Evaluation of the bioprotective potential of gastrointestinal digest fractions of Arthrospira sp. and Nannochloropsis sp. suspensions treated with high pressure homogenisation†
Received
16th October 2023
, Accepted 13th December 2023
First published on 3rd January 2024
Abstract
Microalgae are promising plant-like food sources rich in proteins and bioactive compounds with nutraceutical potential. This research investigated the health-promoting effects of high pressure homogenisation (HPH)-treated microalgal suspensions (8% w/v) of Arthrospira and Nannochloropsis species. These microalgal suspensions were treated using HPH at 300 bar, 600 bar, and 900 bar for a single pass. To gain valuable information on the bioavailability of potentially bioactive compounds, gastrointestinal digests obtained after in vitro simulated human gastrointestinal digestion of HPH-treated microalgal suspensions were examined for total phenolics, pigments, and antioxidant activity. Furthermore, their bioprotective potential was evaluated on an oxidatively stressed (induced by hydrogen peroxide) Caco-2 cell culture model system. Results showed that increasing the homogenisation pressure resulted in increased chlorophyll a and carotenoids release for both Arthrospira and Nannochloropsis species. Nannochloropsis sp. experienced an improved phenolics yield with HPH but a similar positive impact of HPH was not observed for Arthrospira sp. probably because of its inherent high phenolic content. Similarly, only Nannochloropsis sp. suspensions showed higher antioxidant activity by FRAP assay at 900 bar treatment. HPH-treated gastrointestinal digests of Arthrospira sp. treated at 600 bar and 900 bar demonstrated cell recovery and viability on stressed Caco-2 cells. However, there was an insignificant bioprotective effect from the HPH-treated Nannochloropsis digests. In general, it appears that the ideal homogenizing pressures for Arthrospira and Nannochloropsis suspensions are 300 and 900 bar, respectively. Overall, this study shows the potential of HPH as an efficient tool to produce functional foods and ingredients, particularly from Arthrospira sp. suspensions.
Sustainability spotlight
With minimal competition to existing land and water resources, microalgae have exhibited great potential as a more sustainable food source. Microalgal biomass can be used as a functional food ingredient for nutrient enrichment and health supplementation, as well as for its structuring potential. Since microalgal cells are enveloped by complex cell wall structures, disruption methods are required to enable release of valuable biofunctional components. High pressure homogenization (HPH) is a highly effective, and economically and environmentally sustainable microalgal disruption method due to its limited energy consumption and limited carbon dioxide emissions, among others. In consideration of SDGs 2, 3, 12, and 13, this study provides an understanding of the nutritional and functional potential of microalgae as influenced by HPH for sustainable utilisation in the food industry.
|
1 Introduction
Microalgae are a diverse group of highly efficient, photosynthetic marine organisms that can inhabit a variety of ecosystems. Adapting to extreme ecological conditions leads to their genetic and metabolic diversity, enabling microalgae to produce a broad spectrum of biologically active metabolites.1 Microalgae are abundant producers of bioactive molecules that include proteins, polysaccharides, polyphenolics, carotenoids, antioxidants, peptides, minerals, and essential vitamins.2 As such, microalgae are continually gaining popularity with their potential health benefits with the increasing number of related studies on their nutraceutical uses and subsequent commercialisation approaches.3,4
Among the numerous microalgal species, Arthrospira sp., Chlorella, Dunaliella salina, and Haematococcus pluvialis are the main microalgal biomass produced and utilised at the commercial level for various beneficial bioactive compounds in food, feed, and pharmaceutical applications.5Arthrospira sp. has been converted into tablets or capsules as health supplements or into powder as supplementary food ingredients.6 It is a major source of phycobiliproteins and other compounds, such as vitamin B-12, phenolic compounds, and carotenoids.7Chlorella sp. is considered a nutraceutical rich in proteins, lipids, polysaccharides, pigments, and vitamins.8D. salina is rich in a wide variety of carotenoids, including β-carotene that shows anticancer properties.9H. pluvialis is an important source of carotenoid astaxanthin that was reported to have several therapeutic applications.10
Microalgal cells are typically encapsulated by complex multilayer extracellular coverings that can limit the availability of valuable bioactive compounds. As such, cell disruption is the most critical step in the extraction of microalgal metabolites.11 High pressure homogenisation (HPH) is considered as a highly effective and scalable mechanical cell disruption technology for microalgal cells to extract intracellular components.12,13 Besides maximal disintegration of microalgal cells, HPH can influence the microstructural and rheological profiles of treated microalgal suspensions.14 HPH may also increase the availability of intracellular components of microalgae for improved nutritional benefits.12,15
There have been studies on microalgae's health-promoting properties due to bioactive compounds with a reportedly high antioxidant capacity.16 The antioxidant effect of microalgal molecules (e.g., enzymes, pigments, phytochelatins, polyphenols, or polysaccharides) provides protection from oxidative stress, which is a biological phenomenon that can lead to damage to biological systems and multiple degenerative diseases.17,18 Antioxidants are thought to protect cell constituents against oxidative damage through the scavenging of free radicals.19 However, pharmaceutical claims based on the antioxidant potential are still limited.20 Recent investigations have reported the intestinal barrier-protective activities of microalgal extracts of Chlorella sp., Arthrospira sp., and Synechococcus sp. in Caco-2 cells.21 In addition, isolated carotenoid extracts from Scenedesmus obliquus demonstrated improved bioaccessibility in Caco-2 cells.22 These studies show the potential of microalgae as a functional food for gut health maintenance.
This work aimed to determine the impact of HPH on the in vitro bioprotective potential of microalgal biomass with a focus on Arthrospira sp. and Nannochloropsis sp. suspensions. Arthrospira sp. is a suitable microalgal species for assessment since it is commercially used for food applications. In contrast to the fragile peptidoglycan cell walls of Arthrospira sp.,23Nannochloropsis sp. has a bilayered trilaminar outer layer, resulting in a more rigid cell wall.24 To the best of our knowledge, the impact of processing on the health-related properties of microalgal suspensions has not been investigated, particularly in its beneficial effects on human gut health. To investigate the health-promoting effects of HPH-treated microalgae, the pigment content, total phenolic content, and antioxidant activities using FRAP and DPPH assays were examined after the samples were subjected to in vitro simulated gastrointestinal digestion, which provides information on the bioavailability of food bioactive compounds. The bioprotective potential of the gastrointestinal digests from the microalgal suspensions on oxidatively stressed Caco-2 cells was also evaluated, where this cell line is chosen to represent the intestinal epithelial barrier.
2 Materials and methods
2.1 Microalgae and sample preparation
Freeze-dried biomass of Arthrospira sp. from BioBalance New Zealand (Collingwood, NZ) and wet paste of Nannochloropsis sp. from Reed Mariculture (California, USA) were obtained and prepared as described by Magpusao et al.14 (2021). Nannochloropsis sp. paste was maintained at −20 °C during storage and transport, then freeze-dried immediately upon arrival in the laboratory. The freeze-dried powder was vacuum-packed and kept at −20 °C storage until treatment.
2.2 High pressure homogenisation
Homogeneous microalgal aqueous suspensions were prepared using a modified protocol by Bernaerts et al. (2017).25 Briefly, powdered biomass was suspended overnight in distilled water at 8% (w/v) and then mixed using a homogeniser (ULTRA-TURRAX®, Krackeler Scientific, NY, USA) at 5000 rpm for 5 min. Suspensions were passed once through the high pressure homogeniser (PandaPlus 2000, GEA Niro Soavi, Italy) at 300, 600 and 900 bar. Treated samples were collected, cooled in an ice-water bath, and aliquoted into sealed containers. Untreated samples (non-HPH, 0 bar) served as control suspensions for this experiment. Samples were immediately immersed into liquid nitrogen for quick-freezing and then stored at −20 °C until analysis. Three independently prepared suspensions were used for the triplicate measurements.
2.3 Soluble protein determination
The protein contents of the samples were determined using the Lowry method with modifications.26 After mixing, 0.1 mL aliquot of suspension was placed in Eppendorf tubes, added with 1 mL of the Lowry reagent, and vortexed. After 10 min of incubation at room temperature, 0.1 mL of diluted Folin–Ciocalteu reagent was added, and the mixture was vortexed. After 30 min incubation, sample absorbance was measured at 750 nm using a spectrophotometer (Specord 50 Plus, Analytic Jena, Germany). The protein concentration was quantified with bovine serum albumin (BSA) solution as standard and reported as % (w/w) soluble proteins.
2.4 Simulated in vitro human gastrointestinal digestion
In vitro simulated human gastrointestinal digestion of microalgal suspensions was evaluated according to the standardised static in vitro digestion protocol27 with modifications.28 One (1) g of thawed sample was mixed with saliva juice and incubated for 5 min at 37 °C with constant shaking at 55 strokes per min. Alpha-amylase solution was added and incubated for 5 min at 37 °C with constant shaking. pH was adjusted to 3.0 using HCl (1 M) and added with pepsin solution, followed by incubation at 37 °C for 2 h with shaking at 55 strokes per min to simulate gastric digestion. pH was adjusted to 7.0 using NaOH (1 M) to deactivate pepsin, followed by the addition of pancreatin/bile solution and incubated at 37 °C for 2 h with constant shaking at 55 strokes per min to simulate intestinal digestion. The resulting mixture was filtered through Whatman grade 1 filter paper and stored at −80 °C until analysis. The mixtures, referred to as gastrointestinal digests, were used in all proceeding assays.
2.5 Quantification of free amino acids
Hydrolysed protein was assessed by o-phthalaldehyde (OPA) spectrophotometric assay.29 The hydrolysis rate was monitored by taking digest samples at different time points along gastric and intestinal digestion phases. Enzymes were deactivated by adding 0.5 mL of collected digest samples into Eppendorf tubes pre-filled with 0.5 mL of 20% (w/v) TCA, vortexed, and stored at −80 °C until analysis. After thawed samples were centrifuged to separate the supernatant from residual digested biomass and transferred into a 96-well plate, an OPA reagent was added with a 2
:
15 ratio (sample
:
OPA). The plate was immediately placed in the microplate reader (Synergy 2, BioTek Instruments, Vermont, USA), mixed for 30 s, allowed to react for 90 s, and absorbance was measured at 340 nm. The peptide concentration of protein hydrolysates was calculated as L-serine equivalents with reference to the L-serine standard curve made up of different concentrations (0, 25, 50, 100, 150, 200, 300, 400, and 500 mg L−1).
2.6 Pigment content of gastrointestinal digests
The determination of pigment content was based on a modified methanol extraction protocol.30 First, the microalgal suspension was mixed in methanol, vortexed for 1 min, and allowed to stand for 30 min at room temperature. Then, the solvent was recovered by centrifugation at 4000g for 10 min at 4 °C. Supernatant absorbance was measured at a spectrum from 350 to 850 nm using the spectrophotometer (Specord 50 Plus, Analytic Jena, Germany). Chlorophyll a, chlorophyll b, and carotenoids were determined as μg mL−1 using eqn (1)–(3):31 | Chlorophyll a (Ca) = 15.65A666 − 7.34 | (1) |
| Chlorophyll b (Cb) = 27.05A653 − 11.21A666 | (2) |
|  | (3) |
2.7 Total phenolic content of gastrointestinal digests
The total phenolic content was estimated using a modified version of the Folin–Ciocalteu method.32 In a 96-well plate, 20 μL samples were mixed with 100 μL of diluted Folin–Ciocalteu reagent and allowed to stand at room temperature for 5 min. Afterwards, 80 μL of sodium bicarbonate (75 g L−1) was added and incubated at room temperature in the dark for 60 min. Absorbance was measured at 765 nm against methanol as blank using a microplate reader (Synergy 2, BioTek Instruments, Vermont, USA). The total phenolic content of the samples was quantified with gallic acid as standard and reported as gallic acid equivalent (GAE) per g dry weight of microalgae.
2.8 Ferric reducing antioxidant power (FRAP) of gastrointestinal digests
The FRAP assay was determined using the method by Xiao et al. (2020).33 FRAP reagent was prepared with TPTZ (2,4,6-tripyridyl-S-triazine) solution (10 mM) in HCl (40 mM), acetate buffer (300 mM, pH 3.6), and iron(III) chloride solution (20 mM) in a 1
:
10
:
1 ratio, respectively. In a 96-well plate that contained 10 μL of samples, 240 μL of FRAP reagent was added. The plate was placed immediately in the microplate reader (Synergy 2, BioTek Instruments, Vermont, USA), preheated to 37 °C and gently shaken for 10 s. After incubation for 5 min at 37 °C, absorbance was read at 593 nm against methanol as blank. The FRAP antioxidant activity of samples was calculated with reference to Trolox as standard and reported as μM Trolox per g dry weight of microalgae.
2.9 DPPH-radical scavenging activity of gastrointestinal digests
2,2-Di(4-tert-octylphenyl)-1-picrylhydrazyl (DPPH) was used for the radical scavenger assay.34 In a 96-well plate, 100 μL of prepared DPPH (0.1 mM in methanol) was added with 100 μL of the gastrointestinal digests. The plate was placed immediately in the microplate reader fitted with temperature control of 25 °C and shaken for 10 s. The ability of the sample to scavenge DPPH was determined by following the decrease in absorbance at 517 nm for 60 min against methanol blank. The antioxidant activity refers to the DPPH inhibition when absorbance reduction reaches a plateau, typically within 20 to 30 min of the 25 °C incubation. The following formula is used to calculate the DPPH inhibition activity: |  | (4) |
where Abscontrol and Abssample are the absorbances of the DPPH control and gastrointestinal digest samples, respectively.
2.10 Determination of the bioprotective potential of gastrointestinal digests using Caco-2 cell lines
Human Caco-2 (colon cancer) cell lines (HTB-37; American Type Culture Collection, Rockville, Maryland, USA) were cultured in FBS-supplemented Dulbecco's modified Eagle's medium (DMEM, Gibco, Grand Island, New York, USA) in 50 cm2 plastic flasks at 37 °C under a humidified atmosphere of 5% CO2. The cell culture medium was changed every 2–3 days after reaching approximately 70% confluence.
2.10.1 Induction of oxidative stress.
Caco-2 cells previously grown as described above were trypsinised (0.5% Trypsin–EDTA, Gibco) and seeded at a density of 1 × 104 cells in a 96-well plate. Cells were cultured for 48 h under conditions previously indicated. The culture medium was removed and replaced with 6 μg mL−1 microalgal gastrointestinal digests in a complete medium for further incubation for 24 h. Afterwards, the medium with the digests was removed, and cells were washed with phosphate-buffered saline (PBS). Caco-2 cells were exposed to 500 μM H2O2 for 1 h. Four independent trials were conducted to assess cell viability using MTT and membrane LDH release assays.
2.10.2 MTT cell viability assay.
After Caco-2 cells were oxidatively stressed, 100 μL of 3-(4,5-dimethylthiazol-2-yl)-2,5-diphenyltetrazolium bromide (MTT) solution was added to each well, and the plate was incubated for 4 h at 37 °C under a humidified atmosphere of 5% CO2. After medium removal, formazan crystals were dissolved in 10% (w/v) sodium dodecyl sulfate (SDS) in HCl (10 mM). The absorbance was read at 517 nm on the microplate reader. Cell viability was calculated using the equation below and reported in normalised form as a percentage of viable cells (%): |  | (5) |
where Abscontrol and Abssample are the absorbances of the MTT control and gastrointestinal digest samples, respectively.
2.10.3 Membrane lactate dehydrogenase (LDH) release assay.
To evaluate LDH release as an indicative measure of possible cell membrane damage of Caco-2 cells, the culture supernatant from the previous step was harvested using a commercial kit (Cytotoxicity Detection Kit (LDH); Roche Diagnostics, Mannheim, Germany) according to the manufacturer's instructions. Culture supernatant was removed from the experimental culture plate, and 100 μL of reaction cocktail was mixed with the wells containing the residual cells. After 30 min of incubation at 37 °C, absorbance was measured at 490 nm using a microplate reader. Cytotoxicity (%), as indicated by the cell membrane integrity, was calculated as a percentage of cells using the following equation: |  | (6) |
where low control refers to absorbance of untreated cells (without exposure to the gastrointestinal digest), and high control refers to absorbance of cells that have been fully lysed by the lysis reagent included in the commercial kit.
2.11 Statistical analysis
The data are provided as mean ± standard deviation. The Student's t-test was used to compare control cells and cells treated with the digests. Differences with p < 0.05 were statistically significant. Statistical analysis was performed with Minitab 18 software (Minitab Inc., PA, USA) and GraphPad Prism Version 9.0 (GraphPad Software, San Diego, CA, USA).
3 Results and discussion
3.1 Effect of HPH on the in vitro protein human digestibility of microalgal suspensions
In the present study, Arthrospira had higher soluble protein contents across all homogenising pressures (28.98–30.81%) than Nannochloropsis (3.83–8.71%) (data not shown). Nannochloropsis had protein contents that were generally improved with the application of HPH, although the increase in protein did not follow a linear increase with the intensity of HPH. This is in agreement with reports of HPH allowing extraction of water-soluble proteins because of the effective disruption.12 For Arthrospira, the highest protein content was extracted at 300 bar, not being significantly affected by HPH at higher homogenising pressures of 600 and 900 bar. This result contrasts with the reported increase in the extraction yield of intracellular compounds (i.e., ionic substances, proteins, and carbohydrates) with elevated homogenising pressure levels and/or the number of passes due to extensive algal cell disruption.15,35 The lack of extraction efficacy of intracellular components has been associated with agglomeration, although this phenomenon is less associated with HPH.12 Aggregation of Arthrospira cells into larger particles, which were assumed to be composed of denatured proteins, has been reported with another processing method.25 HPH-induced aggregation has also been observed in other microalgal species, including Tetraselmis, Isochrysis, and Nannochloropsis species.14
In this study, only a small amount of protein from any HPH-treated microalgal suspension was digested during the gastric phase (see the ESI†) compared to the subsequent small intestinal digestion phase after the addition of pancreatin. Therefore, Fig. 1 presents the free amino groups of the protein hydrolysates expressed as L-serine equivalents during the suspensions' 4 hours small intestinal digestion period. Arthrospira had higher L-serine equivalent values (>50 mg per L L-serine equivalent) across the whole digestion period than Nannochloropsis, which may be due to the higher soluble protein content. However, the proteins in the untreated samples of the Arthrospira suspension appeared to be more readily hydrolysed at a significant level (p < 0.05) than those in HPH-treated samples. Similarly, protein digestibility for Nannochloropsis was not substantially affected by HPH with increasing homogenisation pressures. HPH may have altered the protein structure particularly with possible aggregate formation that influences the protein digestibility of microalgal suspensions.
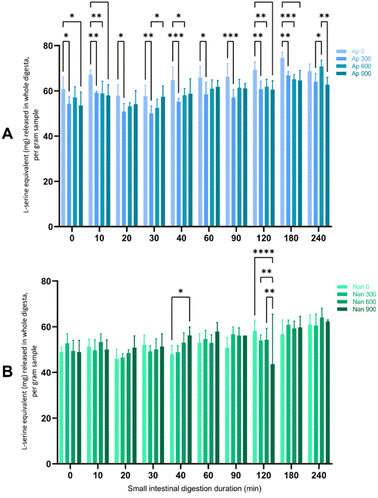 |
| Fig. 1
In vitro small intestinal protein digestion of (A) Arthrospira sp. and (B) Nannochloropsis sp. Values are mean ± standard deviation (n = 6). Significant differences are indicated by brackets with asterisks representing P value classification (p < 0.05). | |
Protein digestibility of algal products is influenced by a complex variety of factors, including cell wall structures, polysaccharides, and antinutritional factors.36 This is supported by the reported variation in hydrolysis and digestibility yield of C. vulgaris that was attributed to taxonomic differences in cell wall structures and physicochemical compositions.37 Incomplete hydrolysis has been reported in the microalgae S. almeriensis due to hydrophobic membrane proteins.38 The minimal impact of HPH on the digestibility of the intestinal digests is analogous to the slight change of pepsin digestibility of Chlorella protein as reported by Komaki et al. (1998).39 In their work, HPH slightly enhanced the digestibility of the Chlorella protein as indicated by the slight increase of pepsin digestibility with the treatment. Yet, compared to other treatments such as pulsed electric field, HPH had a higher degree of hydrolysis that signified more effective liberation of intracellular amino acids in S. almeriensis.38 Nonetheless, the current results show that further investigation is needed to elucidate the impact of HPH on in vitro protein digestibility, such as complementary determination of the presence of other factors (i.e., polysaccharides, amino acid profile, enzyme inhibitors). In the current work, the simulated in vitro digestion was performed to yield fractions of gastrointestinal digests that can reflect the impact of the HPH treatment on microalgal suspensions when ingested in the human gut.
3.2 Pigment content of gastrointestinal digests
Table 1 includes the chlorophyll a and carotenoid contents of the gastrointestinal digests from the samples that were subjected to in vitro simulated gastrointestinal digestion. Both chlorophyll a and carotenoids in Arthrospira digests were significantly higher at elevated homogenising pressures (0.12–0.36 μg mL−1 and 0.19–0.29 μg mL−1, respectively) suggesting the influence of HPH. Despite having lower pigment content, Nannochloropsis digests were likewise observed to show the positive impact of HPH with improved pigment release for both chlorophyll a and carotenoids as the pressure increased (0.11–0.24 μg mL−1 and 0.10–0.17 μg mL−1, respectively). For all samples, chlorophyll b was not detected. Unlike chlorophyll a that is ubiquitous in all algal classes, chlorophyll b is exclusive for some algal classes only.40 HPH has proven to be successful in completely disrupting microalgal cell walls resulting in the release of pigments along with other intracellular materials.15,41 The results of this study indicate the direct proportionality to the homogenising pressure used wherein the more intense the HPH treatment, the greater the amount of pigments released. This is desirable since valuable pigments are important contributors to the antioxidant properties of microalgae.20 However, very high pressures may not be fully beneficial since protein-pigment aggregation may arise with mechanical stress due to HPH and accompanying chemical changes with cell disruption.42 Total pigment content may be reduced due to the gelatinisation process that occurs with increased temperatures43 that may occur at higher HPH pressures with extended treatment time.
Table 1 Pigment (μg mL−1) of untreated (non-HPH) and HPH-treated microalgal suspensions. Values are mean ± standard deviation (n = 3). Means with different superscripts in the same row indicate a significant difference (p < 0.05)
Microalgae |
Homogenising pressure |
Chlorophyll a |
Carotenoids |
Arthrospira sp. |
Non-HPH |
0.18 ± 0.01b |
0.13 ± 0.00b |
300 bar |
0.29 ± 0.01a |
0.25 ± 0.02ab |
600 bar |
0.36 ± 0.05a |
0.29 ± 0.02a |
900 bar |
0.35 ± 0.05a |
0.29 ± 0.04a |
Nannochloropsis sp. |
Non-HPH |
0.11 ± 0.01b |
0.10 ± 0.02b |
300 bar |
0.10 ± 0.00b |
0.10 ± 0.01b |
600 bar |
0.13 ± 0.03b |
0.12 ± 0.03ab |
900 bar |
0.24 ± 0.02a |
0.17 ± 0.02a |
3.3 Total phenolic content of gastrointestinal digests
The gastrointestinal digests of the HPH-treated microalgal suspensions were evaluated for their total phenolic contents and antioxidant activities (Table 2). Arthrospira had higher total phenolic contents (240.13–247.78 mg GAE per g DM) while Nannochloropsis had lower contents (195.63–225.82 mg GAE per g DM), although the difference is not considerably significant. Only Nannochloropsis appears to be significantly affected by HPH where higher homogenising pressure improved the total phenolic content. The high phenolic content of Arthrospira has been mainly attributed to gallic, chlorogenic, 4-hydroxybenzoic, caffeic, and cinnamic acids.44
Table 2 Total phenolic contents, FRAP, and DPPH of untreated and HPH-treated microalgal suspension gastrointestinal digests. Values are mean values ± standard deviation. Different letters within each micro-algae group for each column indicate a significant difference (p < 0.05)
|
Homogenising pressure |
Total phenolic content (mg GAE per g dry mass) |
FRAP (μM Trolox per g dry mass) |
DPPH (% antioxidant activity) |
Arthrospira sp. |
Non-HPH |
245.64 ± 8.67a |
99.67 ± 8.66ab |
47.82 ± 0.90a |
300 bar |
247.78 ± 17.06a |
103.10 ± 11.93a |
48.24 ± 1.18a |
600 bar |
244.41 ± 12.43a |
85.68 ± 5.67b |
46.72 ± 0.93a |
900 bar |
240.13 ± 4.67a |
97.24 ± 9.29ab |
46.91 ± 1.64a |
Nannochloropsis sp. |
Non-HPH |
195.63 ± 9.37b |
94.31 ± 11.28a |
54.19 ± 3.03a |
300 bar |
201.39 ± 6.11b |
76.67 ± 7.78ab |
55.58 ± 0.66a |
600 bar |
206.08 ± 6.68b |
62.65 ± 9.14b |
53.22 ± 3.29a |
900 bar |
225.82 ± 4.55a |
88.97 ± 8.47a |
50.36 ± 2.95a |
In general, HPH is considered to improve the extractability and stability of phytochemicals, such as phenolic compounds and anthocyanins,45 although it is important to note that the impact of HPH is dependent on the food matrices. In fruit and vegetable products, the HPH technique has been identified as a favourable method to offer stabilized anthocyanin-rich emulsions.46 It has also been reported that HPH typically causes structural changes that enhance the bioavailability of bioactive compounds.47 Nevertheless, there are very limited studies on the impact of HPH on the phenolic content of microalgae. This study displays the potential of HPH in improving the functionality of microalgae due to the significant increase of total phenolic content with increased homogenising pressure. The rich presence of total phenolic content and resulting antioxidant properties in microalgae have been investigated.44,48 The antioxidant properties of phenolic compounds have been linked to various clinical conditions, such as cancer, diabetes, and other neurodegenerative diseases,49 hence the pharmaceutical potential of microalgae.
3.4 Antioxidant capacity of gastrointestinal digests by DPPH and FRAP assays
The antioxidant capacity of the gastrointestinal digests was evaluated by two different assays as shown in Table 2. FRAP assay refers to the free radical scavenging activity that can provide an estimate of antioxidants while the DPPH radical scavenging assay is based on the hydrogen donating ability of antioxidants.44 The ferric reducing capacities displayed minimal significant difference among the microalgal species. For Arthrospira, the impact of homogenising pressure was not clear and the FRAP values did not display significantly changing behaviour relative to the pressure level. On the other hand, Nannochloropsis displayed a general trend of reduction in FRAP activity with increasing pressure despite the difference being minimal. The results show that HPH did not assist in the extraction improvement of antioxidant molecules for the microalgal species. However, significant increase of FRAP activity was only observed at 900 bar for Nannochloropsis (88.9 μm Trolox per g), which suggests greater potential of HPH at higher pressures. In terms of % antioxidant activity determined by DPPH assay, Nannochloropsis had higher values (50.35–55.58%), although HPH did not significantly influence the antioxidant activities. Arthrospira had lower values (46.72–48.24% AA) and was similarly not significantly influenced by HPH.
There is limited investigation on the impact of HPH and other processing methods on microalgal antioxidant activities. Sonication had dissimilar effects dependent on the microalgal species, wherein increased antioxidant activity was reported for A. platensis but reduction for N. gaditana and S. almeriensis.50 Antioxidant activities as impacted by HPH appear to be more differentiated by their ferric reducing capacities. The limited consequence of homogenising pressure on the microalgal digest suspensions was comparable for the DPPH radical scavenging activities and total phenolic contents. The restrictions associated with assessing antioxidant activity with the DPPH assay have been observed to be less biologically relevant than the other assays.48 Others reported that antioxidant capacities of some microalgae extracts could not be majorly associated with their phenolic components.32 Due to the antioxidant potential being linked to the presence of polyphenols and other bioactive compounds in a complex mechanism, a link between the antioxidant capacity of samples and the release or degradation of specific components could not be explicitly made.50 Other variables that could influence the antioxidant capacities and radical scavenging activities of microalgae are solvent polarity and dose-dependency.32,51–53
3.5 Bioprotective effect of microalgal suspension gastrointestinal digests on Caco-2 cells
As an initial step in determining the bioprotective effect of the samples, the concentration of gastrointestinal digests was tested from 3 to 50% (w/v) diluted in FBS-supplemented Dulbecco's modified Eagle's medium, where the optimal concentration was selected based on the non-cytotoxicity effect to the Caco-2 cells. The addition of 6 μg mL−1Arthrospira and Nannochloropsis digests showed no significant cytotoxicity against the Caco-2 cells after 24 h of treatment but displayed pronounced cytotoxicity at 48 and 72 h. Hence, this dose and treatment time were used in the cell line experiments (data not shown). The simulated gastrointestinal digests of the Arthrospira and Nannochloropsis suspensions were evaluated based on their ability to aid bioprotection of Caco-2 cells against oxidative stress induced by H2O2.
The induction of oxidative stress on the Caco-2 cells is critical in understanding the beneficial impact of microalgae biomass/extracts upon ingestion into the human body. Oxidative stress is generally prompted by a wide range of environmental factors that lead to the cellular accumulation of reactive oxygen species (ROS).54 While ROS production can be controlled by the intrinsic defence mechanism of antioxidant enzymes, intense stress can lead to overproduction of ROS to excess levels. At this point, cellular damage may occur through modification of nucleic acids, oxidation of proteins, and lipid peroxidation.55 Oxidative damage and apoptosis in the unicellular green algae C. reinhardtii due to H2O2 exposure (10 mM) has been attributed to the alteration of mitochondrial membrane potential.55
The bioprotective effect of HPH-treated microalgal suspensions against oxidative stress was evaluated through the MTT and LDH release assays. Based on the results shown based on the MTT assay (Fig. 2), the exposure to 500 μM H2O2 induced oxidative stress of Caco-2 cells pre-incubated with either Arthrospira or Nannochloropsis digests caused 50% cell viability. For both species, the LDH release assay (Fig. 3) shows that the cell viability of all cell cultures exposed to the microalgal digests was significantly (p < 0.05) lower than that of the control groups. The low cell viability (<50%) was consistent for both non-stressed and stressed cells exposed to untreated and HPH-treated suspensions. This indicated that the leakage of lactate dehydrogenase may have contributed to the toxic effect upon Caco-2 cells.
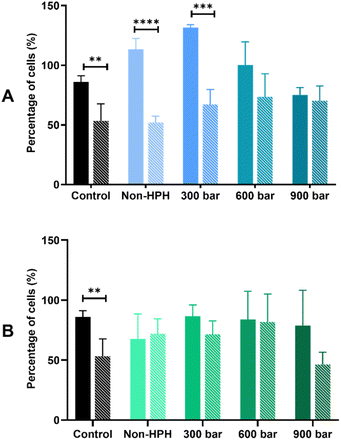 |
| Fig. 2 Bioprotective effect on stressed Caco-2 cells after 24 hours exposure to microalgal suspension gastrointestinal digests of (A) Arthrospira sp. and (B) Nannochloropsis sp. by MTT assay. Control refers to Caco-2 cells without any microalgal digests. Solid bars refer to non-stressed cells with the digests, and patterned bars refer to H2O2-stressed cells. Data are expressed as means ± standard deviations (n = 4). * indicates a significant difference (p < 0.05) between non-stressed and stressed cells. | |
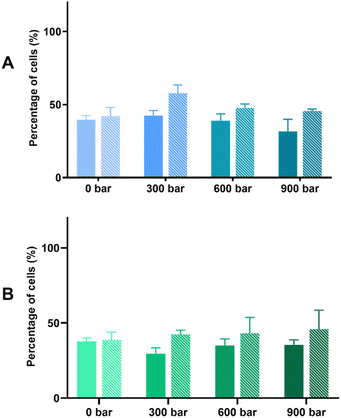 |
| Fig. 3 Bioprotective effect on stressed Caco-2 cells after 24 hours exposure to microalgal suspension gastrointestinal digests of (A) Arthrospira sp. and (B) Nannochloropsis sp. by the membrane LDH release assay. Solid bars refer to non-stressed cells with the digests, and patterned bars refer to H2O2-stressed cells. Data are expressed as means ± standard deviations (n = 4). * indicates a significant difference (p < 0.05) between non-stressed and stressed cells. | |
In the MTT assay shown in Fig. 2A, cell cultures with the non-HPH and 300 bar Arthrospira digests had the cell viability of stressed cells being significantly different from that of the non-stressed cells. This indicates that cell viability was not improved with the exposure to the digests that were not treated or minimally treated with HPH. In contrast, with the exposure to Arthrospira digests from 600 and 900 bar, the absence of a significant difference between the non-stressed and stressed cells shows that cell viability was improved indicating bioprotective effect. It appears that HPH-treated digests at 600 bar had the most effective bioprotective effect on the stressed Caco-2 cells since it resulted in higher cell viability compared to the other digests. This is supported by the elevated chlorophyll a and carotenoids at this homogenising pressure as presented in Table 1. This indicates the potential of improving the antioxidant potential of HPH-treated Arthrospira suspensions. The cell viability of Caco-2 cells with the Nannochloropsis digests was generally slightly lower than those with the Arthrospira digests with HPH. Fig. 2B shows that untreated and HPH-treated Nannochloropsis digests were not able to provide a bioprotective effect on the stressed Caco-2 cells. The results of the LDH release assay for both Arthrospira and Nannochloropsis (Fig. 3) show no significant differences between the non-stressed and stressed cells. LDH release can be used as a marker of the potential protective role of the samples against membrane leakage of epithelial cells caused by oxidative stress.21 This implies that the bioprotective effect of Arthrospira is not due to the recovery of the membrane but may be due to other mechanisms. Other microalgae extracts have shown a protective role, such as T. suecica that showed repairing activity in the human lung cancer line when applied to cells after oxidative stress.53 Aqueous extracts of Chlorella, Arthrospira, and Synechococcus species showed antioxidant potential in Caco-2 cells by preventing intracellular oxidant burst, cell membrane damage, and apoptosis induced by oxidative stress.21
The difference in the results of the assays conducted in this current research work could be attributed to two things: (1) most investigations on Caco-2 cells have checked the bioprotective effect of the microalgal suspensions, and (2) Caco-2 cells are exposed to material suspensions and not intestinal digests that have undergone simulated in vitro gastrointestinal digestion. Another factor to be considered is the form of microalgal extract that is used for cell treatments. Aqueous extracts of Dunaliella were markedly more cytotoxic to breast cancer cells than ethanolic and hydroalcoholic extracts.56 As with radical scavenging activity, reduction on cell proliferation in Caco-2 cells due to cytotoxicity of utilised metabolites occurs in a dose and time-dependent manner.57 The relationship between antioxidant activity (i.e., FRAP) and cytotoxicity against cell lines appears to be not directly correlated.48 Nevertheless, the current study shows that as phenolic compounds may occur abundantly in microalgae, selected species may have intestinal protective mechanisms by assisting in recovery of oxidatively stressed Caco-2 cells. Additionally, the application of HPH can improve this health-promoting potential of microalgae-based antioxidant compounds.
4 Conclusions
Generally, HPH had minimal impact on the extracted soluble protein from HPH-treated Arthrospira while enhancing those from Nannochloropsis suspensions. The results show that HPH had minimal and no clear effect on the in vitro protein digestibility in terms of the L-serine equivalent of the protein hydrolysates for all the microalgal species. To gain a better insight into the protein digestibility of the microalgal species, it would be beneficial to investigate using assays, such as protein efficiency ratio (PER), digestibility coefficient (DC), and net protein utilisation (NPU).
HPH had a significant impact on the amount of chlorophyll a and carotenoids in the gastrointestinal digests of both Arthrospira and Nannochloropsis with increased homogenising pressure resulting in significantly higher pigment amounts. Arthrospira had the highest phenolic contents without the impact of HPH while only Nannochloropsis had improved yield of phenolics with HPH treatment. Similarly, only Nannochloropsis suspensions showed higher antioxidant activity at 900 bar while the others were not positively affected. To further elucidate the biological activities of the gastrointestinal digests, cell culture experiments using Caco-2 cell lines were performed. Exposure of oxidatively stressed Caco-2 cells to HPH-treated gastrointestinal digests of Arthrospira treated at 600 bar and 900 bar showed recovery and improved cell viability. This result shows the potential of HPH in enhancing the health-promoting properties of antioxidant compounds in Arthrospira suspensions because of the bioprotective effect in the Caco-2 cells. In contrast, this study did not observe any significant bioprotective effect from the HPH-treated Nannochloropsis digests. Therefore, the protective mechanism of the gastrointestinal digests could not be attributed to their ability to prevent membrane leakage of epithelial cells. There is a need for further investigation with other assays to elucidate the protective mechanism of HPH-treated microalgal suspensions. Additionally, future studies could involve isolating active components in the microalgae and characterising their effects and finding synergistic effects of the different antioxidant molecules.
Author contributions
Conceptualization: I. O. and B. K.; methodology: J. M., I. O. and B. K.; validation: J. M., I. O. and B. K.; formal analysis: J. M. and I. O.; investigation: J. M. and I. O.; writing—original draft preparation: J. M.; writing—review and editing: J. M., I. O., B. K.; supervision: I. O. and B. K. All authors have read and agreed to the published version of the manuscript.
Conflicts of interest
The authors declare no conflict of interest.
Acknowledgements
The authors would like to acknowledge the New Zealand Development Scholarship funded through the Ministry of Foreign Affairs and Trade for the doctoral scholarship. This work is supported by the Riddet Institute, a New Zealand Centre of Research Excellence.
Notes and references
- M. G. De Morais, B. D. S. Vaz, E. G. De Morais and J. A. V. Costa, Biologically Active Metabolites Synthesized by Microalgae, BioMed Res. Int., 2015, 2015, 835761 Search PubMed.
- R. Sathasivam, R. Radhakrishnan, A. Hashem and E. F. Abd_Allah, Microalgae metabolites: a rich source for food and medicine, Saudi J. Biol. Sci., 2019, 26(4), 709–722 CrossRef CAS PubMed.
- E. Jacob-Lopes, M. M. Maroneze, M. C. Deprá, R. B. Sartori, R. R. Dias and L. Q. Zepka, Bioactive food compounds from microalgae: an innovative framework on industrial biorefineries, Curr. Opin. Food Sci., 2019, 25, 1–7 CrossRef.
- M. L. Wells, P. Potin, J. S. Craigie, J. A. Raven, S. S. Merchant and K. E. Helliwell,
et al., Algae as nutritional and functional food sources: revisiting our understanding, J. Appl. Phycol., 2017, 29(2), 949–982 CrossRef CAS PubMed.
-
G. Rajauria, L. Cornish, F. Ometto, F. E. Msuya and R. Villa, Identification and selection of algae for food, feed, and fuel applications, in Seaweed Sustainability, Elsevier Inc., 2015, pp. 315–46, DOI:10.1016/B978-0-12-418697-2/00012-X.
- R. Raja, A. Coelho, S. Hemaiswarya, P. Kumar, I. S. Carvalho and A. Alagarsamy, Applications of microalgal paste and powder as food and feed: An update using text mining tool, Beni-Suef Univ. J. Basic Appl. Sci., 2018, 7(4), 740–747, DOI:10.1016/j.bjbas.2018.10.004.
- B. d. S. Vaz, J. B. Moreira, M. G. d. Morais and J. A. V. Costa, Microalgae as a new source of bioactive compounds in food supplements, Curr. Opin. Food Sci., 2016, 7, 73–77, DOI:10.1016/j.cofs.2015.12.006.
- C. Safi, B. Zebib, O. Merah, P. Y. Pontalier and C. Vaca-Garcia, Morphology, composition, production, processing and applications of Chlorella vulgaris: A review, Renewable Sustainable Energy Rev., 2014, 35, 265–278 CrossRef.
- K. R. Jayappriyan, R. Rajkumar, V. Venkatakrishnan, S. Nagaraj and R. Rengasamy, In vitro anticancer activity of natural β-carotene from Dunaliella salina EU5891199 in PC-3 cells, Biomed. Prev. Nutr., 2013, 3(2), 99–105, DOI:10.1016/j.bionut.2012.08.003.
- M. M. Mendes-Pinto, M. F. J. Raposo, J. Bowen, A. J. Young and R. Morais, Evaluation of different cell disruption processes on encysted cells of Haematococcus pluvialis: effects on astaxanthin recovery and implications for bio-availability, J. Appl. Phycol., 2001, 13(1), 19–24 CrossRef.
- W. N. Phong, P. L. Show, T. C. Ling, J. C. Juan, E. P. Ng and J. S. Chang, Mild cell disruption methods for bio-functional proteins recovery from microalgae—Recent developments and future perspectives, Algal Res., 2018, 31, 506–516, DOI:10.1016/j.algal.2017.04.005.
- N. Grimi, A. Dubois, L. Marchal, S. Jubeau, N. I. Lebovka and E. Vorobiev, Selective extraction from microalgae Nannochloropsis sp. using different methods of cell disruption, Bioresour. Technol., 2014, 153, 254–259, DOI:10.1016/j.biortech.2013.12.011.
- C. Safi, L. Cabas Rodriguez, W. J. Mulder, N. Engelen-Smit, W. Spekking and L. A. M. van den Broek,
et al., Energy consumption and water-soluble protein release by cell wall disruption of Nannochloropsis gaditana, Bioresour. Technol., 2017, 239, 204–210, DOI:10.1016/j.biortech.2017.05.012.
- J. Magpusao, S. Giteru, I. Oey and B. Kebede, Effect of high pressure homogenization on microstructural and rheological properties of A. platensis, Isochrysis, Nannochloropsis and Tetraselmis species, Algal Res., 2021, 56, 102327, DOI:10.1016/j.algal.2021.102327.
- D. Carullo, B. D. Abera, A. A. Casazza, F. Donsì, P. Perego and G. Ferrari,
et al., Effect of pulsed electric fields and high pressure homogenization on the aqueous extraction of intracellular compounds from the microalgae Chlorella vulgaris, Algal Res., 2018, 31, 60–69, DOI:10.1016/j.algal.2018.01.017.
- M. F. G. Assunção, R. Amaral, C. B. Martins, J. D. Ferreira, S. Ressurreição and S. D. Santos,
et al., Screening microalgae as potential sources of antioxidants, J. Appl. Phycol., 2017, 29(2), 865–877 CrossRef.
- J. T. Cirulis, J. A. Scott and G. M. Ross, Management of oxidative stress by microalgae, Can. J. Physiol. Pharmacol., 2013, 91(1), 15–21 CrossRef CAS PubMed.
- O. Blokhina, E. Virolainen and K. V. Fagerstedt, Antioxidants, oxidative damage and oxygen deprivation stress: A review, Ann. Bot., 2003, 91, 179–194 CrossRef CAS PubMed.
- A. Scalbert, I. T. Johnson and M. Saltmarsh, Polyphenols: antioxidants and beyond, Am J Clin Nutr, 2005, 81(1), 215–217 CrossRef PubMed.
- U. T. Ferdous and Z. N. B. Yusof, Medicinal Prospects of Antioxidants From Algal Sources in Cancer Therapy, Front. Pharmacol., 2021, 12, 1–22 Search PubMed.
- W. Guo, S. Zhu, G. Feng, L. Wu, Y. Feng and T. Guo,
et al., Microalgae aqueous extracts exert intestinal protective effects in Caco-2 cells and dextran sodium sulphate-induced mouse colitis, Food Funct., 2020, 11(1), 1098–1109 RSC.
- T. C. do Nascimento, P. N. Pinheiro, A. S. Fernandes, D. C. Murador, B. V. Neves and C. R. de Menezes,
et al., Bioaccessibility and intestinal uptake of carotenoids from microalgae Scenedesmus obliquus, LWT, 2021, 140, 110780, DOI:10.1016/j.lwt.2020.110780.
-
C. Sili, G. Torzillo and A. Vonshak, Arthrospira (Spirulina), in Ecology of Cyanobacteria II: Their Diversity in Space and Time, ed. B. A. Whitton, Springer Science+Business Media B.V., 2012, pp. 677–705 Search PubMed.
- M. J. Scholz, T. L. Weiss, R. E. Jinkerson, J. Jing, R. Roth and U. Goodenough,
et al., Ultrastructure and composition of the Nannochloropsis gaditana cell wall, Eukaryotic Cell, 2014, 13(11), 1450–1464 CrossRef PubMed.
- T. M. M. Bernaerts, A. Panozzo, V. Doumen, I. Foubert, L. Gheysen and K. Goiris,
et al., Microalgal biomass as a (multi)functional ingredient in food products: Rheological properties of microalgal suspensions as affected by mechanical and thermal processing, Algal Res., 2017, 25, 452–463, DOI:10.1016/j.algal.2017.05.014.
- C. V. González-López, M. d. C. Cerón-García, F. G. Acién Fernández, C. S. Bustus, Y. Chisti and J. M. Fernández-Sevilla, Protein measurements of microalgal and cyanobacterial biomass, Bioresour. Technol., 2010, 101, 7587–7591 CrossRef PubMed.
- M. Minekus, M. Alminger, P. Alvito, S. Ballance, T. Bohn and C. Bourlieu,
et al., A standardised static in vitro digestion method suitable for food-an international consensus, Food Funct., 2014, 5(6), 1113–1124 RSC.
- S. B. M. Abduh, S. Y. Leong, D. Agyei and I. Oey, Understanding the properties of starch in potatoes (Solanum tuberosum var. Agria) after being treated with pulsed electric field processing, Foods, 2019, 8(5), 159 CrossRef CAS PubMed.
- P. Khrisanapant, B. Kebede, S. Y. Leong and I. Oey, A comprehensive characterisation of volatile and fatty acid profile of legume seeds, Foods, 2019, 8(651), 6–9 Search PubMed.
- S. Kulkarni and Z. Nikolov, Process for selective extraction of pigments and functional proteins from Chlorella vulgaris, Algal Res., 2018, 35, 185–193, DOI:10.1016/j.algal.2018.08.024.
- A. R. Wellburn, The Spectral Determination of Chlorophylls a and b, as well as Total Carotenoids, Using Various Solvents with Spectrophotometers of Different Resolution, J. Plant Physiol., 1994, 144(3), 307–313, DOI:10.1016/S0176-1617(11)81192-2.
- H. B. Li, K. W. Cheng, C. C. Wong, K. W. Fan, F. Chen and Y. Jiang, Evaluation of antioxidant capacity and total phenolic content of different fractions of selected microalgae, Food Chem., 2007, 102(3), 771–776 CrossRef CAS.
- F. Xiao, T. Xu, B. Lu and R. Liu, Guidelines for antioxidant assays for food components, Food Front., 2020, 1(1), 60–69 CrossRef.
- H. H. Senousy, S. Abd Ellatif and S. Ali, Assessment of the antioxidant and anticancer potential of different isolated strains of cyanobacteria and microalgae from soil and agriculture drain water, Environ. Sci. Pollut. Res., 2020, 27(15), 18463–18474 CrossRef CAS PubMed.
- C. Shene, T. Monsalve and D. Vergara, High pressure homogenization of Nannochloropsis oculata for the extraction of intracellular components : Effect of process conditions and culture age, Eur. J. Lipid Sci. Technol., 2016, 631–639 CrossRef CAS.
- L. Machů, L. Mišurcová, D. Samek, J. Hrabě and M. Fišera, In vitro digestibility of different commercial edible algae products, J. Aquat. Food Prod. Technol., 2014, 23(5), 423–435, DOI:10.1080/10498850.2012.721873.
- A. Kose, M. O. Ozen, M. Elibol and S. S. Oncel, Investigation of in vitro digestibility of dietary microalga Chlorella vulgaris and cyanobacterium Spirulina platensis as a nutritional supplement, 3 Biotech, 2017, 7(3), 1–7 CrossRef PubMed.
- S. Akaberi, C. Gusbeth, A. Silve, D. S. Senthilnathan, E. Navarro-López and E. Molina-Grima,
et al., Effect of pulsed electric field treatment on enzymatic hydrolysis of proteins of Scenedesmus almeriensis, Algal Res., 2019, 43, 101656, DOI:10.1016/j.algal.2019.101656.
- H. Komaki, M. Yamashita, Y. Niwa, Y. Tanaka, N. Kamiya and Y. Ando,
et al., The effect of processing of Chlorella vulgaris: K-5 on in vitro and in vivo digestibility in rats, Anim. Feed Sci. Technol., 1998, 70(4), 363–366 CrossRef CAS.
-
A. N. Das, Algal Biorefinery: An Integrated Approach, Agrarian Movements in India: Studies on 20th Century Bihar, ed. D. Das, Springer International Publishing, New Delhi, India, 2015, p. 479 Search PubMed.
- S. Jubeau, L. Marchal, J. Pruvost, P. Jaouen, J. Legrand and J. Fleurence, High pressure disruption: A two-step treatment for selective extraction of intracellular components from the microalga Porphyridium cruentum, J. Appl. Phycol., 2013, 25(4), 983–989 CrossRef CAS.
- F. Ba, A. V. Ursu, C. Laroche and G. Djelveh, Haematococcus pluvialis soluble proteins: Extraction, characterization, concentration/fractionation and emulsifying properties, Bioresour. Technol., 2016, 200, 147–152, DOI:10.1016/j.biortech.2015.10.012.
- L. Gouveia, A. P. Batista, A. Raymundo and N. Bandarra, Spirulina maxima and Diacronema vlkianum microalgae in vegetable gelled desserts, Nutr. Food Sci., 2008, 38(5), 492–501 CrossRef.
- H. H. Abd El-Baky, F. K. El Baz and G. S. El-Baroty, Production of phenolic compounds from Spirulina maxima microalgae and its protective effects in vitro toward hepatotoxicity model, Afr. J. Pharm. Pharmacol., 2009, 3(4), 133–139 CAS.
- S. X. M. Yong, C. P. Song and W. S. Choo, Impact of High-Pressure Homogenization on the Extractability and Stability of Phytochemicals, Front. Sustain. Food Syst., 2021, 4, 1–13 Search PubMed.
- K. Marszałek, Ł. Woźniak, B. Kruszewski and S. Skapska, The effect of high pressure techniques on the stability of anthocyanins in fruit and vegetables, Int. J. Mol. Sci., 2017, 18(2), 277 CrossRef PubMed.
- E. Betoret, N. Betoret, P. Rocculi and M. Dalla Rosa, Strategies to improve food functionality: Structure-property relationships on high pressures homogenization, vacuum impregnation and drying technologies, Trends Food Sci. Technol., 2015, 46(1), 1–12, DOI:10.1016/j.tifs.2015.07.006.
- J. Matos, C. L. Cardoso, P. Falé, C. M. Afonso and N. M. Bandarra, Investigation of nutraceutical potential of the microalgae Chlorella vulgaris and Arthrospira platensis, Int J Food Sci. Technol., 2020, 55(1), 303–312 CrossRef CAS.
- W. Dröge, Free radicals in the physiological control of cell function, Physiol. Rev., 2002, 82(1), 47–95 CrossRef PubMed.
- M. Martínez-Sanz, A. Garrido-Fernández, A. Mijlkovic, A. Krona, A. Martínez-Abad and J. M. Coll-Marqués,
et al., Composition and rheological properties of microalgae suspensions: Impact of ultrasound processing, Algal Res., 2020, 49, 101960, DOI:10.1016/j.algal.2020.101960.
- I. Jerez-Martel, S. García-Poza, G. Rodríguez-Martel, M. Rico, C. Afonso-Olivares and J. L. Gómez-Pinchetti, Phenolic profile and antioxidant activity of crude extracts from microalgae and cyanobacteria strains, J. Food Qual., 2017, 2017, 2924508 CrossRef.
- M. B. E. N. Hafsa, M. B. E. N. Ismail and M. Garrab, Antimicrobial, antioxidant, cytotoxic & anticholinestrase activities of water-soluble polysaccharides extracted from microalgae Isochrysis galbana and Nannochloropsis oculata, J. Serb. Chem. Soc., 2017, 82(5), 509–522 CrossRef.
- C. Sansone, C. Galasso, I. Orefice, G. Nuzzo, E. Luongo and A. Cutignano,
et al., The green microalga Tetraselmis suecica reduces oxidative stress and induces repairing mechanisms in human cells, Sci. Rep., 2017, 7, 1–12, DOI:10.1038/srep41215.
- P. Moradas-Ferreira, V. Costa, P. Piper and W. Mager, The molecular defences against reactive oxygen species in yeast, Mol. Microbiol., 1996, 19(4), 651–658 CrossRef CAS PubMed.
- S. L. Vavilala, K. K. Gawde, M. Sinha and J. S. D'Souza, Programmed cell death is induced by hydrogen peroxide but not by excessive ionic stress of sodium chloride in the unicellular green alga Chlamydomonas reinhardtii, Eur. J. Phycol., 2015, 50(4), 422–438, DOI:10.1080/09670262.2015.1070437.
- F. Elleuch, P. Baril, M. Barkallah, F. Perche, S. Abdelkafi and I. Fendri,
et al., Deciphering the biological activities of Dunaliella sp. Aqueous extract from stressed conditions on breast cancer: From in vitro to in vivo investigations, Int. J. Mol. Sci., 2020, 21(5) CAS.
- C. Fernández-Blanco, G. Font and M. J. Ruiz, Oxidative stress of alternariol in Caco-2 cells, Toxicol. Lett., 2014, 229(3), 458–464, DOI:10.1016/j.toxlet.2014.07.024.
|
This journal is © The Royal Society of Chemistry 2024 |