DNA remaining in the yeast cells would need to be considered. In this case, as the yeast growth systems will be optimised with respect to recombinant protein production there is minimal scope to tailor the cellular proteome and physiology of the residual yeast. Both the yeast strain and growth conditions will be selected based on optimisation of the recombinant protein expression rather than biomass productivity or properties. For example, while protein expression platforms have often been developed using strains that growth most rapidly at 30 °C or above, it may be preferrable for protein expression and stability and energy efficiency to operate at a lower temperature.84,85
2.4 Lactose fermenting yeast
Lactose is a disaccharide sugar present in whey, an abundant waste in cheese manufacturing86,87 that is a potential low-cost feedstock for yeast biomass production. However, Saccharomyces is not able metabolise lactose unless it has been pre-hydrolysed into glucose and galactose.88 While this can be done using the enzyme lactase (β-galactosidase),89 to avoid the cost and complication of lactose hydrolysis, it is instead possible to grow yeasts that have the ability to consume lactose directly. Such lactose-utilising yeast include such as certain species of Kluyveromyces90 and Candida91 that can produce lactase.92,93
Much research has been devoted to investigating the use of lactose-fermenting yeast to produce ethanol from whey.50,67,88,94,95 In this case, the yeast is grown anaerobically to maximise ethanol yield, with the yeast biomass a by-product. Yeast biomass is a more valuable product than ethanol, and there have been a few recent studies of the production of yeast extracts and ingredients from lactose fermenting yeast, in particular Kluyveromyces marxianus. This includes production of yeast biomass as single cell protein,96 for yeast extracts,97 and for the production of RNA-rich extracts.98 Importantly for aerobic biomass production, K. marxianus does not exhibit the Crabtree effect, whereas K. lactis produces ethanol under aerobic conditions, but to a lesser extent than S. cerevisiae.99 Importantly for food ingredient production, K. marxianus is considered a GRAS organism (generally recognised as safe), owing to its long history of use in products such as cheese and kafir.100
Candida is another lactose-fermenting yeast that has been investigated for the production of ethanol from acid whey.95Candida species are also encountered in various food applications101 and beer production,102 with a recently published review of literature pertaining to the potential application of Candida yeasts in food applications available.103 However, as some Candida species, in particular Candida albicans,104 are known to be pathogenic, it has been recommended that suitable testing must be done before using a given strain of Candida for applications.101 Arguably the most important species for food applications is Candida utilis, which is the name of asexual/anamorphic form of the yeast Cyberlindnera (Pichia) jadinii.105,106Cyberlindnera jadinii/Candida utilis has long been used for animal feed applications, has GRAS status, and is considered to have much promise for food applications.106 Importantly for aerobic yeast biomass production, species of Candida including Cyberlindnera jadinii/Candida utilis,107Candida tropicalis108 and Candida albicans109 are Crabtree negative, meaning that high biomass yields can be obtained in batch cultures.
Whey is the primary source of lactose, and it can be used directly as the growth medium for yeast production. The composition of whey varies depending on the cheesemaking process, but all forms contain significant amounts of lactose 4.5–6%.94 The concentration of lactose in combination with the growth yield define the yeast biomass concentration that can be obtained. While the lactose concentration can theoretically be increased (e.g. by membrane filtration or evaporation), the additional process step adds complication and cost. The use of concentrated whey can also place osmotic stress on the yeast.110 Whey also contains nitrogen sources including proteinaceous and non-protein nitrogen compounds such as urea.87 The pH of sweet whey is higher (pH 6–7) than that of acid whey (pH < 5).87
3. Controlled production of future yeast biomass supplies
For any future dedicated yeast production facility, there is the opportunity to design the process to tailor the properties of the biomass or to lower the cost of production. Controllable parameters that will influence the properties and cost of the produced biomass include yeast strain selection, reactor design and operation, growth medium composition, the timing of yeast harvesting and the mode of cultivation (Fig. 1). The remaining sections of this review will explore the interactions between yeast cultivation, metabolism and physiology in the context of yeast as a food ingredient. The review focusses on the yeast properties of most importance to food ingredient functionality; the proteome, which determines the amino acid profile and content,32 and the cell wall composition (including β-glucan content) which influences cell strength.111
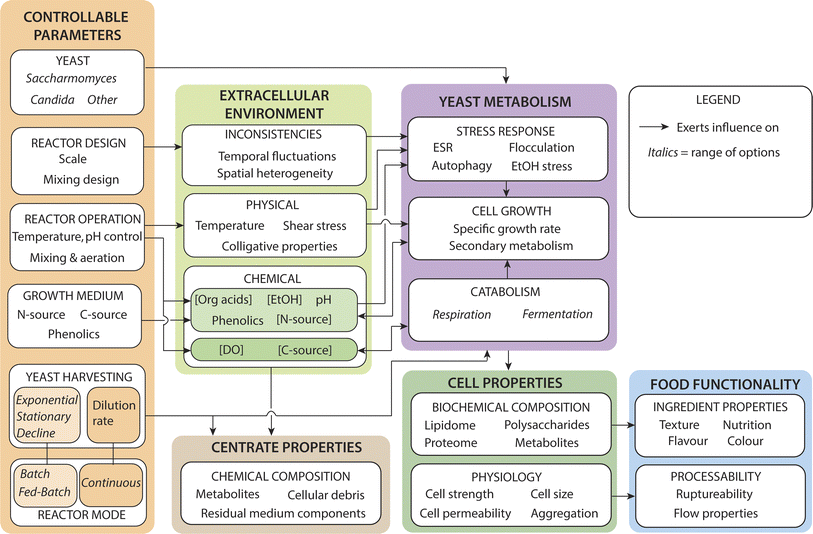 |
| Fig. 1 Diagram illustrating key connections between the controllable parameters for yeast production, the extracellular environment, yeast metabolism, the properties of the harvested cells and centrate, and the functionality of the harvested yeast for food ingredient production. | |
3.1 Leveraging yeast diversity
As a model organism S. cerevisiae has been very widely studied in comparison to other yeast species. Even within this species there is diversity, with measurable metabolic differences between different strains of S. cerevisiae.112,113 As discussed above, accessing alternative feedstocks requires looking beyond S. cerevisiae, and there is an enormous diversity of yeast that could be exploited including 10–16 species of Saccharomyces and >150 species of Candida.105 Metabolic traits such as the Crabtree effect56 and the ability to utilise various sugars differ amongst yeast,63 which directly influences yeast production. In addition, yeast strains and species vary in other production-related parameters such as the maximum specific growth rate114 and tolerance to inhibitors115 including ethanol.116 As such, selection of a yeast species must consider the metabolism and growth performance in relation to the available substrate and overall medium composition.
In addition to affecting the yeast production process, differences in metabolic traits will affect the biochemical composition and physiology of the cells, which will in turn influence the ingredient properties (Fig. 1). Most of the scientific literature pertaining to the key traits of relevance to food ingredient functionality, have been performed on S. cerevisiae. Currently, there is significantly less knowledge of the functional properties of non-Saccharomyces biomass including Yarrowia, Kluyveromyces and Candida as food ingredients. Only some of the many yeast species have been studied in relation to functional food properties, such as probiotic capability and bioactive metabolites.117 However, comparative studies of different yeasts in relation to food ingredient production are lacking and represent a worthwhile topic for future research. One recent study compared yeast extract production from S. cerevisiae, S. boulardii and K. marxianus grown aerobically on glucose in fed-batch culture.97 Levels of volatile metabolites, proteins and amino acids varied between the species, but all yeast extracts contained high levels of flavour-enhancing amino acids including glutamic acid.97 The limited knowledge of non-Saccharomyces yeasts is an impediment to the production of yeast biomass from lactose and lignocellulosic sugars.
The cell wall is a key component of yeast to consider in relation to food ingredient production, typically comprising around 15–30% of the dry weight of yeast cells.105,118 The cell walls of yeasts make the cells physically robust and help control interactions with the extracellular environment.119 They are generally considered to be complex interconnected network comprised of β 1,3-glucan fibres, branched β 1,6-glucan, chitin and glycoproteins.118 While yeast cell walls have the same overall biochemical components, the relative abundance differs considerably.118,120 β-Glucan is a major biochemical component of yeast cell walls (typically 50–70 wt% for S. cerevisiae)121,122 that has received ongoing interest in regard to potential health benefits.123–125 Importantly, the cell walls of Candida126 and Yarrowia,126Cyberlindnera/Pichia jadinii127 and Kluyveromyces128 all include β-glucan, although the exact composition varies. Yeast cell walls also contain proteins as a key structural unit that contributes significantly to the overall protein content of the biomass. A recent in silico proteomic study comparing cell wall proteins expressible amongst 92 different yeasts found that proteins required for cell wall biosynthesis and remodelling were more highly conserved between the species than those involved in flocculation and aggregation.119
3.2 Maximising yeast production efficiency
Expanded yeast production will require the use of less costly and more abundant substrates than molasses. In addition, highly efficient and cost-effective processes that maximise biomass yield and productivity will be needed to commoditise yeast proteins as a food ingredient. Yeast biomass can be produced using three different fermenter operational modes: batch, fed-batch and continuous. These reactor modes have inherent differences that are consequential to many aspects of yeast ingredient production (Fig. 2). Most importantly, continuous fermentation offers much higher volumetric productivities (g L−1 d−1) compared to batch and fed-batch operations due to the avoidance of process interruptions and the sustained high biomass concentrations in the bioreactors.8 As such continuous cultivation has the potential to greatly reduce the cost of future yeast production compared to conventional fed-batch production of baker's yeast. However, prolonged cultivation in continuous cultures can increase the risk of contamination by foreign organisms or genetic variants in the production strain arising from repeated cell division cycles.
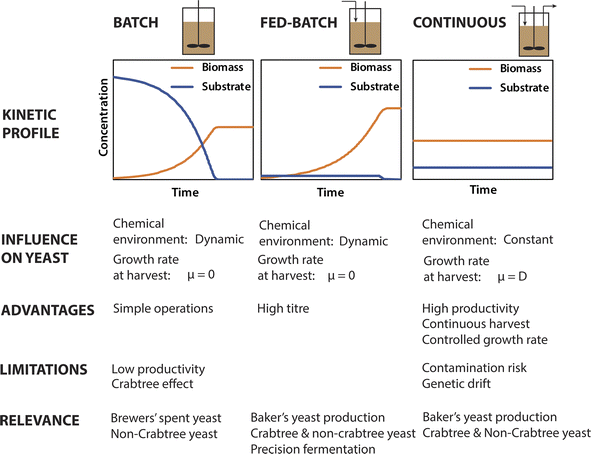 |
| Fig. 2 Comparison of batch, fed-batch and continuous yeast production modes. | |
Continuous baker's yeast production has been attempted industrially in the past,129 however it has not been favoured industrially,130 presumably due to operational challenges such as increased risk of contamination and genetic variants. As baker's yeast must be tightly controlled to ensure consistent activity in baking applications,131 genetic variation and contamination (even by non-pathogenic microbes) presents a major issue. However, these issues may represent less of a problem for yeast biomass produced solely as a protein supply. In which case, the possibility of open, continuous cultivation not involving tight aseptic controls could also be considered to further reduce the costs.132 This could be applicable if strains of yeast can be used that are tolerant to extreme conditions such as low pH or the presence of inhibitory compounds.
Batch and fed-batch operations, which are relevant to beer fermentation and conventional baker's yeast production respectively, are dynamic, involving inconstant extracellular environments (e.g. cell, substrate and metabolite concentrations) and corresponding changes in yeast metabolism and cell physiology (e.g. altered rate cell division, or environmental stress responses). Cells are harvested periodically at the end of batch and fed-batch operations at which point the cells have ceased replication.133 In current practice, baker's yeast is typically grown in fed-batch culture for 12–15 hours, with the cells harvested just 1–2 hours after cessation of substrate feeding,53 representing an early stationary phase. In contrast, continuously harvested biomass is still growing at the specific growth (μ) corresponding to the chosen dilution rate (D). Further, continuous cultures are typically operated at steady state, in which the extracellular environment and yeast metabolism are constant and controlled by operational parameters such as the dilution rate, feed substrate concentration, pH, and temperature. As such, the properties of the harvested cells can be tuned to some extent by controlling operational parameters, in particular the dilution rate (as μ = D). However, as maximal biomass productivity is achieved at high dilution rates close to μmax134 it may not be practical to operate at low dilution rates even if biomass with more favourable functional properties could be produced.
3.3 Effect of production parameters on yeast cell properties
The physiological and metabolic state of the yeast cells upon harvesting define the raw material properties for ingredient manufacture. The effect of production parameters on the properties of the harvested yeast is therefore important to understand. During exponential growth yeast cells divide via budding, using a highly regulated cell cycle.135 Cells have characteristic proteomes and cell physiology tuned to their growth in different environmental conditions. In S. cerevisiae, the rate of cell division (as controlled by chemostat dilution rate) has recently been shown to directly control the allocation of proteins devoted to biosynthesis.136 This is consistent with another study that showed a greater percentage of the proteome of rapidly growing S. cerevisiae is allocated to translation than in slower growing yeast, and that an excess of ribosomes (beyond the active translational capacity) is maintained in order to rapidly respond to more favourable growth conditions.137 Thus, both the overall amount and the relative abundance of different proteins in the S. cerevisiae proteome is directly influenced by growth rate. Proteomic remodelling in relation to growth rate should occur in other relevant yeasts, and this could be a topic for future research.
As explained above, the growth rate of yeast harvested from batch and fed-batch fermenters will be zero, whereas it can be controlled in continuous fermentation (Fig. 2). This has important implications for yeast biomass as a food ingredient, in which protein is the major desirable nutrient. For example, the protein content and amino acid profile of yeast biomass is important to its nutritional value. Importantly, the amino acid content of S. cerevisiae changes as a function of growth conditions, with the total protein and free amino acid content decreasing during the stationary phase.138 More recently, it was shown that differences in the proteomes of yeast grown under different conditions (aerobic versus anaerobic) significantly influenced the amino acid profile of yeast hydrolysates, and therefore their nutritional and taste attributes.32 With further research it may be possible to deliberately influence the amino acid profile of yeast biomass by selecting growth conditions and media for specific strains that result in proteomes with characteristic amino acid profiles, for example rich in specific amino acids.
Yeast cells multiply via budding, which is a complex, highly regulated process requiring controlled growth and division of the cell wall.139 The cell wall composition and strength are important in relation to yeast as a food ingredient. In one study of both lager and ale yeasts there was no significant difference in the mechanical strength of cells harvested at the start, middle or end of a 100 hours batch fermentation.140 However, in a study on baker's yeast,133 cells grown in chemostats at low dilution rates (D = 0.06–0.12 h−1) were found to be stronger than those grown at high dilution rates (D = 0.22–0.28 h−1). Likewise, cells harvested in the late stationary of batch cultures were stronger than those harvested earlier in the batch cycle. It was proposed that the increase in strength at low D could be due to lower proportion of freshly budded cells and/or stronger walls in slower growing yeast.133 Significant differences in the mechanical strength of other microalgae cells has been observed as a function of a prolonged stationary phase (resulting from nitrogen source depletion), which could be attributed to thickened cell walls,111 which is also known to occur in the early stationary phase for S. cerevisiae.121 The physical strength of cells is an important property for ingredient production, which is dependent on the cell wall composition and thickness.111 The mechanical strengths of yeast cells such as S. cerevisiae,141Pichia pastoris,142Yarrowia lipolytica143 are all high, requiring multiple passes through a high-pressure homogeniser at over 1000 bar to achieving a high degree of cell rupture. Data describing the comparative strength of yeast in other genera such as Kluyveromyces and Candida appear to be lacking. Cell strength has been found to be highly dependent on the strain/species of yeast, with twice as much force required to rupture a strain of lager yeast compared to a strain of ale yeast.140 This has major implications for the processability of yeast biomass, as cell rupture is an energy intensive process144 which is dependent on cell strength.111 Therefore, if mechanical cell rupture is to be used to release intracellular components such as whole proteins, it is important to consider cell strength as a parameter in the selection of a candidate yeast strain. Further, as dynamic entities, yeast respond to environmental stimuli via adaptive cellular physiology,145 resulting in divergent physical properties of the cells.
In the stationary phase present in fed-batch cultivation, cells respond to challenges such as starvation or high ethanol concentrations. This is relevant to yeast biomass that is stored following production and prior to processing. These conditions represent environmental stresses that can trigger an environment stress response (ESR) that results in adaptive reprogramming of cell metabolism and physiology including the cell wall.146 The ESR in S. cerevisiae has been studied extensively and involves a complex array of changes, including the expression of various stress response proteins.147,148 In S. cerevisiae the expression of ∼900 genes can be altered as part of the ESR.149 Despite the apparent magnitude of changes in the proteome that result from the expression of various stress proteins, this does not appear to have yet been considered in relation to the functional properties of yeast as food, for example the amino acid profile of the yeast. This is an important aspect to consider, as environmental stresses could be imposed incidentally or deliberately during production to alter the properties of the yeast for food applications.
3.4 Effect of reactor conditions, growth medium and accumulation of metabolites
The extracellular environment in which the yeast grow is determined by the composition of the growth medium and the operation bioreactor or fermenter. As the yeast respond metabolically and physiologically to their environment, yeast biomass properties can be influenced in deliberate ways based on an understanding of yeast responses.
Temperature is an important and controllable parameter that has a major effect on yeast growth rate, metabolite production, physiology and proteome.150 Each strain of yeast has a preferred temperature range,150 below which the growth rate is suboptimal and above which temperature stress occurs.151 For dedicated yeast biomass production, productivity is favoured by operating at optimal growth temperatures. However, for beer and wine production the metabolite profiles are crucial for flavour profiles, and high cell yields are not required. As such, the operational temperature is dictated by flavour considerations and if often suboptimal with respect to growth.17 For recombinant protein production (‘precision fermentation’), the aim is to maximise protein expression, which depends on the concentration of cells in combination with the expression rate per cell. In this case, the growth of yeast is intermediate to the main objectives, and the optimal temperature may152 or may not153 coincide with that which confers maximal growth. Temperature is a controllable parameter in industrial yeast production, although in very large reactors there can be limits to the extent and consistency of cooling that can be provided. As aerobic metabolism is much more exothermic than anaerobic fermentation, the cooling capacity must be much higher. As heat generation is proportional to cell concentration, the cooling load will increase during batch and fed-batch operation, while remaining constant in continuous mode.
Beer fermentation is predominantly anaerobic, as the aim to produce alcohol and flavours rather than biomass. Oxygen is only made available at the start of beer brewing to boost cell growth and shorten the lag phase. For the other yeast production processes including baker's yeast, lactose fermenting yeast and recombinant protein production, aerobic growth is desired to maximise biomass and protein yield. It has recently been shown that the presence or absence of oxygen also influences the protein composition of the yeast with important implications for the flavour and nutritional properties of the biomass.32 Maintaining dissolved oxygen levels is critical to maximising productivity, and for obligate aerobes such as Komagataella phaffii19 it is essential. In S. cerevisiae there is not a straight-forward threshold dissolved oxygen threshold, as it is a combination of dissolved oxygen and glucose concentrations that dictate whether the cells respire aerobically or undergo fermentation.54 Further, high concentrations of dissolved oxygen can result in oxidative stress due to the presence of radical oxygen species.154 The dissolved oxygen concentration in the bioreactor is dependent on the mixing and aeration system (supply) and the oxygen demand, which will increase as a function of cell concentration and specific oxygen demand. Providing oxygen can be a challenge in high-density, aerobic processes, particular at the very large scales of relevance to yeast food production, and can ultimately limit the scale of the reactor.51 Large fermenters also subject the cells to greater environmental fluctuations due to the challenges involved in mixing large volumes, which can influence the physiology of the cells.155
The growth medium is the repository of the required substrate (carbon source) and nitrogen source, and potentially contains other constituents such as phenolics that may influence yeast properties. As shown in Table 1, there is much variation in the characteristics of growth medium used for current and future yeast biomass production. A recent review has highlighted the significant variations in the total protein content (30–70%) of different yeast species grown on different waste substrates, confirming the important influence of growth media on yeast biomass properties.20 Although much effort has been spent on developing ideal growth media for baker's yeast production,156 to a large extent the formulation of media is dictated by external factors such as the cost and availability of the raw materials. The carbon source is the major component of the growth media and a significant contributor to the cost of yeast production. While molasses is less expensive than refined sugar, the use of waste sugars such as lactose or lignocellulosic hydrolysates could help reduce the cost. More needs to be understood about the influence different substrates have on the properties of the resultant yeast, to assist media formulations aimed at balancing product quality and the cost of production.
In addition to the components needed for yeast growth, the media may contain phenolics (e.g. from molasses,49 malt157 or hops158). Phenolics can adsorb onto the cells159 or carry over into the final product in the extracellular growth medium. Yeast metabolites also accumulate in the growth medium that can influence the organoleptic properties of the biomass. While most of extracellular metabolites are removed upon harvesting of the yeast for nutritional applications, their presence can affect the physiology of the yeast cells. In particular, ethanol can invoke stress responses in yeast,160 including altering gene expression and remodelling their lipidome to adapt membranes to high ethanol concentrations.161 Yeasts also have cell wall-associated responses to a range of environmental stresses relating to growth medium components, such as osmotic and chemical stresses.122 For example, S. cerevisiae has been shown to adapt its cell wall (increased stiffness and β-glucan content) in response to acetic acid stress.162 Accumulation of yeast-derived organic acids put a downward pressure on pH, however this can be actively controlled to stay within favourable limits.
3.5 Effect of yeast biomass storage
In addition to the stationary phase occurring the fermenter, it is likely in some instance that yeast cells will be subjected to prolonged periods of storage under starved conditions prior to ingredient processing. This could include transportation from a brewery or yeast production facility and/or storage at a processing plant. Although the yeast can be stored at cold temperatures to slow down metabolic activity and cell death, extended periods of nutrient starvation can eventually trigger autophagy and ultimately cell death. Autophagy is a cellular self-digestion process that occurs in all eukaryotic cells that can help survival by recycling cell resources to essential functions during starvation.163–165 Autophagy can ultimately lead to cell death, which can also occur via programmed necrotic or apoptosis processes.166,167 Cell death results in loss of cellular function, homeostasis and integrity.168 Cell death is linked with autolysis in which hydrolytic enzymes are released from vacuoles to degrade cell constituents, and which is a process that can be intentionally triggered as part of yeast extract production.30,32 Although autophagy and cell death have been extensively studied, there appear to be few dedicated studies investigating these processes in relation to yeast storage and the resulting effect of cell and protein degradation on food ingredient production.
There are clearly very large differences in the metabolism and physiology of yeast growing under different cultivation modes and conditions. Such differences would directly affect any processing performed immediately following harvest. Further, it is also likely that any differences in the harvested yeast would continue to have an influence during subsequent processing or biomass storage (which would represent an extended stationary phase). In this regard, it is important to understand to what extent the metabolic and physiological state of the cells during growth has a permanent effect on the composition or physiology of the harvested cells. However, the dynamics of these changes and the implications for food ingredients have not yet been considered in literature. Most investigations into the dynamics of yeast adaptations have so far involved transcriptomic studies,121,169 which provide insights into how quickly the cells' genetic expression system changes. However, the effects of gene expression take time to materialise in the proteome and cell physiology, which are the characteristics of practical interest for food ingredients. One previous study has been performed on the dynamical response of yeast to anaerobiosis, revealing the changes in the transcriptome occurred much more rapidly (within a single cell cycle) than the resultant remodelling of the cell wall (occurring over at least 4 cell generations).170 The persistent of various cell properties over multiple generations, such as cell wall and protein composition, has important implications for harvested yeast biomass, in which the cells are unlikely to continue cell division during cold storage or processing stages such as autolysis. As such, undertaking further targeted research into the dynamics and persistence of post-production changes in yeast will be important.
4. Research gaps and future direction
There is a wealth of scientific knowledge of yeast that can be applied to production of food ingredients. There is also a rapidly increasing amount of research specifically relating to the production and use of yeast in food. Nonetheless, as a complex and emerging area, there are some important research gaps that should be targeted for future studies.
4.1 Non-Saccharomyces cerevisiae yeasts
While S. cerevisiae has been very widely studied, much less is known about other species making them more difficult to predict and control. In particular, Kluyveromyces, Candida, Yarrowia and Komagataella phaffii have much future relevance for utilisation of lactose and lignocellulosic sugars, and biomass generated from lipid and recombinant protein production and therefore warrant comprehensive investigation.
4.2 Yeast proteome
The extent to which yeast protein and amino acid profiles can be influenced by strain selection or genetic modification, media composition, growth conditions and storage is yet to be fully understood. Further, while the amino acid profile is understood to affect nutrition and flavour of yeast ingredients, the influence of differences in yeast proteomes on other functional properties is less clear and requires controlled studies to be undertaken. The rise of proteomics and genomics presents a new opportunity to understand the extent of protein divergence between different yeast species. It will be interesting to see whether strain selection/modification or controlled growth will be the most effective lever for tailoring yeast biomass with specific protein or amino acid profiles.
4.3 Yeast cell wall
The composition and strength of the cell wall will affect downstream processing and the nutritional and textural properties of yeast ingredients. These cellular properties have been shown to vary between species and as a function of environmental conditions, and further investigation is needed to fully understand how they can be beneficially influenced during production.
4.4 Continuous culture
Continuous culture has potential advantages over conventional fed-batch operation for producing food-grade yeast supplies, including higher productivity and the ability to tune and control growth parameters. To take advantage of this, further research is needed to understand the rates of genetic drift in relation to potential impacts on biomass quality, and how specific growth rates relate to desirable attributes amongst different yeasts.
4.5 Downstream processing
Following production of yeast, the biomass must be harvested and stored, and may be subjected to further downstream processing to convert the biomass into useful products. For example, cell rupture may be required to recover and purify intracellular proteins and extract glucan from cell walls. There is much still to be learned in relation to downstream processing of yeast, in particular for novel strains.
4.6 Yeast as a food
A significant amount of research in the discipline of food science is now needed to characterise the functional properties and digestibility of yeast biomass in the context of different food systems and formulations. It is still not yet properly understood how complex mixtures of yeast proteins and cell wall materials interact with different food matrices. There will be variations in the properties of biomass generated from different feedstock–species–process combinations that are yet to be understood. These variations will represent both a challenge and an opportunity for food scientists making yeast-based protein ingredients. Comparative studies should carefully report the species and growth conditions of the biomass used in experiments to enable links between production and functionality to be determined. Research to inform regulation of not-yet-approved yeast species and GMO yeast is also required, particularly in relation to potential allergenicity and toxicity.
4.7 Technoeconomic assessments (TEA) and life cycle analyses (LCA)
While internal analysis of these technologies can be performed internally by commercial entities, there would be value in publicly available, rigorous TEAs and LCAs that can provide confidence for investment in scaling up of such technologies. Such work can build upon relevant elements of TEAs and LCAs that are already available for lignocellulosic biorefineries171–173 or yeast-derived oils.174
5. Conclusions
Overall, realising the potential of yeast to reduce the environmental impact of food production will require vast increases in the scale of both production and consumption. Major challenges to achieving this include the bringing cost of production down, ensuring adequate supplies of raw materials in particular the carbon source, increasing global fermenter capacity, and understanding how to more extensively utilise yeast protein in human foods. The first challenge will require utilising new sustainable feedstocks, yeast strains and production processes such as continuous cultivation that can drastically reduce the cost of production. The latter challenge will require innovation to incorporate large quantities of yeast proteins into palatable consumer products, and overcoming any regulatory barriers associated recombinant yeast biomass. Leveraging our extensive knowledge of yeast will help realise the opportunity to significantly expand the nutritional yeast industry.
Author contributions
G. Martin wrote and finalised the manuscript. S. Chan contributed to the initial drafting.
Conflicts of interest
The authors have no competing interests to declare.
Acknowledgements
This work was supported by the Australian Research Council and Bega Foods under the Linkage Project funding scheme (project number LP190100090).
References
- M. Henchion, M. Hayes, A. M. Mullen, M. Fenelon and B. Tiwari, Future Protein Supply and Demand: Strategies and Factors Influencing a Sustainable Equilibrium, Foods, 2017, 6(7), 53 CrossRef PubMed.
- F. Humpenöder, B. L. Bodirsky, I. Weindl, H. Lotze-Campen, T. Linder and A. Popp, Projected environmental benefits of replacing beef with microbial protein, Nature, 2022, 605(7908), 90–96, DOI:10.1038/s41586-022-04629-w.
- T. Linder, Making the case for edible microorganisms as an integral part of a more sustainable and resilient food production system, Food Secur., 2019, 11(2), 265–278, DOI:10.1007/s12571-019-00912-3.
- A. Durkin, T. Finnigan, R. Johnson, J. Kazer, J. Yu, D. Stuckey and M. Guo, Can closed-loop microbial protein provide sustainable protein security against the hunger pandemic?, Curr. Res. Biotechnol., 2022, 4, 365–376, DOI:10.1016/j.crbiot.2022.09.001.
- S. Matassa, N. Boon, I. Pikaar and W. Verstraete, Microbial protein: future sustainable food supply route with low environmental footprint, Microb. Biotechnol., 2016, 9(5), 568–575, DOI:10.1111/1751-7915.12369.
- T. Upcraft, W.-C. Tu, R. Johnson, T. Finnigan, N. Van Hung, J. Hallett and M. Guo, Protein from renewable resources: mycoprotein production from agricultural residues, Green Chem., 2021, 23(14), 5150–5165, 10.1039/D1GC01021B.
- R. P. Anupama, Value-added food:: Single cell protein, Biotechnol. Adv., 2000, 18(6), 459–479, DOI:10.1016/S0734-9750(00)00045-8.
- R. B. Vasey and K. A. Powell, Single-Cell Protein, Biotechnol. Genet. Eng. Rev., 1984, 2(1), 285–311, DOI:10.1080/02648725.1984.10647802.
- A. Ritala, S. T. Häkkinen, M. Toivari and M. G. Wiebe, Single Cell Protein—State-of-the-Art, Industrial Landscape and Patents 2001–2016, Front. Microbiol., 2017, 8 DOI:10.3389/fmicb.2017.02009.
- B. Bajić, D. Vučurović, Đ. Vasić, R. Jevtić-Mučibabić and S. Dodić, Biotechnological Production of Sustainable Microbial Proteins from Agro-Industrial Residues and By-Products, Foods, 2022, 12(1), 107, DOI:10.3390/foods12010107.
- M. Areniello, S. Matassa, G. Esposito and P. N. L. Lens, Biowaste upcycling into second-generation microbial protein through mixed-culture fermentation, Trends Biotechnol., 2023, 41(2), 197–213, DOI:10.1016/j.tibtech.2022.07.008.
- N. Singh, R. R. Singhania, P. S. Nigam, C.-D. Dong, A. K. Patel and M. Puri, Global status of lignocellulosic biorefinery: Challenges and perspectives, Bioresour. Technol., 2022, 344, 126415, DOI:10.1016/j.biortech.2021.126415.
- S. K. Nandy and R. K. Srivastava, A review on sustainable yeast biotechnological processes and applications, Microbiol. Res., 2018, 207, 83–90, DOI:10.1016/j.micres.2017.11.013.
- F. Randez-Gil, I. Córcoles-Sáez and J. A. Prieto, Genetic and phenotypic characteristics of baker's yeast: relevance to baking, Annu. Rev. Food Sci. Technol., 2013, 4, 191–214, DOI:10.1146/annurev-food-030212-182609.
- S. Maicas, The Role of Yeasts in Fermentation Processes, Microorganisms, 2020, 8(8), 1142, DOI:10.3390/microorganisms8081142.
- K. F. Chai, K. R. Ng, M. Samarasiri and W. N. Chen, Precision fermentation to advance fungal food fermentations, Curr. Opin. Food Sci., 2022, 47, 100881, DOI:10.1016/j.cofs.2022.100881.
-
D. E. Briggs, C. A. Boutlon, P. A. Brookers and R. Stevens, Brewing: Science and Practice, 2004 Search PubMed.
- A. R. Gorter de Vries, J. T. Pronk and J. G. Daran, Lager-brewing yeasts in the era of modern genetics, FEMS Yeast Res., 2019, 19(7) DOI:10.1093/femsyr/foz063.
- L. Bernauer, A. Radkohl, L. G. K. Lehmayer and A. Emmerstorfer-Augustin, Komagataella phaffii as Emerging Model Organism in Fundamental Research, Front. Microbiol., 2021, 11 DOI:10.3389/fmicb.2020.607028.
- M. E. Jach, A. Serefko, M. Ziaja and M. Kieliszek, Yeast Protein as an Easily Accessible Food Source, Metabolites, 2022, 12(1), 63 CrossRef CAS PubMed.
- M. Ciudad-Mulero, V. Fernández-Ruiz, C. Cuadrado, C. Arribas, M. M. Pedrosa, J. De, J. Berrios, J. Pan and P. Morales, Novel gluten-free formulations from lentil flours and nutritional yeast: Evaluation of extrusion effect on phytochemicals and non-nutritional factors, Food Chem., 2020, 315, 126175, DOI:10.1016/j.foodchem.2020.126175.
- M. Ciudad-Mulero, L. Barros, Â. Fernandes, J. D. J. Berrios, M. Cámara, P. Morales, V. Fernández-Ruiz and I. C. F. R. Ferreira, Bioactive compounds and antioxidant capacity of extruded snack-type products developed from novel formulations of lentil and nutritional yeast flours, Food Funct., 2018, 9(2), 819–829, 10.1039/C7FO01730H.
- E. A. Yamada and V. C. Sgarbieri, Yeast (Saccharomyces cerevisiae) Protein Concentrate: Preparation, Chemical Composition, and Nutritional and Functional Properties, J. Agric. Food Chem., 2005, 53(10), 3931–3936, DOI:10.1021/jf0400821.
-
P. Coelho, N. Komora, A. Xavier, F. Centeno and A. Raymundo, New Formulas in Mayonnaise Production – A New Rheological Perspective for Systems with Yeast Protein Extract (YPE), In Proceedings of the Iberian Meeting on Rheology (IBEREO 2024), ed. A. Raymundo, V. D. Alves, I. Sousa and F. J. Galindo-Rosales, Springer Nature Switzerland, 2024, Cham, pp 181–184 Search PubMed.
- A. Singh, M. Meena, D. Kumar, A. K. Dubey and M. I. Hassan, Structural and functional analysis of various globulin proteins from soy seed, Crit. Rev. Food Sci. Nutr., 2015, 55(11), 1491–1502, DOI:10.1080/10408398.2012.700340.
- N. Grasso, N. L. Lynch, E. K. Arendt and J. A. O'Mahony, Chickpea protein ingredients: A review of composition, functionality, and applications, Compr. Rev. Food Sci. Food Saf., 2022, 21(1), 435–452, DOI:10.1111/1541-4337.12878.
- M. Southan and F. MacRitchie, Molecular Weight Distribution of Wheat Proteins, Cereal Chem., 1999, 76(6), 827–836, DOI:10.1094/CCHEM.1999.76.6.827.
- Z. Tao, H. Yuan, M. Liu, Q. Liu, S. Zhang, H. Liu, Y. Jiang, D. Huang and T. Wang, Yeast Extract: Characteristics, Production, Applications and Future Perspectives, J. Microbiol. Biotechnol., 2023, 33(2), 151–166, DOI:10.4014/jmb.2207.07057.
-
LeSaffre, Global Yeast Extract Market, 2018, https://biospringer.com/wp-content/uploads/2018/07/GLOBAL-YEAST-EXTRACT-MARKET-2018-BIOSPRINGER.pdf, accessed 2019 30 June.
- D. Tomé, Yeast Extracts: Nutritional and Flavoring Food Ingredients, ACS Food Sci. Technol., 2021, 1(4), 487–494, DOI:10.1021/acsfoodscitech.0c00131.
- T. F. Reyes, Y. Chen, R. Z. Fraser, T. Chan and X. Li, Assessment of the potential allergenicity and toxicity of Pichia proteins in a novel leghemoglobin preparation, Regul. Toxicol. Pharmacol., 2021, 119, 104817, DOI:10.1016/j.yrtph.2020.104817.
- S. Sirisena, S. Chan, N. Roberts, S. Dal Maso, S. L. Gras and G. J. O. Martin, Influence of yeast growth conditions and proteolytic enzymes on the amino acid profiles of yeast hydrolysates: Implications for taste and nutrition, Food Chem., 2024, 437, 137906, DOI:10.1016/j.foodchem.2023.137906.
- E. F. Vieira, J. Carvalho, E. Pinto, S. Cunha, A. A. Almeida and I. M. P. L. V. O. Ferreira, Nutritive value, antioxidant activity and phenolic compounds profile of brewer's spent yeast extract, J. Food Compos. Anal., 2016, 52, 44–51, DOI:10.1016/j.jfca.2016.07.006.
- A. Ritala, S. T. Hakkinen, M. Toivari and M. G. Wiebe, Single Cell Protein—State-of-the-Art, Industrial Landscape and Patents 2001–2016, Front. Microbiol., 2017, 8, 2009 CrossRef PubMed.
- J. Ma, Y. Sun, D. Meng, Z. Zhou, Y. Zhang and R. Yang, Yeast proteins: The novel and sustainable alternative protein in food applications, Trends Food Sci. Technol., 2023, 135, 190–201, DOI:10.1016/j.tifs.2023.04.003.
- H. Sun, Y. Sun, X. Tang, Y. Cui, D. Meng, Y. Zhang, K. Li, H. Guo, H. Chen and R. Yang, The interaction mechanism and the functionality of yeast protein with hydrophilic and hydrophobic bioactive molecules, Food Biosci., 2023, 52, 102448, DOI:10.1016/j.fbio.2023.102448.
- S. Wang, F. Huang, Y. Zhao, K. Ouyang, H. Xie, H. Xiong, Y. Zhang, Z. Chen and Q. Zhao, Slow-digestive yeast protein concentrate: An investigation of its in vitro digestibility and digestion behavior, Food Res. Int., 2023, 174(Pt 1), 113572, DOI:10.1016/j.foodres.2023.113572.
- A. E. V. Martini, M. W. Miller and A. Martini, Amino acid composition of whole cells of different yeasts, J. Agric. Food Chem., 1979, 27(5), 982–984 CrossRef CAS PubMed.
- Y. Chen, F. Li and J. Nielsen, Genome-scale modeling of yeast metabolism: retrospectives and perspectives, FEMS Yeast Res., 2022, 22(1) DOI:10.1093/femsyr/foac003.
- J. Nielsen, Yeast systems biology: model organism and cell factory, Biotechnol. J., 2019 DOI:10.1002/biot.201800421.
- M. F. Cesur, T. Çakır and P. Pir, Genome-Wide Analysis of Yeast Metabolic Cycle through Metabolic Network Models Reveals Superiority of Integrated ATAC-seq Data over RNA-seq Data, mSystems, 2022, 7(3), e01347, DOI:10.1128/msystems.01347-21.
- A. Jaeger, E. K. Arendt, E. Zannini and A. W. Sahin, Brewer's Spent Yeast (BSY), an Underutilized Brewing By-Product, Fermentation, 2020, 6(4), 123 CrossRef CAS.
- A. Zeko-Pivač, K. Habschied, B. Kulisic, I. Barkow and M. Tišma, Valorization of Spent Brewer's Yeast for the Production of High-Value Products, Materials, and Biofuels and Environmental Application, Fermentation, 2023, 9(3), 208 CrossRef.
- A. De Iseppi, G. Lomolino, M. Marangon and A. Curioni, Current and future strategies for wine yeast lees valorization, Food Res. Int., 2020, 137, 109352, DOI:10.1016/j.foodres.2020.109352.
- J. Lisičar, T. Scheper and S. Barbe, Turning Industrial Baker's Yeast Manufacture into a Powerful Zero Discharge Multipurpose Bioprocess, Ind. Biotechnol., 2017, 13(4), 184–191, DOI:10.1089/ind.2017.0018.
- J. Lisičar Vukušić, T. Millenautzki and S. Barbe, Bridging the Implementation Gap between Pomace Waste and Large-Scale Baker's Yeast Production, AgriEngineering, 2023, 5(4), 2238–2252 CrossRef.
- A. O. Ejiofor, Y. Chisti and M. Moo-Young, Culture of Saccharomyces cerevisiae on hydrolyzed waste cassava starch for production of baking-quality yeast, Enzyme Microb. Technol., 1996, 18(7), 519–525, DOI:10.1016/0141-0229(95)00166-2.
- I. Keturah, B. Sandrasegarampillai and A. Vasanthy, Baker's yeast biomass production with rice as carbon and soy meal as nitrogen sources, Malays. J. Microbiol., 2014, 10(3), 205–214 Search PubMed.
- F. Kong, S. Yu, F. Zeng and X. Wu, Phenolics Content and Inhibitory Effect of Sugarcane Molasses on α-Glucosidase and α-Amylase In Vitro, Sugar Technol., 2016, 18(4), 333–339, DOI:10.1007/s12355-015-0385-y.
- A. J. Mawson, Bioconversions for whey utilization and waste abatement, Bioresour. Technol., 1994, 47(3), 195–203, DOI:10.1016/0960-8524(94)90180-5.
- P. V. Attfield, Stress tolerance: The key to effective strains of industrial baker's yeast, Nat. Biotechnol., 1997, 15(13), 1351–1357, DOI:10.1038/nbt1297-1351.
- J. Zamani, P. Pournia and H. A. Seirafi, A novel feeding method in commercial Baker's yeast production, J. Appl. Microbiol., 2008, 105(3), 674–680, DOI:10.1111/j.1365-2672.2008.03781.x.
- S. George, G. Larsson, K. Olsson and S. O. Enfors, Comparison of the Baker's yeast process performance in laboratory and production scale, Bioprocess Eng., 1998, 18(2), 135–142, DOI:10.1007/PL00008979.
- K. Furukawa, E. Heinzle and I. J. Dunn, Influence of oxygen on the growth of Saccharomyces cerevisiae in continuous culture, Biotechnol. Bioeng., 1983, 25(10), 2293–2317, DOI:10.1002/bit.260251003.
- C. Malina, R. Yu, J. Björkeroth, E. J. Kerkhoven and J. Nielsen, Adaptations in metabolism and protein translation give rise to the Crabtree effect in yeast, Proc. Natl. Acad. Sci. U. S. A., 2021, 118(51), e2112836118, DOI:10.1073/pnas.2112836118.
- T. Pfeiffer and A. Morley, An evolutionary perspective on the Crabtree effect, Front. Mol. Biosci., 2014, 1 DOI:10.3389/fmolb.2014.00017.
- K. Dairaku, Y. Yamasaki, K. Kuki, S. Shioya and T. Takamatsu, Maximum production in a bakers' yeast fed-batch culture by a tubing method, Biotechnol. Bioeng., 1981, 23(9), 2069–2081, DOI:10.1002/bit.260230911.
- S. O. Enfors, J. Hedenberg and K. Olsson, Simulation of the dynamics in the Baker's yeast process, Bioprocess Eng., 1990, 5(5), 191–198, DOI:10.1007/BF00376225.
- F. Randez-Gil, P. Sanz and J. A. Prieto, Engineering baker's yeast: room for improvement, Trends Biotechnol., 1999, 17(6), 237–244, DOI:10.1016/s0167-7799(99)01318-9.
-
R. Joseph, Yeasts: production and commercial uses, In Encyclopedia of Food Microbiology, ed. R. K. Robinson, Elsevier, 1999, pp 2335–2341 Search PubMed.
- B. Hahn-Hägerdal, M. Galbe, M. F. Gorwa-Grauslund, G. Lidén and G. Zacchi, Bio-ethanol – the fuel of tomorrow from the residues of today, Trends Biotechnol., 2006, 24(12), 549–556, DOI:10.1016/j.tibtech.2006.10.004.
- V. Sharma, M.-L. Tsai, P. Nargotra, C.-W. Chen, P.-P. Sun, R. R. Singhania, A. K. Patel and C.-D. Dong, Journey of lignin from a roadblock to bridge for lignocellulose biorefineries: A comprehensive review, Sci. Total Environ., 2023, 861, 160560, DOI:10.1016/j.scitotenv.2022.160560.
- J. M. Francois, C. Alkim and N. Morin, Engineering microbial pathways for production of bio-based chemicals from lignocellulosic sugars: current status and perspectives, Biotechnol. Biofuels, 2020, 13(1), 118, DOI:10.1186/s13068-020-01744-6.
- H. Tamakawa, S. Ikushima and S. Yoshida, Ethanol Production from Xylose by a Recombinant Candida utilis Strain Expressing Protein-Engineered Xylose Reductase and Xylitol Dehydrogenase, Biosci., Biotechnol., Biochem., 2011, 75(10), 1994–2000, DOI:10.1271/bbb.110426.
- J. T. Cunha, P. O. Soares, A. Romaní, J. M. Thevelein and L. Domingues, Xylose fermentation efficiency of industrial Saccharomyces cerevisiae yeast with separate or combined xylose reductase/xylitol dehydrogenase and xylose isomerase pathways, Biotechnol. Biofuels, 2019, 12(1), 20, DOI:10.1186/s13068-019-1360-8.
- M. Bettiga, O. Bengtsson, B. Hahn-Hägerdal and M. F. Gorwa-Grauslund, Arabinose and xylose fermentation by recombinant Saccharomyces cerevisiae expressing a fungal pentose utilization pathway, Microb. Cell Factories, 2009, 8(1), 40, DOI:10.1186/1475-2859-8-40.
- A. Tesfaw, The current trends of bioethanol production from cheese whey using yeasts: biological and economical perspectives, Front. Energy Res., 2023, 11 DOI:10.3389/fenrg.2023.1183035.
- W. Dessie, F. Xin, W. Zhang, J. Zhou, H. Wu, J. Ma and M. Jiang, Inhibitory effects of lignocellulose pretreatment degradation products (hydroxymethylfurfural
and furfural) on succinic acid producing Actinobacillus succinogenes, Biochem. Eng. J., 2019, 150, 107263, DOI:10.1016/j.bej.2019.107263.
- S. B. Jilani and D. G. Olson, Mechanism of furfural toxicity and metabolic strategies to engineer tolerance in microbial strains, Microb. Cell Factories, 2023, 22(1), 221, DOI:10.1186/s12934-023-02223-x.
- E. Agcam, A Kinetic Approach to Explain Hydroxymethylfurfural and Furfural Formations Induced by Maillard, Caramelization, and Ascorbic Acid Degradation Reactions in Fruit Juice-Based Mediums, Food Anal. Methods, 2022, 15(5), 1286–1299, DOI:10.1007/s12161-021-02214-x.
- W. Sun, Z. Zhang, X. Li, X. Lu, G. Liu, Y. Qin, J. Zhao and Y. Qu, Production of single cell protein from brewer's spent grain through enzymatic saccharification and fermentation enhanced by ammoniation pretreatment, Bioresour. Technol., 2024, 394, 130242, DOI:10.1016/j.biortech.2023.130242.
- R. C. Carranza-Méndez, M. L. Chávez-González, L. Sepúlveda-Torre, C. N. Aguilar, M. Govea-Salas and R. Ramos-González, Production of single cell protein from orange peel residues by Candida utilis, Biocatal. Agric. Biotechnol., 2022, 40, 102298, DOI:10.1016/j.bcab.2022.102298.
- U. Jomnonkhaow, P. Plangklang, A. Reungsang, C.-Y. Peng and C.-Y. Chu, Valorization of spent coffee grounds through integrated bioprocess of fermentable sugars, volatile fatty acids, yeast-based single-cell protein and biofuels production, Bioresour. Technol., 2024, 393, 130107, DOI:10.1016/j.biortech.2023.130107.
- Y. Tian, J. Li, J. Meng and J. Li, High-yield production of single-cell protein from starch processing wastewater using co-cultivation of yeasts, Bioresour. Technol., 2023, 370, 128527, DOI:10.1016/j.biortech.2022.128527.
- K. Liu, S. Huang, L. Zhang, Y. Xiong, X. Wang, Y. Bao, D. Li and J. Li, Efficient production of single cell protein from biogas slurry using screened alkali-salt-tolerant Debaryomyces hansenii, Bioresour. Technol., 2024, 393, 130119, DOI:10.1016/j.biortech.2023.130119.
- J. Mano, N. Liu, J. H. Hammond, D. H. Currie and G. Stephanopoulos, Engineering Yarrowia lipolytica for the utilization of acid whey, Metab. Eng., 2020, 57, 43–50, DOI:10.1016/j.ymben.2019.09.010.
- M. Groenewald, T. Boekhout, C. Neuvéglise, C. Gaillardin, P. W. M. van Dijck and M. Wyss, Yarrowia lipolytica: Safety assessment of an oleaginous yeast with a great industrial potential, Crit. Rev. Microbiol., 2014, 40(3), 187–206, DOI:10.3109/1040841X.2013.770386.
- R. Yang, Z. Chen, P. Hu, S. Zhang and G. Luo, Two-stage fermentation enhanced single-cell protein production by Yarrowia lipolytica from food waste, Bioresour. Technol., 2022, 361, 127677, DOI:10.1016/j.biortech.2022.127677.
- E. C. Peterson, R. Siao, G. G. Chua, C. T. Busran, R. Pavlovic, A. Thong, C. Hermansen, N. Sofeo, Y. Kanagasundaram, M. Weingarten and N. Lindley, Single cell protein and oil production from solid cocoa fatty acid distillates co-fed ethanol, Bioresour. Technol., 2023, 387, 129630, DOI:10.1016/j.biortech.2023.129630.
- E. Voutilainen, V. Pihlajaniemi and T. Parviainen, Economic comparison of food protein production with single-cell organisms from lignocellulose side-streams, Bioresour. Technol. Rep., 2021, 14, 100683, DOI:10.1016/j.biteb.2021.100683.
- M. Banovic and K. G. Grunert, Consumer acceptance of precision fermentation technology: A cross-cultural study, Innovat. Food Sci. Emerg. Technol., 2023, 88, 103435, DOI:10.1016/j.ifset.2023.103435.
- M. A. Augustin, C. J. Hartley, G. Maloney and S. Tyndall, Innovation in precision fermentation for food ingredients, Crit. Rev. Food Sci. Nutr., 2023, 1–21, DOI:10.1080/10408398.2023.2166014.
- M. Huang, G. Wang, J. Qin, D. Petranovic and J. Nielsen, Engineering
the protein secretory pathway of Saccharomyces cerevisiae enables improved protein production, Proc. Natl. Acad. Sci. U. S. A., 2018, 115(47), E11025–e11032, DOI:10.1073/pnas.1809921115.
- K.-K. So, N. M. T. Le, N.-L. Nguyen and D.-H. Kim, Improving expression and assembly of difficult-to-express heterologous proteins in Saccharomyces cerevisiae by culturing at a sub-physiological temperature, Microb. Cell Factories, 2023, 22(1), 55, DOI:10.1186/s12934-023-02065-7.
- M. Karbalaei, S. A. Rezaee and H. Farsiani, Pichia pastoris: A highly successful expression system for optimal synthesis of heterologous proteins, J. Cell. Physiol., 2020, 235(9), 5867–5881, DOI:10.1002/jcp.29583.
- G. W. Smithers, Whey-ing up the options – Yesterday, today and tomorrow, Int. Dairy J., 2015, 48, 2–14, DOI:10.1016/j.idairyj.2015.01.011.
- M. I. G. Siso, The biotechnological utilization of cheese whey: A review, Bioresour. Technol., 1996, 57(1), 1–11, DOI:10.1016/0960-8524(96)00036-3.
- P. M. R. Guimarães, J. A. Teixeira and L. Domingues, Fermentation of lactose to bio-ethanol by yeasts as part of integrated solutions for the valorisation of cheese whey, Biotechnol. Adv., 2010, 28(3), 375–384, DOI:10.1016/j.biotechadv.2010.02.002.
- P. Schulz and S. S. H. Rizvi, Hydrolysis of Lactose in Milk: Current Status and Future Products, Food Rev. Int., 2021, 1–20, DOI:10.1080/87559129.2021.1983590.
- J. A. Varela, M. Puricelli, R. A. Ortiz-Merino, R. Giacomobono, S. Braun-Galleani, K. H. Wolfe and J. P. Morrissey, Origin of Lactose Fermentation in Kluyveromyces lactis by Interspecies Transfer of a Neo-functionalized Gene Cluster during Domestication, Curr. Biol., 2019, 29(24), 4284–4290, DOI:10.1016/j.cub.2019.10.044.
- O. D. Ianieva, H. O. Voronina and V. S. Pidhors'kyi, [Isolation and characterization of lactose-fermenting yeasts Candida kefyr], Tsitol. Genetika, 2013, 47(6), 43–50 CAS.
-
C. Belloch, A. Querol and E. Barrio, Yeasts and molds – Kluyveromyces spp, Encyclopedia of Dairy Sciences, 2011, pp. 754–764, DOI:10.1016/B978-0-12-374407-4.00499-4.
- S. A. de Bales and F. J. Castillo, Production of Lactase by Candida pseudotropicalis Grown in Whey, Appl. Environ. Microbiol., 1979, 37(6), 1201–1205, DOI:10.1128/aem.37.6.1201-1205.1979.
- A. R. Prazeres, F. Carvalho and J. Rivas, Cheese whey management: A review, J. Environ. Manage., 2012, 110, 48–68, DOI:10.1016/j.jenvman.2012.05.018.
- A. E. Ghaly and A. A. El-Taweel, Effect of micro-aeration on the growth of Candida pseudotropicalis and production of ethanol during batch fermentation of cheese whey, Bioresour. Technol., 1995, 52(3), 203–217 CrossRef CAS.
- J. S. S. Yadav, S. Yan, C. M. Ajila, J. Bezawada, R. D. Tyagi and R. Y. Surampalli, Food-grade single-cell protein production, characterization and ultrafiltration recovery of residual fermented whey proteins from whey, Food Bioprod. Process., 2016, 99, 156–165, DOI:10.1016/j.fbp.2016.04.012.
- F. Demirgül, Ö. Şimşek, F. Bozkurt, E. Dertli and O. Sağdıç, Production and characterization of yeast extracts produced by Saccharomyces cerevisiae, Saccharomyces boulardii and Kluyveromyces marxianus, Prep. Biochem. Biotechnol., 2022, 52(6), 657–667, DOI:10.1080/10826068.2021.1983833.
- R. Palma, A. Brandelli and M. Ayub, Production of yeast extract from whey using Kluyveromyces marxianus, Braz. Arch. Biol. Technol., 2003, 46 DOI:10.1590/S1516-89132003000100017.
- A. Hagman, T. Säll and J. Piškur, Analysis of the yeast short-term Crabtree effect and its origin, FEBS J., 2014, 281(21), 4805–4814, DOI:10.1111/febs.13019.
- S.-C. Lo, C.-Y. Yang, D. C. Mathew and C.-C. Huang, Growth and autolysis of the kefir yeast Kluyveromyces marxianus in lactate culture, Sci. Rep., 2021, 11(1), 14552, DOI:10.1038/s41598-021-94101-y.
- G. V. de Melo Pereira, B. L. Maske, D. P. de Carvalho Neto, S. G. Karp, J. De Dea Lindner, J. G. P. Martin, B. de Oliveira Hosken and C. R. Soccol, What Is Candida Doing in My Food? A Review and Safety Alert on Its Use as Starter Cultures in Fermented Foods, Microorganisms, 2022, 10(9) DOI:10.3390/microorganisms10091855.
- L. Petruzzi, M. Corbo, M. Sinigaglia and A. Bevilacqua, Brewers's Yeast in Controlled and Uncontrolled Fermentation, with a Focus on Novel, Non-Conventional and Superior Strains, Food Rev. Int., 2015, 32, 150725081628007, DOI:10.1080/87559129.2015.1075211.
- M. Kieliszek, A. M. Kot, A. Bzducha-Wróbel, S. BŁażejak, I. Gientka and A. Kurcz, Biotechnological use of Candida yeasts in the food industry: A review, Fungal Biol. Rev., 2017, 31(4), 185–198, DOI:10.1016/j.fbr.2017.06.001.
- C. J. Nobile and A. D. Johnson, Candida albicans Biofilms and Human Disease, Annu. Rev. Microbiol., 2015, 69, 71–92, DOI:10.1146/annurev-micro-091014-104330.
-
J. Webster and R. Weber, Introduction to Fungi, Cambridge University Press, 2007, DOI:10.1017/CBO9780511809026.
- M. Sousa-Silva, D. Vieira, P. Soares, M. Casal and I. Soares-Silva, Expanding the Knowledge on the Skillful Yeast Cyberlindnera jadinii, J. Fungi, 2021, 7(1), 36 CrossRef CAS PubMed.
- M. Imura, K. Nitta, R. Iwakiri, F. Matsuda, H. Shimizu and E. Fukusaki, Comparison of metabolic profiles of yeasts based on the difference of the Crabtree positive and negative, J. Biosci. Bioeng., 2020, 129(1), 52–58, DOI:10.1016/j.jbiosc.2019.07.007.
- R. H. De Deken, The Crabtree Effect: A Regulatory System in Yeast, Microbiology, 1966, 44(2), 149–156, DOI:10.1099/00221287-44-2-149.
- S. Dashko, N. Zhou, C. Compagno and J. Piškur, Why, when, and how did yeast evolve alcoholic fermentation?, FEMS Yeast Res., 2014, 14(6), 826–832, DOI:10.1111/1567-1364.12161.
- S. Ozmihci and F. Kargi, Ethanol fermentation of cheese whey powder solution by repeated fed-batch operation, Enzyme Microb. Technol., 2007, 41(1), 169–174, DOI:10.1016/j.enzmictec.2006.12.016.
- B. H. J. Yap, S. A. Crawford, R. R. Dagastine, P. J. Scales and G. J. O. Martin, Nitrogen deprivation of microalgae: effect on cell size, cell wall thickness, cell strength, and resistance to mechanical rupture, J. Ind. Microbiol. Biotechnol., 2016, 43(12), 1671–1680, DOI:10.1007/s10295-016-1848-1.
- C. Camarasa, I. Sanchez, P. Brial, F. Bigey and S. Dequin, Phenotypic landscape of Saccharomyces cerevisiae during wine fermentation: evidence for origin-dependent metabolic traits, PLoS One, 2011, 6(9), e25147 CrossRef CAS PubMed.
- K. Uebayashi, H. Shimizu and F. Matsuda, Comparative analysis of fermentation and enzyme expression profiles among industrial Saccharomyces cerevisiae strains, Appl. Microbiol. Biotechnol., 2018, 102(16), 7071–7081, DOI:10.1007/s00253-018-9128-9.
- P. Monteiro de Oliveira, D. Aborneva, N. Bonturi and P.-J. Lahtvee, Screening and Growth Characterization of Non-conventional Yeasts in a Hemicellulosic Hydrolysate, Front. Bioeng. Biotechnol., 2021, 9 DOI:10.3389/fbioe.2021.659472.
- S. Rebello, A. Abraham, A. Madhavan, R. Sindhu, P. Binod, A. Karthika Bahuleyan, E. M. Aneesh and A. Pandey, Non-conventional yeast cell factories for sustainable bioprocesses, FEMS Microbiol. Lett., 2018, 365(21) DOI:10.1093/femsle/fny222.
- I. A. Ndubuisi, C. O. Amadi, T. N. Nwagu, Y. Murata and J. C. Ogbonna, Non-conventional yeast strains: Unexploited resources for effective commercialization of second generation bioethanol, Biotechnol. Adv., 2023, 63, 108100, DOI:10.1016/j.biotechadv.2023.108100.
- A. K. Rai, A. Pandey and D. Sahoo, Biotechnological potential of yeasts in functional food industry, Trends Food Sci. Technol., 2019, 83, 129–137, DOI:10.1016/j.tifs.2018.11.016.
- X. Xie and P. N. Lipke, On the evolution of fungal and yeast cell walls, Yeast, 2010, 27(8), 479–488, DOI:10.1002/yea.1787.
- M. Lozančić, B. Žunar, D. Hrestak, K. Lopandić, R. Teparić and V. Mrša, Systematic Comparison of Cell Wall-Related Proteins of Different Yeasts, J. Fungi, 2021, 7(2), 128 CrossRef PubMed.
- G. V. Gautério, R. M. da Silva, F. C. Karraz, M. A. Z. Coelho, B. D. Ribeiro and A. C. Lemes, Cell disruption and permeabilization methods for obtaining yeast bioproducts, Cleaner Chem. Eng., 2023, 6, 100112, DOI:10.1016/j.clce.2023.100112.
- F. M. Klis, P. Mol, K. Hellingwerf and S. Brul, Dynamics of cell wall structure in Saccharomyces cerevisiae, FEMS Microbiol. Rev., 2002, 26(3), 239–256, DOI:10.1016/S0168-6445(02)00087-6.
- R. A. Ribeiro, N. Bourbon-Melo and I. Sá-Correia, The cell wall and the response and tolerance to stresses of biotechnological relevance in yeasts, Front. Microbiol., 2022, 13 DOI:10.3389/fmicb.2022.953479.
- M. Vlassopoulou, M. Yannakoulia, V. Pletsa, G. I. Zervakis and A. Kyriacou, Effects of fungal beta-glucans on health – a systematic review of randomized controlled trials, Food Funct., 2021, 12(8), 3366–3380, 10.1039/D1FO00122A.
- M. M. Canaan, J. C. Reis-Canaan, M. G. Zangerônimo, E. F. Andrade, T. Gonçalves, M. C. A. Pereira, R. R. Lima, V. Pardi, R. M. Murata and L. J. Pereira, Yeast Beta-Glucans Ingestion Does Not Influence Body Weight: A Systematic Review and Meta-Analysis of Pre-Clinical Studies, Nutrients, 2021, 13(12) DOI:10.3390/nu13124250.
- A. B. C. Samuelsen, J. Schrezenmeir and S. H. Knutsen, Effects of orally administered yeast-derived beta-glucans: A review, Mol. Nutr. Food Res., 2014, 58(1), 183–193, DOI:10.1002/mnfr.201300338.
- J. Lin, M. J. Wester, M. S. Graus, K. A. Lidke and A. K. Neumann, Nanoscopic cell-wall architecture of an immunogenic ligand in Candida albicans during antifungal drug treatment, Mol. Biol. Cell, 2016, 27(6), 1002–1014, DOI:10.1091/mbc.E15-06-0355.
- A. F. Camargo Guarnizo, A. L. Woiciechowski, M. D. Noseda, L. A. Zevallos Torres, A. Zandona Filho, L. Pereira Ramos, L. A. J. Letti and C. R. Soccol, Pentose-rich hydrolysate from oil palm empty fruit bunches for β-glucan production using Pichia jadinii and Cyberlindnera jadinii, Bioresour. Technol., 2021, 320, 124212, DOI:10.1016/j.biortech.2020.124212.
- P. Vaithanomsat, N. Boonlum, C. Trakunjae, W. Apiwatanapiwat, P. Janchai, A. Boondaeng, K. Phalinphattharakit, H. Nimitkeatkai and A. Jarerat, Functionality of Yeast β-Glucan Recovered from Kluyveromyces marxianus by Alkaline and Enzymatic Processes, Polymers, 2022, 14(8) DOI:10.3390/polym14081582.
- A. Olson, Manufacture of bakers yeast by continuous fermentation. Plant and process, Soc. Chem. Ind. Monogr., 1961, 12, 18–93 Search PubMed.
-
G. Reed and T. W. Nagodawithana, Baker's Yeast Production, In Yeast Technology, ed. G. Reed and T. W. Nagodawithana, Springer Netherlands, 1990, pp 261–314 Search PubMed.
-
G. Reed and T. W. Nagodawithana, Use of Yeast in Baking, In Yeast Technology, ed. G. Reed and T. W. Nagodawithana, Springer Netherlands, 1990, pp 315–368 Search PubMed.
- T. Li, X. B. Chen, J. C. Chen, Q. Wu and G. Q. Chen, Open and continuous fermentation: products, conditions and bioprocess economy, Biotechnol. J., 2014, 9(12), 1503–1511, DOI:10.1002/biot.201400084.
- S. F. Siddiqi, M. Bulmer, P. Ayazi Shamlou and N. J. Titchener-Hooker, The effects of fermentation conditions on yeast cell debris particle size distribution during high pressure homogenisation, Bioprocess
Eng., 1995, 14(1), 1–8, DOI:10.1007/BF00369846.
-
H. W. Blanch and D. C. Clark, Biochemical Engineering, CRC Press, 2nd edn, 1996, DOI:10.1201/9780429258732.
- F. Li, T. Long, Y. Lu, Q. Ouyang and C. Tang, The yeast cell-cycle network is robustly designed, Proc. Natl. Acad. Sci. U. S. A., 2004, 101(14), 4781–4786, DOI:10.1073/pnas.0305937101.
- J. Xia, B. J. Sánchez, Y. Chen, K. Campbell, S. Kasvandik and J. Nielsen, Proteome allocations change linearly with the specific growth rate of Saccharomyces cerevisiae under glucose limitation, Nat. Commun., 2022, 13(1), 2819, DOI:10.1038/s41467-022-30513-2.
- E. Metzl-Raz, M. Kafri, G. Yaakov, I. Soifer, Y. Gurvich and N. Barkai, Principles of cellular resource allocation revealed by condition-dependent proteome profiling, Elife, 2017, 6 DOI:10.7554/eLife.28034.
- A. G. Moat, F. Ahmad, J. K. Alexander and I. J. Barnes, Alteration in the Amino Acid Content of Yeast During Growth Under Various Nutritional Conditions, J. Bacteriol., 1969, 98(2), 573–578, DOI:10.1128/jb.98.2.573-578.1969.
- E. Cabib and J. Arroyo, How carbohydrates sculpt cells: chemical control of morphogenesis in the yeast cell wall, Nat. Rev. Microbiol., 2013, 11(9), 648–655, DOI:10.1038/nrmicro3090.
- B. Barazani, S. Warnat, T. Hubbard and A. J. MacIntosh, Mechanical Characterization of Individual Brewing Yeast Cells Using Microelectromechanical Systems (MEMS): Cell Rupture Force and Stiffness, J. Am. Soc. Brew. Chem., 2017, 75(3), 236–243, DOI:10.1094/ASBCJ-2017-3464-01.
- E. M. Spiden, B. H. J. Yap, D. R. A. Hill, S. E. Kentish, P. J. Scales and G. J. O. Martin, Quantitative evaluation of the ease of rupture of industrially promising microalgae by high pressure homogenization, Bioresour. Technol., 2013, 140, 165–171 CrossRef CAS PubMed.
- X. Garcia-Ortega, C. Reyes, J. L. Montesinos and F. Valero, Overall Key Performance Indicator to Optimizing Operation of High-Pressure Homogenizers for a Reliable Quantification of Intracellular Components in Pichia pastoris, Front. Bioeng. Biotechnol., 2015, 3, 107, DOI:10.3389/fbioe.2015.00107.
- L. Drévillon, M. Koubaa and E. Vorobiev, Lipid extraction from Yarrowia lipolytica biomass using high-pressure homogenization, Biomass Bioenergy, 2018, 115, 143–150, DOI:10.1016/j.biombioe.2018.04.014.
- B. H. J. Yap, G. J. Dumsday, P. J. Scales and G. J. O. Martin, Energy evaluation of algal cell disruption by high pressure homogenization, Bioresour. Technol., 2015, 184, 280–285 CrossRef CAS PubMed.
- A. Kolkman, M. M. A. Olsthoorn, C. E. M. Heeremans, A. J. R. Heck and M. Slijper, Comparative Proteome Analysis of <em>Saccharomyces cerevisiae</em> Grown in Chemostat Cultures Limited for Glucose or Ethanol, Mol. Cell. Proteomics, 2005, 4(1), 1–11, DOI:10.1074/mcp.M400087-MCP200.
- J. Yang and S. Tavazoie, Regulatory and evolutionary adaptation of yeast to acute lethal ethanol stress, PLoS One, 2020, 15(11), e0239528, DOI:10.1371/journal.pone.0239528.
- N.-X. Lin, Y. Xu and X.-W. Yu, Overview of yeast environmental stress response pathways and the development of tolerant yeasts, Syst. Microbiol. Biomanuf., 2022, 2(2), 232–245, DOI:10.1007/s43393-021-00058-4.
- A. Szopinska and P. Morsomme, Quantitative Proteomic Approaches and Their Application in the Study of Yeast Stress Responses, OMICS A J. Integr. Biol., 2010, 14(6), 639–649, DOI:10.1089/omi.2010.0045.
- A. P. Gasch, P. T. Spellman, C. M. Kao, O. Carmel-Harel, M. B. Eisen, G. Storz, D. Botstein and P. O. Brown, Genomic expression programs in the response of yeast cells to environmental changes, Mol. Biol. Cell, 2000, 11(12), 4241–4257, DOI:10.1091/mbc.11.12.4241.
- K. Y. F. Lip, E. García-Ríos, C. E. Costa, J. M. Guillamón, L. Domingues, J. Teixeira and W. M. van Gulik, Selection and subsequent physiological characterization of industrial Saccharomyces cerevisiae strains during continuous growth at sub- and- supra optimal temperatures, Biotechnol. Rep., 2020, 26, e00462, DOI:10.1016/j.btre.2020.e00462.
- K. A. Morano, C. M. Grant and W. S. Moye-Rowley, The response to heat shock and oxidative stress in Saccharomyces cerevisiae, Genetics, 2012, 190(4), 1157–1195, DOI:10.1534/genetics.111.128033.
- J. A. Joseph, S. Akkermans and J. F. M. Van Impe, Effects of Temperature and pH on Recombinant Thaumatin II Production by Pichia pastoris, Foods, 2022, 11(10) DOI:10.3390/foods11101438.
- M. Dragosits, J. Stadlmann, J. Albiol, K. Baumann, M. Maurer, B. Gasser, M. Sauer, F. Altmann, P. Ferrer and D. Mattanovich, The Effect of Temperature on the Proteome of Recombinant Pichia pastoris, J. Proteome Res., 2009, 8(3), 1380–1392, DOI:10.1021/pr8007623.
- A. Baez and J. Shiloach, Effect of elevated oxygen concentration on bacteria, yeasts, and cells propagated for production of biological compounds, Microb. Cell Factories, 2014, 13(1), 181, DOI:10.1186/s12934-014-0181-5.
- K. A. Reijenga, B. M. Bakker, C. C. van der Weijden and H. V. Westerhoff, Training of yeast cell dynamics, FEBS J., 2005, 272(7), 1616–1624, DOI:10.1111/j.1742-4658.2005.04582.x.
- P. Gélinas, Search of Perfect Growth Media for Baker's Yeast Production: Mapping Patents, Compr. Rev. Food Sci. Food Saf., 2012, 11(1), 13–33, DOI:10.1111/j.1541-4337.2011.00168.x.
- D. Szwajgier, Content of individual phenolic acids in worts and beers and their possible contribution to the antiradical activity of beer, J. Inst. Brew., 2009, 115(3), 243–252, DOI:10.1002/j.2050-0416.2009.tb00376.x.
- D. De Keukeleire, Fundamentals of beer and hop chemistry, Quím. Nova, 2000, 23 DOI:10.1590/S0100-40422000000100019.
- S. Razmkhab, A. Lopez-Toledano, J. M. Ortega, M. Mayen, J. Merida and M. Medina, Adsorption of Phenolic Compounds and Browning Products in White Wines by Yeasts and Their Cell Walls, J. Agric. Food Chem., 2002, 50(25), 7432–7437, DOI:10.1021/jf025733c.
- J. Ding, X. Huang, L. Zhang, N. Zhao, D. Yang and K. Zhang, Tolerance and stress response to ethanol in the yeast Saccharomyces cerevisiae, Appl. Microbiol. Biotechnol., 2009, 85(2), 253–263, DOI:10.1007/s00253-009-2223-1.
- M. Lairón-Peris, S. J. Routledge, J. A. Linney, J. Alonso-del-Real, C. M. Spickett, A. R. Pitt, J. M. Guillamón, E. Barrio, A. D. Goddard and A. Querol, Lipid Composition Analysis Reveals Mechanisms of Ethanol Tolerance in the Model Yeast Saccharomyces cerevisiae, Appl. Environ. Microbiol., 2021, 87(12), e00440, DOI:10.1128/AEM.00440-21.
- R. A. Ribeiro, M. V. Vitorino, C. P. Godinho, N. Bourbon-Melo, T. T. Robalo, F. Fernandes, M. S. Rodrigues and I. Sá-Correia, Yeast adaptive response to acetic acid stress involves structural alterations and increased stiffness of the cell wall, Sci. Rep., 2021, 11(1), 12652, DOI:10.1038/s41598-021-92069-3.
- E. Cebollero and F. Reggiori, Regulation of autophagy in yeast Saccharomyces cerevisiae, Biochim. Biophys. Acta, 2009, 1793(9), 1413–1421, DOI:10.1016/j.bbamcr.2009.01.008.
- E. Delorme-Axford and D. J. Klionsky, Transcriptional and post-transcriptional regulation of autophagy in the yeast Saccharomyces cerevisiae, J. Biol. Chem., 2018, 293(15), 5396–5403, DOI:10.1074/jbc.R117.804641.
- R. Iwama and Y. Ohsumi, Analysis of autophagy activated during changes in carbon source availability in yeast cells, J. Biol. Chem., 2019, 294(14), 5590–5603, DOI:10.1074/jbc.RA118.005698.
- C. Falcone and C. Mazzoni, External
and internal triggers of cell death in yeast, Cell. Mol. Life Sci., 2016, 73(11–12), 2237–2250, DOI:10.1007/s00018-016-2197-y.
- P. Rockenfeller and F. Madeo, Apoptotic death of ageing yeast, Exp. Gerontol., 2008, 43(10), 876–881, DOI:10.1016/j.exger.2008.08.044.
- E. V. Grosfeld, V. A. Bidiuk, O. V. Mitkevich, E. Ghazy, V. V. Kushnirov and A. I. Alexandrov, A Systematic Survey of Characteristic Features of Yeast Cell Death Triggered by External Factors, J. Fungi, 2021, 7(11) DOI:10.3390/jof7110886.
- G. J. Smits, J. C. Kapteyn, H. van den Ende and F. M. Klis, Cell wall dynamics in yeast, Curr. Opin. Microbiol., 1999, 2(4), 348–352, DOI:10.1016/S1369-5274(99)80061-7.
- L.-C. Lai, A. L. Kosorukoff, P. V. Burke and K. E. Kwast, Dynamical Remodeling of the Transcriptome during Short-Term Anaerobiosis in <i>Saccharomyces cerevisiae: Differential Response and Role of Msn2 and/or Msn4 and Other Factors in Galactose and Glucose Media, Mol. Cell. Biol., 2005, 25(10), 4075–4091, DOI:10.1128/MCB.25.10.4075-4091.2005.
- E. Gnansounou and A. Dauriat, Techno-economic analysis of lignocellulosic ethanol: A review, Bioresour. Technol., 2010, 101(13), 4980–4991, DOI:10.1016/j.biortech.2010.02.009.
- K. K. Kapanji, K. F. Haigh and J. F. Görgens, Techno-economics of lignocellulose biorefineries at South African sugar mills using the biofine process to co-produce levulinic acid, furfural and electricity along with gamma valeractone, Biomass Bioenergy, 2021, 146, 106008, DOI:10.1016/j.biombioe.2021.106008.
- I. Valdez-Vazquez and A. Sanchez, Proposal for biorefineries based on mixed cultures for lignocellulosic biofuel production: a techno-economic analysis, Biofuels, Bioprod. Biorefin., 2018, 12(1), 56–67, DOI:10.1002/bbb.1828.
- N. Bonatsos, C. Marazioti, E. Moutousidi, A. Anagnostou, A. Koutinas and I. K. Kookos, Techno-economic analysis and life cycle assessment of heterotrophic yeast-derived single cell oil production process, Fuel, 2020, 264, 116839, DOI:10.1016/j.fuel.2019.116839.
|
This journal is © The Royal Society of Chemistry 2024 |
Click here to see how this site uses Cookies. View our privacy policy here.