Encapsulation of bioactive compounds extracted from haritaki pulp (Terminalia chebula Retzius): characterization of physical, thermal, and morphological properties
Received
10th August 2023
, Accepted 2nd February 2024
First published on 22nd February 2024
Abstract
The bioactive compounds of haritaki (Terminalia chebula Retzius) were microencapsulated using zein and starch as the encapsulating agents, utilizing both conventional (encapsulator) and advanced (freeze drying) techniques. It has been found that freeze-drying is a highly effective method for producing bioactive compounds with high encapsulation efficiency and yield. The bioactive compounds were extracted using supercritical fluid extraction. The optimized conditions for the supercritical fluid extractor were flow rate 3.34 ml min−1, pressure 166.94 bar, temperature 51.97 °C, time 67.47 min and CO2 mass flow rate of 5 kg h−1, kept constant for all the experiments. Comparing the different drying techniques, fresh haritaki produced the highest recovery of phenolic chemicals. Different combinations of starch
:
zein were tested for encapsulating the bioactive compounds, and based on the yield, encapsulation efficiency, and powder density, 100% starch presented the best yield (65.45 ± 2.58), encapsulation efficiency (75.95 ± 0.88), powder bulk density (0.61 ± 0.01) and tapped density (0.72 ± 0.01). Encapsulates were found to have an uneven and rough shape based on the SEM analysis. The combination of 70
:
30 (starch
:
zein) showed the largest mass loss (68.12%) using TGA, whereas 100% zein (58.63%) showed the lowest mass loss. From the DSC curves, it was noticed that the maximum onset point (88.40) and peak point (120.40) came from using 50
:
50 (starch
:
zein) encapsulates, while the minimum onset point (77.70) and peak point (107.00) from 70
:
30 (starch
:
zein) encapsulates. The endpoint was seen in 100% zein (142.70) and 100% starch encapsulates (142.50) whereas 70
:
30 (starch
:
zein) encapsulates (122.30) showed a minimum value. The bond stretching and vibrations of 100% zein encapsulates were the greatest, while those made from 100% starch encapsulates had very little stretching and low bond intensities, according to the FTIR spectra. The XRD patterns showed that the only encapsulate with a strong peak were the 50
:
50 (starch
:
zein). According to this study, freeze-drying the bioactive chemicals of haritaki extract can successfully encapsulate them, and the encapsulates can then be used to manufacture functional foods.
Sustainability spotlight
Encapsulation of Terminalia chebula pulp extract was done by different methods like encapsulation in sodium alginate and using starch : zein in various proportions. The properties of the encapsulates were studied and compared. The results of the research lead to a sustainable approach for delivery of bioactive compounds from a medicinal plant like T. chebula which may be further used for the development of functional food products.
|
1. Introduction
Haritaki (Terminalia chebula Retzius) is a very famous rejuvenating herb, and a medium to large-sized tree distributed throughout tropical and subtropical Asia, including China, Bangladesh, Bhutan and Tibet. The food and flavour industries are looking for novel food components to use in the development of food supplements. The consumption of haritaki provides nutrition and could aid in the prevention of many degenerative illnesses such as cancer, neurological and cardiovascular disorders, ageing, and so on.1 The economic worth of the raw material and the profitability of haritaki processing could both increase with the extraction of the beneficial chemicals found in the pulp. The use of bioactive chemical extracts in food is also subject to a number of restrictions, including their limited stability, which is altered by solvents, pH, temperature, oxygen, light, and enzymes.
Encapsulation is described as a process that surrounds small particles or droplets with a coating or embeds them in a homogeneous or heterogeneous matrix to produce small capsules with a variety of useful properties.2,3 Encapsulation is also used to increase the stability of compounds using processes such as freeze-drying, particularly significant for processing haritaki. However, because encapsulation takes place under various time and temperature conditions, the resulting products have varying qualities.3 Various techniques are used to microencapsulate residue extracts and other bioactive substances from natural sources, such as phenolic compounds from the peel of pomegranate (Punica granatum L.) and grape (Vitis labrusca var. Bordo) and jaboticaba (Myrciaria jaboticaba) and blackberry (Rubus fruticosus) and grape pomace.4,5
Encapsulation of bioactive compounds is a vital step before introducing them into the food system. This process is essential to safeguard these compounds from the rigors of food processing and manufacturing, where exposure to factors like heat, light, and moisture can lead to degradation and loss of efficacy.6 By providing a protective barrier, encapsulation ensures the stability of bioactive compounds during these processes, extending the shelf life of food products.7 Additionally, encapsulation enhances the solubility of bioactive compounds, making them more adaptable to a variety of food formulations. It minimizes interactions with other ingredients, preserving the overall quality of the food product. The controlled release of encapsulated compounds during digestion or processing further optimizes their bioavailability.8 Encapsulation also serves to mask any undesirable tastes or odours associated with certain bioactive compounds, facilitating their incorporation into diverse food products without compromising the sensory attributes of the food. Ultimately, encapsulation plays a pivotal role in enhancing the overall suitability, stability, and efficacy of bioactive compounds in the food system, making them more versatile for integration into a wide array of food applications.9
The capacity to form films, biodegradability, gastrointestinal tract resistance, viscosity, solids content, hygroscopicity and cost, should all be taken into consideration when using different encapsulating agents, both individually and in combination.4 Bioactive substances have been encapsulated using a variety of techniques using food-grade polymers and proteins.10 Zein, a protein found in corn that is alcohol-soluble and high in prolamine, is one example. The amino acids of zein consist of one hydrophilic and three lipophilic components.11 It provides good biodegradability, mechanical properties, tolerance to high temperature, film formation capability, biological compatibility, and is a barrier towards moisture and oxygen, thereby making it useful for encapsulating lipophilic bioactive chemicals.12 The zein protein is stable for up to 120 min in a simulated stomach environment, but becomes unstable in less than 30 s in a simulated intestinal environment. This demonstrates that zein would improve the bio-accessibility of the bioactive chemical by increasing its protection against the gastric environment and encouraging release of the compound only in intestinal fluid.13 Using a phase separation approach and drying in a rotary evaporator to create a powder,14 zein nanoparticles were used for encapsulating carotene, thereby achieving regulated administration and improved pharmacokinetic properties. Oil and surfactants have also been employed as carriers for lipophilic compounds in addition to zein.15
There have been studies on the microencapsulation of fruit and plant extracts that have been published. These studies discuss the antioxidant capacities,16,17 storage stability,16,17 and physico-chemical properties.16 A report on the physical characteristics of microencapsulated acerola pomace extracts has been published.18 The decision to opt for freeze drying in our study was driven by the specific characteristics and requirements of the encapsulation process. Freeze drying, or lyophilization, is known for its ability to preserve the integrity of sensitive bioactive compounds. Unlike spray drying, which involves exposure to higher temperatures, freeze drying occurs at lower temperatures, minimizing the risk of thermal degradation.19 This is particularly crucial when dealing with heat-sensitive microcapsules, as it helps maintain the structural and functional attributes of the encapsulated materials. Additionally, freeze drying often results in microcapsules with superior reconstitution properties.20 The freeze-drying process creates porous structures that facilitate rapid rehydration upon exposure to liquid, allowing for a quick release of the encapsulated contents. This property can be advantageous in applications where rapid dissolution or release of the encapsulated material is desirable. While we acknowledge the economic considerations associated with freeze drying, especially in comparison to the more cost-effective spray drying method, our choice was driven by the need to prioritize the preservation of the efficacy of the bioactive compound.20 Freeze-drying is the most common encapsulation process, not a sophisticated or advanced method, but a superior one;21 it is a good microencapsulation method for delicate bioactive substances because materials are not subjected to such high temperatures as when utilizing alternative methods.22,23 Additionally, due to the smaller number of processes involved, the freeze-drying technique is easier to employ than conventional techniques.
Till now, very little research has been published on the microencapsulation of the bioactive components extracted from haritaki pulp. Thus, the main aim of this study is to extract the bioactive compounds from haritaki pulp, using supercritical fluid extraction and to microencapsulate them using freeze drying, we then investigate the physical, thermal, and morphological features of the dried encapsulates.
2. Materials and methods
2.1. Material
Haritaki was collected from the horticulture section of Tezpur University, Assam, India. Chemicals of the analytical grade required for the current study were purchased from Himedia in Mumbai, India.
2.2. Extraction of bioactive compounds from haritaki
The fruit was cleaned, and the pulp and seed were separated. A laboratory tray dryer (Labotech, BDI-51, B. D. Instrumentation, Ambala, India) was used for drying the pulp at 40 °C. After this, the dried pulp was crushed and put through a 100-mesh screen before being sealed in polythene bags with an aluminium laminate until further use. The supercritical fluid extractor (SFE) vessel (Applied Separations, USA) was filled with 1 g of haritaki pulp powder, and the extracts were collected in glass tubes within a separator. According to the experimental design, the extraction was carried out under a variety of extraction circumstances. An ethanol and water mixture was utilized as the extraction co-solvent in a 1
:
1 ratio. Carbon dioxide was pressurized using a high-pressure pump and then charged at the necessary pressure into the extraction vessel. In accordance with the experimental design, the extraction vessel holding the sample was allowed to heat in the oven, and a thermocouple was used to track the temperature. The extract was left in a glass vial after the dissolved chemicals in supercritical CO2 were run through a heated micrometer valve that was inflated at room temperature and pressure. For every extraction experiment, a fixed flow rate of CO2 (5 mL min−1) was fed into the extractor. The flow rate was consistently maintained using the SFE's built-in flow meter system. The optimized conditions used in the SFE were flow rate 3.34 (mL min−1), pressure 166.94 (bar), temperature 51.97 (°C), time 67.47 (min) and a CO2 mass flow rate of 5 kg h−1, kept constant for all the experiments. The extraction conditions of the phytochemicals were similar to our previous study.24
2.3. Encapsulation of bioactive compounds
2.3.1. Alginate encapsulation.
Through the use of an encapsulator, sodium alginate was used to encapsulate the extract (Buchi B-390, India). Using a magnetic stirrer, 4 g of sodium alginate was slowly added to distilled water (100 mL) and stirred (1500 rpm) until it was entirely dissolved. Then 10 mL of the extract was added, which was then extruded via an encapsulator (300 μm nozzle size) into a solution of 2% CaCl2, stirred continuously using a glass rod, and stored (4 °C) for further analysis.25
2.3.2. Freeze drying.
In order to produce the haritaki encapsulate using the advanced technique, different combinations of hydrocolloids were prepared to increase the yield and encapsulation of the bioactive constituents. Potato starch and zein were mixed in ratios of 0
:
100, 30
:
70, 50
:
50, 70
:
30, and 100
:
0 (w/w) in water and heated at 80 °C for 10 min on a hot-plate with a magnetic stirrer (AI-022, Alfa instruments, India) rotating at 560 rpm, after cooling we added 10 mL of the extract. The prepared mixture was kept in a freeze dryer (−86 °C, Lyolab, India) for 24 h at a pressure of 5 mm Hg. The resulting porous solids were pulverised using a mortar and pestle, put through a sieve with a mesh size of 25, and then immediately put into glass containers with screw-on lids and kept in a freezer (−18 °C).
2.4. Characterization of the physical properties of the encapsulates
2.4.1. Moisture content and water activity.
The AOAC 18th ed. technique was used to determine the moisture content of the freeze-dried powder (925.10). An electronic dew point water activity meter (Aqualab Series 4TE, Decagon Devices, Inc., Pullman, Washington, USA) was used to determine the water activity of the powder.
2.4.2. Process yield (PY).
The amount of powder collected after freeze drying compared to the original amount of feed solution, was used as the gravimetric measure of the process yield of the powder.26 |  | (1) |
2.4.3. Encapsulation efficiency.
The haritaki's encapsulation efficiency was determined by dividing the amount of encapsulated haritaki by the amount of total extract in the freeze-dried powder. Using the same organic solvent (petroleum ether), the surface extract was likewise extracted, however at room temperature as opposed to a higher temperature.27 Powdered haritaki (5 g) in capsules was steeped in 75.0 mL of petroleum ether (1
:
15 w/v, as in hot pentane Soxhlet extraction) in a conical flask. To help wash the powder from the oleoresin on the surface, the flask was occasionally swirled. Filtered petroleum ether was then mixed with an equivalent volume of new solvent, and the process was carried out three times. Petroleum ether was then collected, filtered, evaporated at 40 °C under vacuum, and finally, under a steady stream of nitrogen, until all of the remaining solvent was evaporated. Weighing the extractable surface haritaki (WSO), its percentage was also determined. The percentage was calculated using the mean and standard deviation of three determinations ±SD, |  | (2) |
where, EEL: the effectiveness of haritaki's encapsulation, WTO: weight of the total haritaki computed in a sample of powder (based on a 1
:
4 weight ratio of haritaki to powder in the initial formulation of the emulsion), WSO: weight of the extractable surface haritaki extract empirically discovered in the same weight of powder.
2.4.5. Colour properties.
Using Hunter Colour Lab, the colour of the fruit slices was assessed periodically as they dried (Hunter Associates Laboratory Inc., Reston, VA, USA). L* (lightness to darkness), a* (redness to greenness), and b* were used to express the colour values (yellowness to blueness).29 The total colour difference (ΔE*) was calculated using the following equation: | ΔE* = [(ΔL*)2 + (Δa*)2 + (Δb*)2]1/2. | (7) |
2.4.6. Fourier transform infrared spectroscopy (FTIR) spectra.
An FTIR spectrophotometer was used to record the FTIR spectra (Thermo Nicolet Model: 6700, UK). Before the measurement, the materials were mixed with KBr and formed into pellets. The apparatus was standardised using a KBr pellet as a blank, and the spectra were obtained in the 400–4000 cm−1 range.30
2.4.7. Surface morphology.
The surface morphologies of the encapsulates were examined using a scanning electron microscope (SEM) (JEOL JSM 6700F) at 500–2000× magnification.31
2.5. Thermodynamic properties
2.5.1. Differential scanning calorimetry.
Using differential scanning calorimetry (DSC TA-Q20), the thermal stability of the freeze-dried encapsulate was examined. To do this, water (14 mL) was poured with a micro syringe to the freeze-dried encapsulate (10 mg db) in the DSC pans, which were then sealed and reweighed. The samples were heated at a rate of 10 °C min−1 while being scanned from 35 °C to 150 °C with an empty aluminium pan serving as the reference. Using the software Universal Analysis 2000 3.9A, parameters including onset, peak, final, and gelatinization temperatures, were determined from the thermographs of the samples.32
2.5.2. Thermo-gravimetric analysis (TGA).
A Mettler-Toledo TGA/SDTA851E was used to calculate the mass loss of the freeze-dried encapsulation. The sample mass (6 mg) was kept constant during the test while temperatures between 30 °C and 500 °C were administered at a heating rate of 10 °C min−1 under nitrogen.32
2.6. Statistical analysis
In the present study, IBM SPSS Statistics Version 20.0, Armonk, NY: IBM Corporation package, was used for the statistical analysis of data, and the means were separated using Duncan's multiple range test (p < 0.05). All the data were presented as the mean with standard deviation.
3. Results and discussion
3.1. Encapsulation of bioactive compounds using conventional and advanced methods
The bioactive compounds from haritaki pulp powder were extracted using SFE and were encapsulated via conventional methods (alginate-matrix) and advanced methods (freeze-drying). The extracted bioactive compounds were already identified using LC-MS in one of our previous studies.33Fig. 1 and 2 show the encapsulates prepared from the different techniques. Freeze dried microcapsules were prepared using different ratios of potato starch and zein (0
:
100; 30
:
70; 50
:
50; 70
:
30; 100
:
0), where certain problems arose during the mixing of starch and zein powder due to their hydrophobic nature. The maximum 65.45% and 75.95% yield and encapsulation efficiency, respectively, were obtained in 100% starch; and the minimum 46.71% and 43.86% yield and encapsulation efficiency, respectively, were obtained in 100% zein.
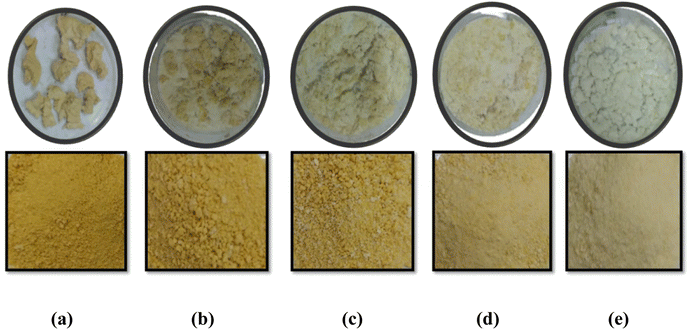 |
| Fig. 1 Haritaki extract encapsulates obtained from freeze-drying (a) starch : zein (0 : 100), (b) starch : zein (30 : 70), (c) starch : zein (50 : 50), (d) starch : zein (70 : 30), (e) starch : zein (100 : 0). | |
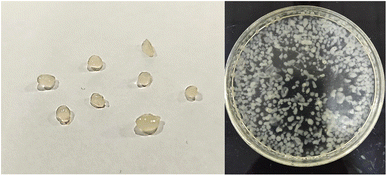 |
| Fig. 2 Encapsulation of bioactive compounds. | |
The zein protein does not form a homogenous mixture when heated with water along with the various proportions of starch. Although, it is been reported that zein is an excellent matrix to develop capsules, as well as providing improved bioavailability in simulated gastro-intestinal tracts. In the present investigation, it was observed that 100% zein did not produce good quality of phytochemical-loaded capsules while the 100% potato starch successfully produced a loaded capsule, which is attributed to the high solubility and biocompatible nature of starch. It has already been established that potato starch is an excellent binder, tablet excipient, disintegrant, matrix former and release retardant agent.34 We examined the phytochemical encapsulation effectiveness and yield of encapsulates made using both methods – alginate matrix and freeze-drying. Results show that compared to encapsulates made using the freeze-drying technique (65.45% and 75.95% yield and encapsulation efficiency, respectively), alginate encapsulated capsules had a lower yield (53.90%) and encapsulation efficiency (56.06%) of phytochemicals, when the ratio of starch and zein is (100
:
0). Thus, freeze dried encapsulates were selected for further characterization and to identify the physical, thermal, and morphological features.
Conventional techniques are the old techniques that do not use any advance pre-treatment instruments or strategies, whereas advanced techniques are modern techniques with improvised efficacy. Spray-drying and freeze-drying proved to be suitable processes to prepare ciriguela peel extract microcapsules to be used as a source of phenolic compounds for foods, such as drinks, cookies, bakery products, among others, as well as pharmaceuticals and cosmetics.35 LC-MS analysis showed the presence of a total of 36 compounds in the pulp extract which include chebulic acid, quercetin 3-O-glucuronide, methyl 2-furoate, 1,3,6-tri-O-galloylglucose, 2-hydroxychromene-2-carboxylate, 2,6-digalloylglucose, 8-hydroxyluteolin 8-glucoside 3′-sulfate, di-trans,poly-cis-decaprenyl diphosphate, methyl N-methylanthranilate, 5-aminoimidazole-4-carboxamide-1-β-D-ribofuranosyl 5′-monophosphate, (3β,19α)-3,19,23,24-tetrahydroxy-12-oleanen-28-oic acid, dihydrodeoxystreptomycin, and ellagic acid. According to our earlier research, these substances have biochemical characteristics that are crucial for the creation of polyherbal products and related items.33
3.2. Characterization of the physical properties of encapsulates
3.2.1. Process yield and encapsulation efficiency.
The maximum yield of the encapsulate was observed in the case of 100% starch followed by 70
:
30, 50
:
50, 30
:
70, and 0
:
100 starch
:
zein, respectively, as shown in Table 1. Thus, the encapsulates with 100% starch showed that the materials for the walls have good film-forming capabilities, ratios of 70
:
30, and 50
:
50 also present good interactions, better than 100% zein.36 As is reported, starch is a common material in pharmaceutical applications due to its biocompatibility and biodegradability, and the fact that it is cheap and available in large quantities. Besides, phytochemicals encapsulate with lower yield as the quantity of zein increases, suggesting that zein does not exhibit very good film-formation ability. The optimum is to use a low level of zein to encapsulate, improving the microbead properties;37 zein can form tough, glossy, greaseproof films resistant to microbial attack, with excellent flexibility and compressibility.
Table 1 Physical properties of freeze-dried encapsulatesa
Parameters |
Starch : zein (0 : 100) |
Starch : zein (30 : 70) |
Starch : zein (50 : 50) |
Starch : zein (70 : 30) |
Starch : zein (100 : 0) |
Values are means ± standard deviation of three determinations (n = 5). Values followed by a different superscript letter across a row are significantly different (p ≤ 0.05).
|
Bulk density (g cm−3) |
0.48 ± 0.01b |
0.29 ± 0.00d |
0.29 ± 0.00d |
0.34 ± 0.00c |
0.61 ± 0.01a |
Tapped density (g cm−3) |
0.51 ± 0.00b |
0.35 ± 0.00d |
0.35 ± 0.00d |
0.42 ± 0.00c |
0.72 ± 0.01a |
Carr's index (%) |
7.14 ± 2.15c |
17.55 ± 1.23ab |
15.88 ± 1.83ab |
18.47 ± 1.63a |
14.42 ± 1.68b |
Hausner ratio (%) |
1.07 ± 0.02c |
1.21 ± 0.01ab |
1.18 ± 0.02ab |
1.22 ± 0.02a |
1.16 ± 0.02b |
a
w
|
0.32 ± 0.01e |
0.50 ± 0.00a |
0.42 ± 0.01b |
0.40 ± 0.00c |
0.37 ± 0.01d |
Moisture (%) |
6.03 ± 0.06e |
6.44 ± 0.05c |
6.28 ± 0.04d |
6.85 ± 0.06b |
7.32 ± 0.04a |
Encapsulation efficiency (%) |
43.86 ± 2.72d |
51.50 ± 0.17c |
55.36 ± 1.80c |
63.42 ± 0.43b |
75.95 ± 0.88a |
Yield (%) |
46.71 ± 2.85d |
49.92 ± 0.29cd |
51.86 ± 1.66bc |
53.95 ± 0.29b |
65.45 ± 2.58a |
L* |
82.72 ± 0.96c |
85.39 ± 0.41b |
80.90 ± 1.67c |
76.44 ± 1.22d |
96.67 ± 1.09a |
a* |
2.01 ± 0.22c |
1.21 ± 0.13d |
2.91 ± 0.53b |
5.09 ± 0.58a |
−1.15 ± 0.31e |
b* |
24.49 ± 1.26c |
21.70 ± 0.35d |
28.43 ± 1.27b |
30.33 ± 0.86a |
6.96 ± 0.21e |
The efficacy of encapsulation varied between 43.86–75.95% showing a noticeable difference in the phytochemicals encapsulated within the zein and starch at ratios of 70
:
30, 50
:
50, 30
:
70, and 0
:
100. The maximum encapsulation was achieved with 100% starch indicating the excellent film forming properties of starch compared to zein. But the opposite result was obtained previously,38 where the authors encapsulated capsaicin through the use of whey protein and modified starch, and microcapsules with a higher percentage of modified starch in their composition attained lower encapsulation. These results were similar to those of encapsulation of phytosterol (efficiency 96.2%) obtained by spray drying, in which Gum Arabic and maltodextrin were used. Encapsulation efficiency relies on a few factors such as emulsion formulation and type of wall material.26 Modified native potato starch has been shown to be a better wall material than maltodextrin; both combine very well with tara gum in the microencapsulation of propolis and honey.39
3.2.2. Moisture content and water activity.
The moisture content of powder has a significant role in estimating flow behaviour, shelf stability and the cohesive nature.40 The moisture level of the capsules was between 6% and 7.32%, which is slightly greater than the 4% minimum requirement for powder used in the food business. Agglomeration lowers the scattering of active substances and prevents microbial development, microcapsules with lower humidity can result in the reduction of mildew degradation and moisture absorption, enhancing the physical and chemical stability. For dry powders, water activity (aw) is a quantitative index whose value is used to determine a food’s shelf life (Table 1). Water activity of the samples ranged from 0.32 to 0.50, indicating that the encapsulates will be stable for a longer period of time.41
3.2.3. Bulk and tapped density.
Bulk, tapped, and true density are the important characteristic features of powder. There was a significant difference (p < 0.05) in the encapsulated powder. The highest bulk density was shown in the ratio of 100
:
0 (starch
:
zein) while the lowest was observed in 30
:
70 (starch
:
zein) and 50
:
50 (starch
:
zein), see Table 1. Powders with higher bulk density require less volume for packaging and vice versa. Similar results were noticed in the tapped density of encapsulates. The tapped density of a powder represents its random dense packing. Tapped density values are higher for more regularly shaped particles (i.e., spheres), as compared to irregularly shaped particles.28
3.2.5. Colour properties.
Colour is an important parameter to assess the acceptability of powder. In the present study, colour parameters – L*, a* and b* values – were calculated for the freeze dried encapsulates and the results are summarized in Table 1. There was significant difference among the samples due to their different composition. The highest L* was recorded by the 100
:
0 (starch
:
zein) followed by 30
:
70 (starch
:
zein) and the lowest value was seen in the 70
:
30 (starch
:
zein). In the case of the a* value, the encapsulates produced by 70
:
30 (starch
:
zein) had the maximum followed by 50
:
50 (starch
:
zein) while the minimum value was recorded by 100% starch. The colour characteristics may have been significantly affected by the encapsulation produced vs. freeze drying, and variations were associated with the browning response and the repeat association of amylose at higher temperatures.43 Encapsulation of the extract using zein gives yellow and slight blue, because the encapsulated extract has a green and bluish appearance due to being rich in phytochemicals. On the other hand, the b* value shows a yellow to blue tinge, that is significantly affected by combination and freeze drying of the encapsulates. Encapsulates prepared in 70
:
30 (starch
:
zein) had a higher value while the minimum value was seen in 100% starch encapsulates.
3.2.6. Surface morphology of the powder.
The surface morphologies of the freeze dried encapsulates are presented in Fig. 3. It can be observed that, due to the partial collapse of the polymeric gel network, the encapsulates contained rough, uneven microparticles with heterogeneous surface morphologies that were indicative of materials that had been dried.44 The size of the microcapsules varied from 50 to 80 μm for all the samples. The encapsulation powder's uniformity of microstructure after freeze-drying, boosted its ability to protect the phenolic extractants.42 Similar results were reported in the microcapsules loaded with the phytochemicals of jabuticaba skin and seeds (Myrciaria cauliflora).45
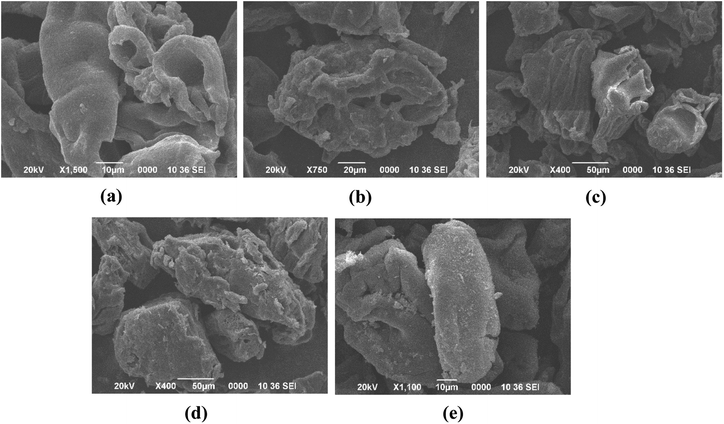 |
| Fig. 3 SEM images of haritaki encapsulates obtained from freeze-drying: (a) starch : zein (0 : 100), (b) starch : zein (30 : 70), (c) starch : zein (50 : 50), (d) starch : zein (70 : 30), (e) starch : zein (100 : 0). | |
3.2.7. FTIR spectra of the powder.
The FTIR spectra of native potato starch, which contains peaks at wave numbers 1459, 1379, 1339, 1260, 1193, 1066, 1022, 939, and 867 cm−1, shows the presence of amylose and amylopectin (Fig. 4). All of these peaks were caused by the presence of different bonds, such as C–H, C–O–C, and O–H, which were anticipated using an IR chart. Bands in encapsulated samples that included various zein and starch quantities showed a little shifting. N–H and C–N stretching represents the presence of amines, which also indicates the presence of various alkaloids (containing N). Zein and starch inclusion caused certain alterations that showed the presence of more amide bonds and OH groups in the insoluble fraction.46 The existence of polysaccharides and C–H bending, respectively, was suggested by peaks at 1459 and 1350 cm−1; and a vibration transmittance band between 1060 and 990 cm−1 highlighted47 strain deformations of the C–O–C and flexion of the OH. FTIR bond stretching of C–O–H, CH2OH represents the presence of polyphenols.47 The C–O–H deformation mode and CH2OH were both represented by the band at 1242 cm−1. On the other hand, the band at 1094 cm−1 displayed the C–O–H bending modes, while the peak at 1163 cm−1 appeared to be caused by the coupling of the C–O and C–C stretching modes. With all of the aforementioned, the CH2 bending mode would have formed at 1344 cm−1 for the IR bands.48 Others claim that the carbon and hydrogen atoms were discovered to be linked to the vibrational bands (bending and deformation) that appear in the range 1500–1300 cm−1.49 IR bands between 800–1200 cm−1, 1500–1200 cm−1, and 800–100 cm−1 were discovered. While bands in the range 1500–1200 cm−1 and 800–100 cm−1 were predominately dominated by the deformational modes of the CH/CH2 and CO groups, respectively, it was proposed that these bands represented the stretching vibrations of the distinctive C–O and C–C groups. Similar to this, all infrared spectra of the starch samples showed peaks at 995–965 cm−1 that looked to reflect
C–H bending, and peaks at 867 cm−1 that indicate the presence of certain di substituted, meta aromatic compounds with minor C–H bond stretching.50 The transmittance (%) for the encapsulated extract and haritaki extract in the FTIR were around 1 to 50 and 1 to 75, respectively, when compared with each other. When the pulp was microwave-treated, the distinctive broad peaks at 3351 to 3404 cm−1 attributed to the O–H stretching of the hydroxyl group were of low intensity. The band's intensity increases somewhat under ultrasound treatment, suggesting that the starch's microstructure has greater capacity to retain bound water due to ultrasonic vibrations. Enzymatic pulp treatment, on the other hand, has a wider peak than these two methods. The later peaks at 2100 to 2065 cm−1 are enhanced by ultrasonic treatment and are related to the C–H stretching of the glucose unit. The vibration of C–O stretching, C
O, C–O–H, C-1–H, and bending are attributed to the peaks at 1647 to 1652, 1385, 1218, and 1055 cm−1, respectively. Moreover, the band appearing at 923 to 936 cm−1 was attributed to anhydrous glucose ring stretching vibrations.33
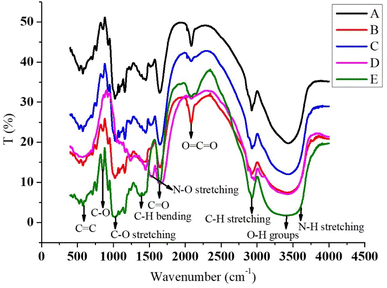 |
| Fig. 4 FTIR spectra of encapsulates: (A) starch : zein (0 : 100), (B) starch : zein (30 : 70), (C) starch : zein (50 : 50), (D) starch : zein (70 : 30), (E) starch : zein (100 : 0). | |
3.2.8. XRD.
The XRD spectra of the freeze-dried haritaki capsules are shown in Fig. 5. The samples obtained with starch
:
zein (50
:
50) showcased dense diffraction peaks at 17.5° and weaker ones between 19° to 25°. The ratio of starch
:
zein (0
:
100), starch
:
zein (30
:
70) and starch
:
zein (100
:
0) had a slightly intense diffraction at 16°, whereas the weak diffraction peak was observed in the ratio 70
:
30 of starch
:
zein. In general, zein levels in microcapsules decreased, and some peaks gradually vanished. Starch
:
zein microcapsules (50
:
50) only have a strong diffraction peak at 17.5°, indicating that the majority of the microcapsules were in an amorphous state. The amorphous state is often characterised by a higher water solubility. A similar result was previously obtained.38
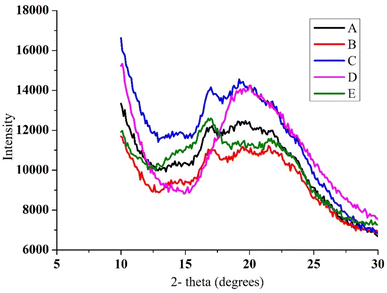 |
| Fig. 5 XRD pattern of encapsulates: (A) starch : zein (0 : 100), (B) starch : zein (30 : 70), (C) starch : zein (50 : 50), (D) starch : zein (70 : 30), (E) starch : zein (100 : 0). | |
3.3. Thermodynamic properties
3.3.1. DSC.
The DSC results revealed significant change in the thermal parameters such as onset temperature (To), peak temperature (Tp), and conclusion temperature (Tc) (Table 2 and Fig. 6). Encapsulates prepared using zein and starch in the various concentrations gelatinized at different temperatures. The onset temperature (To), peak temperature (Tp), and conclusion temperature (Tc) of 100% zein encapsulate was 81.80, 114.80 and 142.70 °C, respectively. The 100% starch encapsulate had a To, Tp, and Tc of 83.90, 117.70 and 142.50 °C, respectively. From this result it can be assumed that starch encapsulates required a higher temperature than zein encapsulates. These results are in agreement with previous work.42,51 Gelatinization temperatures (To, Tp and Tc) and enthalpies of starch from various sources including corn, rice, wheat, and potato have been found to be significantly different.52 The amounts of amylose and amylopectin, as well as the size, shape, and ratio of the starch granules, all influence the temperature at which gelatinization takes place.53 Numerous legume starches showed varying melting temperatures and a decrease in enthalpy of gelatinization upon the addition of hydrocolloids. The starch content, temperature at which the food is fully heated, and pace of amylopectin retrogradation are the factors that determine these parameters.
Table 2 Thermal properties and mass loss of different encapsulates
Parameters |
Starch : zein (0 : 100) |
Starch : zein (30 : 70) |
Starch : zein (50 : 50) |
Starch : zein (70 : 30) |
Starch : zein (100 : 0) |
Onset temperature To (°C) |
81.80 |
87.30 |
88.40 |
77.70 |
83.90 |
Peak temperature Tp (°C) |
114.80 |
117.30 |
120.40 |
107.00 |
117.70 |
Conclusion temperature Tc (°C) |
142.70 |
138.10 |
138.70 |
122.30 |
142.50 |
![[thin space (1/6-em)]](https://www.rsc.org/images/entities/char_2009.gif) |
Mass loss (%)
|
Decomposition (230–350 °C) |
58.63 |
62.06 |
62.82 |
68.12 |
67.23 |
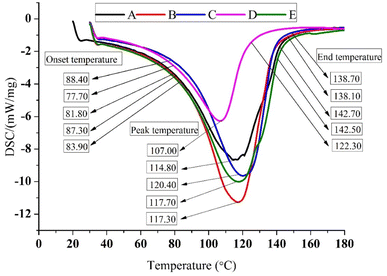 |
| Fig. 6 Thermal properties of different encapsulates: (A) starch : zein (0 : 100), (B) starch : zein (30 : 70), (C) starch : zein (50 : 50), (D) starch : zein (70 : 30), (E) starch : zein (100 : 0). | |
3.3.2. TGA.
Thermal deterioration is demonstrated via TGA by measuring mass loss as a function of temperature. With the temperature rising (from 230 to 350 °C), volatile chemicals in the encapsulates immediately began to evaporate, which is why the first weight loss was noticed (Table 2 and Fig. 7). During TGA, various temperature ranges had an impact on how various materials performed. The first mass loss did not vary significantly as encapsulate composition increased. Maximum mass loss was observed in 70
:
30 (starch
:
zein) encapsulates followed by 100% starch encapsulates. There were no significant differences recorded between the 30
:
70 (starch
:
zein) and 50
:
50 (starch
:
zein) encapsulates. Previous studies suggest that the first stage of weight loss is similar to the physical dehydration of starch, while the second stage entailed chemical and thermal degradation.54 At the third stage, chemical oxidation caused weight loss, which resulted in varied mass losses at temperatures between 300 and 400 °C. This stage, which is a combustion stage, is what completely oxidised the organic materials. At this point, a sharp loss in mass was seen as a result of the release of volatile compounds, this could have been caused by the release of water molecules, other tiny molecular species, the synthesis of additional carbonaceous residues, and heat condensation between the hydroxyl groups of the starch chains to create either segment.55 According to the results of the TGA on the encapsulates, thermal degradation took place between 230 and 350 °C. Depending on the concentration, the ratio of the starch's amylose and amylopectin content and their interaction with the protein zein, may result in varying heat stability and distinct disintegration mechanisms.56
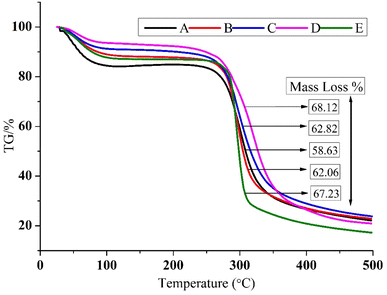 |
| Fig. 7 Mass loss of different encapsulates: (A) starch : zein (0 : 100), (B) starch : zein (30 : 70), (C) starch : zein (50 : 50), (D) starch : zein (70 : 30), (E) starch : zein (100 : 0). | |
4. Conclusion
Haritaki extract was successfully encapsulated using different combinations of starch and zein by means of freeze-drying.
Different combinations of starch
:
zein were tested for encapsulating the bioactive compounds, and 100% starch encapsulates presented better yield (65.45 ± 2.58), encapsulation efficiency (75.95 ± 0.88), powder bulk density (0.61 ± 0.01) and tapped density (0.72 ± 0.01), among the combinations. The combination of 70
:
30 (starch
:
zein) showed the largest mass loss (68.12%) using TGA analysis, whereas 100% zein (58.63%) showed the lowest mass loss. From the DSC curves, it was noticed that the maximum onset point (88.40) and peak point (120.40) were presented by 50
:
50 (starch
:
zein) encapsulates, while the minimum onset point (77.70) and peak point (107.00) were depicted by 70
:
30 (starch
:
zein) encapsulates. The endpoint was seen in 100% zein (142.70) and 100% starch encapsulates (142.50) whereas 70
:
30 (starch
:
zein) encapsulates (122.30) showed a minimum value. From the SEM study, it was verified that encapsulates had a rough and irregular shape. The TGA study revealed the maximum loss in mass in the 70
:
30 (starch
:
zein) combination while the minimum mass loss occurred in 100% zein. From the DSC curves, it was noticed that the maximum onset point and peak point were presented by 50
:
50 (starch
:
zein) encapsulates, while the minimum onset point and peak point were depicted by 70
:
30 (starch
:
zein) encapsulates. The maximum endpoint was seen in 100% starch encapsulates whereas 30
:
70 (starch
:
zein) encapsulates showed a minimum value. FTIR spectra revealed that 100% zein encapsulates had maximum stretching and vibrations in the bond while encapsulates prepared from 100% starch had minimum stretching with poor intensities. XRD patterns detailed no sharpness in the encapsulates, except for the 50
:
50 (starch
:
zein). The encapsulation process of haritaki was initiated by the chemical interaction between the composite wall materials (starch and zein) in an amorphous state, and was verified using FTIR and XRD. In future work, the thermal, pH and storage stabilities of the encapsulates should be tested, which will help in establishing the process for industry use. Haritaki could also be promoted as a viable source for bioactive compounds and functional ingredient in the clean-label food industry. Also, the encapsulates can be valorized for the development of functional products.
Data availability
The authors declare that data will be made available on request.
Author contributions
Avinash Kumar Jha: methodology, validation, formal analysis, investigation, writing – original draft; Nandan Sit: conceptualization, validation, writing – review and editing, visualization, supervision, project administration.
Conflicts of interest
The authors declare no conflict of interest.
Acknowledgements
The authors acknowledge the support received under projects UGC-SAP and DST-FIST from the Government of India through the Department of Food Engineering and Technology, Tezpur University. The authors would also like to express their sincere gratitude towards Tezpur University for providing a Research and Innovation grant (File no. TU/Fin/R/18-19/339) to carry out the project. Also, thanks to the Sophisticated Analytical Instruments Facility (SAIF), IIT Bombay, for providing LC-MS facilities, and the Sophisticated Analytical Instrument Center (SAIC), Tezpur University, for providing facilities for the characterization of the samples. The support received from CSIR-SRF (File no. 09/796(0113)2020/EMR-I) is duly acknowledged.
References
- A. Kumar, S. Kumar, A. Rai and B. Ram, Int. J. Pharm., Chem. Biol. Sci., 2017, 7(4), 381 CAS.
- K. M. Khazaei, S. M. Jafari, M. Ghorbani and A. H. Kakhki, Carbohydr. Polym., 2014, 105, 57–62, DOI:10.1016/j.carbpol.2014.01.042.
- L. S. Kuck and C. P. Z. Noreña, Food Chem., 2016, 194, 569–576, DOI:10.1016/j.foodchem.2015.08.066.
- P. I. Silva, P. C. Stringheta, R. F. Teófilo and I. R. N. de Oliveira, J. Food Eng., 2013, 117(4), 538–544, DOI:10.1016/j.jfoodeng.2012.08.039.
- S. S. Santos, L. M. Rodrigues, S. C. da Costa, R. D. C. Bergamasco and G. Madrona, Chem. Eng. Trans., 2017, 57, 1837–1842, DOI:10.3303/CET1757307.
- G. L. Zabot, F. Schaefer Rodrigues, L. Polano Ody, M. Vinícius Tres, E. Herrera, H. Palacin and L. Olivera-Montenegro, Polymers, 2022, 14(19), 4194, DOI:10.3390/polym14194194.
- S. M. T. Gharibzahedi and B. Smith, Compr. Rev. Food Sci. Food Saf., 2021, 20(2), 1250–1279, DOI:10.1111/1541-4337.12699.
- Q. Ye, N. Georges and C. Selomulya, Trends Food Sci. Technol., 2018, 78, 167–179, DOI:10.1016/j.tifs.2018.05.025.
- M. Sharma, K. K. Dash, L. S. Badwaik and G. V. S. Bhagya Raj, J. Food Process Eng., 2023, 46, e14447, DOI:10.1111/jfpe.14447.
- A. Alehosseini, B. Ghorani, M. Sarabi-Jamab and N. Tucker, Crit. Rev. Food Sci. Nutr., 2018, 58(14), 2346–2363, DOI:10.1080/10408398.2017.1323723.
- A. O. Elzoghby, W. M. Samy and N. A. Elgindy, J. Controlled Release, 2012, 161(1), 38–49, DOI:10.1016/j.jconrel.2012.04.036.
- V. A. Gaona-Sánchez, G. Calderón-Domínguez, E. Morales-Sánchez, J. J. Chanona-Pérez, G. Velázquez-de la Cruz and J. V. Méndez-Méndez, Food Hydrocolloids, 2015, 49, 1–10, DOI:10.1016/j.foodhyd.2015.03.003.
- T. J. Fu, U. R. Abbott and C. Hatzos, J. Agric. Food Chem., 2002, 50(24), 7154–7160, DOI:10.1021/jf020599h.
- A. Jain, G. Sharma, V. Kushwah, G. Ghoshal, A. Jain, B. Singh and O. P. Katare, Artif. Cells, Nanomed., Biotechnol., 2018, 46, 402–412, DOI:10.1080/21691401.2018.1428811.
- L. G. Gómez-Mascaraque, R. Perez-Masiá, R. González-Barrio, M. J. Periago and A. López-Rubio, Food Hydrocolloids, 2017, 73, 1–12, DOI:10.1016/j.foodhyd.2017.06.019.
- A. N. Negrão-Murakami, G. L. Nunes, S. S. Pinto, F. S. Murakami, E. R. Amante, J. C. C. Petrus and R. D. Amboni, LWT--Food Sci. Technol., 2017, 79, 561–567, DOI:10.1016/j.lwt.2016.11.002.
- G. L. Nunes, B. C. B. Boaventura, S. S. Pinto, S. Verruck, F. S. Murakami, E. S. Prudêncio and R. D. D. M. C. Amboni, J. Food Eng., 2015, 151, 60–68, DOI:10.1016/j.jfoodeng.2014.10.031.
- G. E. G. Moreira, M. G. M. Costa, A. C. R. de Souza, E. S. de Brito, M. D. F. D. de Medeiros and H. M. de Azeredo, LWT--Food Sci. Technol., 2009, 42(2), 641–645, DOI:10.1016/j.lwt.2008.07.008.
- D. Nowak and E. Jakubczyk, Foods, 2020, 9(10), 1488, DOI:10.3390/foods9101488.
- L. Pudziuvelyte, M. Marksa, K. Sosnowska, K. Winnicka, R. Morkuniene and J. Bernatoniene, Molecules, 2020, 25(9), 2237, DOI:10.3390/molecules25092237.
- L. Šturm, I. G. O. Črnivec, K. Istenič, A. Ota, P. Megušar, A. Slukan and N. P. Ulrih, Food Bioprod. Process., 2019, 116, 196–211, DOI:10.1016/j.fbp.2019.05.008.
- K. Papoutsis, J. B. Golding, Q. Vuong, P. Pristijono, C. E. Stathopoulos, C. J. Scarlett and M. Bowyer, Foods, 2018, 7(7), 115, DOI:10.3390/foods7070115.
- A. Rezvankhah, Z. Emam-Djomeh and G. Askari, Drying Technol., 2020, 38(1–2), 235–258, DOI:10.1080/07373937.2019.1653906.
- A. K. Jha and N. Sit, Ind. Crops Prod., 2021, 170, 113769, DOI:10.1016/j.indcrop.2021.113769.
- A. K. Gupta, M. Yumnam, M. Medhi, P. Koch, S. Chakraborty and P. Mishra, J. Food Process. Preserv., 2021, 45(5), e15380, DOI:10.1111/jfpp.15380.
- A. E. Edris, D. Kalemba, J. Adamiec and M. Piątkowski, Food Chem., 2016, 204, 326–333, DOI:10.1016/j.foodchem.2016.02.143.
- D. A. Botrel, R. V. de Barros Fernandes, S. V. Borges and M. I. Yoshida, Food Res. Int., 2014, 62, 344–352, DOI:10.1016/j.foodres.2014.02.003.
- V. Kumar, A. K. Gupta, B. Naik and H. A. Makroo, Int. J. Biol. Macromol., 2023, 235, 123898, DOI:10.1016/j.ijbiomac.2023.123898.
- D. Das, D. Das, A. K. Gupta and P. Mishra, Acta Aliment., 2020, 49(3), 295–306, DOI:10.1556/066.2020.49.3.8.
- M. Bashir and S. Haripriya, Int. J. Biol. Macromol., 2016, 93, 476–482, DOI:10.1016/j.ijbiomac.2016.09.009.
- A. K. Gupta, P. Mishra, M. Senapati and P. P. Sahu, J. Food Eng., 2021, 306, 110637, DOI:10.1016/j.jfoodeng.2021.110637.
- A. K. Jha, S. Kumari, A. K. Gupta and A. Shashank, J. Food Process. Preserv., 2021, 45(10), e15829, DOI:10.1111/jfpp.15829.
- A. K. Jha and N. Sit, Sustainable Chem. Pharm., 2023, 33, 101098, DOI:10.1016/j.scp.2023.101098.
-
A. K. Nayak, M. S. Hasnain, A. K. Dhara and S. C. Mandal, Herbal Biomolecules in Healthcare Applications, 2022, pp. 613–642, DOI:10.1016/B978-0-323-85852-6.00011-1.
- M. E. da Silva Júnior, M. V. R. L. Araújo, A. C. S. Martins, M. dos Santos Lima, F. L. H. da Silva, A. Converti and M. I. S. Maciel, Sci. Rep., 2023, 13(1), 15222, DOI:10.1038/s41598-023-40390-4.
- M. V. Álvarez-Henao, N. Saavedra, S. Medina, C. J. Cartagena, L. M. Alzate and J. Londoño-Londoño, Food Chem., 2018, 256, 181–187, DOI:10.1016/j.foodchem.2018.02.059.
- G. Reineccius and Y. Meng, Microencapsulation Food Ind., 2023, 293–308, DOI:10.1016/B978-0-12-821683-5.00021-2.
- T. Zhang, S. Yu, X. Tang, C. Ai, H. Chen, J. Lin and X. Guo, Food Hydrocolloids, 2022, 124, 107208, DOI:10.1016/j.foodhyd.2021.107208.
- C. A. Ligarda-Samanez, D. Choque-Quispe, E. Moscoso-Moscoso, M. L. Huamán-Carrión, B. S. Ramos-Pacheco, G. De la Cruz and R. Sucari-León, Foods, 2023, 12(9), 1873, DOI:10.3390/foods12091873.
- S. Santhalakshmy, S. J. D. Bosco, S. Francis and M. Sabeena, Powder Technol., 2015, 274, 37–43, DOI:10.1016/j.powtec.2015.01.016.
- P. Susantikarn and N. Donlao, Int. Food Res. J., 2016, 23(3), 1327 CAS.
- H. Pashazadeh, O. Zannou, M. Ghellam, I. Koca, C. M. Galanakis and T. M. Aldawoud, Foods, 2021, 10(6), 1396, DOI:10.3390/foods10061396.
- K. S. Pramodrao and C. S. Riar, Food Hydrocolloids, 2014, 35, 613–619, DOI:10.1016/j.foodhyd.2013.08.006.
- N. D. A. Arriola, P. M. de Medeiros, E. S. Prudencio, C. M. O. Müller and R. D. M. C. Amboni, Food Biosci., 2016, 13, 32–40, DOI:10.1016/j.fbio.2015.12.001.
- D. D. C. S. Mendes, E. R. Asquieri, R. D. Batista, C. C. de Morais, D. P. R. Ascheri, I. Y. L. de Macêdo and E. de Souza Gil, LWT--Food Sci. Technol., 2021, 137, 110460, DOI:10.1016/j.lwt.2020.110460.
- A. Rezaei, A. A. Nasirpour and H. Tavanai, Food Hydrocolloids, 2016, 60, 461–469, DOI:10.1016/j.foodhyd.2016.04.027.
- J. Ferreira-Villadiego, J. Garcia-Echeverri, M. V. Mejia, J. Pasqualino, P. Meza-Catellar and H. Lambis, Chem. Eng. Trans., 2018, 65, 763–768, DOI:10.3303/CET1865128.
- J. J. Cael, J. L. Koenig and J. Blackwell, Biopolymers, 1975, 14(9), 1885–1903, DOI:10.1002/bip.1975.360140909.
- K. C. Schuster, H. Ehmoser, J. R. Gapes and B. Lendl, Vib. Spectrosc., 2000, 22(1–2), 181–190, DOI:10.1016/S0924-2031(99)00080-6.
-
J. R. Mohrig, C. N. Hammond and P. F. Schatz, Techniques in Organic Chemistry, Macmillan, 2010 Search PubMed.
- M. Irani, G. M. M. Sadeghi and I. Haririan, Int. J. Biol. Macromol., 2017, 97, 744–751, DOI:10.1016/j.ijbiomac.2017.01.073.
- N. Singh, J. Singh, L. Kaur, N. S. Sodhi and B. S. Gill, Food Chem., 2003, 81(2), 219–231, DOI:10.1016/S0308-8146(02)00416-8.
- P. R. Chang, R. Jian, P. Zheng, J. Yu and X. Ma, Carbohydr. Polym., 2010, 79(2), 301–305, DOI:10.1016/j.carbpol.2009.08.007.
- L. A. Acevedo, N. E. Korson, J. M. Williams and L. K. Nicholson, J. Biomol. NMR, 2019, 73(8), 493–507, DOI:10.1007/s10858-019-00258-0.
- P. Malumba, M. D. Bungu, K. J. Katanga, L. Doran, S. Danthine and F. Béra, Food Chem., 2016, 212, 305–312, DOI:10.1016/j.foodchem.2016.05.181.
- R. M. D. Soares, A. M. F. Lima, R. V. B. Oliveira, A. T. N. Pires and V. Soldi, Polym. Degrad. Stab., 2005, 90(3), 449–454, DOI:10.1016/j.polymdegradstab.2005.04.007.
|
This journal is © The Royal Society of Chemistry 2024 |