DOI:
10.1039/D4FB00093E
(Review Article)
Sustainable Food Technol., 2024,
2, 1391-1408
Virgin coconut oil: wet production methods and food applications – a review
Received
29th March 2024
, Accepted 5th July 2024
First published on 8th July 2024
Abstract
Globally, the demand for functional foods is increasing because of their positive impact on health. In recent times, Virgin Coconut Oil (VCO) has been gaining prominence as a healthy functional food, owing to its bioactive compounds such as polyphenols and medium-chain fatty acids. As a result, it exhibits potential health benefits such as cardioprotective, neuroprotective, hepatoprotective, antidiabetic, hypolipidemic, and anti-inflammatory effects. These health benefits of VCO enhanced its role mainly in the food, nutraceutical, and pharmaceutical industries. VCO is obtained from fresh coconut kernels without any heat, shear, or the addition of chemicals. In this review, different methods such as centrifugation, fermentation, freezing–thawing followed by centrifugation, and enzymatic treatment followed by centrifugation for the production of VCO are discussed. This review also discusses the application of recent novel methods such as supercritical fluid extraction, ultrasonication, mega-sonication, microwave treatment, etc., for the production of VCO. The development of value-added convenience food products such as oleogels, emulsions, and encapsulated powders incorporating VCO is discussed. The present review also discusses the use of VCO as a natural solvent for extracting bioactive compounds, such as polyphenols, pigments, etc., from plants and other natural sources.
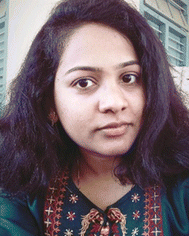 N. Sai Prasanna | Sai Prasanna N received her B. Tech degree in Agricultural Engineering (2017) from Acharya N G Ranga Agricultural University (Andhra Pradesh), India, and her M. Tech degree in Food Process Engineering (2019) from IIT Kharagpur, India. Currently, she is pursuing her PhD under the joint supervision of Prof. KSMS Raghavarao and Dr Nilesh Choudhary at the Chemical Engineering Department, Indian Institute of Technology Tirupati, India. She received the most prestigious Prime Minister's Fellowship (sponsored by SERB-CII, India) in 2023. Her research is focused on nanotechnology and membrane processing for food applications, post-harvest engineering, and extraction of bioactive compounds from bio-waste. |
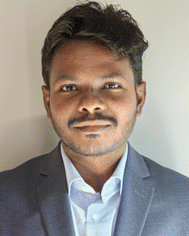 Murugesan Selvakumar | Murugesan Selvakumar received his B. Tech degree in Biotechnology (2017) from the Kamaraj College of Engineering and Technology, Tamil Nadu, India, and his M. Tech degree in Biotechnology (2019) from the University College of Engineering Tiruchirappalli, Anna University, Tamil Nadu, India. Currently, he is pursuing his PhD under the joint supervision of Prof. KSMS Raghavarao and Dr Trivikram Reddy Nallamilli at the Chemical Engineering Department, Indian Institute of Technology Tirupati, India. He received the most prestigious Prime Minister's Fellowship (sponsored by SERB-CII, India) in 2022. His research is mainly focused on soft matter applications in foods such as emulsions, encapsulation, oleogels, and hydrogels. |
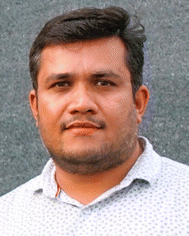 Nilesh Choudhary | Dr Nilesh Choudhary received his B. Tech in Chemical Engineering from UECU/RGPV and his PhD from AcSIR/CSIR-NCL, India, and completed his postdoctoral studies at KAUST KSA. Currently, Dr Nilesh is working as an Assistant Professor at the Department of Chemical Engineering, Indian Institute of Technology Tirupati, India. He does solution thermodynamic process modelling and molecular simulations for separation, purification, and membrane processes. He is developing the core area in supercritical fluid and pressure-assisted extraction processes. His areas of interest are liquid–liquid, solid–liquid, and soil–liquid–vapor phase equilibrium associated with separation and membrane processing. Nilesh also worked on molecular simulation and thermodynamics modelling for gas hydrate-based purification and separation processes and CO2/chemical flooding-based enhanced oil recovery. |
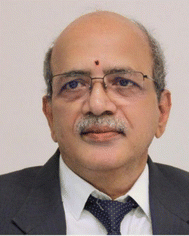 K. S. M. S. Raghavarao | Prof. Raghavarao is a Chemical Engineer (B. Tech, from Andhra University and PhD from ICT, Mumbai). After PDF at NIST-Colorado, he joined CFTRI and retired as the Director of CSIR-CFTRI. Currently, he is working at IIT Tirupati. He has guided 25 and is currently guiding 8 PhD students at IIT Tirupati. He is the recipient of the prestigious Lifetime Achievement Award of the IAEF (Nantes, France), NASI-Reliance, and VASVIK awards for applied research, besides the National Fellowships of Academies (FNAE, FASc, and FNAAS). He has over 160 publications (with an ‘h’ index of 60) and citations over 12000, about 28 international patents and 55 Indian patents. |
Sustainability spotlight
Building sustainable food production and human diets is an urgent matter after the COVID-19 pandemic outbreak. Coconut (Cocos nucifera L.) is the most widely spread plantation crop, which provides numerous health benefits. Among the various value-added products obtained from coconut, Virgin Coconut Oil (VCO) is a unique product gaining more prominence in the food, nutraceutical, and pharmaceutical industries due to its several health benefits. This manuscript emphasizes wet processing (both conventional and novel) methods of VCO production and highlights the potential health benefits of VCO. Through this review, we contribute valuable insights into economically viable, natural, eco-friendly, and sustainable technologies for producing VCO at the industrial level. Owing to consumers' awareness of natural, safe, and healthy products, the development of various VCO-based food formulations is also explored in the present review for their use in sustainable food applications.
|
1. Introduction
Coconut (Cocos nucifera L.) belongs to the Arecaceae (palm) family and is widespread in some tropical and subtropical countries. Coconut trees are widely cultivated in the southern regions of India. The coconut tree is respected as the “tree of life” (Kalpa vriksha), since all parts of it have economic value and nutritional benefits.1,2 In 2022, the global production of coconuts was recorded to be 62.41 million tonnes.3 The coconut fruit mainly consists of kernel (51.7% w/w), water (9.8% w/w), and shell (38.5% w/w).4 Edible parts of the coconut fruit contain essential nutrients, minerals (iron, calcium, magnesium, phosphorus, and selenium) and vitamins (C, E, B1, B3, B5, and B6),5 which provide a boost to the market for value-added products from coconut, thereby increasing the income of farmers. Value-added products from coconut include virgin coconut oil, coconut gratings, coconut milk, coconut water, desiccated coconut, and coconut whey. These products possess good nutritional, medicinal, and biological properties, thus finding applications in the nutraceutical, cosmetic, and food industries.4
Coconut oil is popular all over the world due to its nutritional and health benefits. Coconut oil is used for cooking purposes, as a skin moisturizer, and in infant foods. Commercially available coconut oils are obtained from dried coconut kernels (copra) through methods such as mechanical expelling and solvent extraction. The obtained coconut oil is subjected to refining (chemical or physical) processes. In the refining process, coconut oil is treated with a mixture of citric and phosphoric acids or aqueous phosphoric acids (0.05–0.1%) and heated to remove phospholipids.6 The obtained oil is then bleached with adsorbents such as bleaching earth, activated carbon, etc., and finally deacidified/steam deodorized. Due to the high temperatures (about 204–245 °C) involved in the deodorization step, the bioactive components such as polyphenols and tocopherols are lost in refined coconut oil (RCO).7 Because of these refining processes, RCO loses the typical pleasant coconut odour and taste.6,7 On refining, RCO will have higher levels of saturated fats (∼90%) and low levels of unsaturated fats (mainly oleic acid (6%) and linoleic acid (2%)).1 RCO is used as a natural skin moisturizer,8 and its high smoke point makes it a suitable medium for cooking methods such as seasoning, shallow frying and deep-frying of food products.9,10
Nowadays, virgin coconut oil (VCO) is gaining more prominence in the food, nutraceutical, and pharmaceutical industries due to its several health benefits, as discussed in Section 2. The production methods of VCO are classified into wet and dry methods. In the dry method, fresh coconut kernel is grated and quickly dehydrated at 40–50 °C to reduce the moisture content to 2–5% (wet basis), followed by pressing or expelling to produce VCO.1,7 VCO obtained through this method has a low moisture content, thus arresting microbial contamination. Though higher oil yields (∼46–49% w/w) are obtained in the dry method,7 the bioactive compounds in VCO are diminished as the temperature exceeds 40 °C during drying or expelling operation, in turn reducing its oxidative stability.11 In the wet method, coconut milk is expelled from fresh coconut kernels and demulsified to obtain VCO.12,13 The wet methods for the production of VCO are shown in Fig. 1. As the wet methods operate at ambient temperature, the loss of bioactive components such as polyphenols, tocotrienols, and tocopherols is minimal.14 The colourless nature, original coconut flavour and taste, and excellent nutritional value of VCO produced through wet processing methods enhanced its applications in salad dressings, bakery items, confectionery products, infant foods, and skin and hair care products, as well as a vehicle for drug delivery. Although the fatty acid profiles of VCO and RCO are very similar, VCO is considered a healthy functional food oil owing to its medium chain fatty acids (MCFAs) and health benefits. The global demand for VCO is expected to grow ∼2.5% over the next five years.15 However, VCO production at an industrial level faces challenges, particularly in terms of yield and retention of bioactive compounds. In this review, more focus was given to the VCO produced by wet processing methods. Different forms of VCO, such as oleo-gels, emulsions, encapsulated powders, etc., for convenience and functional food applications are also discussed.
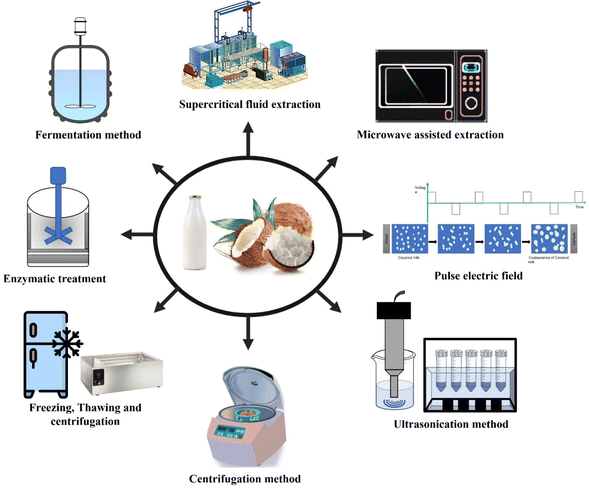 |
| Fig. 1 Different wet methods for VCO production. | |
2. Health benefits of VCO
VCO is valued as a functional food due to its rich MCFAs, mainly lauric acid (C12:0, ∼48–53%),16 caprylic acid (C8:0, 5.22%),17 and capric acid (C10:0, 5.41%),17 and these MCFAs are easily digestible.18 VCO has a significant fraction of phytochemicals such as polyphenols, tocopherols, tocotrienols, flavonoids, phytosterols, and vitamin A.19–21 Lauric acid in VCO is converted to monolaurin during digestion, which provides immunity to the body and keeps humans (even infants) away from getting viral or bacterial infections.22,23 VCO intake improved the metabolic function in stunted children (under 5 years of age) and also optimized their growth and development, thereby contributing to the health of those children.24 Due to the presence of MCFAs and phenolic compounds, VCO combats oxidative stress and inflammation.25–27 VCO consumption by adults with metabolic syndrome was reported to improve neurodegenerative disorders, especially Alzheimer's and Parkinson's Disease.25,26,28 VCO consumption was reported to increase high-density lipoprotein and lower low-density lipoprotein significantly in people with diabetes mellitus and dyslipidaemia, thus indicating its antidiabetic potential.29,30 VCO offers other health benefits such as cardioprotective,31,32 hypolipidemic, hepatoprotective,29 anti-viral and immunomodulatory properties,19etc. Current research is mainly focused on animal studies, and human clinical studies on VCO, as a supplement, are being carried out to identify those components responsible for the health benefits discussed above.
3. Wet production methods
VCO is produced from the kernels of fresh and matured coconuts without the use of any heat, shear, or chemicals.13,18 Coconuts with a maturity level of 10–12 months are de-husked, deshelled, pared (removal of brown testa), washed, and grated. Coconut milk is expelled from the coconut gratings using a screw press and demulsified to produce VCO.12 The flowchart of sequential processes involved in the wet processing of coconut for VCO production is shown in Fig. 2.
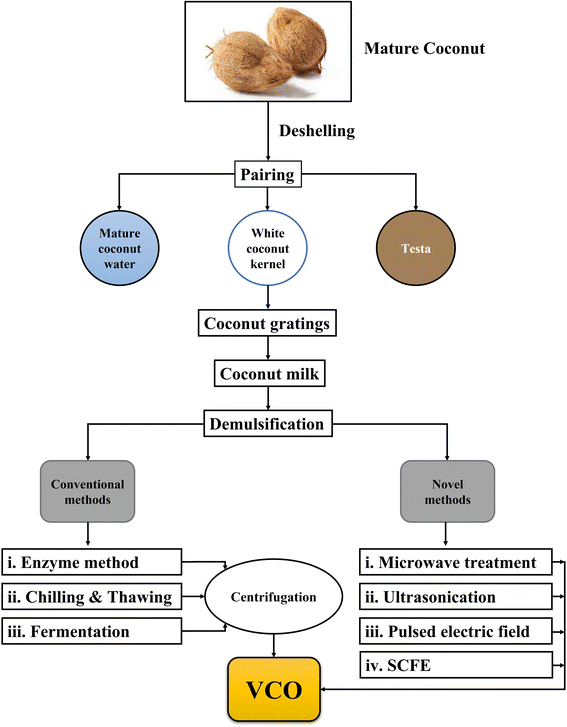 |
| Fig. 2 Flowchart for wet processing of virgin coconut oil. | |
Coconut milk is an O/W emulsion in which small spherical droplets of oil are dispersed in a continuous (aqueous) phase. It contains ∼55 wt% water,33 35 wt% fat,34 3.8 wt% protein,34 and small contents of carbohydrates and ash. It is stabilized due to naturally occurring proteins (such as globulins and albumins) and phospholipids. These proteins in coconut milk are adsorbed at the interface between the oil and water, minimizing its interfacial tension. The stability of coconut milk emulsion depends on the quality and quantity of proteins. At the initial stage, coconut milk appears homogeneous and stable. However, after a few hours, coconut milk becomes unstable and separates into a creamy oil phase and an aqueous phase.35 Generally, O/W emulsions are thermodynamically unstable because of unfavourable contact between dispersed and continuous phases.36 Thus, the physical structure of coconut milk emulsions will change over time through various demulsification mechanisms (creaming, flocculation, and coalescence), resulting in complete phase separation, as shown in Fig. 3. These demulsification mechanisms are described briefly below.
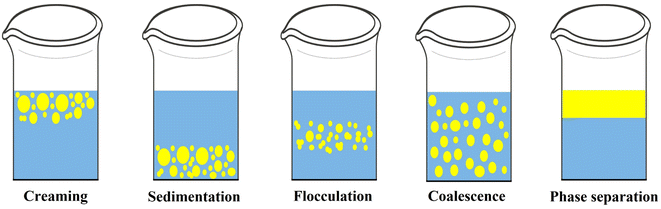 |
| Fig. 3 Demulsification mechanisms. | |
(a) Creaming and sedimentation occur due to buoyancy and gravitational force, which break the emulsion into two phases. Since the oil droplets have a lower density than the continuous phase, they move to the top surface of the emulsion, forming a creamy oil phase (creaming). While the continuous phase (water) settles at the bottom, resulting in sedimentation.37
(b) When two or more oil droplets come closer to each other, they form an aggregate. This is called flocculation.37 Factors such as the water fraction in emulsions, density and viscosity of the oil phase, temperature, and electrostatic field influence the flocculation mechanism.38
(c) When two or more dispersed oil droplets combine, they form a larger droplet. This is called the coalescence mechanism, which promotes the destabilization of an emulsion into two phases. Factors such as the interfacial velocity, interfacial tension, flocculation rate, and temperature can influence this mechanism.38
The conventional wet processing and novel processing methods for VCO production are discussed in this section.
3.1. Centrifugation
By centrifugation of coconut milk, it is possible to break emulsions into two phases, i.e., heavy (aqueous) phase and lighter (oil) phase.39,40 Centrifugation can promote demulsification mechanisms such as creaming and coalescence. The process parameters involved in this method and the oil yields are shown in Table 1. The yields of VCO obtained from coconut milk were reported as 29.5%39 and 13.53%.41 In this method, the yield of VCO depends on the centrifugation speed (rpm), time, and temperature. As the centrifugation time increases, the time for the separation of oil droplets from coconut milk will be longer, thus resulting in higher oil yields. The oil yields also increased with the centrifugal speed.39 The coconut milk may solidify at temperatures below 5 °C.42 Centrifugation temperatures above 50 °C cause the denaturation of proteins in coconut milk, which affects their applications when recovered. Therefore, temperatures in the range of 25–40 °C are suitable to produce VCO through the centrifugation method.41 Though the equipment involved is simple, low yield and high operation time are the major drawbacks of this method.
Table 1 Summary of oil yield obtained through various methods for VCO production
Method |
Coconut milk |
Process conditions |
Yield (%) |
Reference |
Centrifugation |
Coconut milk |
6000–12 000 rpm, 105 min, 30 °C |
15.58–29.5 |
39
|
Centrifugation |
Coconut milk |
12 000 rpm, 15–120 min, 40 °C |
9.27–13.53 |
41
|
Centrifugation |
Grated coconut and water (1 : 1) |
4000 rpm, 30 min, room temperature |
54.4 ± 1.1 |
40
|
Chilling–thawing and centrifugation |
Coconut milk : water (1 : 1) |
3600 gyr for 10 min, 0 °C for 24 h, 50 °C |
86.62 ± 3.63 |
43
|
Chilling–thawing and centrifugation |
Coconut milk |
5 °C for 6 h, 29 ± 2 °C, 3585 gyr for 10 min, followed by 4880 gyr for 15 min |
92 |
12
|
Chilling–thawing and centrifugation |
Coconut milk |
4 °C for 4 h, ambient temperature for 2 h, 8000 gyr for 15 min |
20.54 ± 0.47 |
44
|
Chilling, thawing and centrifugation |
Grated coconut and water (1 : 1) |
0 °C for 6 h, room temperature |
69.37 |
40
|
Chilling–thawing and centrifugation |
Coconut grating : water (1 : 2) |
4 °C; 50 °C; 6000 rpm for 45 min |
20.44 |
18
|
Chilling–thawing and centrifugation |
Coconut milk : water (1 : 1) |
5 °C for 24 h; 50 °C; 3600 gyr for 10 min |
70 ± 0.80 |
45
|
Chilling–thawing and centrifugation |
Coconut milk |
4 °C for 24 h; 50 °C; 5000 rpm for 10 min |
38.8 |
46
|
Centrifugation followed by freezing–thawing and centrifugation |
Grated coconut : water (2 : 1) |
4 °C for 4 h; 50 °C until coconut cream reached room temperature; 6000 rpm for 15 min |
86.28 ± 0.023 |
35
|
Fermentation |
Coconut milk : water (1 : 1) |
Saccharomyces cerevisiae (2% W/V), 36 h at room temperature |
na |
43
|
Fermentation |
Grated coconut : water (1 : 1) |
Lactic acid bacteria, yeast and mold, incubated at 70–80 °C for 16–24 h |
20 |
47
|
Fermentation |
Coconut milk |
Lactic acid bacteria, incubated at 35–40 °C for 20–24 h |
na |
48
|
Fermentation |
Coconut milk : water (1 : 1) |
S. cerevisiae (0.3% (w/v), incubated at room temperature for 24 h |
92.73 |
49
|
Fermentation |
Coconut grating : water (1 : 2) |
Incubated for 72 h, 6000 rpm for 45 min |
9.43 |
18
|
Fermentation |
Grated coconut : water (1 : 1) |
Lactic acid bacteria (2.5%, 5%, 10%, 20%, and 50% inoculation ratios), 37 °C for 48 h |
25 |
50
|
Fermentation method |
Coconut milk : water (1 : 1) |
Baker's yeast (Saccharomyces cerevisiae) incubated for 36 h |
72 ± 2.00 |
45
|
Fermentation and enzymatic treatment |
Grated coconut |
Crude papain from papaya latex (1 g) fermented at 55 °C, incubated at 80 °C for 15 min |
27.70 |
11
|
Fermentation |
Grated coconut : water (1 : 1) |
Tempeh yeast (2%), incubated at 37 °C for 24 h |
33.25 |
51
|
Bread yeast (2%), incubated at 37 °C for 24 h |
28.88 |
Tape yeast (2%) incubated at 37 °C for 24 h |
24.41 |
Enzyme treatment |
Coconut milk |
0.1% of aspartic protease (2500 tyrosine units per g) incubated at 25 °C for 3 h |
76 |
12
|
Enzyme treatment |
Coconut milk |
0.1% of aspartic protease (2500 tyrosine units per g) incubated at 37 °C for 3 h |
83 |
12
|
Combined enzymatic treatment and chilling, thawing and centrifugation |
Coconut milk |
0.1% of aspartic protease (2500 tyrosine units per g) incubated at 37 °C for 3 h; 5 °C for 6 h; 29 ± 2 °C, 4880 g for 15 min |
94.50 |
12
|
Enzymatic treatment and centrifugation |
Coconut milk : water (1 : 1) |
Papain enzyme (0.1% (w/w); for 3 h at 55 °C, 4900 g for 25 min |
60.09 |
43
|
Enzymatic treatment and centrifugation |
Coconut milk |
Crude protease extract ((10 U g−1 protein), incubated at 28–30 °C for 6 h, 4900 g for 30 min, 4900 g at room temperature for 30 min |
92.39 ± 2.11 |
52
|
Enzymatic treatment |
Grated coconut : water (1 : 1) |
Crude papain enzyme (0.5% (w/v), incubated at 60 °C for 24 h |
24 – 25 |
53
|
Enzymatic treatment and centrifugation method followed by freeze–thawing and centrifugation |
Coconut gratings : water (1 : 1) |
• Papain (0.05%) enzyme, incubated for 2 h, 3585 g for 30 min |
72.36 ± 3.0 |
54
|
• Cellulase (0.05%) enzyme incubated for 2 h |
• Freezing (5 h) and thawing and 4880 g for 30 min |
Enzymatic treatment and centrifugation |
Coconut milk |
Papain enzyme (0.1% (w/w)), incubated for 3 h at 55 °C, 4900 rpm for 25 min |
49.60 |
46
|
Chilling followed by mega-sonic treatment and centrifugation |
Coconut pulp : water (1 : 4) |
Bath frequency (2 MHz), distance between the transducer and vessel wall (3 cm), energy density (32.5 kJ kg−1) and time (5 min) |
2.7 |
55
|
Ultrasonic treatment followed by centrifugation, freezing–thawing and centrifugation |
Grated coconut : water (2 : 1) |
Horn-type probe, diameter (6 mm), frequency (20–25 kHz), power (350 W), temperature (55 °C) and time (10 min with 30 s on–off) |
93.0 |
35
|
Microwave assisted extraction treatment followed by centrifugation |
Grated coconut : water (1 : 5) |
Power (1000 W), frequency (2.5 GHz), time (1 min), 4.31 kW kg−1, 45 °C |
20 |
56
|
Microwave treatment followed centrifugation, freezing–thawing and centrifugation |
Grated coconut : water (2 : 1) |
Power (450 W), frequency (2450 MHz), and time (2 min) |
91.53 ± 0.139 |
35
|
Pulsed electric field followed by centrifugation, freezing–thawing and centrifugation |
Grated coconut : water (2 : 1) |
Voltage (40 kV cm−1), distance between the two electrodes (1.8 cm) and time (12.32 min) |
93.41 ± 0.002 |
35
|
Ultrasound assisted extraction followed by centrifugation |
Grated coconut : water (1 : 5) |
Horn-type probe, diameter (40 mm), power (200 W), time (2.5 min), frequency (24 kHz), and temperature (45 °C) |
10–16 |
56
|
Ultrasound assisted enzyme extraction followed by centrifugation |
Coconut milk |
Soursop enzyme, ultrasound frequency (42 kHz), extraction temperature (30 °C) and time (60 min) |
66.7 |
57
|
Supercritical fluid extraction |
Fresh coconut |
CO2 flow rate (10 g min−1), temperature (60 °C), time (60 min) and pressure (300 bar) |
28.84 |
58
|
Freezing and thawing followed by microwave treatment |
Coconut milk |
Freezing (−17 °C for 24 h), thawing (room temperature) |
23.83 ± 0.76 |
59
|
Power (500 W), time (15.33 ± 0.76 min) |
Microwave treatment followed by centrifugation |
Coconut milk |
Power (720 W), 12 000 rpm for 105 min |
46.88 |
60
|
3.2. Chilling–thawing and centrifugation
The temperatures used for chilling were in the range of 4 to 10 °C and −4 °C for freezing.7 In this method, coconut milk is chilled in a refrigerator and thawed at 40–50 °C in a water bath until it reaches ambient temperature, and later centrifuged to obtain clear VCO.12,13,35,44 In chilling/freezing, the oil droplets in coconut milk are separated due to the difference in the freezing points of the components present in it. This method promotes the coalescence of oil droplets in coconut milk, resulting in its demulsification.12 VCO yields reported by various researchers are shown in Table 1. Raghavendra and Raghavarao (2010) reported an oil yield of 92% (w/w),12 which is higher than that of centrifugation alone. VCO obtained through this method has good antioxidant potential with acceptable sensory quality. However, freezing and thawing steps have fixed and operating costs, which significantly increase on scaleup.61
3.3. Fermentation
The fermentation of coconut milk uses the acid-producing metabolism of its endogenous microorganisms, which disrupts the oil–water interface of coconut milk emulsion,11,48,62 facilitating the separation of VCO from aqueous whey.21 Microorganisms such as lactic acid bacteria (Lactobacillus delbrueckii, L. casei, L. fermentum and L. plantarum) at different inoculum levels are widely used for the fermentation of coconut milk.47,48,50 Nguyen-Son et al.50 fermented coconut milk using lactic acid bacteria at 37 °C for 48 h and reported an oil yield of 25% (v/v). Some yeasts (such as tape yeast, tempeh yeast, and baker's yeast) are also used in the fermentation of coconut milk to produce VCO.43,45,49,51 The oil yields reported by various researchers are shown in Table 1. VCO produced by this method has desirable characteristics such as a distinct coconut aroma, low rancidity, and longer shelf life.21,61 However, lactic acid bacteria used in this process release lactic acid, affecting the sensory qualities (like sour aroma, acidity, and rancidity) of the VCO produced.15,21 High levels of the inoculum of microorganisms used in this process release lipase, which hydrolyses VCO to form free fatty acids (FFAs). Also, the presence of excess moisture augments the formation of FFAs in VCO. These FFAs are partially degraded to form methyl ketones, thereby resulting in an undesirable rancid odour of VCO.15 Villarino et al.63 reported that the VCO samples produced from the fermentation process showed a distinctly more rancid odour than that from the centrifugation process (followed by vacuum evaporation). Since this method involves the least financial investment, less energy input and labour, it is suitable for microenterprises. However, this method produces VCO of low quality (with a yellow tinge and fermentation odor), besides being a time-consuming process.11,16,21,61
3.4. Enzymatic treatment and centrifugation method
In this method, enzymes are added to coconut milk and incubated under optimum conditions of time and temperature, followed by centrifugation to obtain VCO.13,46,52,54 The protease enzyme used in this method breaks the peptide bonds of the proteins present in coconut milk, forming shorter fragments of peptides, which, in turn, decrease their emulsifying properties, leading to the separation of coconut milk into two phases (VCO and coconut whey).13 The rate of the enzyme reaction is regulated by the enzyme concentration and incubation time and temperature. An increase in enzyme concentration causes a change in the oil colour, from colourless to a yellowish tinge. Excessive incubation temperature and time accelerate the oxidation and hydrolysis reactions, which in turn lower the oil quality. On the other hand, low temperatures cause ineffective functioning of enzymes, resulting in low oil yields.53 Accordingly, incubation temperature and time and appropriate enzyme concentration are found to be crucial parameters for the enzymatic production of VCO.
Coconut milk treated with aspartic protease (0.1%) and incubated at 37 °C for 3 h resulted in oil yields of 83% (w/w)13 and 92% (w/w).52 The oil yields reported by various researchers are shown in Table 1. Recently, crude enzymes (papain, protease, and bromelain) isolated from fruit wastes, such as papaya peels, pineapple peels, unripe papaya latex, and alginate-immobilized bromelain enzymes, have also shown considerable potential to produce VCO from coconut milk.11,64–66 Since severe heating is not involved in this method, damage to bioactive components is minimal. VCO produced by this method showed a high retention of phenolic compounds, which provides protection against rancidity.13,67 The high cost and unavailability of specific enzymes are the major disadvantages of this method.67
3.5. Novel processing technologies
Novel technologies such as ultrasonication, microwave treatment, and supercritical fluid extraction have gained wide attention for various food processing applications. These processes are being explored for the production of vegetable oils and fats from the seeds of various plant crops, such as canola, pumpkin, papaya, raspberry, sunflower, safflower, etc. The application of recent and advanced technologies to produce VCO from fresh coconut is discussed in the following section.
3.5.1. Supercritical fluid extraction.
Supercritical fluid extraction (SCFE) is successively applied to produce natural food ingredients and plays a pivotal role in the agricultural, pharmaceutical, and cosmetic industries.68 In this method, supercritical fluids are used as solvents to extract valuable compounds from solid food materials. These supercritical fluids have both liquid-like and gas-like behaviour above critical pressure and temperature.69 This method is considered to be green and environmentally friendly as it does not involve organic solvents. This method operates at high pressures and temperatures, depending on the critical point of the supercritical fluids used.68
Supercritical carbon dioxide (SC-CO2) is a widely used solvent due to its advantages, such as low cost, easy availability, non-toxic nature, low viscosity, high selectivity, high diffusivity, recyclability, etc.58,70 SC-CO2 is considered as a food-grade solvent and labeled as Generally Recognized as Safe (GRAS).71 SC-CO2 has a critical pressure of 7.38 MPa and a critical temperature of 31.1 °C, making it an ideal choice for thermally labile food materials.72 By reducing the pressure after the completion of SCFE, SC-CO2 is easily separated from extracted materials and recycled. Zuknik et al.72 investigated the solubility of VCO in SC-CO2 over a range of temperatures (40 to 80 °C) and pressures (20.7 to 34.5 MPa). He reported that the solubility of VCO increased with an increase in temperature over the pressure range between 31 and 34.5 MPa, while the solubility of VCO decreased with an increase in temperature in the pressure range between 20.7 and 24.1 MPa. Aytac et al.58 employed SCFE to extract VCO from fresh coconut, and the process conditions are mentioned in Table 1. The obtained VCO had a lauric acid content as high as 53.19% of the total fatty acid content.58 Since the extraction temperature involved here is above 40 °C, there might be chances of degradation of bioactive compounds present in VCO, which need to be investigated. The oil yield from this method was reported to be low (28.84% w/w).58
3.5.2. Ultrasonication.
In recent years, low-frequency (20–100 kHz) and high-frequency (1–10 MHz) ultrasonication processes have been used for food applications. Ultrasound (US) works on the principle of the cavitation effect or oscillation/phenomenon. VCO is produced from a homogenized fresh coconut–water mixture by ultrasonication, and the oil yield through this method was reported to be 10–16% (w/w).56 US-assisted enzymatic extraction followed by centrifugation was employed to produce VCO from coconut milk and the oil yield was reported to be 66.67% (w/w).57 The process conditions and oil yields obtained by other researchers are mentioned in Table 1. The cavitation effect in ultrasound treatment forms microbubbles in the coconut milk, and sudden bursts of these bubbles break down lipoprotein bonds, thereby easily separating the oil phase. The major parameters involved in this process are the power and frequency of ultrasound, temperature, time of sonication, and solvent-liquid ratio.69 This method uses a simple experimental setup and requires a smaller amount of solvent, but involves a temperature rise during the process.35,73,74
3.5.3. Mega-sonication.
Currently, ultrasound treatment at high frequency (0.4–4 MHz) is termed mega-sonic treatment in food processing. This technique is being used for various food processing operations, such as defoaming, emulsification, homogenization, non-solvent separation in oil extraction processes, etc.75 Mega-sonic separation is being explored as an alternative to existing separation techniques (such as gravity separation, centrifugation, and filtration) and can be used in food industries to enhance process efficiencies.76 This method has been employed for the destabilization of emulsions like natural raw milk, which thus separates into two phases (fat and whey).77 In this method, acoustic radiation (primary) forces move continuous and dispersed phases in aqueous liquid suspensions/emulsions towards pressure nodes and anti-nodes of standing waves, respectively, thus resulting in two separate phases (dispersed and continuous). Fat/oil globules in natural emulsions, like milk, are moved towards pressure anti-nodes, and at these nodal planes, Bjerknes (secondary acoustic) forces act on the fat globules, promoting their rapid coalescence into larger aggregates.78,79 The mega-sonication promotes demulsification mechanisms such as creaming, flotation or sedimentation, and coalescence of the dispersed phase in aqueous liquid suspensions/emulsions.55,78–80 The separation of oil droplets through mega-sonic waves depends on the mega-sonic frequency and power, temperature, the time of sonication, the distance between transducer plates, and the solid–liquid ratio.77,81 The micro-streaming effects (caused by stable cavitation bubbles) of mega-sonication facilitated the wall breakage of oil cells, thus enhancing the oil yields. This method has been successfully applied in aqueous-assisted oil extraction from olives, palm nuts, and avocado fruits.82 Li et al.55 studied the effect of mega-sonication (2 MHz and 32.5 kJ kg−1 for 5 min) on chilled (5 °C for 20 h) aqueous mixtures (of coconut pulp), followed by centrifugation to obtain VCO. They reported that VCO recovery was enhanced by 2.7% compared with heating (60 °C for 30 min) of coconut pulp–water mixtures. The impact of this method on oil quality, retention of bioactive compounds, and nutrition needs to be investigated for the large-scale production of VCO.
3.5.4. Microwave treatments.
Microwave irradiation has gained attention both in industry and academia due to its efficiency and potentiality for clean food processing.83 In this method, demulsification occurs due to a combination of thermal and non-thermal effects. The polar water molecules twist (due to dipole polarization) at a high speed under a high-frequency electromagnetic field, which in turn reduces the stability/zeta potential of the oil–water interface film. This results in emulsified droplets being more likely to collide and coalesce, thus leading to the separation of the O/W emulsion into two phases (oil and water).84
Microwave (MW) irradiation is chosen for the demulsification of coconut milk and has been shown to improve VCO yield compared to conventional methods (heating and centrifugation). MW irradiation breaks the hydrogen bonds between water and protein molecules, thus reducing their emulsifying properties.35,60 The process conditions of MW treatment and respective VCO yields are mentioned in Table 1. Martinez-Padilla et al.56 extracted VCO from coconut endosperm–water mixtures using a microwave technique followed by centrifugation and the oil yield is reported as 20% (w/w). The physicochemical properties of VCO obtained through microwave treatment showed no significant difference when compared with commercial VCO.59 The temperature rise caused by MW treatment can degrade bioactive compounds such as phenolic compounds present in VCO. This method takes a shorter time than conventional methods. However, the temperature rise can influence the oxidative stability of VCO and can also cause protein denaturation in coconut milk, hindering its fractionation.59 Therefore, optimizing the temperature and time of microwave irradiation is very crucial for obtaining high-quality VCO. To date, VCO recovery has been studied using this method at a laboratory scale. Further investigation is required for industrial scale production.
3.5.5. Pulsed-electric field method.
A pulsed-electric field (PEF) uses electric waves for a short time (from microseconds to milli-seconds) that are applied to the liquid food product, which is kept in between two electrodes in the chamber. An electric field strength in the range of 0.5–3 kV cm−1 causes irreversible permeabilization of plant and animal tissues.85 The application of low-voltage PEF induces the aggregation of oil droplets in O/W emulsions. A sudden decrease in the applied frequency induces the coalescence of aggregated droplets, leading to demulsification and separation of the emulsion into two phases.86 Dewi et al.87 attempted to demulsify coconut milk using PEF (3.277 kV cm−1 and a frequency of 2.56 kHz) and produced VCO with a total yield of 17.57% (w/w). Negi et al.35 employed PEF followed by freezing–thawing and centrifugation to produce VCO, and the process conditions are mentioned in Table 1. In this study, the VCO yield reported was 93.41 ± 0.002% (w/w).35 This method of demulsification uses a simple experimental setup and requires no harsh chemicals. However, PEF application causes the unavoidable occurrence of electrochemical reactions at the electrode–food interface.88,89 The impact of this method on the sensory quality and bioactive compounds of VCO needs to be investigated in the future.
4. Physico-chemical properties affecting VCO quality
The quality of VCO depends on factors such as the maturity levels of fresh coconut, processing protocol, packaging, and storage conditions. The ever-increasing demand for VCO highlights the need for maintaining quality standards including its sensory attributes and shelf-life. The quality standards of VCO for human consumption are standardized by the Asian Pacific Coconut Community (APCC), Jakarta. The quality and shelf-life of VCO are assessed through several physicochemical properties, such as moisture, volatile matter, peroxide value, iodine value, saponification value, refractive index, insoluble impurities, and free fatty acids. In general, the sensory quality (such as colour, aroma, and taste) of oil deteriorates due to rancidity or lipid oxidation, microbial action, and improper storage conditions.90 Rancidity is the result of complex free radical reactions of free fatty acids with oxygen, resulting in the oxidative degradation of lipids (fats and oils). Rancidity is responsible for the off-flavour, off-odours, loss of colour and texture, and overall quality of VCO during storage, further limiting its shelf life.41
In oils, free fatty acids (FFAs) are inherently present at low concentrations, which increase during storage over a long period, eventually resulting in rancidity. The residual moisture levels in VCO increase the FFA content. High levels of FFAs, together with moisture content, reduce the oil stability, leading to the formation of rancidity/off-flavours.39 Since reducing FFA levels is often difficult, removing residual moisture can lower FFA amounts in VCO.87 Lipoxygenase and lipase are the most common enzymes in coconut kernels. These lipase enzymes in coconut milk hydrolyze the ester bonds of triacyl glycerol and increase FFAs,91 in turn, resulting in the rancidity of VCO. The presence of residual moisture also elevates lipase activity, affecting the sensory quality of VCO during the storage period.21 Hence, lipase activity should be effectively arrested in order to control rancidity. Thus, the lipase activity, FFAs, and residual moisture are the critical factors that determine the quality and shelf-life of VCO.
The APCC recommendation for the moisture level in VCO is ≤0.3% w/w. Adsorbents, such as activated charcoal, zeolites, rice husk, bentonite, etc., are used for the removal of moisture from VCO. These adsorbents are found to significantly reduce the FFA content and peroxide values, besides moisture. After adsorption, VCO is reported to meet the quality standards as per the APCC.92–96 However, the adsorption process needs to be investigated further for upscaling and possible adoption by VCO processing industries.
The quality of VCO produced by wet methods (discussed in Section 3) depends on the presence of bioactive compounds, such as phenolic compounds and tocopherols. These compounds act as natural antioxidants, thereby improving the oxidative stability of VCO. Phenolic compounds extracted from beetroot, cinnamon, ginger, and strawberries are added to VCO at a ratio of 1
:
1
:
1
:
1 and the 1500 ppm level.97 The mixture of phenolics exhibited antioxidant potential and retarded lipid oxidation even at very low concentrations.98 VCO is added to an oleoresin mixture (ginger
:
garlic
:
cinnamon
:
nutmeg
:
cloves
:
black pepper) in a ratio of 1
:
1
:
1
:
1
:
1
:
1.97 These antioxidants are found to reduce the rancidity of this spice-flavoured VCO by neutralizing the free radicals. Spice-flavoured VCO is found to have high sensory quality in terms of colour, aroma, taste, texture, and overall consumer acceptability.97
5. Food applications of VCO
Currently, consumers' preference leans towards natural, safe, and healthy products. Thus, the need for developing functional foods is increasing to address the growing demand for healthier food options. Owing to its MCFAs, VCO is emerging as a healthy alternative as a therapeutic or functional food ingredient. Attempts are being made to expand its food applications, which are discussed here in this section.
5.1. VCO as a low-fat substitute
Due to growing health concerns among consumers, high-fat ingredients in milk and dairy products such as cheese,99 mayonnaise,100 ice-cream,101 and cheese analogues102 are being replaced by vegetable oils or fats, including VCO. Milk fat in ice cream is replaced with VCO, which shows good appearance, texture, and flavor.101 Spice spreads are developed using VCO, trans-free fat, spice blend, and coconut residue. These spice spreads exhibited good spreadability, stability, and sensory acceptability.103 A corn milk-based cheddar cheese analogue was prepared with VCO (15–25% w/w) and an emulsifier (Tween80), which exhibited good colour, aroma, taste, and texture.102 The replacement of soybean oil with VCO in mayonnaise formulation improved its quality while retaining the organoleptic properties.100 Mohammed et al. (2022) developed an emulsion with VCO and observed that it mimicked the properties of a low-fat replacer.104 In this study, emulsified VCO functioned as an egg replacer in mayonnaise formulation with improved viscosity, texture, and stability. The development of egg-free mayonnaise is gaining high importance as a good product for vegan and health-conscious consumers. Therefore, the sensory and storage stability of the egg-free VCO mayonnaise formulations need to be further investigated. Since VCO was reported to alter favourably the ratio of low-density and high-density lipoprotein cholesterols, besides reducing total cholesterol, phospholipids, and triglycerides in the blood,102 it can be employed as a low-fat substitute in dairy products.
5.2. VCO based oleogels
Oleogels are defined as liquid vegetable oils entrapped within a thermo-reversible three-dimensional gel matrix using oleo-gelators/structural agents to form semi-solid fats. The characteristics of oleogelators, such as molecular weight and concentration, hydrophobic/hydrophilic nature, crystallinity, and glass transition temperature, influence the final properties (such as opacity, texture, melting temperature, and oil binding capacity) of tailored oleogels. The type of processing method employed, process parameters involved, and degree of unsaturation of the liquid oil phase also influence the final properties of oleogels.105 Currently, research in oleogel formulations is being carried out to encapsulate and deliver liposoluble bioactive compounds for food, nutraceutical, and pharmaceutical applications.105,106
Oleogels are being developed with VCO as an oil phase through a fatty acid crystallization mechanism.107–111 In this gelation mechanism, the oleo-gelators are dispersed in the oil phase (VCO) and then heated and cooled sequentially. These oleo-gelators rearrange themselves during the cooling step to form a crystalline/polymeric oleogel network.105,112 The structural components used in VCO-based oleogels and their food applications are mentioned in Table 2. Oleogels developed using VCO and stearic acid are reported to possess textural properties such as firmness, stickiness, spreadability, and adhesion.107 Such oleogels demonstrated the potency to replace solid fats (i.e., saturated and trans fatty acids) in food formulations, such as shortenings and spreads in confectionery, bakery, dairy, meat products, and fried snack products.107 Edible oleogels developed using VCO and ethyl cellulose showed good thermal stability and high oil-binding capability. Edible VCO-beeswax oleogels were reported to improve the stability of bioactive compounds of VCO against oxidation during storage.111 Further, oleogels can also be utilised to develop edible oleofilms and oleogel-based 3D-printed foods.107
Table 2 Summary of various VCO products and their food applications
System/product |
Structural components |
Method/Mechanism |
Food applications |
References |
VCO based oleogels |
VCO (20% w/w), stearic acid (40% w/w, lycopene (extracted from tomatoes) (40% w/w) |
Fatty acid crystallization method |
Encapsulation and delivery of lycopene |
109
|
VCO based oleogels |
VCO and steric acid (2–10%) |
Fatty acid crystallization method |
As a fat replacer in food products |
107
|
VCO based oleogels |
VCO (95, 90, and 85% w/w) and ethyl cellulose (5, 10, and 15% w/w) |
Fatty acid crystallization method |
Delivery of bioactive compounds |
108
|
VCO based oleogels |
VCO (94, 91, and 88% w/w) and beeswax (6, 9, and 12% w/w)/candelilla wax (6, 9, and 12% w/w) |
Fatty acid crystallization method |
As a fat replacer in food products |
110
|
VCO based oleogels |
VCO (92.4, 90, 87.5, and 84.5% w/w), and beeswax (7.7, 10, 12.5, and 15.5% w/w) |
Fatty acid crystallization method |
As a shortening replacer in cookies |
111
|
VCO based creamy nanoemulsion |
VCO (23.6% loaded with 2.5 w/w% ketoprofen), Tween 80 (30.53%), water (45.87%) |
Homogenization (10 000 rpm for 5 min) |
Used for drug delivery |
113
|
O/W nano-emulsion |
VCO (32%), Tween 80 (32%) and water (36%) |
Homogenization (10 000 rpm for 5 min) |
Drug delivery |
114
|
VCO based nanoemulsion |
VCO (2% w/w), sodium caseinate (3% w/w) and water (95% w/w) |
Homogenization (11 000 rpm for 15 min) followed by ultrasonication (60% amplitude with a frequency of 20 kHz ± 50 Hz and a power of 750 W for 5 min) |
To ensure uniform distribution of oil/fat in croaker surimi gel |
115
|
VCO based nanoemulsion |
β-glucan powder, α-tocopherol, VCO, epigallocatechin gallate |
Improved oxidative stability and lowered microbial loads in croaker surimi paste |
115
|
O/W nanoemulsions |
VCO and hydrogenated castor oil (5 wt%), and water |
Homogenization (10 800 rpm for 5 min) |
Food, pharmaceutical and cosmetics industries |
116
|
W/O nano-emulsion |
VCO (10 wt%), emulsifiers (green tea seed oil, whey protein isolate, soy lecithin, and tea saponin) (0.5–10 wt%) |
Homogenization (20 000 rpm for 2 min) followed by the ultrasonication method (320 W and 15 min) |
Food and beverages |
117
|
VCO based nano-emulsion |
4% VCO, 1% lycopene, 93% water, 2% lecithin |
Ultrasonication (600 W cm2, 20 kHz for 30 min) |
As a delivery system for lycopene in yoghurt and enhanced its anti-oxidant properties |
118
|
VCO based nano-emulsion |
Ethanolic black cumin oleoresin extract (1%), VCO (3%), Tween 80 (17.3%), PEG 400 (8.7%), and 70% water (70%) |
Homogenization (30 000 rpm for 30 min) |
Not available |
119
|
O/W emulsion |
VCO (9.4%), gum arabic (1.3%), xanthan gum (1%), modified starch (1.3%) stevia (1%, w/v), potassium sorbate (0.1%, w/v) and citric acid (0.08%, w/v) |
Homogenization (7000 rpm for 1.5 min) |
As a food supplement |
120
|
O/W emulsion |
VCO (7.5 wt%), gum arabic (1 wt%), xanthan gum (0.8 wt%), modified starch (1 wt%), stevia (1 wt%), potassium sorbate (0.1 wt%), citric acid (0.08 wt%) |
Homogenization (7000 rpm for 1.5 min) |
Not available |
121
|
VCO (10 wt%), gum arabic (1 wt%), xanthan gum (0.8 wt%), modified starch (2 wt%), stevia (1 wt%), potassium sorbate (0.1 wt%), citric acid (0.08 wt%) |
O/W emulsion |
VCO (50% (v/v)), whey protein isolate, retinyl acetate (0.70 mg) |
Hand homogenizer mixer (speed setting 5) |
To encapsulate vitamin A |
122
|
O/W emulsion |
VCO (80%), water (18%), honey (2%), emulsifier (Tween 80 : span 80, 2 : 3) (0.75%) and citric acid (0.02–0.06 g/100 mL emulsion) |
Homogenization method (15 000 rpm for 4 min) |
Not available |
123
|
O/W emulsion |
VCO (80%), water (16%), sucrose (4%), citric acid (0.02%), emulsifier (Tween 80 : Span 80, 2 : 3) (0.75%) |
Homogenization (15 000 rpm for 4 min) |
Not available |
124
|
Encapsulated VCO powder |
VCO (40%), maltodextrin, sodium caseinate, gum arabic, whey protein concentrate, gelatin and soy lecithin |
Spray drying (flow rate: 10 mL min−1, inlet temperature: 180 ± 5 °C and outlet temperature: 85 ± 5 °C) |
Not available |
125
|
Encapsulated VCO powder |
Maltodextrin (13.0%), sodium caseinate (4.4%), soy lecithin (1.0%), VCO (11.6%) and water (70%) |
Supercritical CO2 – spray drying (16 MPa, 40 °C and 3 mL min−1) |
Not available |
126
|
(O/W) Pickering emulsion |
VCO (30% w/w), maltodextrin, microcrystalline cellulose |
Homogenization (14 000 rpm for 5 min) |
As a non-dairy creamer |
127
|
Encapsulation of VCO powder |
Spray drying (flow rate: 5 mL min−1, inlet temperature: 150 ± 3 °C and outlet temperature: 70 ± 3 °C) |
Encapsulated VCO powder |
Gum arabic : maltodextrin (75 : 25% w/w), VCO (25% w/w), Tween 80 |
Spray drying (flow rate: 6 mL min−1, inlet air temperature: 160 °C and outlet air temperature: 50–60 °C) |
Not available |
54
|
Emulsion followed by encapsulation of VCO powder |
VCO (10%), sodium caseinate, maltodextrin, soy lecithin (30%) and water (60%) |
Homogenization (8000 rpm, 180 MPa), spray drying and freeze drying |
Non-dairy cream powder |
128
|
VCO microspheres |
Hydrolyzed VCO (5%), sodium alginate (2%), CaCl2 (2%), PEG (1%), aquadest |
Extrusion through a syringe |
Not available |
129
|
5.3. VCO based emulsions
Nowadays, emulsions are gaining importance as novel carriers of food supplements, protecting and facilitating the delivery of functional compounds, such as lipids, phenolics, vitamins, flavonoids, and alkaloids, which are derived from natural sources.130 The development of VCO-based emulsions was initiated recently, and the details are given in Table 2. Sweeteners such as sorbitol, honey, glucose, and stevia are added to O/W emulsions, with VCO as the oil phase, to enhance stability besides the sweetness.124 These sweeteners are found to increase the viscosity of the emulsion, thereby preventing demulsification mechanisms.121,123 Antimicrobial agents such as citric acid and potassium sorbate are also being added to VCO-based emulsions to enhance their shelf life.123 VCO-based emulsions prepared with natural polysaccharides, such as gum arabic, xanthan gum, modified starch, and stevia, are found to exhibit good stability for 3 months, when stored at room temperature (25 °C).121 VCO-based emulsions will indirectly increase the ease of incorporation of VCO into food products.120–122
Nano-emulsions with droplet sizes in the range of 10 to 1000 nm emerged as kinetically stabilized emulsions and have received considerable attention for food applications due to their high stability. Nano-emulsions developed with VCO as a liquid phase and their food applications113–119 are briefly mentioned in Table 2. A nano-emulsion was prepared with ethanolic Noni fruit extract (0.3% w/w) and VCO (4.5% w/w) and showed stability for 5 days.131 Paramita et al. (2022) developed a nano-emulsion using VCO, alpha-cyclodextrin, and Tween80 with a total solid content of 49.29% (w/w).132 Such a VCO-based nano-emulsion showed no changes in the color, odour, and phase separation even after 3 weeks of storage at a temperature of 4 °C.
Emulsions stabilized using solid (colloidal) particles instead of low molecular weight surfactants are called Pickering emulsions. These solid particles are adsorbed at the oil–water interface, thus increasing the emulsion stability against Ostwald ripening, flocculation, and coalescence.133 A VCO-in-water Pickering emulsion is prepared using microcrystalline cellulose and maltodextrin through the homogenization method.127,134 The emulsion is found to be stable for 3 months.127,134 Pickering emulsions with VCO as an oil phase are found to have desirable appearance, rheological properties, and stability and could replace unhealthy fats (saturated fatty acids and trans-fats) in food products.133 These Pickering emulsions, being surfactant-free, are gaining increased importance in food applications.
In recent times, emulsions have also been used to develop emulsified films for packaging applications. VCO was incorporated into corn starch/sodium alginate/gum arabic emulsion, and later a composite film was fabricated from it. Such emulsified films have shown improved water and oxygen barrier properties.135 The addition of VCO to potato starch films improved their mechanical and water barrier properties. Such VCO-incorporated films are also reported to inhibit the growth of Staphylococcus aureus, Listeria monocytogenes and Escherichia coli, thus enhancing their application for food packaging.136
5.4. Encapsulated VCO powders
VCO has bioactive compounds such as phenolic compounds (protocatechuic, vanillic, gallic, caffeic, syringic, ferulic, and p-coumaric acids),137 which are susceptible to degradation by exposure to moisture, temperature, oxygen, and light. The encapsulation technique provides a protective barrier to bioactive compounds and increases their dispersibility, oxidative stability, and bioavailability.138 The most widely used microencapsulation techniques include spray-drying,127,134 freeze-drying,128 fluidized bed coating,139,140 and coacervation phase separation, followed by freeze-drying.141 Spray-drying is a commonly used encapsulation technique for converting liquid foods into powders, as it offers the advantages of drying at wet bulb temperature, quick evaporation, low contact time with hot air, and low cost.128 VCO as a core material is mixed with wall materials to form an emulsion and later spray-dried to form encapsulated powders. The wall materials for developing encapsulated VCO powders are mentioned in Table 2. VCO encapsulated with maltodextrin and sodium caseinate showed high encapsulation efficiency (80.51%) with good antioxidant activity and oxidative stability.125 Nurhadi et al.127 prepared a Pickering emulsion and spray-dried it to produce encapsulated VCO powders that can be used as a non-dairy creamer in food formulations. In spray-drying, even though VCO powders are obtained at wet bulb temperature with a shorter contact time, the dried powders travel along with hot air to the cyclone separator. Thus, heat-sensitive VCO is destroyed during this travel period. Also, the produced encapsulated powders have a wide particle size distribution.
Mohammed et al.128 developed a VCO non-dairy cream powder through spray drying, followed by fluidized bed coating/drying. In the fluidized bed coating, the emulsion is sprayed over the fluidized bed and dried using an air stream under controlled conditions of temperature and humidity.140 Such encapsulated VCO powders improved the reconstitution properties, oxidative stability, and flowability.128 Encapsulation by fluidized bed coating has the advantages of low operating cost, temperature control, and high thermal efficiency. However, the process is time-consuming.140
The Supercritical Fluid Extraction of Emulsions (SFEE) is the latest and emerging alternative process for encapsulating bioactive compounds142 and can be easily scaled up.71 The supercritical fluids used in this method rapidly extract the organic phase (containing wall material and bioactive compounds) from emulsion143 and produce tailor-made encapsulated microparticles/microcapsules that are free from solvent traces.71,144 Such produced microspheres are reported to have high encapsulation efficiencies and high bioactive ingredient loading.126,144 In this method, a VCO-based emulsion is sprayed into the precipitation chamber and gets solubilized in SC-CO2 (which is continuously fed into the chamber). Reducing the pressure in the precipitation chamber and releasing SC-CO2 until it reaches ambient pressure enable the formation of encapsulated VCO powders.126 Hee et al. (2017) encapsulated VCO from an O/W emulsion using SC-CO2 with mild operating temperature (∼40 °C) and pressure (16 MPa) and reported an encapsulation efficiency of 80.2%.126 This SFEE process offers advantages such as precise control of the particle size and morphology of encapsulated powders with greater homogeneity. Also, the reduced particle size results in an increased surface area, consequently increasing the solubilization rate as well as stability in aqueous systems.142
VCO produced by the fermentation process was reported to have antifungal effects against Candida species and other fungi.145 Hariyadi et al.129 hydrolysed VCO using Rhizomucor miehei lipase and later encapsulated it using sodium alginate by extruding through a syringe to obtain VCO microspheres. These microspheres were reported to possess good antibacterial and antifungal activity. Encapsulation eases the handling of VCO in the form of free-flowing powder, extending its shelf life during storage. These encapsulated VCO powders increased the convenience in functional food applications.
5.5. Virgin coconut oil extracts
Conventional solvent extraction is a common method used to extract bioactive compounds from natural resources. After extraction, organic solvent removal is needed. These organic solvents are often toxic and strictly regulated worldwide nowadays. As an alternative, vegetable oils are used as green solvents for the extraction of natural compounds. These vegetable oils are found to increase extraction yields, protect bioactive compounds from degradation, and enhance their bio-accessibility.146 Thus, the use of vegetable oils as solvents aligns with the principles of green chemistry and also promotes safer and more sustainable extraction methods in various food and pharmaceutical industries.
VCO as a green solvent in extraction processes is being explored, thus offering more environmentally friendly and sustainable options. VCO has been used as a solvent to extract propolis from Trigons sp.,147 flavonoids from propolis,148 xanthone, α-mangosteen, γ-mangosteen from mangosteen pericarp,149,150 β-sitosterol from green coffee,151etc. Such VCO-extracted polyphenols enhanced the antibacterial activity against E. coli when compared with conventional extracts (water, ethanol, and propylene glycerol).147,152 The findings mentioned in Table 3 support that VCO can be used as a green solvent to extract bioactive compounds from natural sources. Wahyuni and Murdinah98 extracted carotenoids from seaweed (Padina sp.) powder using VCO as solvent. Such enriched oils could be directly applied in functional food formulations and as cosmetic ingredients. Sungpad et al.155 employed VCO as a solvent medium to extract α-mangosteen from mangosteen pericarp, and such extracted VCO was further used as a dispersed phase for nano-emulsion and nano-emulgel formulations. Such developed formulations are reported to have greater antioxidant and antibacterial activities as compared with bulk extracts. Also, the formulated nano-emulgels showed higher mangosteen release (87–92%) than their normal emulgels (74–78%).155 Such formulated VCO emulsions/emulgels have the potential to deliver polyphenols in food supplements, nutraceuticals, pharmaceuticals, and cosmetic applications.
Table 3 Summary of VCO used as extraction solvent for the recovery of bioactive compounds from different natural sources
Bioactive compounds |
Method of extraction |
Extraction conditions |
Yield |
References |
Propolis from Trigons sp. |
Solvent extraction |
Incubated at 40 °C in a shaker in a dark room for 7 days |
Not available |
147
|
α-Mangostin from mangosteen pericarp |
SC-CO2 extraction (VCO as co-solvent) |
Pressure (35 MPa), temperature (60 °C), VCO (20% w/w), and CO2 flow rate (1.7 g min−1) |
17.55 ± 2.55% |
146
|
α-Mangostin from mangosteen pericarp |
Solvent extraction |
Incubated at 40 °C for 24 h |
252.95 ± 14.72 mg/100 g |
150
|
γ-Mangostin from mangosteen pericarp |
117.37 ± 16.99 mg/100 g |
Polyphenols from mangosteen pericarp |
Ultrasound-assisted extraction |
Feed/solvent ratio (1 : 6.6), time (7 min), intensity (20 kHz) |
360.32 ± 13.92 mg GAE/100 g |
149
|
Xanthone from mangosteen pericarp |
SC-CO2 extraction |
Pressure (430 bar), extraction (70 °C), VCO (40% w/w), and CO2 flow rate (1.08 ± 0.30 kg h−1) |
31%; 28.2 mg g−1 extract |
153
|
Flavonoids from propolis |
Solvent extraction |
Incubated in a water bath for 2.5 h at 50 °C |
1.1% |
148
|
Flavonoids from propolis |
SC-CO2 extraction |
Pressure (150 bar), temperature (50 °C) and CO2 flowrate (25 g min−1) |
25.2% |
148
|
Astaxanthin from cincalok |
Ultrasound-assisted extraction |
Intensity (20 kHz), probe (13 mm), time (3 min), amplitude (30%) |
100.62 μg g−1 of dry cincalok |
154
|
Carotenoid |
Maceration |
Feed/solvent ratio (1 : 10(w/w)),temperature (65 °C), and time (1 h) |
2.73 ± 0.36 μg g−1 |
98
|
β-Sitosterol from green coffee |
Ultrasound-assisted extraction |
Feed/solvent ratio (8 : 5 w/w), intensity (70 kHz), temperature (50 °C), sand time (5 min) |
393.80 ± 11.13 mg kg−1 |
151
|
The recovery yields of polyphenols and other bioactive compounds extracted through conventional extraction methods with VCO as solvent are reported to be low. To overcome this problem, non-conventional extraction methods, such as supercritical fluid extraction, microwave treatment, ultrasonication, etc., are simultaneously performed with VCO as a green solvent to achieve maximum phenolic recovery rates along with improved bioactivity. The extraction conditions and recovery yields of bioactive compounds from various plant sources through non-conventional extraction methods and VCO used as a green solvent are mentioned in Table 3. Ultrasound-assisted extraction (UAE) with VCO as solvent achieved a high polyphenol yield (360.32 ± 13.92 mg GAE/100 g) from mangosteen pericarp.149 Ultrasound exerts a cavitation effect, allowing greater penetration of VCO into the solid matrix. As a result, the targeted bioactive compounds quickly diffuse from the solid feed to the solvent phase (VCO), thus achieving higher extraction yields. The low viscosity of VCO also contributes to high extraction yields.149 Compared with olive, sesame, and grape seed oils, VCO as a solvent in UAE achieved a high astaxanthin concentration (100.62 μg g−1 dry mass) from cincalok. Also, the fatty acid profile of VCO showed no significant difference after extracting astaxanthin from cincalok.154
VCO is used as a co-solvent in SC-CO2 extraction to recover phenolic compounds (α-mangosteen and xanthones) from mangosteen pericarp146,153 and flavonoids from propolis.148 In SC-CO2 extraction, VCO increased the extraction efficiency of desired compounds from solid feed. Besides the solvent/feed ratio, temperature, and pressure maintained in SC-CO2 extraction, the degree of penetration of VCO into solid feed is influenced largely by its viscosity and interfacial tension. In the SC-CO2 extraction of mangosteen pericarp, higher bio-accessibility (91%) of α-mangosteen was obtained with VCO as co-solvent than conventional ethanolic (30%) and hexane (50%) extractions.153
4. Conclusions and future perspectives
The increasing popularity of VCO due to its bioactive compounds and associated health benefits has been portrayed. Current wet methods and recent novel processing methods for VCO production have been presented including their advantages/disadvantages and challenges. However, novel methods such as SCFE and microwave, ultrasound, and pulsed electric field treatments to produce VCO need further research on the quality of oil and retention of phenolic and bioactive compounds in order to establish their large-scale industrial applications.
The yield and quality (i.e., flavour and composition) of VCO will differ depending on the process methods employed, age and variety of coconuts, geographical location, and pre-treatments of coconut milk. High yield, low moisture and free fatty acid contents, acceptable colour and flavour, and high retention of bioactive compounds are essential characteristics that influence the choice of wet methods for VCO production. Therefore, further research should focus on VCO production methods with improved economics, i.e., high oil yield, while meeting the quality standards.
Due to the growing awareness of the health benefits of VCO, the focus on the development of food products incorporating VCO is gradually increasing. The stability of VCO incorporated in the matrices of convenience foods needs to be studied in detail. In the present review, VCO is highlighted as a functional food as well as a functional food ingredient. In conclusion, further research will be helpful in promoting the consumption of VCO as such or in food formulations for health benefits.
Data availability
This review paper does not present new data. Therefore, the data availability details are not applicable.
Conflicts of interest
The authors declare that they have no known competing financial interests or personal relationships that could have appeared to influence the work reported in this paper.
References
- D. R. Wickramasinghe Mudiyanselage and I. Wickramasinghe, J. Agric. Food Res., 2023, 12, 100554 CAS.
- A. Narayanankutty, J. T. Job, A. Moothakoottil Kuttithodi, A. Sasidharan, P. B. Benil, V. Ramesh, M. Farouk Elsadek, H. Rizwana and M. M. Essam El-Din, J. King Saud Univ., Sci., 2023, 35, 102404 CrossRef.
- W. Y. Koh, X. X. Lim, B. H. Khor, B. Rasti, T. C. Tan, R. Kobun and U. Uthumporn, Beverages, 2024, 10, 34 CrossRef.
- P. M. Divya, B. S. Roopa, C. Manusha and P. Balannara, J. Food Sci. Technol., 2023, 60, 441–452 CrossRef CAS PubMed.
- H. M. Henrietta, K. Kalaiyarasi and A. S. Raj, J. Sustain. Environ. Manage., 2022, 1, 257–264 Search PubMed.
-
Oils & Fats International, 2017, pp. 25–27.
- S. Sundrasegaran and S. H. Mah, eFood, 2020, 1, 381–391 CrossRef.
- A. Deen, R. Visvanathan, D. Wickramarachchi, N. Marikkar, S. Nammi, B. C. Jayawardana and R. Liyanage, J. Sci. Food Agric., 2021, 101, 2182–2193 CrossRef CAS PubMed.
- A. Habarakada, P. A. B. N. Perumpuli, W. T. V. Thathsaranee and I. P. Wanninaika, Food Res., 2021, 5, 226–235 Search PubMed.
- S. P. Koh and K. Long, J. Trop. Agric. And Fd. Sci., 2012, 40, 35–44 Search PubMed.
- H. H. Jakfar, K. Pontas, R. Mamat, M. R. Salleh, M. Zulrika and Ahmadi, Fermentation, 2023, 9(5), 434 CrossRef CAS.
- S. N. Raghavendra and K. S. M. S. Raghavarao, J. Food Eng., 2010, 97, 341–347 CrossRef CAS.
- S. N. Raghavendra and K. S. M. S. Raghavarao, J. Am. Oil Chem. Soc., 2011, 88, 481–487 CrossRef CAS.
- A. Rohman, Irnawati, Y. Erwanto, E. Lukitaningsih, M. Rafi, N. A. Fadzilah, A. Windarsih, A. Sulaiman and Z. Zakaria, Food Rev. Int., 2021, 37, 46–66 CrossRef CAS.
-
C. B. J. Villarino, A. R. P. Basinang, M. M. M. Velasquez, J. M. D. Pagulayan, P. K. A. Ong and M. C. C. Lizada, MDPI AG, 2021, p. 111.
- R. K. Agarwal, MOJ Food Process. Technol., 2017, 4(2), 00087, DOI:10.15406/mojfpt.2017.04.00087.
- M. de Moura e Dias, N. Pais Siqueira, L. Lopes da Conceição, S. Aparecida dos Reis, F. Xavier Valente, M. Maciel dos Santos Dias, C. de Oliveira Barbosa Rosa, S. Oliveira de Paula, S. L. P. da Matta, L. Licursi de Oliveira, J. Bressan and M. do C. Gouveia Peluzio, J. Funct. Foods, 2018, 48, 472–480 CrossRef CAS.
- N. A. A. Ghani, A. A. Channip, P. Chok Hwee Hwa, F. Ja'afar, H. M. Yasin and A. Usman, Food Sci. Nutr., 2018, 6, 1298–1306 CrossRef CAS.
- I. Angeles-Agdeppa, J. S. Nacis, M. V. Capanzana, F. M. Dayrit and K. V. Tanda, J. Funct. Foods, 2021, 83, 104557 CrossRef CAS PubMed.
- S. K. Amit and R. Jamwal, Food Chem. Adv., 2023, 2, 100203 CrossRef.
- Z. A. Maini and C. M. Lopez, Heliyon, 2022, 8(8), e10154 CrossRef CAS.
- K. Satheeshan, C. Author, B. Seema, A. Meera Manjusha and J. Pharmacogn, Phytochem., 2019, 8(3), 2119–2123 CAS.
- F. O. Nitbani, P. J. P. Tjitda, F. Nitti, J. Jumina and A. I. R. Detha, ChemBioEng Rev., 2022, 9, 442–461 CrossRef CAS.
- R. S. Iswati and I. Nuraini, Embrio, 2023, 15, 112–118 CrossRef.
- P. Sandupama, D. Munasinghe and M. Jayasinghe, J. Future Foods, 2022, 2, 41–52 CrossRef.
- K. S. Ibrahim and E. M. El-Sayed, Neurophysiology, 2020, 52, 169–175 CrossRef CAS.
- S. P. Illam, A. Narayanankutty, S. P. Kandiyil and A. C. Raghavamenon, J. Food Sci., 2021, 86(5), 1620–1628, DOI:10.1111/1750-3841.15705.
- E. Mansouri, S. Asghari, P. Nikooei, M. Yaseri, A. Vasheghani-FarahanI and M. J. Hosseinzadeh-Attar, Nutr. Neurosci., 2024, 27, 487–498 CrossRef CAS PubMed.
- B. R. L. de A. Meireles, M. A. Alcântara, I. de, L. B. Polari, A. G. de Souza, N. A. dos Santos, C. V. B. Grisi and A. M. T. de M. Cordeiro, J. Therm. Anal. Calorim., 2022, 147, 3591–3598 CrossRef CAS.
- A. Setyawati, M. S. Sangkala, S. Malasari, N. Jafar, E. L. Sjattar, S. Syahrul and H. Rasyid, Nutrients, 2023, 15, 1–10 CrossRef PubMed.
- M. H. de Vasconcelos, R. L. Tavares, M. L. da V. Dutra, K. S. Batista, A. B. D'Oliveira, R. O. Pinheiro, R. de A. Pereira, M. dos S. Lima, M. G. da S. S. Salvadori, E. L. de Souza, M. Magnani, A. F. Alves and J. de S. Aquino, Food Funct., 2023, 14, 6455–6469 RSC.
- C. MEI CHAN, S. S. SH ABDULLAH, K. L. ABDULLAH, I. ZAINAL ABIDIN and Y. BEE WAH, Clin. Exp. Health Sci., 2022, 12, 799–804 CrossRef.
- A. Tansakul and P. Chaisawang, J. Food Eng., 2006, 73, 276–280 CrossRef.
- X. Lu, H. Su, J. Guo, J. Tu, Y. Lei, S. Zeng, Y. Chen, S. Miao and B. Zheng, Food Hydrocolloids, 2019, 96, 385–395 CrossRef CAS.
- A. Negi, S. Nimbkar, R. Thirukumran, J. A. Moses and V. R. Sinij, Food Chem., 2023, 137415 Search PubMed.
- F. A. Perrechil and R. L. Cunha, J. Food Eng., 2010, 97, 441–448 CrossRef CAS.
-
D. J. McClements, Food Emulsions, CRC Press, 2015 Search PubMed.
- G. D. Sorita, S. P. Favaro, A. Ambrosi and M. Di Luccio, Trends Food Sci. Technol., 2023, 133, 99–113 CrossRef CAS.
-
C. Gek Khoo, A. Hamid Nour, N. Abdurahman, C. Khoo and N. Azhari, Production of Virgin Coconut Oil (VCO) by Centrifugation Method, 2011 Search PubMed.
- T. O. Nurah, F. WMADB, C. Ranil, G. Isona and J. Vijay, Afr. J. Food Sci., 2017, 11, 58–66 CrossRef.
- Y. C. Wong and H. Hartina, Orient. J. Chem., 2014, 30, 237–245 CrossRef CAS.
- G. Pairoh and T. Tipvarakarnkoon, J. Med. Bioeng., 2013, 2, 191–195 CAS.
- T. S. T. Mansor, C. Man, A. Afiq and K. Nurul, Int. Food Res. J., 2012, 19(3), 837–845 CAS.
- V. P. Dia, V. V. Garcia, R. C. Mabesa and E. M. Tecson-Mendoza, Philipp. Agric. Sci., 2005, 88, 462–475 Search PubMed.
- N. K. Mohammed, Z. T. Samir, M. A. Jassim and S. K. Saeed, Mater. Today: Proc., 2021, 42, 2000–2005 CAS.
- N. Em, S. Thomachan, S. Gopal, B. Pathrose, S. Kt and R. Molu, Pharm. Innov. J., 2023, 12, 44–48 CrossRef.
- R. Arlee, S. Suanphairoch and P. Pakdeechanuan, Int. Food Res. J., 2013, 20, 2103–2109 Search PubMed.
- Y. Srivastava, A. D. Semwal and A. Majumdar, Cogent Food Agric., 2016, 2(1), 1164929, DOI:10.1080/23311932.2016.1164929.
- Z. Masyithah, Orient. J. Chem., 2017, 33, 3069–3076 CrossRef CAS.
- T. Nguyen-Son and T. Nguyen-Minh, Eur. Online J. Nat. Soc. Sci., 2022, 11(4), 1805–3602 Search PubMed.
-
H. A. Raudya, S. Putra, Rusli and N. Heryana, in IOP Conference Series: Earth and Environmental Science, Institute of Physics, 2023, vol. 1168 Search PubMed.
- T. Senphan and S. Benjakul, J. Lipid Sci. Technol., 2016, 118(5), 761–769 CrossRef CAS.
- L. Amran, B. Februadi, M. Musdalifa, M. Muhpidah and D. Muspirah, Curr. Res. Nutr. Food Sci., 2023, 11, 174–186 Search PubMed.
- T. Kulandasamy, S. K. Rao and W. Farzana, Food Humanity, 2023, 1, 650–661 CrossRef.
- X. Li, L. P. Martínez-Padilla, X. Q. Xu, B. Zisu and P. Juliano, J. Food Eng., 2018, 222, 93–99 CrossRef CAS.
- L. P. Martínez-Padilla, F. S. Hernández-Rojas, M. G. Sosa-Herrera and P. Juliano, J. Food Sci. Technol., 2022, 59, 3857–3866 CrossRef.
- A. Kurniawan, Int. J. Sci. Technol. Res., 2019, 8, 1 Search PubMed.
- E. Aytaç, Sep. Sci. Technol., 2022, 57, 426–432 CrossRef.
- N. Diyanna, M. Yusof, W. A. Fahmi and W. Mohamad, J. Agrobiotechnol., 2019, 10, 35–45 Search PubMed.
-
N. C. Saad, A Novel Process for Demulsification of Coconut Oil Milk Emulsions by Microwave Radiation, UMP, 2010 Search PubMed.
- Y. J. Ng, P. E. Tham, K. S. Khoo, C. K. Cheng, K. W. Chew and P. L. Show, Bioprocess Biosyst. Eng., 2021, 44, 1807–1818 CrossRef CAS PubMed.
- U. Patil and S. Benjakul, J. Food Sci., 2018, 83, 2019–2027 CrossRef CAS.
- B. J. Villarino, L. M. Dy and M. C. C. Lizada, Lwt, 2007, 40, 193–199 CrossRef CAS.
-
S. Harimurti, R. M. Rumagesan and Susanawati, in IOP Conference Series: Materials Science and Engineering, Institute of Physics Publishing, 2020, vol. 874 Search PubMed.
- Y. Rohyami, H. Hidayat, A. Rizky Wijaya and I. Fatimah, Processes, 2022, 10, 315, DOI:10.3390/pr10020315.
- J. Hardi, R. A. P. Buheli and A. Ridhay, AIP Conf. Proc., 2023, 2719, 030028 CrossRef CAS.
- P. P. Soo, Y. Ali, O. M. Lai, C. H. Kuan, T. K. Tang, Y. Y. Lee and E. T. Phuah, Eur. J. Lipid Sci. Technol., 2020, 122, 1–13 CrossRef.
- P. A. Uwineza and A. Waśkiewicz, Molecules, 2020, 25(17), 3847 CrossRef CAS PubMed.
- A. G. Pereira, M. Carpena, L. Cassani, F. Chamorro, J. Simal-Gandara and M. A. Prieto, Food Biosci., 2023, 55, 102960, DOI:10.1016/j.fbio.2023.102960.
- M. Azizi, C. Yunus, M. N. Rozak, L. Nian-Yian, M. Syafiq, H. Ruslan, H. Mohd-Setapar, M. Abbas and A. Zaini, J. Teknol., 2014, 67(2), 11–15 Search PubMed.
- D. F. Tirado, A. Latini and L. Calvo, J. Food Eng., 2021, 290, 110215 CrossRef CAS.
- M. H. Zuknik, N. A. Nik Norulaini, W. S. Wan Nursyazreen Dalila, N. R. Ali and A. K. M. Omar, J. Food Eng., 2016, 168, 240–244 CrossRef CAS.
- R. C. N. Thilakarathna, L. F. Siow, T. K. Tang and Y. Y. Lee, J. Food Sci. Technol., 2023, 60, 1222–1236 CrossRef CAS.
- A. Syahir, S. Sulaiman, M. Mel and H. Veny, Biomass Convers. Biorefin., 2023, 1–11, DOI:10.1007/s13399-023-04400-9.
- T. Leong, P. Juliano and K. Knoerzer, Food Eng. Rev., 2017, 9, 237–256 CrossRef CAS.
- P. Juliano, J. Nutri. Health Food Eng., 2015, 2, 228–231 Search PubMed.
- P. Juliano, A. Kutter, L. J. Cheng, P. Swiergon, R. Mawson and M. A. Augustin, Ultrason. Sonochem., 2011, 18, 963–973 CrossRef CAS.
- T. Leong, P. Juliano, L. Johansson, R. Mawson, S. McArthur and R. Manasseh, Ind. Eng. Chem. Res., 2015, 54, 12671–12681 CrossRef CAS.
- T. Leong, L. Johansson, R. Mawson, S. L. McArthur, R. Manasseh and P. Juliano, Ultrason. Sonochem., 2016, 28, 118–129 CrossRef CAS PubMed.
- T. Leong, L. Johansson, P. Juliano, R. Mawson, S. McArthur and R. Manasseh, Ultrason. Sonochem., 2014, 21, 1289–1298 CrossRef CAS.
- M. A. F. M. Gaber, F. J. Trujillo, M. P. Mansour, C. Taylor and P. Juliano, J. Food Eng., 2019, 252, 60–68 CrossRef CAS.
- P. Juliano, F. Bainczyk, P. Swiergon, M. I. M. Supriyatna, C. Guillaume, L. Ravetti, P. Canamasas, G. Cravotto and X. Q. Xu, Ultrason. Sonochem., 2017, 38, 104–114 CrossRef CAS.
- R. N. Pereira and A. A. Vicente, Food Res. Int., 2010, 43, 1936–1943 CrossRef.
- X. Lv, Z. Song, J. Yu, Y. Su, X. Zhao, J. Sun, Y. Mao and W. Wang, Fuel, 2020, 279, 118417, DOI:10.1016/j.fuel.2020.118417.
- K. Nowosad, M. Sujka, U. Pankiewicz and R. Kowalski, J. Food Sci. Technol., 2021, 58, 397–411 CrossRef PubMed.
- H. Lu, Z. Pan, Z. Miao, X. Xu, S. Wu, Y. Liu, H. Wang and Q. Yang, Chem. Eng. J. Adv., 2021, 100103, 1–17, DOI:10.1016/j.ceja.2021.100103.
- I. A. Dewi, A. F. Mulyadi, F. Aryvyanto and S. Sucipto, AIP Conf. Proc., 2019, 050005, DOI:10.1063/1.5115681.
-
G. Pataro and G. Ferrari, in Pulsed Electric Fields to Obtain Healthier and Sustainable Food for Tomorrow, Academic Press, 2020, pp. 283–310, DOI:10.1016/B978-0-12-816402-0.00013-6.
- S. Liu, S. Yuan and H. Zhang, Molecules, 2022, 27, 1–15 Search PubMed.
-
R. Mozuraityte, V. Kristinova and T. Rustad, in Encyclopedia of Food and Health, ed. B. Caballero, P. M. Finglas and F. Toldrá, Academic Press, Oxford, 2016, pp. 186–190 Search PubMed.
- V. Y. Waisundara, C. O. Perera and P. J. Barlow, Food Chem., 2007, 101, 771–777 CrossRef CAS.
- F. Fatimah and E. C. Sangi, Chem. Prog., 2010, 3(2), 65–69 Search PubMed.
-
J. Y. Parlindungan, H. Hitijahubessy, J. J. Pongkendek, N. B. Sumanik and A. L. Rettob, in Journal of Physics: Conference Series, Institute of Physics Publishing, 2020, vol. 1569 Search PubMed.
- E. Subroto, Int. J. Emerg. Trends Eng. Res., 2020, 8, 3003–3011 CrossRef.
- S. R. Utami, M. Afrilia and R. G. Mahardika, Indones. J. Chem. Res., 2022, 9(3), 197–200 CrossRef CAS.
-
I. Subadra, B. Setiaji and I. Tahir, Prosiding Seminar Nasional DIES Ke 50 FMIPA UGM, 2005, pp. 1–9 Search PubMed.
- S. C. Rangani and K. K. D. S. Ranaweera, Appl. Food Res., 2023, 3(2), 100325, DOI:10.1016/j.afres.2023.100325.
- T. Wahyuni and Murdinah, IOP Conf. Ser. Earth Environ. Sci., 2023, 1137, 12038 CrossRef.
- A. El Gawad IA, J. Nutr. Food Sci., 2015, 5, 1–6 Search PubMed.
- B. J. Muhialdin, L. L. Ying, A. Farouk, A. Shobirin and M. Hussin, J. Food Nutr. Res., 2019, 7, 65–70 CrossRef CAS.
- S. Y. Choo, S. K. Leong and F. S. H. Lu, Food Sci. Technol. Int., 2010, 16, 531–541 CrossRef CAS PubMed.
-
N. Aini, J. Sumarmono, B. Sustriawan, V. Prihananto and E. Priscillia, in IOP Conference Series: Earth and Environmental Science, Institute of Physics Publishing, 2020, vol. 443 Search PubMed.
- V. Hitlamani, M. R. Asha, G. Suresh Kumar and R. Chetana, Food Humanity, 2023, 1, 933–939 CrossRef.
- N. K. Mohammed, H. Ragavan, N. H. Ahmad, A. Shobirin and M. Hussin, Foods Raw Mater., 2022, 10, 76–85 CAS.
- S. Manzoor, F. A. Masoodi, F. Naqash and R. Rashid, Food Hydrocolloids Health, 2022, 2, 100212 CAS.
- A. Puscas, V. Muresan, C. Socaciu and S. Muste, Foods, 2020, 9(1), 70 CrossRef CAS.
- P. E. Thomas, M. Saravanan and P. Prabhasankar, Int. J. Food Sci. Technol., 2023, 58, 1434–1443, DOI:10.1111/ijfs.16305.
- S. S. Silva, L. C. Rodrigues, E. M. Fernandes, F. C. M. Lobo, J. M. Gomes and R. L. Reis, Polymers, 2022, 14(12), 1–16, DOI:10.3390/polym14122473.
- H. Dhulipalla, I. Syed, M. Munshi and R. N. Mandapati, J. Oleo Sci., 2023, 72, 733–743 CrossRef CAS.
- S. Sharma, V. Parmar, R. Sharma and B. Singh, Int. J. Food Sci. Technol., 2023, 58, 3293–3302 CrossRef CAS.
- W. C. Sung and Y.-C. Lin, J. Food Nutr. Res., 2017, 5, 697–707 CrossRef CAS.
- F. Valoppi, A. Salmi, M. Ratilainen, L. Barba, T. Puranen, O. Tommiska, P. Helander, J. Heikkilä and E. Haeggström, Sci. Rep., 2020, 1–13 Search PubMed.
- R. Suraweera, M. H. F. Sakeena, S. Rk, P. Hgp and S. Mhf, Artic. Asian J. Pharm. Clin. Res., 2015, 8(1), 275–279 CAS.
- A. Sanjeewani, M. H. F. Sakeena and N. A. Sanjeewani, Int. J. Sci. Res. Publ., 2013, 3(12), 1–6 Search PubMed.
- A. Gani and S. Benjakul, Food Hydrocolloids, 2018, 82, 34–44 CrossRef CAS.
- S. Pengon, N. Chinatangkul and C. Limmatvapirat, Asian J. Pharm. Life Sci., 2018, 13, 409–414 Search PubMed.
- W. Gao, Z. Jiang, X. Du, F. Zhang, Y. Liu, X. Bai and G. Sun, J. Oleo Sci., 2020, 69, 227–239 CrossRef CAS.
- G. Himanath, R. Shruthy, R. Preetha and V. Sreejit, ACS Food Sci. Technol., 2021, 1, 1538–1549 CrossRef CAS.
- H. Syapitri, S. Letchmi Panduragan, S. Babu, V. Purwandari and C. Masyitah Thaib, Preparation of Black Cumin Extract Nanoemulsion Using the Oil Phase of Virgin Coconut Oil (VCO) Tween 80 and PEG 400 Surfactants, Malays. J. Med. Health Sci., 2022, 18(SUPP2), 2636–9346 Search PubMed.
- Y. P. Khor, S. P. Koh, K. Long, S. Long, S. Z. S. Ahmad and C. P. Tan, Molecules, 2014, 19, 9187–9202 CrossRef PubMed.
- Y. P. Khor, S. P. Koh, K. Long, G. H. Chong and C. P. Tan, J. Am. Oil Chem. Soc., 2018, 95, 1329–1339 CrossRef CAS.
- E. J. Tanglao, A. B. Nanda Kumar, R. R. Noriega, M. E. Punzalan and P. Marcelo, MATEC Web Conf., 2019, 268, 01002 CrossRef CAS.
-
L. Wiyani, A. Aladin and A. Abriana, in Proceedings of the 5th International Conference on Food, Agriculture and Natural Resources (FANRes 2019), Atlantis Press, 2020, vol. 194, pp. 246–250 Search PubMed.
-
L. Wiyani, A. Aladin, S. Yani, Rahmawati and Mustafiah, in AIP Conference Proceedings, American Institute of Physics Inc., 2023, vol. 2595 Search PubMed.
- Y. Y. Hee, C. P. Tan, R. Abdul Rahman, N. Mohd Adzahan, W. T. Lai and G. H. Chong, Int. J. Food Eng., 2015, 11, 61–69 CrossRef CAS.
- Y. Y. Hee, C. P. Tan, R. A. Rahman, M. Noranizan, R. L. Smith and G. H. Chong, J. Supercrit. Fluids, 2017, 130, 118–124 CrossRef CAS.
- B. Nurhadi, S. Selly, S. Nurhasanah, R. A. Saputra and H. R. Arifin, Ital. J. Food Sci., 2022, 34, 67–85 CAS.
- N. K. Mohammed, N. H. Ahmad, B. J. Muhialdin and A. S. Meor Hussin, J. Food Sci. Technol., 2024, 61(3), 528–538, DOI:10.1007/s13197-023-05860-7.
- D. M. Hariyadi, A. Fitri, S. Sudarma, T. Purwanti and T. Erawati, J. Adv. Pharm. Technol. Res., 2022, 13, 238–242 CrossRef CAS PubMed.
- D. C. Widianingrum, H. Khasanah, L. Purnamasari, M. E. Krismaputri and S. G. Hwang, Vet. Med., 2023, 68, 27–32 CrossRef CAS PubMed.
- D. Jose, C. Muenmuang, N. Kitiborwornkul, P. Yasurin, S. Asavasanti, P. Tantayotai and M. Sriariyanun, J. Indian Chem. Soc., 2022, 99, 100729 CrossRef CAS.
- V. Paramita, S. Fitri Novia, H. Dwi Ariyanto, B. Pramudono, H. Yoshii, H. Kusumayanti and R. Amalia, Mater. Today: Proc., 2022, 63, S312–S317 CAS.
- G. Øye, S. Simon, T. Rustad and K. Paso, Curr. Opin. Food Sci., 2023, 50, 101003 CrossRef.
- B. Nurhadi, A. Angeline, N. Sukri and H. R. Arifin, J. Appl. Polym. Sci., 2022, 139(5), 51576, DOI:10.1002/app.51576.
- M. Xiao, B. Tang, J. Qin, K. Wu and F. Jiang, Food Packag. Shelf Life, 2022, 32, 100819 CrossRef CAS.
- Z. Fangfang, B. Xinpeng, G. Wei, G. Wang, Z. Shi and C. Jun, Int. J. Food Sci. Technol., 2020, 55, 192–200 CrossRef CAS.
- S. Neela and N. B. L. Prasad, As. J. Food Ag. Ind., 2017, 2012, 355–363 Search PubMed.
- A. Priyanto, D. A. Hapidin and K. Khairurrijal, ChemBioEng Rev., 2022, 393–408 CrossRef.
- G. Reineccius, S. Patil and V. Anantharamkrishnan, Molecules, 2022, 27, 1854, DOI:10.3390/molecules27061854.
- V. I. Sousa, J. F. Parente, J. F. Marques, M. A. Forte and C. J. Tavares, Polymers, 2022, 14, 1730, DOI:10.3390/polym14091730.
- M. G. Bordón, G. N. Barrera, A. González, P. D. Ribotta and M. L. Martínez, J. Sci. Food Agric., 2023, 103, 3322–3333 CrossRef PubMed.
- D. Cerro, A. Torres, J. Romero, C. Streitt, A. Rojas, S. Matiacevich and S. Machuca, J. Supercrit. Fluids, 2024, 211, 106306 CrossRef CAS.
- C. Prieto, L. Calvo and C. M. M. Duarte, J. Supercrit. Fluids, 2017, 124, 72–79 CrossRef CAS.
- S. Klettenhammer, G. Ferrentino, H. S. Zendehbad, K. Morozova and M. Scampicchio, J. Food Eng., 2022, 312, 110746 CrossRef CAS.
- D. O. Ogbolu, A. A. Oni, O. A. Daini and A. P. Oloko, J. Med. Food, 2007, 10, 384–387 CrossRef CAS PubMed.
- W. Jun, C. Chi, J. Shuen, R. Lee, S. Jr., S. Lee, Y. Yi, S. Yee, W. Kiat, N. Hanani, Z. Abidin, S. Abdul and H. Lim, Process Biochem., 2019, 87, 213–220 CrossRef.
- N. Pujirahayu, H. Ritonga, L. Satya Agustina and Z. Uslinawaty, Int. J. Pharm. Pharm. Sci., 2015, 7, 419–422 CAS.
- P. D. Pattiram, F. Abas, N. Suleiman, E. M. Azman and G. H. Chong, PLoS One, 2022, 17(4), e0266673, DOI:10.1371/journal.pone.0266673.
- C. Sungpud, W. Panpipat, A. Sae Yoon and M. Chaijan, J. Food Sci. Technol., 2020, 57, 4032–4043 CrossRef CAS PubMed.
- C. Sungpud, W. Panpipat, A. Sae Yoon and M. Chaijan, Process Biochem., 2019, 87, 179–186 CrossRef CAS.
- Z. Jia, L. Wan, Z. Huang and W. Zhang, Foods, 12(11), 2235, DOI:10.3390/foods12112235.
- N. S. Thammarat, D. Unit and N. S. Thammarat, Int. Food Res. J., 2020, 27, 111–120 Search PubMed.
- S. L. Kok, W. J. Lee, R. L. Smith, N. Suleiman, K. N. Jom, K. Vangnai, A. H. Bin Sharaai and G. H. Chong, J. Supercrit. Fluids, 2021, 176, 105305 CrossRef CAS.
- D. I. Prayitno, E. N. Dewi, D. Pringgenies and T. H. P. Brotosudarmo, OCL: Oilseeds Fats, Crops Lipids, 2022, 29(15), 1–8, DOI:10.1051/ocl/2022008.
- C. Sungpud, W. Panpipat, M. Chaijan and A. S. Yoon, PLoS One, 2020, 15(1), 1–22, DOI:10.1371/journal.pone.0227979.
|
This journal is © The Royal Society of Chemistry 2024 |
Click here to see how this site uses Cookies. View our privacy policy here.