Recent advances in plant protein modification: spotlight on hemp protein
Received
11th November 2023
, Accepted 18th April 2024
First published on 19th April 2024
Abstract
Plant proteins are attracting increased research attention, and the modification of their functional properties is a key area of current research. Industrial hemp is a new source of protein which can be produced in a sustainable manner. Hemp protein is rich in essential amino acids such as leucine, lysine, and phenylalanine, and also comes with good digestibility and various health benefits. Despite its potential, the scope of its application is limited due to its less-than-desirable technofunctional properties including solubility, emulsifying, encapsulating and gelling properties. This review provides a snapshot of various physicochemical and enzymatic methods that are currently used to enhance the aforementioned properties of plant proteins, particularly focusing on the modification of hemp protein. It compares the structural characteristics, physicochemical properties that can be positively affected by these methods and explains the underlying principles. This review highlights the fact that combination of two or more methods and particularly implementation of protein–polysaccharide complex coacervation and protein–polyphenol conjugation and peptide–polysaccharide conjugation greatly improve the technofunctional properties and help broaden the scope of application of hemp protein.
Sustainability spotlight
It is already a challenge to sustain the food supply for a global population of 8.1 billion. With the human population continuously increasing, the demand for nutritionally balanced food is set to pose an additional challenge. Foods must meet not only the need for calories but also deliver essential physiological and health requirements. Protein, a vital macronutrient, is experiencing a growing demand. Animal-derived proteins are considered more nutritionally suitable for human needs; however, their production poses sustainability constraints due to the high input of nutrients, water, energy, and land. Therefore, society is turning to plant proteins to supplement animal-based ones. Research on new sources of plant proteins is gaining increasing attention. Industrial hemp protein, a by-product of the hemp oil industry, is receiving greater focus as an alternative protein source. In this context, the manuscript provides a concise review of the current status of science and technology regarding the extraction, characterization, and application of hemp protein. This review explores advances that can enhance the techno-functional properties of this protein with a specific focus. Thus, this work contributes to the knowledge base of the UN Sustainable Development Goals (goal 12).
|
1 Introduction
Hemp (Cannabis sativa L.) is a dicotyledonous plant of the order Rosales and the family Cannabaceae, genus Cannabis, similar to commonly known marijuana.1 Tetrahydrocannabinol (THC) and cannabidiol (CBD) are two main compounds of hemp.2 THC is a psychoactive compound, whose content needs to be strictly controlled.3 BD differs from THC in that it has no psychoactive effects and offers significant therapeutic benefits, such as anti-anxiety, arthritis relief, anti-inflammatory properties, and alleviation of neurodegenerative diseases.2,4,5 Unlike medicinal cannabis or marijuana, industrial hemp contains a low concentration (less than 0.2–0.3%) of THC and high levels of CBD, making it suitable as an ingredient of food products.6–8 Hemp was first discovered in Asia and is one of the oldest cultivated crops, with a history of cultivation and use in China dating back 5000 to 6000 years.9–11 In ancient China, hemp was used as a part of traditional medicinal formulations to treat diseases, as a functional food, and as a fiber source.1,10 Today, industrial hemp is grown as an agricultural commodity in more than 30 countries,7 with China and France leading the world as its producers and exporters.7,12,13 Currently, hemp (medicinal and industrial) is grown in most continents especially in Europe and Asia. This review is confined to industrial hemp and the term ‘hemp’ hence forth will be used to represent it.
Different parts of hemp are utilized in various industries. Hemp leaves are commonly used in the production of cigarettes, medicine, and cosmetics.7 Hemp stalks are used as raw materials for high-quality paper, textiles, and construction materials.1,7 Industrial hemp seeds are used as ingredient to produce commonly consumed foods, health foods, beverages, and other high-value products.7 Hemp seed is gaining increased attention in terms of research and application due to its high oil (about 30%) and protein (about 25%) contents.8,14–16 Hemp oil has a rich history of use in China as a medicinal oil.15,17 Currently, hemp oil is used in medicinal and food applications due to its high content of polyunsaturated fatty acids (PUFAs, over 80%), particularly linoleic (ω-6) and α-linolenic (ω-3) acids.7,15,16,18–20 The ratio of ω-6 to ω-3 in hemp oil is between 2
:
1 to 3
:
1, which is similar to the optimal value (2.5) found in the Mediterranean and Japanese diets.7 This ratio is considered beneficial for human health as it is shown to significantly reduce the risk of heart diseases.21 Hemp protein, which is the primary byproduct of hemp seed oil production, has not received due attention and it is less utilized despite immense potential.
With the growing global demand for high-quality plant proteins, hemp protein is gaining increased recognition. Currently, a significant portion of dietary protein comes from animal sources. Due to inefficient conversion of feed into animal proteins and high-water consumption, their production comes with a high cost and sustainability constraints.22 Plant-derived proteins, particularly from oilseeds, are more economical and sustainable options. Sulfur-containing amino acids, such as cysteine and methionine, are physiologically important; however, their content is limited in most plant proteins. Reports indicate that a deficiency in these amino acids can negatively impact animal growth and development.23 Plant proteins, such as pea,24 soybean,23,25 almond26 and chickpea protein27 are particularly deficient in these amino acids. Hemp protein is of interest as it is rich in cysteine and methionine.22
Hemp protein offers a number of benefits for its potential application in food industry. Firstly, the presence of hydrophobic, acidic and branched-chain amino acids at high concentration makes it a valuable source for the production of peptides with antioxidant and antihypertensive properties.28 Secondly, hemp protein is highly digestible (digestibility = 88–91%) compared to many plant proteins as shown by in vitro tests.29,30 Thus, hemp protein could be used to produce new, hypoallergenic, highly digestible ingredients which would be suitable to be used in food products.31 Thirdly, hemp protein is nutritionally rich and provides adequate amount of essential amino acids as recommended by FAO/WHO for infants and children.32 Furthermore, hemp protein is free of gluten and anti-nutritional factors making it suitable for various food industry applications.30 Similar to other plant proteins, such as soy protein and pea protein, hemp protein has the potential to be used in the production of meat analogues, edible films, gluten-free dough, and a component of active packaging.33–36
Hemp protein can be extracted into concentrate (60–70% protein) and isolate (≥90% protein) form.37,38 It is rich in high-quality storage proteins, including salt-soluble edestin (legumin) and water-soluble albumin.39 Edestin and albumin account for 60–80% and 20–30%, respectively of hemp protein obtained from defatted hemp seed.32,39–41 Hemp globulin is made up of 11S edestin and 7S globulin, with 11S edestin making up 80% and 7S globulin accounting for 5% of the hemp storage protein.32,37 Edestin is a 300 kDa homohexamer equivalent to 11S legume globulin and is composed of two subunits linked by disulfide bonds: an acidic subunit (∼34 kDa) and a basic subunit (∼18 and 20 kDa).42 The 7S globulin is composed of a 48 kDa polypeptide.29,32 The water-soluble albumin component mainly contains a 2S albumin of around 10 kDa and other minor proteins below 35 kDa.40,43 Despite above-mentioned merits, the functional properties (solubility, emulsifying properties, foam ability and surface hydrophobicity) of hemp protein are not as good as those of commonly used protein such as pea protein and soy protein which has limited its application in food industry.32,38,44
Due to above mentioned limitation on functional properties, recent studies are focused more on improving these properties in hemp protein concentrate (HPC) and isolate (HPI) by applying physicochemical and enzymatic methods. Published literature indicates that application of these methods can improve the physicochemical properties of hemp proteins to a certain degree. There is a need of comparing the effectiveness of these methods in improving above-mentioned functional properties and identifying the gap in knowledge. Thus, this review aims to survey the recent advances made on the modification of structure and function of HPC and HPI and provide a concise overview of effectiveness of these methods.
2 Modification of plant proteins by using physicochemical and enzymatic methods
In light of sustainable development, increased attention is being paid to apply environmentally friendly and cutting-edge technologies to alter the structure and properties of proteins. The methods which do not use harsh chemicals are better suited for food industry at the same time help protect the environment (Fig. 1). Nonthermal or mildly thermal methods such as high-pressure, ultrasound, pulsed electric field, cold plasma, irradiation, microwave, supercritical fluid extrusion, tribomechanical activation, complexation with polysaccharides and polyphenols have been applied to modify the structure and function of protein molecules.45–50 The physical methods commonly used to improve the properties of plant proteins together with their underlying mechanisms are presented in Fig. 2. Tables 1–3 summarizes the advantages and disadvantages of above-mentioned physical methods.
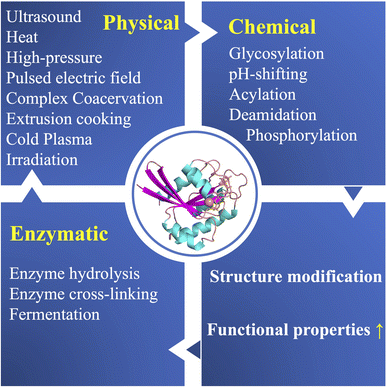 |
| Fig. 1 Schematic diagram highlighting physicochemical and enzymatic methods used to improve functional properties of plant proteins. | |
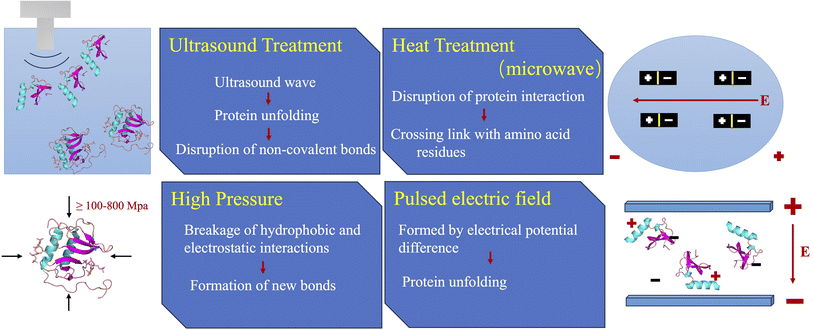 |
| Fig. 2 Schematic diagram presenting the commonly used physical methods used to improve the functional properties of plant proteins and their underlying mechanism. | |
Table 1 Physical methods applied to improve the functional properties of plant proteins
Technology |
Advantages |
Disadvantages |
Scalability |
Protein |
Functional properties |
Reference |
Ultrasound treatment |
Safe and environmentally friendly |
Negatively affect the physical structure of proteins and quality of the final food products |
Industrial scale |
Black bean |
Solubility, EAI, ESI, FC, FS, gel properties (WHC, gel strength) |
38, 53, 54, 82 and 90–93 |
Less nutritional and sensory harming |
May cause protein denaturation |
Pea |
Solubility, FC, FS, ESI |
Simpler and faster |
Hemp |
Solubility, EAI, ESI, FC, FS |
Low cost |
Excessive noise during use lead to higher cost |
Soybean |
Solubility, EAI, ESI |
Heat treatment (microwave heating, radio-frequency heating) |
High efficiency and energy frugality |
Toxic concerns |
Ongoing from pilot-scale to industrial-scale |
Pea |
Creaming stability |
94–98
|
Easy to operate |
Protein denaturation and decrease in solubility |
Rice glutelin |
Solubility, FC, FS |
Hemp |
Solubility (<80 °C), EAI |
Quinoa |
Solubility, WHC, EAI, ESI, gel ability |
High-pressure treatment (high hydrostatic pressure, dynamic high-pressure fluidization) |
Reduction in the processing times |
Enzymatic and oxidative degradation of certain food components |
Industrial-scale |
Kidney beans |
WHC, EAI, ESI, FC |
99–105
|
Ensuring food safety |
Need low temperature storage and distribution |
Sweet potato |
Independent of size and shape of the food |
Soybean |
Gel properties, rheology properties |
Not break covalent bonds |
Solubility, gel properties |
Applied at room temperature |
High installation and cost |
Lentil |
Solubility, EAI, ESI, FC, FS |
Pulsed electric field |
Green, higher extraction yield, lower energy consumption and reduced utilization of solvents |
High investment cost |
Industrial-scale |
Pea |
WHC, OHC, FC, FS |
106 and 107 |
Rice |
WHC, OHC |
Require the development of industrial equipment |
Gluten |
WHC, OHC, FC, FS |
Extrusion cooking |
Easy to texturize plant-based proteins |
Require extremely high temperature which lead to the denaturation of protein |
Industrial-scale |
Soy |
Textural properties |
33, 108 and 109 |
Hemp |
Textural properties |
Peanut |
Textural properties |
Pea |
Textural properties |
Negative effect on solubility |
Wheat gluten |
Textural properties |
Cold plasma |
Shorter treatment time |
Negative effects on the sensory and nutritional characteristics of treated foods |
Ongoing from pilot-scale to industrial-scale |
Pea |
Gel properties |
110–113
|
No thermal damages to food physical properties, flavors and nutritive components |
Wheat |
WHC, OHC, gel properties |
Irradiation |
Short acting time, strong acting force and low energy consumption, no residues |
The dosage needs to be strictly controlled, which is easy to cause irreversible damage |
Industrial-scale |
Soybean |
Solubility, EAI, ESI, WHC, OHC, FC, FS |
114–117
|
Sunflower |
Rheology properties |
Sesame seeds |
Solubility, EAI, ESI |
Complex coacervation |
Sustainable method |
Sensitive to environmental factors (e.g., temperature, ionic strength) |
Lab-scale |
Hemp |
EPS |
84 and 85 |
No chemical agent |
Pea |
Solubility |
Easy to combine with polysaccharide |
Table 2 Chemical methods applied to improve the functional properties of plant proteins
Technology |
Advantages |
Disadvantages |
Scalability |
Protein |
Functional properties |
Reference |
Glycosylation (millard reaction) |
Easy to combine with polysaccharide |
Careful control of the reaction conditions |
Lab-scale |
Soybean |
Solubility, FA, FS EAI, ESI, EPS |
118–125
|
Stable |
Lead to brown colour change and easy to form byproduct |
Pea |
Solubility, EPS |
Black bean |
Solubility, EAI, ESI |
Faba bean |
Solubility, EAI, ESI, FS |
Low cost |
Peanut |
Solubility, ES (TSI) |
pH-shifting |
More flexible structure |
The change of structure is limited, and usually needs to be combined with other modification methods |
Industrial-scale |
Faba protein |
Solubility, FC, FS |
56, 96 and 126–129 |
Pea protein |
Solubility, EAI, FC, digestibility |
Hemp |
Solubility, EAI, ES(TSI) |
Rapeseed |
Solubility |
Chickpea |
Solubility, FC, FS |
Acylation (acetylation and succinylation) |
Increasing protein electrostatic repulsion |
High consumption of solvent |
Lab-scale |
Pea |
Solubility, WHC, OHC, EC, ES, LGC |
130–132
|
Imparting protein spatial site resistance |
Not sustainable |
Soybean |
EAI, ESI, FC, FS |
High specificity, efficiency, safety, and mild reaction conditions |
Need further purification method |
Phycocyanin |
Solubility, EAI, ESI, FA, FS |
Deamidation |
Specificity, efficiency, and mild conditions |
High cost of enzyme |
Lab-scale |
Soybean |
EAI, ESI |
133–135
|
Pea |
Solubility |
Long reaction time |
Coconut |
Solubility, EAI, ESI, FC, FS |
Phosphorylation |
High improvement in WHC and OBC |
Long process duration |
Lab-scale |
Mung bean |
Solubility, WHC, OHC, FC, FS, EAI, ESI |
136
|
High energy consumption |
Low reaction efficiency |
Table 3 Enzymatic methods applied to improve the functional properties of plant proteinsa
Technology |
Advantages |
Disadvantages |
Scalability |
Protein |
Functional properties |
Reference |
EAI: emulsion activity index; ESI: emulsion stability index; WHC: water holding capacity; OHC: oil holding capacity; FC: foam capacity; FS: foam stability; EC: emulsion capacity ES: emulsion stability; TSI: EPS: emulsion physical stability; LGC: least gelation concentration.
|
Enzyme hydrolysis and cross-linking |
High selective modification |
High cost of enzyme |
Ongoing from pilot-scale to industrial-scale |
Faba bean |
Solubility, FC, OHC, EAI, ESI |
137–140
|
Sustainable method |
Long reaction time |
Pea |
Solubility, FC, FS, EC |
Low yield |
Mild reaction and avoid denaturation |
May lead to bitterness formation |
Rice bran |
Solubility, EPS |
Fermentation |
Reduction of beany flavor |
With strong pertinence, the effect of different proteins varies greatly |
Industrial-scale |
Pea |
Solubility |
141
|
Improvement of hydrophilic-lipophilic balance |
Ultrasound treatment, heat treatment, complexation with polysaccharide and/or polyphenol, and enzymatic treatments are primarily used to modify the structure of plant protein aiming to improve its functional properties. Important technological aspects and effectiveness of the methods applied to modify the plant protein are briefly outlined below.
2.1 Ultrasound treatment
Ultrasound is an acoustic wave having a frequency above hearing limit of human ear (∼20 kHz).51 Ultrasound treatment is increasingly applied to improve the functional properties of plant proteins. Ultrasound treatment has shown to improve the properties of soy protein,52,53 pea protein,54,55 and faba bean protein.56 This technology comes in two forms: high-frequency low-intensity ultrasound (HF-LIU; 100 kHz–1 MHz, power < 1 W cm−2) and low-frequency high-intensity ultrasound (LF-HIU; 16–100 kHz, power 10–1000 W cm−2).57
2.2 Complexing with polysaccharide
The modification of properties of plant proteins by complexing with polysaccharide is a classical technology. This can be classified into two methods, namely non-covalent complexation (e.g., electrostatic interaction or complex coacervation) and covalent complexation (e.g., conjugation through Maillard reaction (Fig. 3) and enzymatic crosslinking).58–60 Both complex coacervation and covalent conjugation are commonly used in food research as well as in industrial application. The non-covalent complexation (complex coacervation) is preferred in food plant protein-based formulations where mild thermal treatment is required. This method involves the interaction between two polymers with opposite charge to form a complex coacervate.61–63
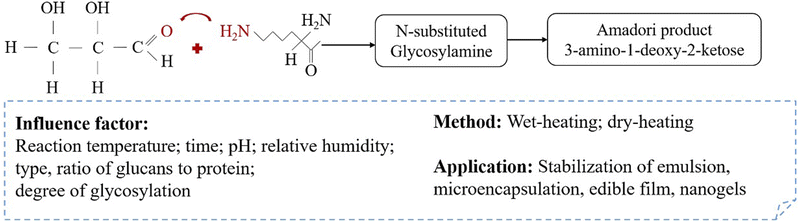 |
| Fig. 3 Schematic diagram illustrating the mechanism of early stage (glycosylation) of Maillard reaction between protein and polysaccharide. | |
2.3 Complexing with polyphenols
The improvement of functional properties of plant proteins can be achieved by complexing them with polyphenols. This process is broadly categorized into two types: non-covalent and covalent bonding. The non-covalent interactions between plant proteins and polyphenols are reversible and are mainly caused by four main types of binding interactions (hydrogen bonding, hydrophobic interaction, electrostatic interaction, and van der Waals force).64,65 Non-covalent interactions between polyphenols and proteins can occur in different pH environments through hydrophobic interactions and hydrogen bonding (Fig. 4A). Non-covalent conjugation between polyphenols and plant proteins has been proven to improve the functional properties of plant proteins. For example, the solubility and emulsifying properties of soy protein was improved by interaction with tea polyphenols through hydrophobic interactions and hydrogen bonding.66 Previous study also reported that interaction between pea protein and chlorogenic acid can improve its foaming and emulsifying properties.67 Unlike non-covalent interaction, covalent bonding is usually permanent and less prone to degradation by environmental stressors.64,68 Nowadays, the conjugation of plant proteins and polyphenols is often carried out through ultrasound assisted alkali treatment, alkali treatment, and enzymatic hydrolysis (Fig. 4B).64 Among these methods, alkali treatment is the most commonly used method due to its low cost and mild and simple conditions.69 Regardless of the method used, covalent modification with polyphenols is considered an effective way to improve functional properties (e.g. interfacial, emulsifying, antioxidant, gelling and antibacterial properties) of proteins. The conjugation between flaxseed protein and phenolic compound significantly improved its interfacial, emulsifying and antioxidant properties.70 The gelling properties and antioxidant properties of soy protein were also improved through the conjugation with tannic acid.71
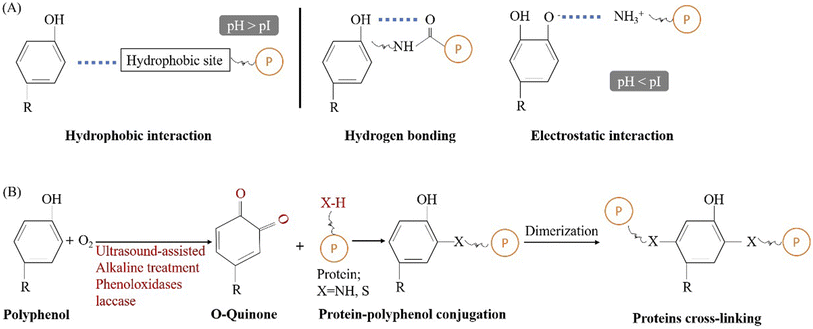 |
| Fig. 4 Schematic diagram illustrating the conjugation between protein and polyphenol. (A) Non-covalent interaction; (B) covalent binding. | |
2.4 Enzymatic treatment
Enzymatic treatments have been widely used to change the function of plant proteins by changing the protein structure particularly breaking down into desirable peptides. Enzymatic hydrolysis has been used to alter the foaming properties of soybean protein,72 surface hydrophobicity of sunflower protein,73,74 and emulsifying properties of rapeseed protein.75,76 Enzymatic modification is preferred over many other methods as it can be carried out in mild process conditions in terms of temperature and pH.77 Enzymatic hydrolysis is extensively used to tailor the function of some proteins to meet specific needs. Generally, enzymatic hydrolysis has shown to improve the solubility of plant proteins (e.g., pea protein,78 faba bean protein79); however, its impact on other functional properties such as emulsifying and foaming properties, depends on the type of protein and degree of hydrolysis. Enzymatic hydrolysis of protein also improved the foaming capacity and emulsifying activity of proteins;78,80 however, the resulting foams and emulsions usually less stable.80,81
2.5 The case of hemp protein
The application of LF-HIU (at 37–109 W cm−2 for 5–24 min) on HPI has shown to improve its solubility in water, emulsion activity index (EAI), emulsion stability index (ESI), foam capacity (FC) and foam stability (FS).82 Liu et al. (2022)38 investigated the impact of application of different power levels (200 W/400 W/600 W) and treatment time (6 min/12 min/24 min) on the technofunctional properties of HPI and optimized these two parameters (400 W/12 min). Schematic diagram on the approach followed in this part of the work is given in Fig. 5. Ultrasound can be used to alter the structure of proteins through cavitation and high shear forces. It ultimately affects the functional properties including emulsifying and foaming properties, and surface hydrophobicity of proteins.83
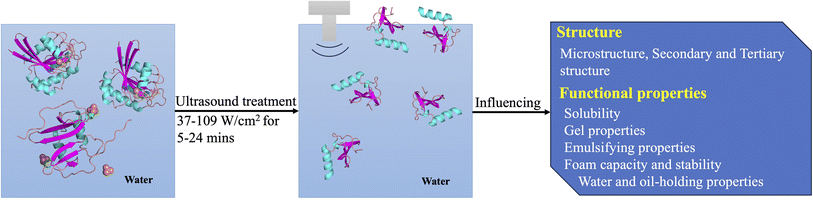 |
| Fig. 5 Schematic diagram illustrating the mechanism and effect of ultrasound treatment on hemp protein. | |
The application of complex coacervation principle on HPI-gum Arabic complex coacervates were produced and characterized.84 Recent study also provide insight into complex coacervation process between commonly available food polysaccharides (gum Arabic, sodium alginate, pectin) and hemp protein, particularly the optimum complex coacervation conditions and nature of resulting HPI-polysaccharide complex coacervates, which help broaden the application of HPI as emulsifiers and encapsulating shell materials.85,86 A schematic diagram on the approach followed in this part of the work is given in Fig. 6.
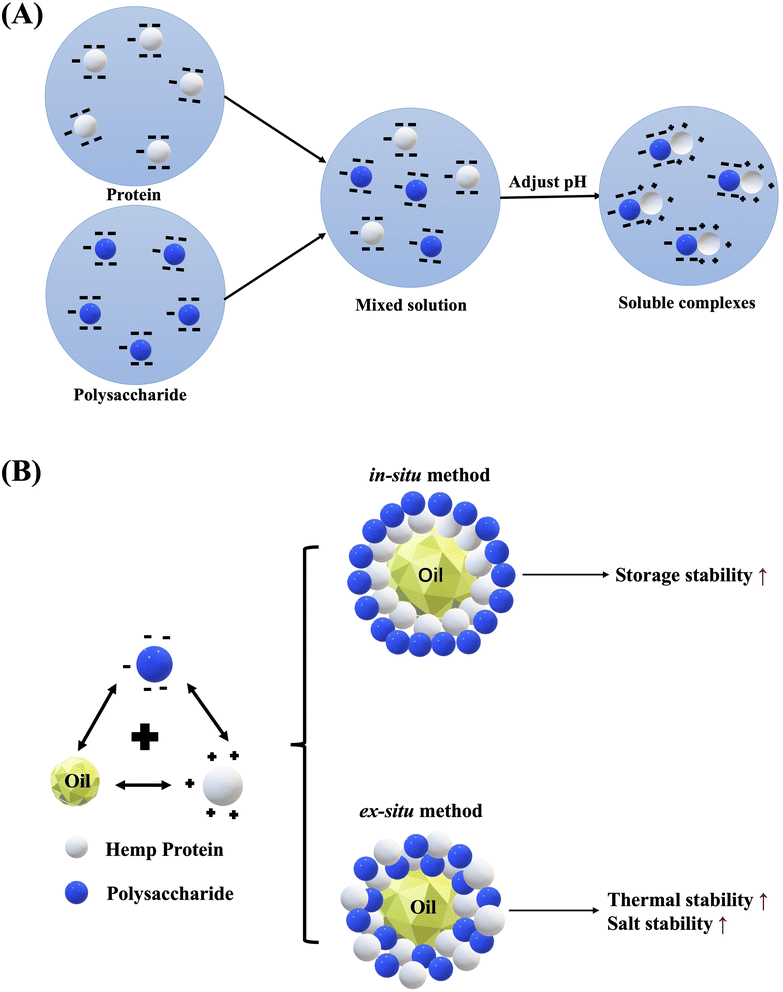 |
| Fig. 6 Schematic diagram illustrating the formation of complex coacervate between protein and polysaccharide. (A) Mechanism; (B) different formation method. | |
Liu et al. (2023)36 investigated the conjugation process of HPI with polyphenols (gallic acid and catechin) applying ultrasound-assisted alkaline method and simple alkaline method. The authors showed that this process can affect the structure and improve the emulsifying, antioxidant and antibacterial properties of HPI.
Various aspects of modification of structure–function of HPI by enzymatic hydrolysis are studied and reported.15,16,31,87,88 In addition, there is also research on the application of the conjugation of hemp peptides with polysaccharides in the field of edible films.89 The active films produced using hemp peptide-carboxymethyl chitosan showed great potential to extend the shelf life of a common perishable fruit (blue berries).89
3 Effect of modification on the structure of plant proteins
The changes in physicochemical properties brought about by the above-mentioned physicochemical and enzymatic hydrolysis methods are closely related to the changes in the molecular structure of plant proteins. The effects of these modification methods on the structure of plant proteins are briefly reviewed below.
3.1 Primary, secondary, tertiary structure and microstructure
The primary structure of a protein refers to the sequence of amino acids that make up its peptide chain. One of the most common methods of modifying the primary structure of a protein is through enzymatic hydrolysis. It has been shown that the enzymatic treatment led to a decrease in molecular weight in pea protein.78 With the increase of the hydrolysis degree (DH), the bands with lower molecular weight (17 kDa, 20–22 kDa, ∼40 kDa, ∼18 and ∼50 kDa) gradually appeared and the bands with higher molecular weight (∼60 kDa, ∼75 kDa and ∼100 kDa) disappeared due to the cleavage of protein chain.78 Similar results were observed in faba bean protein.79 The alteration of secondary structure of a protein directly impacts its functional properties.36,142 The tertiary structure of plant proteins can be determined by intrinsic fluorescence spectroscopy because the amino acid residues in the protein are sensitive to the polarity of the microenvironment.143 It has been reported that ultrasound treatment and pH-shifting can alter the tertiary structure of plant protein (e.g., pea protein,91 black bean protein144). The microstructure of a protein is often used to indicate alterations in its physical structure, including changes in its compactness, formation of aggregates, and changes in particle size. Scanning electron microscopy (SEM) is commonly used to obtain images of protein's microstructure. Ultrasound treatment has been found to alter the microstructure of many plant proteins (e.g., soy protein,53 black bean protein144).
3.2 The case of hemp protein
With regards to HPI, several enzymes have been used for this purpose including alcalase, flavourzyme, neutrase, protamex, pepsin, and trypsin, in order to break down the two subunits of edestin (45 kDa acidic subunit and 20 kDa basic subunit).15,16,31 The basic subunits are found to be more resistant to hydrolysis compared to the acidic subunits, possibly due to differences in their susceptibility.15,16 Among various enzymes, pepsin was found to be the most effective for hydrolyzing of HPI, as indicated by the release rate of soluble peptides reaching as high as 48.5%.15,16
The proportion of the secondary structural features (α-helix, β-sheet and random coil) of HPI can be altered by methods such as pH-shifting, heat treatment, ultrasound treatment, and conjugation with polyphenol. A change in the pH value can have a significant effect on the secondary structure of HPI. It was reported that when the pH of hemp protein solution was decreased to 3.0, the proportion of α-helix decreased and that of β-sheet increased. Conversely, when its pH increased to 7.0, the proportion of α-helix increased and that of β-sheet decreased.145,146 Heat treatment usually leads to a substantial increase in the proportion of β-sheet.31 On the other hand, HPI that was subjected to ultrasound treatment (37–109 W cm−2, 5–24 min) resulted into a decrease of α-helix, an increase of β-sheet, and γ-random contents due to the disruption of hydrogen bonds.53,147 These changes in secondary structure of HPI indicate the increased exposure of protein's hydrophobic region due to shearing force associated with ultrasound.148 The conjugation of protein with polyphenols (gallic acid and catechin) also affects the secondary structure of protein. It leads to an increase of γ-random by disrupting intramolecular interactions brought about by the conjugation process.36
Previous studies have shown that ultrasound treatment (37–109 W cm−2, 5–24 min) can affect the tertiary structure of HPI, leading to a higher degree of unfolding and exposure of hydrophobic groups.38,147 The combination of heat treatment (20–60 °C) and pH shifting (adjusting pH to 12.0 for a short time and then reverting to 7.0) was also found to cause a significant impact on the tertiary structure of HPI due to the loss of compact structure.96 Acetylation and succinylation also found to greatly impact on the tertiary structure of plant protein (e.g., oat protein,149 soy protein150). Both acetylation and succinylation have a significant effect on the tertiary structure of HPI and leads to unfolding of protein molecules and exposure of hydrophobic regions.151 Complexation with polyphenol is a commonly used method to alter the tertiary structure of plant protein (e.g., pea protein,67 rice protein152). Conjugation with polyphenols (gallic acid and catechin) was found to affect the tertiary structure of HPI due to disruption of internal interactions caused by the conjugation process.36 Alkaline and ultrasound-assisted alkaline conjugation between HPI and polyphenols can cause greater exposure of tryptophan residues in a polar environment, which indicates the unfolding of the protein molecule.36
The microstructure of a protein is often used to indicate alterations in its physical structure, including changes in its compactness, formation of aggregates, and changes in particle size. Scanning electron microscopy (SEM) is commonly used to obtain images of protein's microstructure. Ultrasound treatment has been found to alter the microstructure of many plant proteins (e.g., soy protein,53 black bean protein144). Similarly, ultrasound treatment (400–600 W power level for 6–24 min exposure) has also found to disrupt the compact structure of HPI and form smaller aggregates.38 This change in microstructure of protein caused by ultrasound is attributed to the cavitation and high shearing forces generated by it.38 High-pressure homogenize also lead to a significantly change of microstructure of HPI, which decreased the aggregation.105 This may related to the mechanical force formed by high-pressure homogenize to break the large aggregation.105
4 Effect of modification on the physicochemical properties of plant proteins
The change in physicochemical structure of plant proteins by the modification processes described above inevitably leads to the changes in physicochemical properties. The major changes in physicochemical properties are briefly presented below.
4.1 Free sulfhydryl content (–SH group content)
The measurement of –SH group content is commonly used to assess the level of protein unfolding and the formation of conjugates. Ultrasound treatment and phenolic conjugation have shown to impact on the –SH group content of a number of plant proteins (e.g., soy protein,153 zein154).
4.2 Free amino group
Free amino acid content is often used to evaluate changes in a protein's internal structure and to ascertain the degree of formation of conjugates. Published literature shows that both ultrasound treatment and conjugation with polyphenols have a significant impact on the free amino groups of plant proteins (e.g., flaxseed protein,70 soy protein153,155).
4.3 Particle size
Particle size is an important property that influences the functional properties of plant proteins. Previous research has shown that ultrasound treatment, high-pressure homogenization and conjugation with polyphenols could affect the particle size of plant proteins.64,105,156
4.4 Surface hydrophobicity
Surface hydrophobicity is an important functional property of plant proteins. It indicates the number of hydrophobic groups exposed to the hydrophilic medium due to alteration in tertiary structure. The surface hydrophobicity of plant proteins can be affected by ultrasound treatment,53,144 heat treatment,157,158, pH-shifting,129,159 high-pressure homogenize,105 enzymatic hydrolysis,160,161 and conjugation with polyphenols.162,163
4.5 Thermal properties
An observed change of thermal behavior of a protein indicates that it has undergone some degree of change of structure (denaturation). Thermal stability refers to a protein's ability to resist aggregation when it is exposed to heat. Research has demonstrated that ultrasound treatment and acylation (acetylation and succinylation) can enhance the thermal stability of plant proteins.151,164
4.6 The case of hemp protein
Taking hemp protein as an example, it has been observed that ultrasound treatment and polyphenol conjugation have an impact on the –SH group content of HPI. Ultrasound treatment (37 W cm−2, 8 min) significantly increased the –SH group content (1.6-fold), which is related to the shearing force of ultrasound causing the exposure of –SH groups from the interior. This may also be due to the increased surface area and reduced particle size brought about by the ultrasound treatment.147 The amount of –SH group content was found to decrease significantly after conjugation with polyphenols.36 This is most likely due to the –SH group of HPI conjugating with the hydroxyl groups of polyphenols.36
For hemp protein, conjugation of polyphenols with HPI was shown to a lower its free amino groups' content. The covalent bonding reaction between free amino acid groups of protein and the hydroxyl groups of polyphenols is considered to be responsible for this outcome.36 In addition, it was also found that the amount of free amino groups in the HPI-polyphenol conjugates produced through ultrasound-assisted alkaline treatment is significantly higher than in alkaline-induced conjugation, which was attributed to unfolding of the protein molecule and exposure of free amino groups due to forces of ultrasound.36
In the case of HPI, ultrasound treatment (with intensities ranging from 37 to 109 W cm−2 and durations of 5 to 24 min) resulted in a significant reduction of particle size due to fragmentation caused by the high shearing force.38 High-pressure homogenize (90 MPa) reduced the particle size of HPI significantly (83%).105 Similarly, the particle size of both alkali-induced and ultrasound-assisted alkaline-induced HPI-polyphenol conjugates was reduced.36
In the case of HPI, ultrasound treatment (400 W, 12 min) resulted in a significant improvement of surface hydrophobicity as its value increased by more than 2-fold. This was due to the cavitation and high shear forces associated with ultrasound, which resulted in unfolding of HPI and exposure of its hydrophobic regions.38 Heat treatment (20–60 °C) combined with pH shifting showed only a small improvement (1.0–1.2-fold) in surface hydrophobicity.96 High-pressure homogenize (90 MPa) may lead to the decrease of surface hydrophobicity due to the higher content of hydrophilic groups.105 The effect of enzymatic hydrolysis on surface hydrophobicity is reported to vary depending on the type of enzyme and treatment time. Hydrolysis of hemp protein with most enzymes showed an increase of surface hydrophobicity after 2 hours of treatment.15 However, further hydrolysis of hemp protein after 4 hours did not increase its surface hydrophobicity.15 Conjugation of hemp protein with polyphenols (gallic acid and catechin) decreased its surface hydrophobicity indicating to a reduced exposure of the hydrophobic groups.36 It is important to realize that an increase as well as decrease of hydrophobicity can affect the functional properties of HPI. Thus, it is important to identify the desirable range of surface hydrophobicity values.
In the case of HPI, ultrasound treatment increased the denaturation temperature as it caused a breakdown of intermolecular bonds of the proteins and induced changes in their structure.147 Acylation process was reported to increase the denaturation temperature of hemp protein only marginally.151 The above-mentioned improvement in thermal stability is attributed to the increased net charge on the surface of protein by acylation.151
5 Effect of modification on the techno-functional properties of plant protein: the case of hemp protein
A modification of hemp protein in its molecular and microstructural level alters its functional properties. These changes are briefly presented below.
5.1 Protein solubility
Solubility is an important functional property of proteins which impacts the extent to which they can be utilized.8,165 Studies have shown that the solubility of HPI is lower than that of other common plant proteins such as soybean and pea proteins.32,38 Low solubility of HPI, perhaps, is one of the reasons of its low utilization as an ingredient in food products, thus, needing to find ways to improve it. Solubility of proteins is influenced by their native structure and processing induced denaturation and aggregation.38,165,166 Processes that enhance the interaction between water and a protein are generally conducive to improve solubility.38 The processes that favor the formation of insoluble aggregates reduce protein's solubility. As mentioned above, various methods are applied to improve the solubility of HPI, including pH adjustment, heat treatment, pH-shifting, high-pressure homogenize, ultrasound treatment, enzymatic hydrolysis, acylation, and conjugation with polyphenols.
The solubility of proteins including HPI displays a classic U-shaped curve as a function of pH, with the lowest solubility occurring at its isoelectric point (ISP). It is reported that the ISP of hemp protein varies between pH 4.3–6.0 depending on the variety and the method of extraction used.32,41,85,146 The solubility of HPI at its ISP is around 10–15% due to the formation of insoluble aggregates, whereas at pH 9.0, its solubility reaches 75–90%, indicating that it is an alkali-soluble protein.41,146 Ultrasound treatment (20 kHz, 400 W, 12 minutes) was found to significantly improve the solubility of HPI (3.7-fold at pH = 7.0) due to cavitation-induced rupture of hydrogen and hydrophobic bonds, increased exposure of hydrophilic groups, and reduction in particle size.38 Heat treatment of HPI at 20–80 °C (at pH = 7.0) showed only a small improvement (around 1.25-fold) in its solubility.96 However, heat treatment of hemp protein at 80–100 °C for 10 min was found to decrease its solubility due to the formation of insoluble aggregates.16,44 Interestingly, a combining pH-shifting (adjustment of pH to 12.0 for up to 60 min then lowering back to 7.0) and heat treatment (up to 80 °C) was found to increase its solubility quite substantially (4.8-fold) due to the increase of repulsive electrostatic forces.96 High-pressure homogenize treatment lead to the increasing solubility (201%) of HPI due to the soluble protein aggregation.105 A limited enzymatic hydrolysis of HPI with trypsin (2.3–6.7%) was also found to significantly improve (2.8 fold, at pH 7.0) its solubility by releasing soluble peptides and increasing exposure of ionizable side groups.16 Acetylation and succinylation are found to improve solubility of hemp protein across a broad pH range (2.0–10.0).151 At pH 7.0, succinylation and acetylation resulted in a 3.3- and 2.2-fold increase in solubility, respectively.151 The improvement in solubility brought about by succinylation is due to the unfolding of protein molecules which was reported to be due to the replacement of short-range ammonium-carboxyl groups of protein with succinate carboxyl groups leading to an increase in electrostatic repulsion.151 For acetylation, the improvement in solubility is due to the unfolding of protein molecules and a reduction in protein–protein interaction and an increase in protein–water interaction.151 Conjugating HPI with polyphenols through alkaline and ultrasound-assisted alkaline treatment methods has also been found to significantly improve (about 2-fold) solubility by decreasing surface hydrophobicity and particle size.36 Thus, a combination of pH-shifting and heat treatment can be considered as a rational approach to improve the solubility of HPI.
The methods used to improve the solubility of proteins including hemp protein should be carefully considered. If ultrasound treatment is chosen, it is important to be mindful of the power and duration of the treatment as overly intense power–time combination can reduce the solubility.38,82 In the case of acetylation, it is important to control the anhydride level as levels higher than 0.2 g g−1 can decrease solubility instead of increasing.151
5.2 Emulsifying properties
Emulsions are essential part of many food products including milk, beverage, ice cream, butter, and sausage.167 Protein acts as the natural ingredient in food emulsions due to its amphiphilic nature and ability to form interfacial films at oil–water interface.168 The emulsifying properties of proteins are determined by the interfacial properties, functional properties (e.g., solubility) and molecular structure (e.g. flexibility, rigidity).169 Surface hydrophobicity affects the ability of proteins to adsorb at the oil–water interface, and solubility affects the rate with which protein molecules can migrate to the oil–water interface.36,38 HPI has weaker emulsifying properties compared to common plant proteins.32,38 Therefore, it is essential to improve its emulsifying properties to broaden its application in food industry. Emulsifying properties are commonly measured by using two parameters: emulsifying activity (EAI) and emulsifying stability (ESI). The physical stability of emulsion depends on factors such as storage time, temperature, and ionic strength.
Various methods are developed to improve the emulsifying properties of HPI, including ultrasound treatment, heat treatment, pH-shifting, acylation (acetylation and succinylation), and complexing with polysaccharides and polyphenols. It is reported that the ultrasound treatment at suitable power level and exposure time can significantly improve both EAI and ESI. The improvements in these parameters are brought about by the improved solubility, controlled unfolding, and an increase in the surface hydrophobicity. When molecular structure of protein becomes more flexible, it enhances the protein's ability to adsorb at the oil–water interface.38,82 Heat treatment by itself can only minimally affect EAI; however, when combined with pH shifting, it can significantly improve EAI as well as emulsion stability due to increased unfolding of structure and subsequent exposure of hydrophobic amino acid side chains.96
Acylation (acetylation and succinylation) can bring about substantial increase in EAI. Acetylation can significantly increase the EAI.151 It was reported that at 70% succinylation, a 4.5-fold increase of EAI was achieved in HPI. However, at the same degree of acetylation of HPI only yielded 1.8-fold increase in EAI.151 The authors attributed this improvement to controlled unfolding of protein structure and partial exposure of hydrophobic region. Complexing with polysaccharides can improve the ability of HPI to stabilize emulsions.85,168 Similarly, conjugation of HPI with polyphenols (such as gallic acid) also resulted in substantial increased in EAI and ESI.36 This improvement was brought about by the increased negative charge density, smaller particle size and higher surface hydrophobicity.36
Therefore, two of the rationale ways to improve both EAI and ESI of hemp protein are to complex it with polysaccharides and conjugate with polyphenols after preliminarily subjecting it to ultrasound treatment. As mentioned previously, both the ionic complex coacervation and covalent conjugation processes require optimization.
5.3 Water and oil holding capacities
The ability of proteins to retain water is known as water holding capacity (WHC). It is an important property to consider when producing protein-based gels. A suitably controlled WHC contributes to the quality of food by preventing water loss, preserving freshness, providing a moist mouthfeel, and ensuring ideal texture.170,171 The oil holding capacity (OHC) of a protein indicates to its ability to absorb/retain oil or lipid.8 Currently, there is a paucity of study aiming to determine the effect of various treatments on the WHC and OHC of HPI. It has, nevertheless, shown that ultrasound treatment could improve these properties only marginally.82
5.4 Gelling properties
The ability of plant proteins to form gel is important for products such as tofu, plant-based meat and dairy substitutes.172,173 Additionally, protein-based gels can also be used to encapsulate unstable compounds such as vitamin E and β-carotene, to enhance their stability.174–176 Gelation of proteins can be achieved by using heating-cooling, acid and salt treatment, cross-linking agents (such as transglutaminase, polysaccharides, and polyphenols) and microbial fermentation.177–181 The gelling properties of proteins are influenced by changes in their physicochemical properties and functional properties, such as particle size, molecular structure, surface hydrophobicity and state of intermolecular interactions.182 In principle, any method capable of enhancing interaction between protein molecules can improve the gelling properties. The gelling properties of HPI are known to be inferior compared to many common plant proteins. For example, the least gelation concentration of HPI is around 22% which is substantially higher than that of many other plant proteins.145 A number of processes can be applied to improve the gel characteristics of HPI including ultrasound and salt treatments and mixing with other proteins. Ultrasound treatment is reported to significantly improve gelling capacity by reducing the minimum gelling concentration from 22% to 18%, primarily increasing its solubility and structural flexibility.82 For heat-induced gel, changes in NaCl concentration of the HPI dispersions had a greater impact on the rheological properties and microstructure.183 It is reported that salt treatment led to a finer gel network structure due to a charge-shielding effect.183 Studies have found that the gel properties of HPI can be significantly improved by mixing it with other proteins, for example, egg and lentil proteins.184,185 Improvements in gelling properties of HPI can broaden its application.
5.5 Foaming capacity and stability
The ability of a protein to form foam (foaming capacity, FC) and its ability to maintain the foam (foam stability, FS) are important for production of various protein containing foods. FS is a key quality indicator for certain food products such as ice cream.186 In general, the FC of a protein can be enhanced by reducing surface tension. Proteins which are difficult to denature at the air–water interface tend to have a poor FC.187 The FC and FS of a protein depend on various factors such as the speed and amount proteins adsorbed at the air–water interface, speed and extent of change of conformation, and orientation and rearrangement at the interface.156,188 The formation of cohesive viscoelastic film at the air–water interface through protein–protein interactions is important for formation and stability of protein foams.72 Other factors such as concentration, solubility, and surface hydrophobicity also influence protein's foaming ability.169 Currently, there is a dearth of study on the impact of processing methods on the foaming properties of HPI. The application of ultrasound has shown to improve both FC and FS of HPI which is attributed to the partial denaturation and the exposure of hydrophobic regions.82
6 Conclusion
A number of processing methods are studied and applied to improve the functional properties of plant proteins including hemp protein with varying degree of success. The most studied methods include ultrasound treatment, heat treatment, pH shifting, acylation, enzymatic hydrolysis, complex coacervation with polysaccharides, and conjugation with polyphenols. Of these methods, ultrasound treatment appears to be the most effective physical method that can be applied to improve the technofunctional properties of HPI. The pH shifting and heat treatment also appear to improve the technofunctional properties of HPI, particularly its solubility. Acylation also appears to improve the solubility and emulsifying properties. Enzymatic hydrolysis appears to improve the solubility of hemp proteins at the same time it can negatively affect emulsifying and foaming ability, and water and oil holding capacity. Formation of complex coacervate of HPI with polysaccharides are shown to improve the physical stability of emulsions. Conjugation of HPI with polyphenols also reported to significantly improve the solubility, emulsifying ability, and physical stability of emulsion. However, research on improving the functional properties of HPI is still not mainstream and appears to be focused on limited to selective properties and appears to lack comprehensiveness. This review indicates that there is need for further research and theoretical support for the application of protein–polysaccharide complex coacervation and protein–polyphenol conjugation methods for improving the functional properties of hemp protein as they have greater potential. The research and innovation of HPI-polysaccharide complex coacervates and HPI-polyphenol conjugates and hemp peptide–polysaccharide conjugates in food products needs to be prioritized to broaden the application of these ingredients into commercially produced food products.
Data availability
The data that support the findings of this study can be made available upon request.
Author contributions
Xinye Liu: conceptualization, analysis and writing drafts; Feng Xue: analysis, review and providing scholarly input; Benu Adhikari: conceptualization, revising, providing scholarly input.
Conflicts of interest
The authors have no conflict of interest to declare.
Acknowledgements
The authors acknowledge the library and database support from RMIT University and Nanjing University of Chinese Medicine.
References
-
P. Bouloc, S. Allegret and L. Arnaud, Hemp: Industrial Production and Uses, CABI, 2013 Search PubMed.
- I. Adesina, A. Bhowmik, H. Sharma and A. Shahbazi, Agriculture, 2020, 10, 129 CrossRef CAS.
- E. B. Russo, Chem. Biodiversity, 2007, 4, 1614–1648 CrossRef CAS PubMed.
- J. H. Cherney and E. Small, Agronomy, 2016, 6, 58 CrossRef.
- W. Leonard, P. Zhang, D. Ying and Z. Fang, Compr. Rev. Food Sci. Food Saf., 2020, 19, 282–308 CrossRef PubMed.
- P. Cerino, C. Buonerba, G. Cannazza, J. D'Auria, E. Ottoni, A. Fulgione, A. Di Stasio, B. Pierri and A. Gallo, Cannabis Cannabinoid Res., 2020, 6, 19–27 CrossRef PubMed.
- G. Crini, E. Lichtfouse, G. Chanet and N. Morin-Crini, Environ. Chem. Lett., 2020, 18, 1451–1476 CrossRef CAS.
- P. Shen, Z. Gao, B. Fang, J. Rao and B. Chen, Trends Food Sci. Technol., 2021, 112, 1–15 CrossRef CAS.
-
A. De Candolle, Origine des plantes cultivées, G. Baillière et cie, 1883 Search PubMed.
- X. Lu and R. C. Clarke, J. Ind. Hemp, 1995, 2, 26–30 Search PubMed.
-
S. Amaducci and H. J. Gusovius, Industrial Applications of Natural Fibres: Structure, Properties and Technical Applications, 2010, pp. 109–134 Search PubMed.
-
N. Morin-Crini, S. Loiacono, V. Placet, G. Torri, C. Bradu, M. Kostić, C. Cosentino, G. Chanet, B. Martel, E. Lichtfouse and G. Crini, in Green Adsorbents for Pollutant Removal: Innovative materials, ed. G. Crini and E. Lichtfouse, Springer International Publishing, Cham, 2018, pp. 1–34, DOI:10.1007/978-3-319-92162-4_1.
- N. Morin-Crini, S. Loiacono, V. Placet, G. Torri, C. Bradu, M. Kostić, C. Cosentino, G. Chanet, B. Martel, E. Lichtfouse and G. Crini, Environ. Chem. Lett., 2019, 17, 393–408 CrossRef CAS.
- J. D. House, J. Neufeld and G. Leson, J. Agric. Food Chem., 2010, 58, 11801–11807 CrossRef CAS PubMed.
- C.-H. Tang, X.-S. Wang and X.-Q. Yang, Food Chem., 2009, 114, 1484–1490 CrossRef CAS.
- S.-W. Yin, C.-H. Tang, J.-S. Cao, E.-K. Hu, Q.-B. Wen and X.-Q. Yang, Food Chem., 2008, 106, 1004–1013 CrossRef CAS.
- J. C. Callaway, Euphytica, 2004, 140, 65–72 CrossRef.
- A. Pihlanto, P. Mattila, S. Mäkinen and A. M. Pajari, Food Funct., 2017, 8, 3443–3458 RSC.
- D. Rodriguez-Leyva and G. N. Pierce, Nutr. Metab., 2010, 7, 32 CrossRef PubMed.
- S.-S. Teh, A. E.-D. A. Bekhit, A. Carne and J. Birch, Food Chem., 2016, 203, 199–206 CrossRef CAS PubMed.
- G. C. Román, R. E. Jackson, R. Gadhia, A. N. Román and J. Reis, Rev. Neurol., 2019, 175, 724–741 CrossRef PubMed.
- S.-S. Teh, A. E.-D. Bekhit, A. Carne and J. Birch, J. Food Meas. Charact., 2014, 8, 92–104 CrossRef.
- B. Wang, D. Teng, C. Yu, L. Yao, X. Ma and T. Wu, Front. Plant Sci., 2022, 13, 828153 CrossRef PubMed.
- M. G. Nosworthy, A. J. Franczyk, G. Medina, J. Neufeld, P. Appah, A. Utioh, P. Frohlich and J. D. House, J. Agric. Food Chem., 2017, 65, 7790–7796 CrossRef CAS PubMed.
- A. A. Alaswad, B. Song, N. W. Oehrle, W. J. Wiebold, T. P. Mawhinney and H. B. Krishnan, Plant Sci., 2021, 308, 110912 CrossRef CAS PubMed.
- J. D. House, K. Hill, J. Neufeld, A. Franczyk and M. G. Nosworthy, Food Sci. Nutr., 2019, 7, 2932–2938 CrossRef CAS PubMed.
- M. Rafii, P. B. Pencharz, R. O. Ball, C. Tomlinson, R. Elango and G. Courtney-Martin, J. Nutr., 2020, 150, 1834–1844 CrossRef PubMed.
- A. T. Girgih, R. He, S. Malomo, M. Offengenden, J. Wu and R. E. Aluko, J. Funct. Foods, 2014, 6, 384–394 CrossRef CAS.
- X.-S. Wang, C.-H. Tang, X.-Q. Yang and W.-R. Gao, Food Chem., 2008, 107, 11–18 CrossRef CAS.
- G. Mamone, G. Picariello, A. Ramondo, M. A. Nicolai and P. Ferranti, Food Res. Int., 2019, 115, 562–571 CrossRef CAS PubMed.
- S. Wang, J. Wang, F. Xue and C. Li, J. Food Sci. Technol., 2019, 56, 3337–3346 CrossRef CAS PubMed.
- C.-H. Tang, Z. Ten, X.-S. Wang and X.-Q. Yang, J. Agric. Food Chem., 2006, 54, 8945–8950 CrossRef CAS PubMed.
- I. Zahari, F. Ferawati, A. Helstad, C. Ahlström, K. Östbring, M. Rayner and J. K. Purhagen, Foods, 2020, 9, 772 CrossRef CAS PubMed.
- S.-W. Yin, C.-H. Tang, Q.-B. Wen and X.-Q. Yang, J. Agric. Food Chem., 2007, 55, 7399–7404 CrossRef CAS PubMed.
- J. Korus, M. Witczak, R. Ziobro and L. Juszczak, LWT, 2017, 84, 143–150 CrossRef CAS.
- X. Liu, F. Xue and B. Adhikari, Future Foods, 2023, 7, 100210 CrossRef CAS.
- F. Potin, E. Goure, S. Lubbers, F. Husson and R. Saurel, Int. J. Food Sci. Technol., 2022, 57, 436–446 CrossRef CAS.
- X. Liu, M. Wang, F. Xue and B. Adhikari, Future Foods, 2022, 6, 100176 CrossRef CAS.
- B. Fang, L. Chang, J.-B. Ohm, B. Chen and J. Rao, Food Chem., 2023, 405, 135001 CrossRef CAS PubMed.
- S. Odani and S. Odani, Biosci., Biotechnol., Biochem., 1998, 62, 650–654 CrossRef CAS PubMed.
- M. Hadnađev, T. Dapčević-Hadnađev, A. Lazaridou, T. Moschakis, A. M. Michaelidou, S. Popović and C. G. Biliaderis, Food Hydrocolloids, 2018, 79, 526–533 CrossRef.
- S. Patel, R. Cudney and A. McPherson, J. Mol. Biol., 1994, 235, 361–363 CrossRef CAS PubMed.
- S. A. Malomo and R. E. Aluko, Food Hydrocolloids, 2015, 43, 743–752 CrossRef CAS.
- V. Raikos, G. Duthie and V. Ranawana, Plant Foods Hum. Nutr., 2015, 70, 304–309 CrossRef CAS PubMed.
- F. U. Akharume, R. E. Aluko and A. A. Adedeji, Compr. Rev. Food Sci. Food Saf., 2021, 20, 198–224 CrossRef CAS PubMed.
- M. E. M. Braga, M. C. Gaspar and H. C. de Sousa, Curr. Opin. Food Sci., 2023, 50, 100983 CrossRef CAS.
- R. N. Arshad, Z. Abdul-Malek, U. Roobab, M. A. Munir, A. Naderipour, M. I. Qureshi, A. El-Din Bekhit, Z.-W. Liu and R. M. Aadil, Trends Food Sci. Technol., 2021, 111, 43–54 CrossRef CAS.
- G. G. Flores-Rojas, F. López-Saucedo and E. Bucio, Radiat. Phys. Chem., 2020, 169, 107962 CrossRef CAS.
- Z. Zhong, X. Wang, X. Yin, J. Tian and S. Komatsu, Int. J. Mol. Sci., 2021, 22, 12239 CrossRef CAS PubMed.
- M. Gavahian and A. M. Khaneghah, Crit. Rev. Food Sci. Nutr., 2020, 60, 1581–1592 CrossRef CAS PubMed.
- T. S. Awad, H. A. Moharram, O. E. Shaltout, D. Asker and M. M. Youssef, Food Res. Int., 2012, 48, 410–427 CrossRef CAS.
- R. Morales, K. D. Martínez, V. M. Pizones Ruiz-Henestrosa and A. M. R. Pilosof, Ultrason. Sonochem., 2015, 26, 48–55 CrossRef CAS PubMed.
- H. Hu, J. Wu, E. C. Y. Li-Chan, L. Zhu, F. Zhang, X. Xu, G. Fan, L. Wang, X. Huang and S. Pan, Food Hydrocolloids, 2013, 30, 647–655 CrossRef CAS.
- K. Gao, F. Zha, Z. Yang, J. Rao and B. Chen, Food Hydrocolloids, 2022, 125, 107409 CrossRef CAS.
- R. Mozafarpour, A. Koocheki and T. Nicolai, Food Res. Int., 2022, 158, 111520 CrossRef CAS PubMed.
- F. Alavi, L. Chen and Z. Emam-Djomeh, Food Chem., 2021, 354, 129494 CrossRef CAS PubMed.
- H. Hu, I. W. Y. Cheung, S. Pan and E. C. Y. Li-Chan, Food Hydrocolloids, 2015, 45, 102–110 CrossRef CAS.
- H. Chen, J. Gan, A. Ji, S. Song and L. Yin, Food Chem., 2019, 292, 188–196 CrossRef CAS PubMed.
- X. Kan, G. Chen, W. Zhou and X. Zeng, Food Res. Int., 2021, 150, 110740 CrossRef CAS PubMed.
- Y. Lan, J.-B. Ohm, B. Chen and J. Rao, Food Chem., 2020, 307, 125536 CrossRef CAS PubMed.
- B. Muhoza, B. Qi, J. D. Harindintwali, M. Y. Farag Koko, S. Zhang and Y. Li, Food Hydrocolloids, 2022, 124, 107239 CrossRef CAS.
- L. Qiu, M. Zhang, B. Adhikari and L. Chang, Food Hydrocolloids, 2022, 124, 107366 CrossRef CAS.
- Y. P. Timilsena, T. O. Akanbi, N. Khalid, B. Adhikari and C. J. Barrow, Int. J. Biol. Macromol., 2019, 121, 1276–1286 CrossRef CAS PubMed.
- X. Yan, Z. Zeng, D. J. McClements, X. Gong, P. Yu, J. Xia and D. Gong, Compr. Rev. Food Sci. Food Saf., 2023, 22, 1312–1336 CrossRef CAS PubMed.
- T. Ozdal, E. Capanoglu and F. Altay, Food Res. Int., 2013, 51, 954–970 CrossRef CAS.
- G. Chen, S. Wang, B. Feng, B. Jiang and M. Miao, Food Chem., 2019, 277, 632–638 CrossRef CAS PubMed.
- L. Hao, J. Sun, M. Pei, G. Zhang, C. Li, C. Li, X. Ma, S. He and L. Liu, Food Chem., 2022, 383, 132623 CrossRef CAS PubMed.
- X. Sun, R. A. Sarteshnizi and C. C. Udenigwe, Curr. Opin. Food Sci., 2022, 45, 100840 CrossRef CAS.
- H. Jing, J. Sun, Y. Mu, M. Obadi, D. J. McClements and B. Xu, Food Funct., 2020, 11, 7084–7094 RSC.
- L. B. Pham, B. Wang, B. Zisu and B. Adhikari, Food Hydrocolloids, 2019, 94, 20–29 CrossRef CAS.
- Y. Guo, Y.-h. Bao, K.-f. Sun, C. Chang and W.-f. Liu, Food Hydrocolloids, 2021, 112, 106293 CrossRef CAS.
- G. Liang, W. Chen, X. Qie, M. Zeng, F. Qin, Z. He and J. Chen, Food Hydrocolloids, 2020, 105, 105764 CrossRef.
- S. Beaubier, S. Albe-Slabi, A. Aymes, M. Bianeis, O. Galet and R. Kapel, Foods, 2021, 10, 664 CrossRef CAS PubMed.
- R. Kaur and G. Ghoshal, Adv. Colloid Interface Sci., 2022, 306, 102725 CrossRef CAS PubMed.
- S. Beaubier, C. Pineda-Vadillo, O. Mesieres, X. Framboisier, O. Galet and R. Kapel, Food Chem., 2023, 407, 135132 CrossRef CAS PubMed.
- P. Wongsirichot, M. Gonzalez-Miquel and J. Winterburn, Biochem. Eng. J., 2022, 180, 108373 CrossRef CAS.
- A. Mannheim and M. Cheryan, J. Am. Oil Chem. Soc., 1992, 69, 1163–1169 CrossRef CAS.
- X. Shuai, L. Gao, Q. Geng, T. Li, X. He, J. Chen, C. Liu and T. Dai, Foods, 2022, 11, 2368 CrossRef CAS PubMed.
- M. A. Nawaz, R. Buckow, H. Jegasothy and R. Stockmann, Food Bioprod. Process., 2022, 132, 200–210 CrossRef CAS.
- M. d. M. Yust, J. Pedroche, M. d. C. Millán-Linares, J. M. Alcaide-Hidalgo and F. Millán, Food Chem., 2010, 122, 1212–1217 CrossRef CAS.
- A. Mokni Ghribi, I. Maklouf Gafsi, A. Sila, C. Blecker, S. Danthine, H. Attia, A. Bougatef and S. Besbes, Food Chem., 2015, 187, 322–330 CrossRef CAS PubMed.
- G. Karabulut and O. Yemiş, Food Chem., 2022, 375, 131843 CrossRef CAS PubMed.
- J. Su and A. Cavaco-Paulo, Ultrason. Sonochem., 2021, 76, 105653 CrossRef CAS PubMed.
- F. Plati, C. Ritzoulis, E. Pavlidou and A. Paraskevopoulou, Int. J. Biol. Macromol., 2021, 182, 144–153 CrossRef CAS PubMed.
- X. Liu, F. Xue and B. Adhikari, Food Hydrocolloids, 2023, 137, 108352 CrossRef CAS.
- X. Liu, F. Xue and B. Adhikari, Sustainable Food Technol., 2023, 1, 426–436 RSC.
-
R. E. Aluko, in Sustainable Protein Sources, ed. S. R. Nadathur, J. P. D. Wanasundara and L. Scanlin, Academic Press, San Diego, 2017, pp. 121–132, DOI:10.1016/B978-0-12-802778-3.00007-X.
- S. A. Malomo, J. O. Onuh, A. T. Girgih and R. E. Aluko, Nutrients, 2015, 7, 7616–7632 CrossRef CAS PubMed.
- X. Liu, F. Xue, C. Li and B. Adhikari, Int. J. Biol. Macromol., 2022, 202, 26–36 CrossRef CAS PubMed.
- L. Li, Y. Zhou, F. Teng, S. Zhang, B. Qi, C. Wu, T. Tian, Z. Wang and Y. Li, Int. J. Food Sci. Technol., 2020, 55, 1637–1647 CrossRef CAS.
- T. Xiong, W. Xiong, M. Ge, J. Xia, B. Li and Y. Chen, Food Res. Int., 2018, 109, 260–267 CrossRef CAS PubMed.
- S. Yan, J. Xu, S. Zhang and Y. Li, LWT, 2021, 142, 110881 CrossRef CAS.
- B. Xu, S. M. R. Azam, M. Feng, B. Wu, W. Yan, C. Zhou and H. Ma, Ultrason. Sonochem., 2021, 81, 105855 CrossRef CAS PubMed.
- W. Peng, X. Kong, Y. Chen, C. Zhang, Y. Yang and Y. Hua, Food Hydrocolloids, 2016, 52, 301–310 CrossRef CAS.
- M. Zhao, W. Xiong, B. Chen, J. Zhu and L. Wang, Food Hydrocolloids, 2020, 103, 105626 CrossRef CAS.
- Q. Wang, Y. Jin and Y. L. Xiong, J. Agric. Food Chem., 2018, 66, 10827–10834 CrossRef CAS PubMed.
- A. K. Buggy, J. J. McManus, A. Brodkorb, S. A. Hogan and M. A. Fenelon, J. Dairy Sci., 2018, 101, 10819–10830 CrossRef CAS PubMed.
- C. M. Kelleher, J. T. Tobin, J. A. O'Mahony, A. L. Kelly, D. J. O'Callaghan and N. A. McCarthy, Innovative Food Sci. Emerging Technol., 2019, 52, 282–290 CrossRef CAS.
- N. Al-Ruwaih, J. Ahmed, M. F. Mulla and Y. A. Arfat, LWT, 2019, 100, 231–236 CrossRef CAS.
- Z.-K. Zhao, T.-H. Mu, M. Zhang and A. Richel, Food Bioprocess Technol., 2019, 12, 1863–1873 CrossRef CAS.
- L. Piccini, A. Scilingo and F. Speroni, Food Biophys., 2019, 14, 69–79 CrossRef.
- D. Liu, L. Zhang, Y. Wang, Z. Li, Z. Wang and J. Han, Food Chem., 2020, 333, 127530 CrossRef CAS PubMed.
- F. T. Saricaoglu, Int. J. Biol. Macromol., 2020, 144, 760–769 CrossRef CAS PubMed.
-
P. Rovere, in Ultra High Pressure Treatments of Foods, eds. M. E. G. Hendrickx, D. Knorr, L. Ludikhuyze, A. Van Loey and V. Heinz, Springer US, Boston, MA, 2001, pp. 251–268, DOI:10.1007/978-1-4615-0723-9_9.
- X. Zhao, X. Liu and F. Xue, Appl. Food Res., 2023, 3(1), 100285 CrossRef CAS.
- S. Melchior, S. Calligaris, G. Bisson and L. Manzocco, Food Bioprocess Technol., 2020, 13, 2145–2155 CrossRef CAS.
- S. Zhang, L. Sun, H. Ju, Z. Bao, X.-A. Zeng and S. Lin, Food Res. Int., 2021, 139, 109914 CrossRef CAS PubMed.
- S. Samard and G.-H. Ryu, J. Food Process. Preserv., 2019, 43, e14123 CAS.
- O. K. Mosibo, G. Ferrentino, M. R. Alam, K. Morozova and M. Scampicchio, Crit. Rev. Food Sci. Nutr., 2022, 62, 2526–2547 CrossRef PubMed.
- S. Zhang, W. Huang, E. Feizollahi, M. S. Roopesh and L. Chen, Innovative Food Sci. Emerging Technol., 2021, 67, 102567 CrossRef CAS.
- S. Chaple, C. Sarangapani, J. Jones, E. Carey, L. Causeret, A. Genson, B. Duffy and P. Bourke, Innovative Food Sci. Emerging Technol., 2020, 66, 102529 CrossRef CAS.
- S. Zhang, W. Huang, M. S. Roopesh and L. Chen, Food Res. Int., 2022, 154, 111028 CrossRef CAS PubMed.
- A. R. Ganesan, U. Tiwari, P. N. Ezhilarasi and G. Rajauria, J. Food Process. Preserv., 2021, 45, e15070 CAS.
- Y. Wang, A. Zhang, Y. Wang, X. Wang, N. Xu and L. Jiang, Food Funct., 2020, 11, 1635–1646 RSC.
- M. A. Malik, H. K. Sharma and C. S. Saini, Food Hydrocolloids, 2017, 72, 312–322 CrossRef CAS.
- A. B. Hassan, N. S. Mahmoud, K. Elmamoun, O. Q. Adiamo and I. A. Mohamed Ahmed, Radiat. Phys. Chem., 2018, 144, 85–91 CrossRef CAS.
- Y.-F. Liu, I. Oey, P. Bremer, A. Carne and P. Silcock, Compr. Rev. Food Sci. Food Saf., 2019, 18, 986–1002 CrossRef CAS PubMed.
- C. Wen, J. Zhang, W. Qin, J. Gu, H. Zhang, Y. Duan and H. Ma, Food Chem., 2020, 331, 127374 CrossRef CAS PubMed.
- Z.-Z. Xu, G.-Q. Huang, T.-C. Xu, L.-N. Liu and J.-X. Xiao, Int. J. Biol. Macromol., 2019, 131, 601–607 CrossRef CAS PubMed.
- F. Zha, S. Dong, J. Rao and B. Chen, Food Hydrocolloids, 2019, 92, 30–40 CrossRef CAS.
- S. Caballero and G. Davidov-Pardo, Food Chem., 2021, 338, 128083 CrossRef CAS PubMed.
- I. Kutzli, D. Griener, M. Gibis, L. Grossmann, S. K. Baier and J. Weiss, Food Funct., 2020, 11, 4049–4056 RSC.
- H. Jin, Q. Zhao, H. Feng, Y. Wang, J. Wang, Y. Liu, D. Han and J. Xu, Polymers, 2019, 11, 848 CrossRef CAS PubMed.
- F. Alavi, L. Chen, Z. Wang and Z. Emam-Djomeh, Food Hydrocolloids, 2021, 112, 106335 CrossRef CAS.
- H. Ji, X. Tang, L. Li, S. Peng, C. Gao and Y. Chen, Food Hydrocolloids, 2020, 109, 106124 CrossRef CAS.
- A. P. H. de Oliveira, M. H. Omura, É. d. A. A. Barbosa, G. C. Bressan, É. N. R. Vieira, J. S. d. R. Coimbra and E. B. de Oliveira, Colloids Surf., A, 2020, 603, 125156 CrossRef CAS.
- O. Kahraman, G. E. Petersen and C. Fields, Foods, 2022, 11, 587 CrossRef CAS PubMed.
- Y. Li, Y. Cheng, Z. Zhang, Y. Wang, B. K. Mintah, M. Dabbour, H. Jiang, R. He and H. Ma, Ultrason. Sonochem., 2020, 69, 105240 CrossRef CAS PubMed.
- Y. Wang, S. Wang, R. Li, Y. Wang, Q. Xiang, K. Li and Y. Bai, Food Hydrocolloids, 2022, 124, 107351 CrossRef CAS.
- Y. Shen and Y. Li, Food Hydrocolloids, 2021, 117, 106686 CrossRef CAS.
- Z. Lian, S. Yang, S. Dai, X. Tong, P. Liao, L. Cheng, W. Qi, Y. Wang, H. Wang and L. Jiang, J. Sci. Food Agric., 2022, 102, 6454–6463 CrossRef CAS PubMed.
- Y. Li, Z. Zhang and A. Abbaspourrad, Food Hydrocolloids, 2022, 133, 107994 CrossRef CAS.
- Y. Jiang, Z. Wang, Z. He, M. Zeng, F. Qin and J. Chen, LWT, 2022, 154, 112328 CrossRef CAS.
- L. Fang, H. Xiang, D. Sun-Waterhouse, C. Cui and J. Lin, J. Agric. Food Chem., 2020, 68, 1691–1697 CrossRef CAS PubMed.
- S. Meenmanee, A. Rattananukrom, S. Thaiphanit and I. Suppavorasatit, LWT, 2022, 153, 112493 CrossRef CAS.
- M. Hadidi, S. Jafarzadeh and A. Ibarz, LWT, 2021, 151, 112119 CrossRef CAS.
- E. Eckert, J. Han, K. Swallow, Z. Tian, M. Jarpa-Parra and L. Chen, Cereal Chem., 2019, 96, 725–741 CrossRef CAS.
- V. García Arteaga, M. Apéstegui Guardia, I. Muranyi, P. Eisner and U. Schweiggert-Weisz, Innovative Food Sci. Emerging Technol., 2020, 65, 102449 CrossRef.
- X. Zang, C. Yue, Y. Wang, M. Shao and G. Yu, J. Cereal Sci., 2019, 85, 168–174 CrossRef CAS.
- A. Fasim, V. S. More and S. S. More, Curr. Opin. Biotechnol., 2021, 69, 68–76 CrossRef CAS PubMed.
- V. García Arteaga, S. Leffler, I. Muranyi, P. Eisner and U. Schweiggert-Weisz, Curr. Res. Food Sci., 2021, 4, 1–10 CrossRef PubMed.
- Z. Gao, P. Shen, Y. Lan, L. Cui, J.-B. Ohm, B. Chen and J. Rao, Food Res. Int., 2020, 131, 109045 CrossRef CAS PubMed.
- A. Vera, M. A. Valenzuela, M. Yazdani-Pedram, C. Tapia and L. Abugoch, Ultrason. Sonochem., 2019, 51, 186–196 CrossRef CAS PubMed.
- L. Jiang, J. Wang, Y. Li, Z. Wang, J. Liang, R. Wang, Y. Chen, W. Ma, B. Qi and M. Zhang, Food Res. Int., 2014, 62, 595–601 CrossRef CAS.
- S. A. Malomo, R. He and R. E. Aluko, J. Food Sci., 2014, 79, C1512–C1521 CrossRef CAS PubMed.
- P. Shen, Z. Gao, M. Xu, J. Rao and B. Chen, Food Hydrocolloids, 2020, 108, 106065 CrossRef CAS.
- G. Karabulut, H. Feng and O. Yemiş, Plant Foods Hum. Nutr., 2022, 77, 577–583 CrossRef CAS PubMed.
- W. Sun, F. Zhou, D.-W. Sun and M. Zhao, Food Bioprocess Technol., 2013, 6, 1703–1712 CrossRef CAS.
- C.-B. Zhao, H. Zhang, X.-Y. Xu, Y. Cao, M.-Z. Zheng, J.-S. Liu and F. Wu, Process Biochem., 2017, 57, 117–123 CrossRef CAS.
- M. He, L. Li, C. Wu, L. Zheng, L. Jiang, Y. Huang, F. Teng and Y. Li, J. Food Sci., 2021, 86, 1737–1750 CrossRef CAS PubMed.
- S.-W. Yin, C.-H. Tang, Q.-B. Wen and X.-Q. Yang, Int. J. Food Sci. Technol., 2009, 44, 2653–2661 CrossRef CAS.
- X. Jia, M. Zhao, N. Xia, J. Teng, C. Jia, B. Wei, L. Huang and D. Chen, J. Cereal Sci., 2019, 89, 102818 CrossRef CAS.
- S. Yan, F. Xie, S. Zhang, L. Jiang, B. Qi and Y. Li, Colloids Surf., A, 2021, 609, 125641 CrossRef CAS.
- G. Ren, J. Shi, S. Huang, C. Liu, F. Ni, Y. He, X. Luo, T. Li, Y. Song, M. Huang and H. Xie, Food Chem., 2022, 374, 131612 CrossRef CAS PubMed.
- M. Geng, X. Feng, H. Yang, X. Wu, L. Li, Y. Li and F. Teng, Ultrason. Sonochem., 2022, 90, 106172 CrossRef CAS PubMed.
- M. M. Rahman and B. P. Lamsal, Compr. Rev. Food Sci. Food Saf., 2021, 20, 1457–1480 CrossRef CAS PubMed.
- Z. Wang, Y. Li, L. Jiang, B. Qi and L. Zhou, J. Chem., 2014, 2014, 475389 Search PubMed.
- J.-M. Wang, N. Xia, X.-Q. Yang, S.-W. Yin, J.-R. Qi, X.-T. He, D.-B. Yuan and L.-J. Wang, J. Agric. Food Chem., 2012, 60, 3302–3310 CrossRef CAS PubMed.
- S. Jiang, J. Ding, J. Andrade, T. M. Rababah, A. Almajwal, M. M. Abulmeaty and H. Feng, Ultrason. Sonochem., 2017, 38, 835–842 CrossRef CAS PubMed.
- B. P. Lamsal, S. Jung and L. A. Johnson, LWT–Food Sci. Technol., 2007, 40, 1215–1223 CrossRef CAS.
- G. Zhao, Y. Liu, M. Zhao, J. Ren and B. Yang, Food Chem., 2011, 127, 1438–1443 CrossRef CAS.
- X. Pi, J. Liu, Y. Sun, Q. Ban, J. Cheng and M. Guo, Food Chem., 2023, 405, 134820 CrossRef CAS PubMed.
- W. He, K. He, X. Liu, L. Ye, X. Lin, L. Ma, P. Yang and X. Wu, Food Chem., 2023, 415, 135733 CrossRef CAS PubMed.
- Y. Wan, J. Liu and S. Guo, Food Chem., 2018, 245, 542–550 CrossRef CAS PubMed.
- S. M. T. Gharibzahedi and B. Smith, Trends Food Sci. Technol., 2020, 98, 107–116 CrossRef CAS.
- L. Huang, X. Ding, Y. Li and H. Ma, Food Chem., 2019, 279, 114–119 CrossRef CAS PubMed.
- L. Zhou, J. Zhang, L. Xing and W. Zhang, Trends Food Sci. Technol., 2021, 110, 493–512 CrossRef CAS.
- R. S. H. Lam and M. T. Nickerson, Food Chem., 2013, 141, 975–984 CrossRef CAS PubMed.
- L. Amagliani, J. O'Regan, A. L. Kelly and J. A. O'Mahony, Trends Food Sci. Technol., 2017, 64, 1–12 CrossRef CAS.
- Q. Wang and Y. L. Xiong, Compr. Rev. Food Sci. Food Saf., 2019, 18, 936–952 CrossRef CAS PubMed.
- S. M. T. Gharibzahedi, S. Roohinejad, S. George, F. J. Barba, R. Greiner, G. V. Barbosa-Cánovas and K. Mallikarjunan, Trends Food Sci. Technol., 2018, 75, 194–205 CrossRef CAS.
- Y. Ren, L. Huang, Y. Zhang, H. Li, D. Zhao and J. Cao, Lancet Respir. Med., 2022, 10(4), 378–391 CrossRef PubMed.
- A. Pua, V. C. Y. Tang, R. M. V. Goh, J. Sun, B. Lassabliere and S. Q. Liu, Foods, 2022, 11, 875 CrossRef CAS PubMed.
- Y. Shao and C.-H. Tang, Food Res. Int., 2016, 79, 64–72 CrossRef CAS.
- A. Gomes and P. J. d. A. J. M. Sobral, Molecules, 2021, 27(1), 60 CrossRef PubMed.
- Z. L. Wan, J. Guo and X. Q. Yang, Food Funct., 2015, 6, 2876–2889 RSC.
- X. Yang, Y. Ren, H. Liu, C. Huo and L. Li, Int. J. Biol. Macromol., 2021, 185, 462–470 CrossRef CAS PubMed.
- Y. Wan, Y. Li and S. Guo, Food Hydrocolloids, 2021, 113, 106525 CrossRef CAS.
- E. M. Herz, S. Schäfer, N. Terjung, M. Gibis and J. Weiss, ACS Food Sci. Technol., 2021, 1, 1412–1417 CrossRef CAS.
- T. H. Quan, S. Benjakul, T. Sae-leaw, A. K. Balange and S. Maqsood, Trends Food Sci. Technol., 2019, 91, 507–517 CrossRef CAS.
- X. Yang, A. Li, D. Li, Y. Guo and L. Sun, Trends Food Sci. Technol., 2021, 109, 197–210 CrossRef CAS.
- A. Totosaus, J. G. Montejano, J. A. Salazar and I. Guerrero, Int. J. Food Sci. Technol., 2002, 37, 589–601 CrossRef CAS.
- T. Dapčević-Hadnađev, M. Hadnađev, A. Lazaridou, T. Moschakis and C. G. Biliaderis, Food Hydrocolloids, 2018, 81, 481–489 CrossRef.
- F. Alavi, Z. Emam-Djomeh and L. Chen, Food Hydrocolloids, 2020, 107, 105960 CrossRef CAS.
- S. Guidi, F. A. Formica and C. Denkel, Food Res. Int., 2022, 161, 111752 CrossRef CAS PubMed.
- T. Lafarga, C. Álvarez, G. Bobo and I. Aguiló-Aguayo, LWT, 2018, 98, 106–112 CrossRef CAS.
- C. Wen, J. Zhang, H. Yao, J. Zhou, Y. Duan, H. Zhang and H. Ma, Ultrason. Sonochem., 2019, 53, 83–98 CrossRef CAS PubMed.
- N. A. Mir, C. S. Riar and S. Singh, Food Hydrocolloids, 2019, 96, 433–441 CrossRef CAS.
|
This journal is © The Royal Society of Chemistry 2024 |
Click here to see how this site uses Cookies. View our privacy policy here.