Stability of target polyphenols of leaf-added virgin olive oil under different storage conditions over time
Received
2nd March 2024
, Accepted 5th April 2024
First published on 12th April 2024
Abstract
The degree to which olive oil is protected against oxidation and rancidity is highly reliant on the presence and quantities of antioxidant polyphenols in the oil. On this account, the principal polyphenols need to be intactly available (withstand oxidation) in large numbers over the shelf-life of the oil, which often comes with a challenge as the oil is generally exposed to various atmospheric conditions during domestic, or even, throughout manufacturing and commercial situations, and consequently, a drastic depletion of endogenous antioxidants may occur in the oil before being consumed. That being the case, this study examined the stability of target polyphenols of virgin olive oil (VOO) enriched with olive leaf powders (0.3 mm particle size), compared to the non-enriched VOO (control),after oxygen and light exposures over time points. The main purpose was to observe the magnitude of depletions of the chief polyphenols in the leaf-added oils upon the given storage conditions. For each storage condition, there were relatively similar trends of changes in both leaf-added and leaf-free oils (from the same malaxation: 30 and 60 min). However, quantitatively, the leaf-added oils (particularly 30 min malaxation) contained significant proportions of oleuropein and verbascoside over a six-month period; with final concentrations of 5.03 and 4.15 mg kg−1 oil (for oleuropein) and 3.50 and 2.88 mg kg−1 oil (for verbascoside) when exposed to light and oxygen, respectively. On the other hand, only trace levels of these compounds were found in leaf-free oils. Overall, the outcome of this study support the inclusion of olive leaves in the oil because considerable levels of polyphenols, and correspondingly antiradical capacity, remained having been exposed to oxygen and light over the course of time.
Sustainability spotlight
The keepability of polyphenols in virgin olive oil is of importance because their antioxidant abilities rest highly on their quantities over the shelf-life of the oil. The results from the current study support the significant role of olive leaf addition to the oil as considerable amounts of target polyphenols (including oleuropein) remained after being exposed to the detrimental storage conditions (oxygen and light) over time. In respect of the United Nations Sustainable Development Goals, the findings of our study, with potential contributions to promoting food security and sustainable agro-industrial system, fit into the following goals: Goal 2 – “End hunger, achieve food security and improved nutrition and promote sustainable agriculture”. Goal 12 – “Ensure sustainable consumption and production patterns”.
|
Introduction
Virgin olive oil (VOO), compared to the majority of edible oils, generally has a less susceptibility to oxidation with a fairly long shelf-life (12–18 months) because it is relatively high in antioxidants (particularly polyphenols) and low in polyunsaturated fatty acids.1,2 Having said that, inadequate storage of VOO, even extra virgin olive oil (EVOO) that is the superior grade of all, may substantially cause oxidation, to the extent that it may lose its (extra) virgin attributes.1 Indeed, the development of oxidation reaction beyond growing rancidity/off-flavor, can result in significant degradation/depletion of the endogenous polyphenols, with consequent impacts on the nutritional quality of VOO. The occurrence of oil oxidation is not uncommon. Examples are: (i) improper handling/storage conditions (e.g., exposure of oil to light and oxygen) throughout manufacturing, shipping, and marketing, and (ii) mishandling under domestic conditions where the unsealed bottles are often exposed to light and/or oxygen over time. The latter may occur routinely as consumers, although generally health conscious, may not be fully aware of the proper conditions required for the conservation and soundness of the oils they are commonly using. In this respect, previous investigations dealing with the stability of olive oil have discussed potential processing approaches to retain/upgrade the nutritional properties of virgin olive oil for longer shelf life. Examples are: (i) microemulsion of vitamin-C as a potential antioxidant for improving the stability of virgin olive oil (incubated for 21 days, 60 °C). The enriched olive oil (withvitamin-C microemulsion using sesame seed oil extract, 500 μg mL−1) contained lower free acidity that was comparably close to that found in the oil treated with 200 μg mL−1 tertiary butylhydroquinone (synthetic antioxidant),3 (ii) application of bottles with the presence of oxygen active barrier (oxygen scavenger) that proved useful for the preservation of quality and authenticity of EVOO over the 13 months storage period,4 (iii) addition of fresh olive leaves (3%) in advance of oil extraction that enabled greater levels of polyphenols and antioxidant activity (DPPH), compared to those found in leaf-free olive oil,5 and (iv) upgrading the value addition of polyphenols in olive oil through their exposures to olive mill waste water fermented by Lactobacillus plantarum.6
Among which, the effect of incorporating olive leaves into edible oils has been markedly highlighted in the literature. Research studies suggest that the endogenous polyphenols of olive leaves upon their successful transfer to the oil may concentration-dependently intensify the quality and shelf life of the oil.7–11 In this respect, our recent study12 compared a range of particle size fractions of olive leaves added to crushed olives before malaxation (30 and 60 min) of olive paste. Importantly, it was found that the inclusion of olive leaf fractions with 0.3 mm particle size (in pitted crushed olives before 30 min malaxation) significantly enabled: (i) maximum recoveries of target polyphenols (oleuropein: 5.85 mg oleuropein per kg oil, verbascoside: 4.02 mg verbascoside per kg oil, and luteolin: 15.44 mg per kg oil), and antioxidant capacity, and (ii) minimum peroxide value and free acidity in the resulting virgin olive oil. These findings prompted us to extend the study towards storage stability of virgin olive oil enriched with the target polyphenols (through the addition of 0.3 mm olive leaf powders) under severe surrounding conditions (exposed to light and oxygen) over the course of six months. The main purpose was to determine the extent to which the outcome variables (total and individual polyphenols, antioxidant capacity, peroxide value, and free acidity) could be affected under the storage conditions of the leaf-added oils (30 and 60 min malaxation) compared to the non-leaf-added oils (control) over the given time points. The concentrations of the selected phenols (oleuropein, hydroxytyrosol, tyrosol, verbascoside, luteolin, and apigenin) were measured irrespective of whether they are bound molecules (in glycosidic linkage) or free molecules (free aglycones).
Materials and methods
Plant materials and chemicals
Olive mill leaves and olive fruits (Picual), were obtained from “Center for Advanced Studies in Energy and Environment”, University of Jaén, Campus of Las Lagunillas, Jaén, Spain. Sample preparations were as follows: (i) olive leaves – after initial cleaning, washing, drying (37 °C, 48 h), were ground and size reduced to 0.3 mm particle fractions. The leaf powders (vacuum packed in polypropylene bags and refrigerated) were incorporated into the mechanical processing of olive oil extraction within two weeks. (ii) Olive fruits, were manually cleaned to remove foreign objects/bruised olives, laid flat on food-grade/dry trays, refrigerated, and processed for oil extraction within two weeks.
The following chemical were obtained from Sigma-Aldrich (Saint Louis, MO, USA): (i) analytical grade – Folin–Ciocalteu, anhydrous gallic acid (≥98.0%), anhydrous sodium carbonate (≥99%), hydrochloric acid, 2,4,6-tris(2-pyridyl)-s-triazine (TPTZ), ferric chloride (FeCl3), ethanol (99.8%), 2,2-diphenyl-1-picrylhydrazyl (DPPH), 2,20-azino-bis-3-ethylbenzothiazoline-6-sulfonic acid (ABTS), and (±)-6-hydroxy-2,5,7,8-tetramethylchromane-2-carboxylic acid (trolox), acetic acid (99.8%), chloroform (99%), potassium iodide (≥99.0%), n-hexane (≥99%), and methanol (99%), (ii) HPLC grade – phenolic standards (oleuropein, verbascoside, luteolin, hydroxytyrosol, apigenin, and tyrosol with 98% w/w purity), formic acid, and acetonitrile (≥99.9%).
Study design
Preliminary findings – in our earlier study,12 through adding dry ground olive leaves prior to the malaxation, the effects of different particle size fractions of leaves (the primary factor) and their interactions with malaxation time, and olive pitting/non-pitting in the extracted olive oil (the secondary factors) on phenolic recovery and oxidative quality of the resulting virgin olive oils were assessed. Since the results of the previous study provided important insights, it served as a basis in this model project to decide on the choice of independent variables (factors). In this regard, those factors that showed statistically significant effects on the respective dependent variables, were selected in the present study.
That being the case, of the particle sizes examined earlier, the addition of 0.3 mm leaf fractions was selected in the current study to be tested under the storage conditions/durations (Table 1) in terms of the following dependent variables: (i) total phenolic content (TPC), (ii) target polar phenols consisting of oleuropein, verbascoside, hydroxytyrosol, tyrosol, luteolin, and apigenin (iii) antioxidant assays in vitro, and (iv) peroxide value and free acidity of the oil samples.
Table 1 Specifications of the study design
Subject design |
Parameters |
Coded oil samples |
Leaf addition to oil |
- With addition (0.3 mm particle size) |
- Control-30 min - Control-60 min - Leaf-added-30 min - Leaf-added-60 min |
- Without addition (control) |
Malaxation time |
- 30 min |
- 60 min |
Storage condition of oil |
- Without light/oxygen exposure |
- Light exposure only |
- Oxygen exposure only |
Mechanical processing of olive oil extraction
The mechanical extraction of olive oil was carried out using the same operation processing design applied in our earlier experiment.12 The process was initiated with crushing of olives (1000 g) using a blender with a rotation speed/temperature adjustment (Vorwerk Bimby® TM6, Germany) at 1500 rpm for 2 min. The blender was stopped in between and the stones were removed (using a pair of forceps tweezers). At this stage, 3% olive leaves (initially dried at 37 °C, 48 h and size reduced to 0.3 mm particle fractions using a grinder, Tristar KM-2270 Blade Mill, 70 g capacity, 150 W) were added to the crushed/pitted olives and further blended for 4 min (2000 rpm). The crushing was then completed with manual pressing using a ceramic mortar and pestle (4 min) to exert pressure and promote diffusion of the oil out of the plant cell walls. The crushed olives were then subjected to malaxation through which they were mixed at 300 rpm for different durations (30 and 60 min). This step (malaxation) facilitates the coalescence of the oil droplets wherein the free oil can be extracted. The olive paste was then centrifuged (3500 rpm, 5 min) in Thermo-IEC polypropylene centrifuge bottles (27 °C) to obtain a two-phase separation (centrifugation decanting). The oil fraction was collected, weighed and bottled before being subjected to the storage assessments described below. Additionally, through the same procedure as described above, the control samples (without leaves) were prepared for both malaxation times. Temperature remained constant at 27 °C the extraction.
Type of cultivar, growing region, storage/handling conditions were identical for each batch of samples. The pre-processing conditions of olive leaves (drying and grinding parameters) were the same when applied during the processing of oil extraction (for both malaxation times).
Storage of oil samples
The stability of the extracted olive oil samples was analyzed over time points of a six-month storage period under various atmospheric conditions described below. All samples were stored at room temperature (20 ± 5).
Storage of oils without light/oxygen exposure.
The falcon sterile tubes loaded with oil samples, nitrogen flushed, tightly closed with the caps and wrapped with aluminum foils prior to their storage in the dark.
Storage of oils with light exposure only.
The falcon sterile tubes (unwrapped) containing oil samples nitrogen flushed, with caps on (screwed tightly) were stored in a room where the light (from sunlight and/or artificial source) was available throughout the storage.
Storage of oils with oxygen exposure only.
The oil samples without nitrogen gas flush in falcon tubes (with aluminum foils wrapped around) were stored in the dark leaving the lids loose to ensure exposure of oxygen while protecting them from atmospheric light.
Evaluation of target polyphenols through HPLC
Extraction of phenolic fractions from oil samples.
Polyphenols (hydrophilic fraction) were initially separated from the oil matrix (lipophilic fraction) using liquid–liquid extraction method of Lozano-Castellón et al.13 with slight modifications. Briefly, 2 g of oil was first weighed into a centrifuge tube. The oil was then dissolved with 2 mL of n-hexane, shaken for 1 min. Subsequently, the extraction of phenolic compounds was performed using 4 mL of methanol/water (80
:
20 v/v), vortex mixed for 1 min and centrifuged for 5 min (3000 rpm). The hydrophilic phase (methanol/water fraction) was separated from the lipophilic phase (hexane fraction), solvent evaporated, and reconstituted with HPLC-grade methanol/water (50–50, v/v). The phenolic extract, after nitrogen gas flush (Reacti-therm™ Heating Module, Pierce) was filtered through a 0.22 mm polytetrafluoroethylene membrane before HPLC experiments (as described below).
HPLC analysis of target polyphenols.
The chromatographic separations of the selected polyphenols were carried out on a reverse-phase Aquity UPLC BEH C-18 column (100 mm × 2.1 mm i.d., 1.7 mm particle size, Waters Corporation) using UHPLC (Shimadzu Nexera X2 UHPLC) connected to a diode array detector (Shimadzu SPD-M20A), and an integration system (Shimadzu LabSolutions software, Kyoto, Japan). The gradient elution program was based on the method reported by Quero et al.14 with slight modifications as described previously.12 The eluents consisted of water/formic acid (99.9/0.1 v/v) (phase A) and acetonitrile (phase B). The flow rate was 0.3 mL min−1. The column temperature remained constant at 45 °C and the injection volume was 5 μL. In advance of running HPLC, the samples/standards were passed through 0.22 μm syringe filters, and the eluents were passed through 0.22 μm membrane filters.
The chromatograms were monitored at 280 nm and individual phenols were identified with reference to the retention times of the standards and the UV spectra detector. Concentration of each phenol were calculated against the calibration curve (peak area vs. the known concentration) of the corresponding standard. The results were reported as mg target phenol per kg oil.
Evaluation of total phenolic content (TPC) through colorimetric analysis
Primarily, the hydrophilic phenolic extract was separated from the oil matrix by adding 80% aqueous methanol (2 mL) to the oil/hexane solution (1
:
1, w/v). After a 6 hours incubation in the dark, the colorimetric assay for the quantification of total polyphenols was performed using the method of Singleton and Rossi15 with slight modifications as described previously.12 Briefly, the phenolic extract (1 mL) was first reacted with Folin–Ciocalteu reagent (1 mL) before adding 20% sodium carbonate (1 mL). The solution was gently mixed and incubated in the dark (30 min). After the centrifugation at 2500 rpm for 1 min, the blue color fraction was collected and subjected to the UV-vis spectrophotometer for the absorbance reading at 765 nm. The results were expressed as mg gallic acid equivalents per kg oil (mg GAE per kg).
Evaluation of antioxidant capacity (in vitro)
The in vitro antioxidant capacity of the hydrophilic phenolic fraction (isolated from the oil matrix through the extraction of the oil/hexane mixture (1
:
1, w/v) by 80% methanol using the same method conducted for HPLC samples) was examined based on the Trolox Equivalent Antioxidant Capacity (TEAC) via DPPH,16 FRAP,17 and ABTS18 radical scavenging methods as performed and described previously.12 The absorbance readings [(515 nm) for DPPH, (593 nm) for FRAP, and (734 nm) for ABTS] were calculated against Trolox standard curve and the results were expressed as mM trolox equivalents per kg oil (mM TE per kg).
Evaluation of chemical quality of oil samples
The rancidity levels of oil samples over the storage conditions/time points were examined through peroxide value (PV) (for oxidation) and free fatty acids (FFA) (for lipolysis) using titration assays according to the official methods of American Oil Chemists' Society (AOCS)19 as described in our recent study.12 The PV results were reported as milliequivalents of active oxygen per kilogram oil (mEq. O2 per kg). The FFA results were reported as percentage of oleic acids (g/100 g, w/w).
Statistical analysis
The significant differences (p < 0.05) between the mean values (±SD) of all determinations were statistically assessed via Analysis of Variance (ANOVA) using SPSS software, version 27.0. Factorial Repeated Measures ANOVA was used to analyze whether the leaf addition had influence on the changes of each dependent variable across multiple storage times, and whether the magnitude of that influence depended on the malaxation time. The assumption of homogeneity of equal variance was assessed through the Levene's test (homogeneity of variance assumption was not violated when p-value was greater than 0.05). Each experiment was carried out in triplicate.
Results and discussion
The aim of this study was to evaluate the storage stability of virgin olive oils with added leaf powders (particle size of 0.3 mm) obtained from 30 and 60 min malaxations, compared to those without leaves (control). The choice of the particle size and malaxation time were based on the significant findings reported in our earlier study.12 In this regard, the oil samples were assessed for their storage stabilities in terms of polar target/total phenolic content, antioxidant capacity, and free acidity/peroxide values over time points (month 0, month 1, month 3, month 5, and month 6) under a set of atmospheric conditions.
Effect of storage on phenolic content of leaf-added oils
Stability of oleuropein.
As demonstrated in Fig. 1, oleuropein decreased with time under all storage conditions but the greatest loss occurred in the presence of oxygen (up to 30%) followed by those in light exposure (up to 15%). The concentrations remained more stable in light/oxygen protected, with slower drops at each storage time point (up to around 9% loss over the six months). Overall, the data suggest that the presence of olive leaves in the oil obtained from a short-term malaxation (30 min) is of benefit in terms of oleuropein preservation over the storage period. Although this compound in the leaf-added oils declined over the severe storage conditions/times, having being largely present at the initial point (month 0), remained in considerable levels over the storage period (final values: 5.41–4.15 mg kg−1 oil).
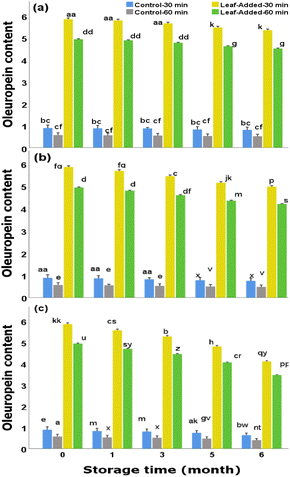 |
| Fig. 1 Changes in oleuropein content of oil samples (mg kg−1 oil) stored over time points at (20 ± 5 °C) under: (a) no light/oxygen exposure; (b) light exposure only; (c) oxygen exposure only. The 30 min and 60 min represent malaxation times. Different letters above the bars indicate statistically significant differences for each olive oil group at different time points of the same storage condition (p < 0.05). Data are shown as mean values ± standard deviation error bars. | |
The storage condition/duration is among the major factors affecting the content of oleuropein which can potentially decompose in the course of the following reactions: (i) oxidation reaction20,21 typically associated with the activities of oxidoreductase enzymes including polyphenoloxidase (PPO) that apparently have the ability to oxidize oleuropein to secondary products such as oleuropein quinone (non-bitter product)20,21 (ii) cleavage of ester bonds20,21 by the actions of enzymes such as esterase, that enables the formation of oleuropein derivatives including hydroxytyrosol, and (iii) de-glycosylation20 that generates oleuropein aglycone (a potential substrate for hydroxytyrosol formation) and glucose.
In the case of oxidation, the activities of oxidizing enzymes can be accelerated by the presence of oxygen which may be inevitably the case over the domestic storage of unsealed oil bottles particularly in the course of longer storage period. The actual mechanisms of oxidation products is yet complicated. Previous studies, dealing with biochemical pathways of bio-phenols during the storage of olive oil, detected new products. For example, in the research performed by Tsolakou et al.,22 through the storage of olive oil (airtight, in the dark at 25 °C for 24 months) a new product (oleocanthalic acid) was detected, using nuclear magnetic resonance and tandem mass spectrometry. This compound, reportedly, was produced from the oxidation of oleocanthal (a principal phenol in olive oil) that occurred during the storage. The authors of this study, through their in vitro assessment, described that oleocanthalic acid in low concentration may defense mechanism against Alzheimer's disorder.
Stability of verbascoside.
Similar to the changes observed for oleuropein content, the leaf-added oils that yielded significantly large amounts of verbascoside at the original point (month 0), despite being affected by the severe storage conditions, continued to represent substantial values over time points for the same storage experiment. As shown in Fig. 2, the trends of changes in verbascoside content showed comparably similar to those observed for oleuropein content, with oxygen exposure being the major risk factor (around 30%) followed by light exposure (around 15%). Also, the addition of olive leaves in the oil produced from the short malaxation is preferable as it maintained significantly large amounts of verbascoside over the same storage condition. Verbascoside, owing to its distinctive molecular structurethat is a heterosidic ester of caffeic acid and hydroxytyrosol, is among the powerful antioxidant compounds. However, this valuable phenol is often found in a trace level in non-enriched olive oil while being rather largely present in non-processed olives and olive leaves. This issue was in part studied in our recent experiment12via comparing different particle size fractions of added olive leaves. It was found that the addition of 0.3 mm of leaves to the pitted olives during crushing, significantly assisted in rising verbascoside content in the resulting VOO. Following up the same approach in the this model study, it was found that such method of leaf addition offers additional advantages in respect of verbascoside content upon storage. In other words, the impacts of oxygen and light, although unfavorably caused depletions, were comparatively less detrimental to the leaf-added oils as they contained relatively significant amounts of verbascoside over the six month period (3.50 and 2.88 mg kg−1, in the presence of light and oxygen, respectively).
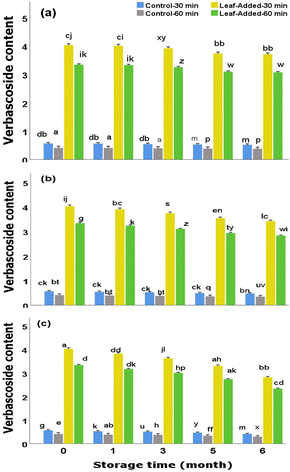 |
| Fig. 2 Changes in verbascoside content of oil samples (mg kg−1 oil) stored over time points at (20 ± 5 °C) under: (a) no light/oxygen exposure; (b) light exposure only; (c) oxygen exposure only. The 30 min and 60 min represent malaxation times. Different letters above the bars indicate statistically significant differences for each olive oil group at different time points of the same storage condition (p < 0.05). Data are expressed as mean values ± standard deviation error bars. | |
Stability of hydroxytyrosol.
As illustrated in Fig. 3, unlike the changes observed for oleuropein and verbascoside, the content of hydroxytyrosol rose upon the six-month storage with oxygen exposure (around 15%), light exposure (around 9%), and no light/oxygen exposure (around 7%). This may suggest that the rise in hydroxytyrosol resulted from simultaneous falls in oleuropein which often takes place throughout the storage of olive oil.23 However it remains difficult to ascertain this (whether the formation of hydroxytyrosol came from hydrolysis of oleuropein aglycone) as other types of hydroxytyrosol-linked secoiridoids could be involved in hydroxytyrosol biosynthesis. In the research of Castillo-Luna et al.,24 the increase in hydroxytyrosol, over the 12 months storage of olive oil (20 °C in the dark), was found to be associated with the degradation of secoiridoid aglycones including oleacein and oleomissional.
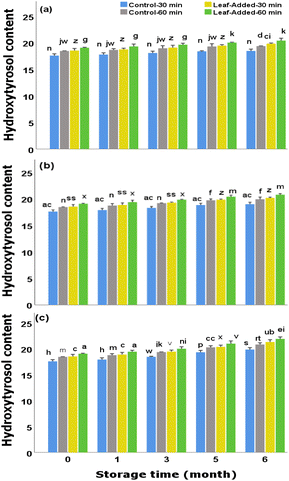 |
| Fig. 3 Changes in hydroxytyrosol content of oil samples (mg kg−1 oil) stored over time points at (20 ± 5 °C) under: (a) no light/oxygen exposure; (b) light exposure only; (c) oxygen exposure only. The 30 min and 60 min represent malaxation times. Different letters above the bars indicate statistically significant differences for each olive oil group at different time points of the same storage condition (p < 0.05). Data are expressed as mean values ± standard deviation error bars. | |
Overall the data here (Fig. 3) demonstrate that the oils without olive leaves that initially contained significant quantities of hydroxytyrosol (though, not as large as leaf-added oils), comparatively maintained a sizable proportion over time points for the same storage condition. That is to say, the storage conditions did not adversely affect the content of hydroxytyrosol in leaf-free oils. Moreover, there were no significant differences between malaxation times (for both control and leaf-added groups) under the same storage condition.
Stability of tyrosol.
Tyrosol and hydroxytyrosol are both in the group of phenolic alcohols, and are structurally similar, only hydroxytyrosol has an extra hydroxyl group with substantially higher antioxidant potential. Similar to the trend of changes observed for hydroxytyrosol, the content of tyrosol increased with the storage time, but proportionally to a lesser extent (Fig. 4). The concentrations rose over the six month period of time by 8% (with oxygen exposure), 5% (with light exposure), and 3% (with no light/oxygen exposure). As seen in Fig. 4, at each time point of the same storage condition, there were no significance differences of tyrosol values between malaxation times of leaf-added oils (leaf-added 30 min vs leaf-added 60 min), as well as between malaxation times of leaf-free oils (control 30 min vs control 60 min).
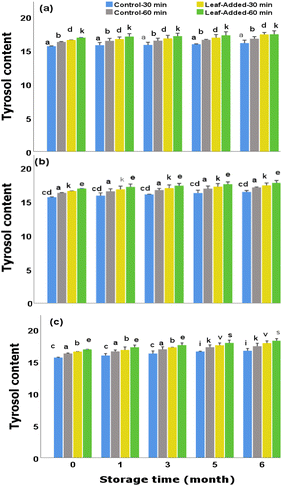 |
| Fig. 4 Changes in tyrosol content of oil samples (mg kg−1 oil) stored over time points at (20 ± 5 °C) under: (a) no light/oxygen exposure; (b) light exposure only; (c) oxygen exposure only. The 30 min and 60 min represent malaxation times. Different letters above the bars indicate statistically significant differences for each olive oil group at different time points of the same storage condition Data are expressed as mean values ± standard deviation error bars. | |
In general, compared to the other major polyphenols of olive leaves, tyrosol may exert less antioxidant power but it has the ability to remain more stable/unaffected during the oil storage.25,26 As mentioned earlier (in section: stability of hydroxytyrosol), the rise in hydroxytyrosol and the drop in oleuropein in the corresponding storage conditions/duration could suggest that oleuropein, through its hydrolytic cleavage, served partly as a substrate for the production of hydroxytyrosol. Similar biotransformation potentially occurred here as tyrosol rather increased under corresponding storage conditions which may explain where it was possibly derived from.
Stability of luteolin.
Luteolin, a flavonoid, is among the key flavone groups in olive oil with competing antioxidant potential. As seen in Fig. 5(a), the use of inert gas (nitrogen) in the headspace of the tubes assisted in preserving more of luteolin over time points (around 7% loss over the six months). On the other hand, the presence of light, followed by the oxygen exposure, caused significant declines in luteolin content. The values in all oil groups dropped by up to around 20% (with light exposure), and 16% (with oxygen exposure) over the course of six-month period.
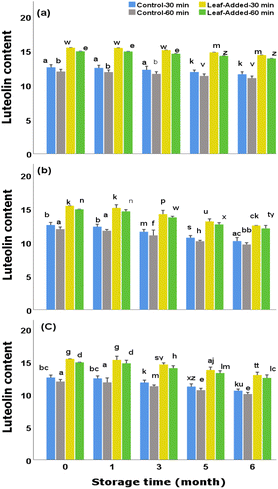 |
| Fig. 5 Changes in luteolin content of oil samples (mg kg−1 oil) stored over time points at (20 ± 5 °C) under: (a) no light/oxygen exposure; (b) light exposure only; (c) oxygen exposure only. The 30 min and 60 min represent malaxation times. Different letters above the bars indicate statistically significant differences for each olive oil group at different time points of the same storage condition Data are expressed as mean values ± standard deviation error bars. | |
In general, to suppress/diminish the adverse effects of light, it is recommended to bottle olive oils in opaque/tinted glass containers, though this is not strictly followed as some olive oil factories rather use transparent bottles. Examining the control groups (oils without leaves), it was found that, although proportionally suffered from light and oxygen exposures, they preserved reasonable content of luteolin over the storage time; final concentrations (30 min malaxation) were 11.70 mg kg−1 (without light/oxygen exposure) 10.30 mg kg−1 (with light exposure), and 10.69 mg kg−1 (with oxygen exposure). However, the oils with added leaves could be preferred over the VOO without leaves as they continued to possess larger content of luteolin for each examined storage (final range:15.60–12.64 mg kg−1). Furthermore, the changes in luteolin content did not depend on the malaxation time as marginal differences were found between the short and long malaxations of leaf-added oils, as well as between the control groups, at each storage time under the same storage condition.
Stability of apigenin.
Apigenin belongs to the same flavonoid sub-class (flavones) as luteolin does. The synergistic bio-functional effects of these two phenols have been well discussed in the literature.27–29 As shown in Fig. 6, regardless of the factor of leaf addition, apigenin remained relatively stable, with slight reductions (around 3%) in the presence of light and oxygen over six months. The data on insignificant losses of apigenin under storage/over time support previous research study wherein neither light nor oxygen affected the content of apigenin (2.4 mg kg−1) over the course of one year storage period.30
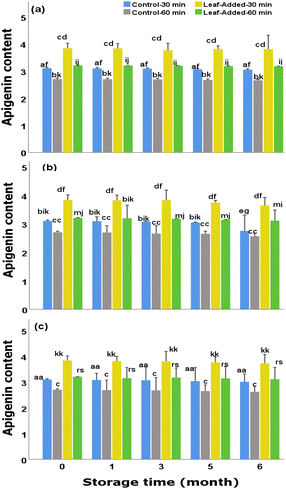 |
| Fig. 6 Changes in apigenin content of oil samples (mg kg−1 oil) stored over time points at (20 ± 5 °C) under: (a) no light/oxygen exposure; (b) light exposure only; (c) oxygen exposure only. The 30 min and 60 min represent malaxation times. Different letters above the bars indicate statistically significant differences for each olive oil group at different time points of the same storage condition (p < 0.05). Data are shown as mean values ± standard deviation error bars. | |
Different from those observed for luteolin changes, the data revealed that the content of apigenin across the oil groups depends heavily on the malaxation time as the magnitude of differences between malaxation times (in both leaf-added and control groups) were significantly high over the storage durations (for the same storage condition). In other words, the ability of olive leaves to optimally maintain the highest apigenin content, throughout the storage, rely substantially on a short-term malaxation (30 min) rather than the extended time.
Total phenolic content and its relation with antiradical capacity under storage.
The changes of TPC in oil samples under different storage conditions are shown in Fig. 7. The data demonstrate that the TPC in all oil groups declined most rapidly at the exposure of light (up to around 36%) and, slightly less sharply at the exposure of oxygen (up to 33%) upon a six month storage period. On the other hand, in the absence of light/oxygen exposure the total phenols remained more stable over the six month period of storage (with 2–5% decline) which explains the favorable effect of nitrogen flushing in the headspace as it enables replacement of oxygen/air with the inert gas, and thereby zero/minimum chance can be provided for the air/oxygen to bring about oxidations. During processing and storage, the detrimental atmospheric conditions such as light and oxygen should not come into contact with the oil. Their exposures to the oil may cause autooxidation or photosensitized oxidation which are partially responsible for the depletion of polyphenols in the oil. As a result, the oil becomes weakly stable and nutritionally degraded.
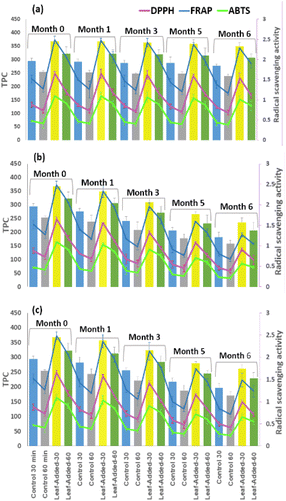 |
| Fig. 7 Comparisons between radical scavenging activities (mM TE per kg) and total phenolic content (TPC) (mg GAE per kg) of oil samples stored over time points at (20 ± 5 °C) under: (a) no light/oxygen exposure; (b) light exposure only; (c) oxygen exposure only. The oil samples: leaf-added (0.3 mm particles) and non-added (Control) obtained for 30 and 60 min malaxation times. Data are expressed as mean values ± standard deviation error bars. | |
The undesirable impact of storage conditions/duration on the decrease in polyphenol content have been highlighted in several research studies.31–33 Regardless of the variations in the experimental conditions, the data observed here on the TPC depletions in the presence of light, support those previously reported wherein the light exposure has been found to be among the key risk factors associated with the loss of phenolic content of virgin olive oil.34–36
Although the phenolic reductions across the oil groups (with/without leaves) followed the same patterns, the leaf-added oils with 30 min malaxation maintained the maximum total phenols over all time points for the same storage experiment. On the other hand, the oils without leaves (particularly with 60 min malaxation) contained the lowest phenolic content over each time point for the same storage trial.
Polyphenols have great potential for disabling free radicals owing to their ability to donate hydrogen atoms to free radicals making them weak for reactivity. This can substantially assist in minimizing the occurrence of oxidative/hydrolytic rancidity in the oil. On this account, polyphenols may contribute to extending the shelf life as well as promoting the nutritional quality of the oil.
Therefore, the greater proportion of polyphenols is expected to result in greater antioxidant capacity in the oil. To shed light on this matter, the TPC results were further compared with antiradical capacity in vitro. As seen in Fig. 7, relatively similar patterns of changes between different radical scavenging activities (DPPH, FRAP, and ABTS). exist for the same oil group, under each storage condition. In addition, the trends relatively correspond with the changes of TPC results for the same storage experiment.
Importantly, the leaf-added oils (from both malaxation times), compared to the control, maintained significantly high levels of TPC, and correspondingly showed maximum antiradical activities over time point of the same storage condition. The final values in the leaf-added oils with 30 min malaxation detected by FRAP, DPPH, and ABTS, respectively, reached the following: [1.27, 0.91, 0.57 mM TE per kg oil (with light exposure)], [1.49, 0.99, 0.66 mM TE per kg oil (with oxygen exposure)], and [2.28, 1.58, and 1.02 mM TE per kg oil (without light/oxygen exposure)].
Based on the data obtained here (Fig. 7) the antioxidant capacity of the oil samples apparently rest highly on the concentrations of polyphenols. That being the case, the findings support the inclusion of olive leaves in the oil during storage as both TPC and antiradical capacity were significantly higher compared to the non-leaf added oils (p < 0.05).
Effect of storage on chemical quality of leaf-added oils
Peroxide value (PV).
The measurement of peroxide value in olive oil is of importance to determine the initial oxidative rancidity of the oil. The occurrence and development of peroxides (the intermediate oxidation products) can continue, beyond the oil processing step, throughout packaging (bottling) and inadequate storage/handling, particularly in the presence of oxygen and light. Regardless of the factor of leaf addition, the data in this study showed increased PV in the range of 20–23% (with oxygen exposure), 25–28% (with light exposure), and around 5% (light/oxygen protected) (Fig. 8).
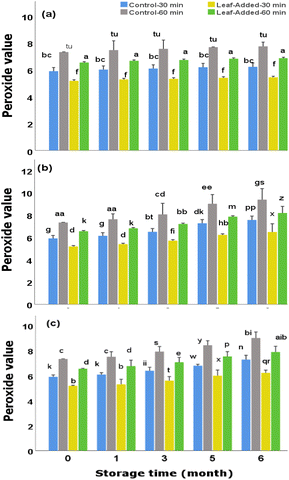 |
| Fig. 8 Changes in peroxide value of oil samples (mEq O2 per kg) stored over time points at (20 ± 5 °C) under: (a) no light/oxygen exposure; (b) light exposure only; (c) oxygen exposure only. The 30 min and 60 min represent malaxation times. Different letters above the bars indicate statistically significant differences for each olive oil group at different time points of the same storage condition. Data are expressed as mean values ± standard deviation error bars. | |
However, all groups of the oil samples showed to be qualified to be ranked as extra virgin olive oil in terms of peroxide value as the results (both added/non-added leaf oils) lied well-below the maximum limit (20.0 mEq O2 per kg oil) required for commercial standard of EVOO. More importantly, the leaf-added oil with 30 min malaxation, represented superior quality in this concept as it continued to have the lowest peroxide values over the time periods of all three storage conditions examined here.
As discussed earlier, the content of TPC dropped significantly with time under light and oxygen. The trends of falls in TPC appear to be in correlation with the rise in peroxide values of the corresponding oil samples for the same malaxation time. That is to say, the leaf-added oils maintained the highest total phenolic content and, concurrently the lowest peroxide values over time points for the same storage conditions.
Free fatty acids (FFA).
Determination of free fatty acids (free acidity) that is primarily the decomposed fatty acids within the fat molecules, is of significance to identify the hydrolytic rancidity of the oil (the hydrolysis of triglycerides that may develop by catalyzing effects of lipase enzymes).
The acidity levels increased by around 18–20% (with oxygen exposure), 12–15% (with light exposure) over the six month storage period (Fig. 9). Having said that, the values in oil samples, including control groups, did not exceed the maximum standard criteria of free acidity designated for EVOO (0.8%, grams of oleic acid/100 g oil) by International Olive Council (IOC).37
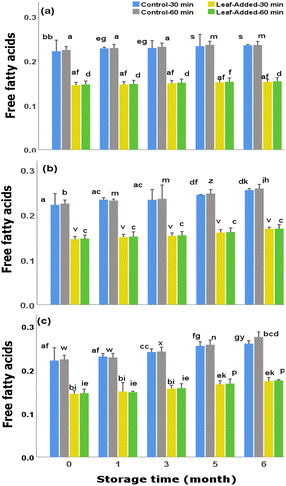 |
| Fig. 9 Changes in free fatty acids of oil samples (% oleic acid) stored over time points at (20 ± 5 °C) under: (a) no light/oxygen exposure; (b) light exposure only; (c) oxygen exposure only. The 30 min and 60 min represent malaxation times. Different letters above the bars indicate statistically significant differences for each olive oil group at different time points of the same storage condition. Data are shown as mean values ± standard deviation error bars. | |
Above all, the leaf-added oils (from both malaxation times) contained the lowest levels of FFA (around 0.17% w/w) over six months with oxygen exposure. In this regard, the inclusion of olive leaves in olive oil can be of value for maintaining minimum free acidity of olive oil, particularly over the extended/inadequate storage conditions that is often the case during domestic uses.
Conclusions
Taken as a whole, the data from this study showed that the leaf-added oils, compared to the oils without leaves, maintained the maximum levels of polyphenols over time points (the magnitudes of depletions were significantly lower than those observed in leaf-free oils under corresponding storage condition). Importantly, the data justify the significant role of the presence of olive leaves in the oil as considerable proportions of oleuropein and verbascoside remained after being exposed to oxygen and light over time.
The outcome of this study can be of use for the researchers dealing with the keepability of other/specific polyphenols (with molecular characteristics similar to those examined in the current study) during an extended period of storage. The data can also be of value from the perspective of consumers as the inclination is often towards the assumption that EVOO sustain its supreme nutritional quality before the expiration date, rather than taking the factor of proper handling/storage into an equal consideration. In support of the findings of this study, future research should focus on an in-depth research on the storage stability of olive oil considering a broader range of factors, including storage temperature and packaging, within a large-scale investigation. Also more research is needed to deeply characterize the phenols of the leaf-added oils to ascertain whether/to what extent they are in bound or free forms.
Author contributions
Fereshteh Safarzadeh Markhali: investigation; methodology; writing manuscript; conceptualization; data analysis; resources. José A. Teixeira: conceptualization; supervision; methodology; review and editing; resources.
Conflicts of interest
There are no conflicts to declare.
Acknowledgements
We thank Eulogio Castro, Center for Advanced Studies in Energy and Environment, University of Jaén, Campus of Las Lagunillas, Jaén, Spain, for supplying the olive fruits and olive leaves. This study was supported by the Portuguese Foundation for Science and Technology (FCT), under the scope of the strategic funding of UID/BIO/04469/2020 unit.
References
-
J. Ayton, R. J. Mailer and K. Graham, in Rural Industries Research and Development Corporation, RIRDC Publication No.12/024, 2012, pp. 1–89 Search PubMed.
- J. R. Morelló, M. J. Motilva, M. J. Tovar and M. P. Romero, Food Chem., 2004, 85(3), 357–364 CrossRef.
- M. Osanloo, N. Jamali and A. Nematollahi, Food Sci. Nutr., 2021, 9(7), 3712–3721 CrossRef CAS PubMed.
- T. Cecchi, P. Passamonti and P. Cecchi, Food Chem., 2010, 120, 730–735 CrossRef CAS.
- D. Sevim, O. Tuncay and O. Koseoglu, J. Am. Oil Chem. Soc., 2013, 90, 1359–1369 CrossRef CAS.
- F. Kachouri and M. Hamdi, Process Biochem., 2004, 39(7), 841–845 CrossRef CAS.
- S. Achat, V. Tomao, K. Madani, M. Chibane, M. Elmaataoui, O. Dangles and F. Chemat, Ultrason. Sonochem., 2012, 19(4), 777–786 CrossRef CAS PubMed.
- M. A. Mezghani, M. Tekaya, A. Mguidich, I. Zouari, M. Ayadi, O. Elloumi, D. Saidana, S. B. Mansour-Gueddes, G. Flamini and B. Mechri, J. Food Meas. Charact., 2023, 17(1), 751–764 CrossRef.
- I. Tarchoune, C. Sgherri, J. Eddouzi, A. Zinnai, M. F. Quartacci and M. Zarrouk, Molecules, 2019, 24, 545 CrossRef PubMed.
- Í. M. G. Marx, S. Casal, N. Rodrigues, R. Cruz, A. C. Veloso, J. A. Pereira and A. M. Peres, Eur. Food Res. Technol., 2022, 248, 171–183 CrossRef.
- S. Ammar, H. Kelebek, A. Zribi, M. Abichou, S. Selli and M. Bouaziz, Food Res. Int., 2017, 100, 477–485 CrossRef CAS PubMed.
- F. Safarzadeh Markhali, J. A. Teixeira and J. Sus, Food Technol., 2023, 1(6), 896–905 Search PubMed.
- J. Lozano-Castellón, A. López-Yerena, A. Olmo-Cunillera, O. Jáuregui, M. Pérez, R. M. Lamuela-Raventós and A. Vallverdú-Queralt, Antioxidants, 2021, 10(4), 540 CrossRef PubMed.
- J. Quero, L. F. Ballesteros, P. Ferreira-Santos, G. R. Velderrain-Rodriguez, C. M. Rocha, R. N. Pereira, J. A. Teixeira, O. Martin-Belloso, J. Osada and M. J. Rodríguez-Yoldi, Antioxidants, 2022, 11(5), 828 CrossRef CAS PubMed.
- V. L. Singleton and J. A. Rossi, Am. J. Enol. Vitic., 1965, 16(3), 144–158 CrossRef CAS.
- W. Brand-Williams, M. E. Cuvelier and C. Berset, LWT–Food Sci. Technol., 1995, 28, 25–30 CrossRef CAS.
- L. F. Benzie and J. J. Strain, Anal. Biochem., 1996, 239(1), 70–76 CrossRef PubMed.
- R. Re, N. Pellegrini, A. Proteggente, A. Pannala, M. Yang and C. Rice-Evans, Free Radical Biol. Med., 1999, 26(9–10), 1231–1237 CrossRef CAS PubMed.
-
AOCS. American Oil Chemists' Society, Official Methods and Recommended Practices of the American Oil Chemists' Society, Champaign, 5th edn, 1998, vol. III Search PubMed.
- A. V. Bounegru and C. Apetrei, Int. J. Mol. Sci., 2022, 23(20), 12569 CrossRef CAS PubMed.
- A. De Leonardis, V. Macciola, F. Cuomo and F. Lopez, Food Chem., 2015, 175, 568–574 CrossRef CAS PubMed.
- A. Tsolakou, P. Diamantakos, I. Kalaboki, A. Mena-Bravo, F. Priego-Capote, I. M. Abdallah, A. Kaddoumi, E. Melliou and P. Magiatis, J. Agric. Food Chem., 2018, 66, 7337–7346 CrossRef CAS PubMed.
- C. Romero and M. Brenes, J. Agric. Food Chem., 2012, 60, 9017–9022 CrossRef CAS PubMed.
- A. Castillo-Luna, I. Criado-Navarro, C. A. Ledesma-Escobar, M. A. López-Bascón and F. Priego-Capote, Food Chem., 2021, 336, 127730 CrossRef CAS PubMed.
- A. Karković Marković, J. Torić, M. Barbarić and C. Jakobušić Brala, Molecules, 2019, 24, 2001 CrossRef PubMed.
- F. Safarzadeh Markhali, J. A. Teixeira and C. M. Rocha, Processes, 2020, 8, 1177 CrossRef.
- Z. B. Jiang, W. J. Wang, C. Xu, Y. J. Xie, X. R. Wang, Y. Z. Zhang, J. M. Huang, M. Huang, C. Xie, P. Liu, X. X. Fan, Y. P. Ma, P. Y. Yan, L. Liu, X. J. Yao, Q. B. Wu and E. L. H. Leung, Cancer Lett., 2021, 515, 36–48 CrossRef CAS PubMed.
- L. Zhang, C. Virgous and H. Si, J. Nutr. Biochem., 2019, 69, 19–30 CrossRef CAS PubMed.
- F. Visioli, C. Galli, G. Galli and D. Caruso, Eur. J. Lipid Sci. Technol., 2002, 104, 677–684 CrossRef CAS.
- L. Caipo, A. Sandoval, B. Sepúlveda, E. Fuentes, R. Valenzuela, A. H. Metherel and N. Romero, Foods, 2021, 10(9), 2161 CrossRef CAS PubMed.
- E. Ghanbari Shendi, D. Sivri Ozay, M. Taha Ozkaya and N. F. Ustunel, Oilseeds Fats, Crops Lipids, 2020, 27(6), 1–8 Search PubMed.
- M. L. Clodoveo, D. Delcuratolo, T. Gomes and G. Colelli, Food Chem., 2007, 102(3), 571–576 CrossRef CAS.
- A. Baiano, G. Gambacorta, C. Terracone, M. A. Previtali, C. Lamacchia and E. La Notte, J. Food Sci., 2009, 74, C177–C183 CAS.
- O. Okogeri and M. Tasioula-Margari, J. Agric. Food Chem., 2002, 50, 1077–1080 CrossRef CAS PubMed.
- F. Gutiérrez and J. L. Fernández, J. Agric. Food Chem., 2002, 50(3), 571–577 CrossRef PubMed.
- G. Luna, M. T. Morales and R. Aparicio, J. Agric. Food Chem., 2006, 54(13), 4790–4794 CrossRef CAS PubMed.
-
IOC, International Olive Council, Trade Standard Applying to Olive Oils and Olive Pomace Oils, 2019 Search PubMed.
|
This journal is © The Royal Society of Chemistry 2024 |