Effect of ZnO nanoparticle application on crop safety and soil environment: a case study of potato planting†
Received
25th September 2023
, Accepted 4th December 2023
First published on 18th December 2023
Abstract
The recent use of ZnO nanoparticles (NPs) in agriculture has gained significant traction. However, the understanding of crop safety and environmental risk posed by ZnO NP exposure remains limited. As a result, this study administered varying concentrations of ZnO NPs (0, 10, 50, 200, 500, and 800 mg kg−1) to potato plants to determine their effect on food and soil quality. The results showed that while the number of tubers decreased, ZnO NPs enhanced the potato tuber quality and commodity rate and significantly increased Zn concentrations within various tissues. X-ray diffraction analysis showed that ZnO NPs at 50 mg kg−1 did not penetrate the potato plants. From a soil environment viewpoint, higher ZnO NP concentrations significantly changed the physical and chemical properties of the soil but not the dominant groups of soil microbial communities. However, noticeable shifts in the relative proportions of these communities occurred. This study is the first to explore the biofortification efficiency and possible environmental risks of ZnO NPs on potato plants, providing a theoretical basis for the safe application of ZnO NPs in agriculture.
Environmental significance
ZnO nanoparticles are the second most produced nanoparticles in the world. Research studies show that more than 70% of the nanoparticles in the environment will eventually accumulate in the soil. A large number of accumulated ZnO nanoparticles may harm the ecosystem and cause nano-specific risks. This study used XRD to determine that ZnO nanoparticles could not enter potato plants, and the potato commodity rate and changes in the community structure of soil bacteria and fungi were studied. An essential assessment of the nano-specific toxicity and environmental risks of ZnO nanoparticles was carried out.
|
1. Introduction
Nanoparticles (NPs) have distinctive properties and advantages that set them apart from traditional particles, leading to their widespread use in various fields (e.g., commercial, biological, cosmetics, and pharmaceutical products).1 Among metallic NPs, ZnO NPs exhibit unique physical and chemical attributes, including high specific surface area, antibacterial activity, and biocompatibility.2,3 In light of the escalating global population and consequent increased food demand, micronutrient deficiencies have surfaced. The slow-release effect of ZnO NPs has the potential to meet Zn requirements at various crop growth stages. Thus, they are used in agricultural production to increase Zn.4,5 Studies have shown that ZnO NPs increased Zn concentrations in soybean and corn,6,7 exhibiting superior Zn enhancement compared to ZnSO4.8 Moreover, ZnO NPs promote plant growth, increasing crop yields.9
The rapid development of nanotechnology has accelerated the production and application of NPs, resulting in their continuous release into the natural environment during manufacturing and application. This exposure to water, atmosphere, and soil can have direct or indirect favorable or adverse effects. As emerging products, ZnO NPs boast potential annual global production estimates in the range of 550–33
400 tons,10 ranking second to TiO2 NPs in environmental presence.11 Compared with atmospheric and aquatic ecosystems, soil is the primary repository for NPs.12 Elevated soil NP concentrations can affect ecosystem functions across multiple levels, influencing plants, soil microorganisms, and soil-dwelling animals. While earlier studies focused on the positive aspects of ZnO NPs, research on nanotoxicology has grown exponentially in recent years. However, information on the fate and toxicity of ZnO NPs in the environment remains limited.13 Thus, the effects of ZnO NPs on plants and the soil environment should be explored.
Soil microorganisms play an essential role in the soil environment, providing ecosystem services, such as nutrient mineralization and recycling, regulating the formation of biochemical cycles and soil structure, and participating in the decomposition of soil, animal and plant residues, organic matter, and other harmful compounds.14,15 The infiltration of NPs, such as TiO2, CeO2, Ag, CuO, and Fe3O4, into the soil has the potential to disrupt soil microbial communities and endanger soil ecosystem health and function.16,17 Research has shown that ZnO NPs reduce the soil microbial abundance, change the bacterial community structure, and compromise the soil health.18
Potato is the fourth largest food crop globally, with China contributing around 0.99 × 108 tons, accounting for 26% of the global annual production and ranking first worldwide.19 In 2015, the Ministry of Agriculture proposed the Potato Staple Food Strategy, promoting the nutritional significance of potatoes in China's diet. During potato tuber growth, direct interaction with ZnO NPs occurs in the soil, but whether NPs enter potato tubers requires further research. In addition, few studies have investigated the effects of ZnO NPs on soil microorganisms in potato cultivation.
Inadequate Zn availability in soil significantly affects crop growth and yield.20 The current approach to alleviating human Zn deficiency revolves around increasing the available soil Zn, thereby enhancing the Zn concentration in edible crop parts.21 Consequently, the use of ZnO NPs as novel Zn fertilizers has become a focal point of research. However, the distinct properties of nanomaterials could pose environmental risks compared to traditional ions. When released into the environment, NPs can alter the microbial diversity and plant growth.22 Given that soil is the principal NP reservoir, it is vulnerable to heightened environmental risks. Therefore, this experiment uses higher ZnO NP concentrations than those typically present in the current soil environment, considering the potential accumulation and spills of these particles.23 This study uses ZnO NPs as Zn sources to explore their effects on the potato tuber yield, quality, and Zn absorption in a pot experiment. Moreover, considering the potential health and environmental risks posed by NPs, the study explores whether ZnO NPs can be absorbed by tubers in particle form, and their potential ramifications on crop nutrient absorption and soil microorganisms.
2. Material and methods
2.1. NPs and soil substrate
ZnO NPs were purchased from Hongsheng Material Science and Technology Co. (China), and were described by the manufacturer as having 99.6% purity and an average diameter of <100 nm. Transmission electron microscopy (TEM, JEOL 100CX, Japan) was undertaken to confirm the morphology of ZnO NPs. The TEM results showed that the NPs easily agglomerated and had a near elliptical shape (Fig. S1, ESI†). The average size of the ZnO NPs was approximately 20 ± 5 nm, which is consistent with the manufacturer's data.
The soil was sourced from Yujiagong Village, Yongshou County, Shaanxi Province, China. The soil samples, collected from the upper 0–20 cm depth, were air-dried and crushed. After passing through a 2 mm sieve, the soil samples were mixed uniformly before laboratory analyses. The soil characteristics included pH 8.02, 8780 mg kg−1 soil organic matter, 49 mg kg−1 total N, 18.8 mg kg−1 available P, 160.29 mg kg−1 available K, and 0.54 mg kg−1 DTPA-Zn. A 5
:
1 soil
:
vermiculite ratio (v/v) was selected to enhance the soil gas permeability and prevent soil compaction.
2.2. Experimental design and NPs addition to the soil substrate
The experimental design comprised six treatments: (1) no Zn (CK), (2) 10 mg kg−1 ZnO NPs (ZnO10), (3) 50 mg kg−1 ZnO NPs (ZnO50), (4) 200 mg kg−1 ZnO NPs (ZnO200), (5) 500 mg kg−1 ZnO NPs (ZnO500), and (6) 800 mg kg−1 ZnO NPs (ZnO800). The treatments were based on pure Zn concentrations. Before sowing, the Zn fertilizer was mixed thoroughly with the soil. Urea, potassium dihydrogen phosphate, and potassium sulfate were added to the test soil to achieve the desired nutrient levels (200.01 mg kg−1 N, 99.99 mg kg−1 P2O5, and 240 mg kg−1 K2O). Each pot (cylindrical, diameter 32 cm, height 30 cm) was filled with 9.25 kg of mixed soil, with the potato tubers planted after 48 h of watering.
Potato tubers (Solanum tuberosum L. cv. Marco) with a smooth appearance, no disease, no deformity, and uniform quality (45.0 ± 3.0 g) were selected and germinated for a week at room temperature. One pre-germinated potato tuber was placed in each pot with the buds facing up. The pots were weighed and watered daily to maintain the water level at 60% of field capacity for 95 days until harvest.
2.3. Sample analyses
Harvested potato plants were divided into roots, stems, leaves, stolons, and tubers. The potato surface was washed with 0.1% HNO3 before recording the total number of tubers per pot and the fresh weight of each tuber. The plant weight, height (determined with a tape measure), and stem thickness (determined with a vernier caliper) were recorded. The tubers were categorized into small, medium, and large sizes using the classification method outlined by Chen et al.24 The commodity rate denotes the proportion of the combined weight of large and medium tubers to the total tuber weight. The various potato parts were oven-dried at 80 °C until a constant weight was achieved to record the dry weights.
After digestion with concentrated nitric acid and hydrogen peroxide, the total nitrogen amount was measured using a flow analyzer, the total phosphorus amount was measured using an ultraviolet spectrophotometer, and the total potassium amount was measured using a graphite furnace atomic absorption spectrometer. After microwave digestion with concentrated nitric acid, the iron, manganese, copper, and Zn concentrations were measured using a graphite furnace atomic absorption spectrometer. Starch concentration was determined using iodine–potassium iodide colorimetry,25 the reducing sugars and soluble sugar concentrations were measured using the dinitrosalicylic acid (DNS) colorimetric method,26 and the soluble protein concentrations were measured using the Coomassie brilliant blue method.27
X-ray diffraction analysis was conducted on individual sampled potato components (roots, stems, leaves, stolons, and tubers) using a diffractometer (D8 ADVANCE A25, Bruker) equipped with a Johansson-focused Ge primary monochromator and a LynxEye linear silicon strip detector in reflective mode. Each treatment had six replicates to enhance accuracy and sensitivity. Data collection involved a step size of 0.02° and exposure time of 12 s per point within a 2θ range spanning 3° to 80°.
After natural drying, the soil samples were analyzed for various parameters: pH, total organic carbon (TOC) (potassium dichromate oxidation spectrophotometric method), total N (TN) (Kjeldahl method), nitrate-N and ammonia-N (continuous flow analysis), available P (Mo–Sb anti spectrophotometric method), available K and metal elements (atomic absorption spectrophotometry).
In addition, microbial sequencing was conducted on a portion of the treated soil using a FastDNA kit (MP Biomedicals LLC, Ohio, USA) to extract DNA from 0.5 g soil samples. The DNA fragments were recovered from the agarose gel using a Geneclean UMIQ-10 kit (Shanghai BIOZERON Biotechnology Co. Ltd, Shanghai, China) and stored at 20 °C. The bacterial primers, 338F (5′-ACTCCTACGGGAGGCAGCA-3′) and 806R (5′-GGACTACHVGGGTWTCTAA-3′), were used to amplify the bacterial 16S rRNA V3–V4 region gene sequence. The fungus amplifies the genes in the ITS1 region based on the primers used in the study, ITS1F (5′-CTTGGTCATTTAGAGGAAGTAA-3′) and ITS2 (5′-GCTGCGTTCTTCATCGATGC-3′).28 PCR amplification was completed at Beijing Biomarker Technologies (Beijing, China). The raw tags were obtained using FLASH v1.2.7 software to merge the reads of each sample through the paired-end sequence data obtained by Hiseq sequencing. Trimmomatic v0.33 was used to filter the spliced raw tags to obtain clean tags. Effective tags were screened using UCHIME v4.2, with the chimera sequence identified and removed to obtain the final valid data.
2.4. Statistical analyses
Network analysis was performed using R studio and Gephi (version 0.9.1) based on Spearman's correlation coefficients (P < 0.05) for analysis and visualization of soil microbes and potato growth indicators. The mineral composition in various organs of wheat was identified from the XRD spectra using Jade 6.5 (Materials Data Inc., California). Except for the XRD test being repeated six times, all treatments were repeated three times, with the obtained data analyzed using SPSS to determine significant differences between treatments (P < 0.05) and for correlation analysis.
3. Results
3.1. Effect of Zn application on the growth phenotype of potato
The potato plant height and leaf dry weight were increased in the low-dose ZnO NP (ZnO200) treatment by 23.11% and 19.90%, respectively, relative to the control, while the high-dose ZnO NP (ZnO500) treatment decreased these parameters. The ZnO NP treatments had a slight inhibitory effect on the stem dry weight and total tuber weight.
Generally, the ZnO NP application reduced total biomass and tuber number per plant, but increased the average single tuber weight (Fig. 1b). The ZnO800 treatment recorded the lowest average number of tubers per plant and total tuber biomass, but the highest average single tuber weight, which was 56.61% higher than the control. Based on the tuber size and weight, the harvested tubers were categorized as large, medium, and small tubers (Fig. 1c). All treatments produced more large and fewer small tubers, with the ZnO200 treatment having the most favorable outcome. Thus, the ZnO NP treatments positively affected the commodity rate of the potatoes, but the high ZnO NP concentrations were not as advantageous as the low ZnO NP concentrations.
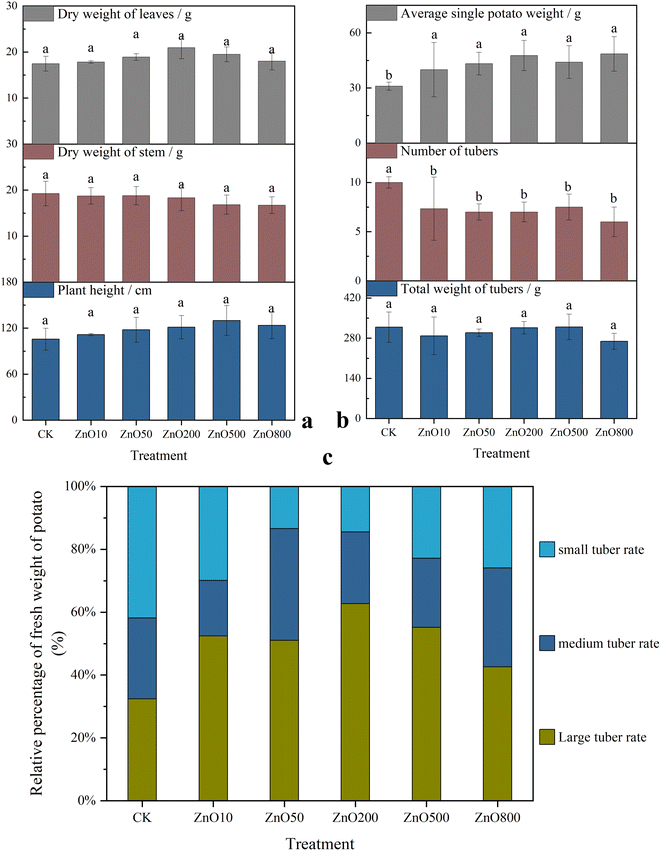 |
| Fig. 1 Effects of ZnO NP treatments on the potato leaf dry weight, stem dry weight, plant height (a), total weight of tubers, number of tubers, average tuber weight (b), and the relative percentage of potato weight (c). Large, medium, and small tuber rates refer to the number of single potato weights ≥40.00 g, 20.00–40.00 g, and ≤20.00 g to the total number of tubers in the treatment, respectively. Bars with different lowercase letters indicate significant differences between different treatments for the same tissue (P < 0.05). | |
3.2. Effects on the elemental concentrations, Zn speciation, and tuber quality
Analyzing the effect of the ZnO NP treatments on the nutrient element concentrations in potato tubers revealed some fluctuations in the N, K, Fe, and Mn concentrations (Table S1†), but they did not significantly differ from the control. The ZnO800 treatment significantly decreased the P and Cu concentrations in the potato tubers, suggesting that high ZnO NP concentrations inhibited the nutrient absorption of potato tubers. The low-concentration ZnO NP treatments had higher N, P, and Cu concentrations in the tubers than CK.
All ZnO NP treatments increased the Zn concentrations in potato tissues to a certain extent, following the order: stem > stolon > root > leaf > tuber (Fig. 2b). The Zn concentration in each part demonstrated a dose-dependent relationship with the ZnO NP treatments. Compared with CK, the Zn content of the potato roots, stems, leaves, stolons, and tubers increased by 107%, 215%, 39%, 162%, and 62% in the Zn10 treatment, 120%, 557%, 91%, 391%, and 82% in the Zn50 treatment, 370%, 1105%, 157%, 601%, and 111% in Zn200 treatment, and 859%, 1730%, 190%, 1036%, and 147% in the Zn500 treatment, respectively. The ZnO800 treatment had the highest Zn concentrations for each plant part, increasing by 1915%, 2140%, 514%, 1970%, and 149% in the roots, stems, leaves, stolons, and tubers, respectively, compared to CK. The X-ray diffraction (XRD) scans of the root, stem, leaf, stolon, and tuber powder of potato in the ZnO50 treatment were compared with those obtained using ZnO NPs as a model compound (Fig. 2a). The spectra of each treatment were matched to the standard cards of ZnO, and peak search, and the fitting and calibration were performed using Jade 6.5 software. The results show that ZnO NP matches the wurtzite hexagonal crystal structure of the ZnO standard (JCPDS 36-1451), and its average grain size is 22.15 nm. Notably, the presence of at least three typical ZnO crystal surface peaks was evident in the model compound, but absent in the potato samples. This observation indicates the absence of ZnO NPs in potato tissues, particularly edible parts.
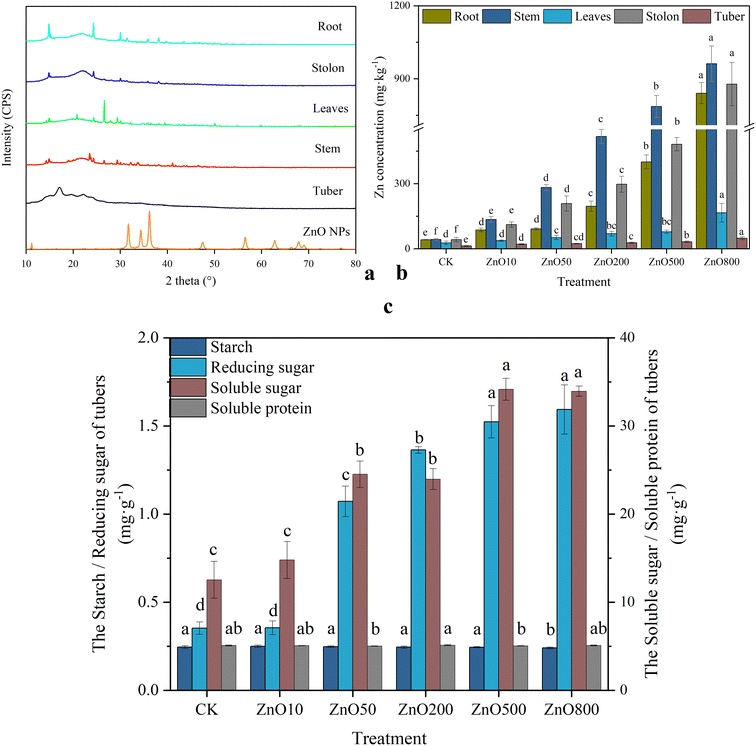 |
| Fig. 2 XRD patterns of Zn speciation of potato tissues treated with ZnO NPs at 50 mg kg−1 (a), Zn distribution in potato plants under different ZnO NP treatments (recorded as Zn accumulation) (b), and starch, reducing sugar, soluble sugar, and soluble protein concentrations in tubers under different ZnO NP treatments (c). Bars with different lowercase letters indicate significant differences between different treatments for the same tissue (P < 0.05). | |
The Zn bioaccumulation capacity of potatoes was assessed using the bioconcentration factor (BCF), with the ability of Zn to transport from belowground to aboveground evaluated using the translocation factor (TF) (Table S2†). All treatments increased the Zn translocation from the roots to stems and from belowground to aboveground parts, but inhibited Zn transfer from roots to tubers. This inhibition could be attributed to ZnO NPs enhancing the biomass of individual potato tubers, leading to a dilution effect that reduced the Zn concentrations in tubers.
The potato tuber quality was assessed by evaluating the starch, reducing sugar, soluble sugar, and soluble protein concentrations (Fig. 2c). Compared with CK, the ZnO NP treatments did not significantly affect the tuber starch and soluble protein concentrations. However, the concentrations of reducing sugars and soluble sugars were notably influenced by Zn application, exhibiting similar concentration trends that were dependent on the ZnO NP concentrations.
3.3. Effects of Zn application on the soil physiochemical properties
Fig. 3 presents changes in the fundamental soil physical and chemical properties. The ZnO800 treatment significantly increased the soil pH, while other treatments did not significantly differ from the control (Fig. 3a). Soil TOC concentration decreased significantly with increasing Zn concentration, with the lowest values in the ZnO800 treatment, 13.33% lower than CK. All ZnO NP treatments decreased soil ammonia-N and nitrate-N concentrations to varying degrees, but soil TN did not significantly change (Fig. 3b). Moreover, the ZnO NP application significantly increased the soil available P concentrations (Fig. 3c), with the highest value in the ZnO500 treatment, 1.25 times higher than CK. The ZnO NP treatments also had a significant dose-effect relationship with soil Zn and DTPA-Zn contents (Fig. 3d).
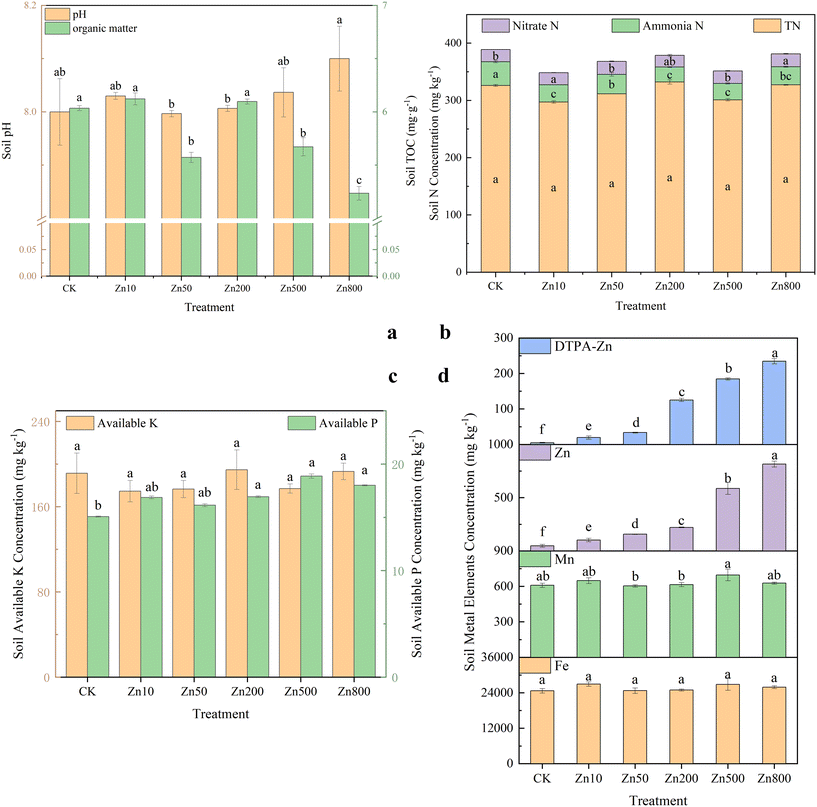 |
| Fig. 3 Soil pH (a), TN, nitrate-N and ammonia-N (b), available P and available K (c), and elemental (d) under different ZnO NP treatments. Bars with different lowercase letters indicate significant differences between different treatments of the same tissue (P < 0.05). | |
3.4. Effects of NPs on the microbial community structure in potato rhizosphere soil
The α-diversity was evaluated to reflect the impact of ZnO NPs on the microbial abundance and species diversity of the potato rhizosphere soil (Table 1). The ZnO50 treatment significantly increased the number of OTUs and enhanced the species diversity for bacteria. However, the remaining ZnO NP treatments decreased the diversity of the soil microbial species. The fungal abundance in soil is generally lower than that of bacteria. Low concentrations of ZnO NPs decreased the number of fungal species and species diversity, but significantly increased the number of OTUs and fungal abundance. Consequently, ZnO NP treatments can reduce the fungal diversity.
Table 1 Bacterial and fungal diversity in potato-cultivated soils
|
Treatment |
OTU number |
Chao 1 |
Shannon |
Simpson |
Note: different lowercase letters indicate significant differences between treatments (P < 0.05). |
Bacterial diversity |
CK |
1750a |
1728a |
6.44b |
0.0046b |
ZnO50 |
1756a |
1724a |
6.48a |
0.0044b |
ZnO800 |
1666b |
1633b |
5.93c |
0.0093a |
Fungi diversity |
CK |
662b |
688b |
5.18a |
0.0190c |
ZnO50 |
686ab |
718a |
4.48b |
0.0513a |
ZnO800 |
719a |
729a |
4.78b |
0.0395b |
The bacterial community groups at the genus level exhibited similarity across treatments (Fig. 4a). However, the ZnO NP treatments changed the relative abundance of bacteria, increasing the relative abundance of Sphingomonas, Subgroup_6, Subgroup_7, and Microscillaceae and decreasing the relative abundance of Gemmatimonadaceae compared to CK. The ZnO NP treatments did not significantly affect the dominant groups of fungal communities at the genus level (Fig. 4b). The control group had a higher percentage of unclassified fungi than the ZnO NP treatments, indicating that ZnO NPs reduced fungal diversity, possibly due to the stress of ZnO NPs on sensitive bacteria. Chaetomium and Cladosporium were the dominant groups. Unlike bacteria, the high ZnO NP concentration did not significantly affect the fungal community structure.
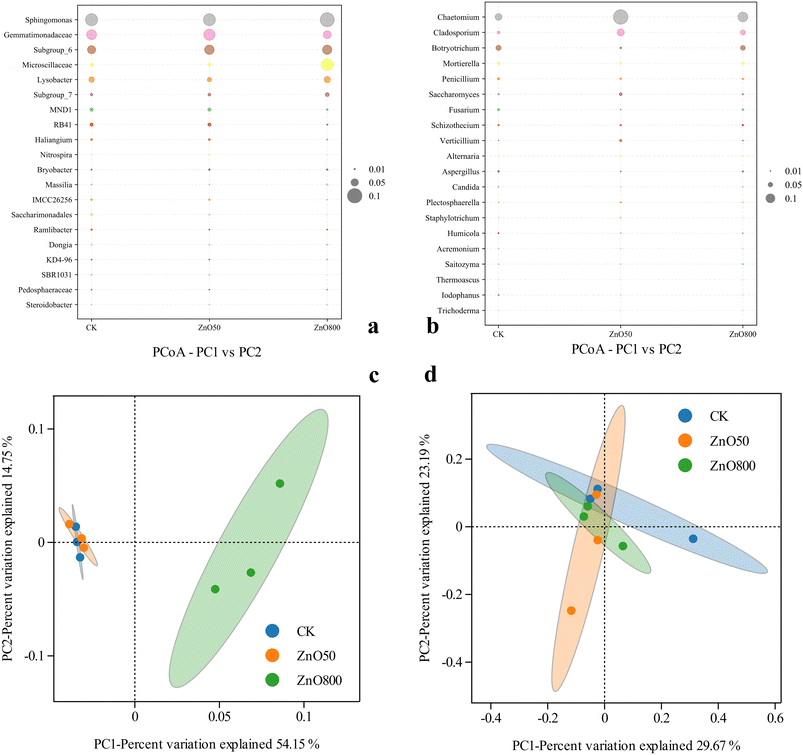 |
| Fig. 4 Bubble chart of the species distribution at the genus level of soil bacteria (a) and fungi (b), and principal coordinate analysis (PCoA) of potato-cultivated soil bacteria (c) and fungi (d). | |
Principal coordinate analysis (PCoA) was conducted at the genus level using Bray–Curtis dissimilarity to visualize the results (Fig. 4c). The soil bacterial community in the ZnO50 treatment shows a high degree of overlap and similarity with CK, while the ZnO800 treatment did not overlap the other treatments, resulting in a significant separation effect. Hence, the high ZnO NP concentrations significantly changed the soil bacteria community structure, and reduced the soil bacterial abundance and diversity. As shown in Fig. 4d, the other groups had a low degree of overlap between treatments, resulting in clear separation effects. In general, ZnO NPs increased soil fungal abundance and decreased fungal diversity.
3.5. Relationship between soil microorganisms and potato growth indicators
Fig. 5 shows the correlations between soil microorganisms, Zn concentrations, and potato yield. The network comprises 66 nodes and 212 edges, with 94 positive and 118 negative correlations. The network's diameter is 10, and the modularity index is 4.535. Notably, potato yield strongly correlated with soil microorganisms, with Microscillaceae and Subgroup_7 positively correlated with various soil and potato Zn concentrations, while TFroot–tuber negatively correlated with Microscillaceae and Subgroup_7. Soil bacteria can affect Zn absorption in various potato parts. Ramlibacter negatively correlated with the proportion of large potatoes and average weight per potato. Penicillium positively correlated with nitrate-N and negatively correlated with ammonia-N. Subgroup_6 and soil nitrate-N positively correlated with plant height, while Verticillium positively correlated with tuber P and Cu concentrations.
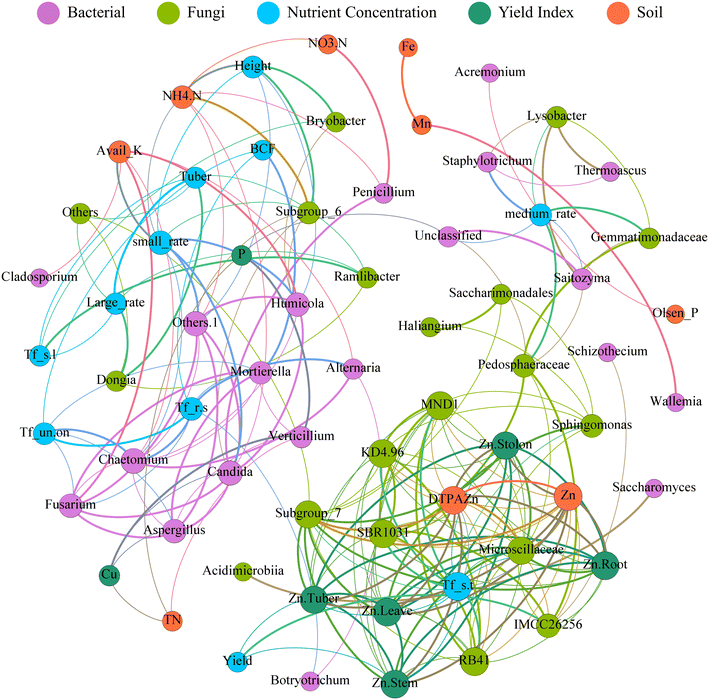 |
| Fig. 5 Network of interactions between the soil microorganisms and the potato elemental concentrations and yield. Line thickness indicates the strength of the correlation between the two indicators. Nodes are colored according to classification. Connections indicate strong correlations (Pearson's correlation coefficient >0.8, P < 0.05). | |
4. Discussion
The ZnO NP treatments in this study generally increased Zn absorption and transport in potato plants, promoting plant growth. Other studies have also shown that adding ZnO NPs to farmland soil significantly increased tissue Zn concentrations.6,29 This is likely because the extended contact of ZnO NPs with soil facilitates their slow release, enhancing their bioavailability and plant uptake.30 However, very high ZnO NP concentrations decreased the promotion effect on potato growth, as reported by Singh et al.31 Our study found that ZnO800 treatment significantly reduced the potato tuber P content, which is similar to Elshayb et al.,32 possibly because of the antagonistic effect between the Zn and tuber P content, and the dissolution of ZnO NPs in soil and the formation of zinc phosphate combined with the bioavailable P in soil.33
The ZnO NP treatments increased the average tuber weight, but decreased the number of potato tubers. They also increased the proportion of large tubers and decreased the proportion of small tubers, consistent with Rahman et al.34
We did not detect any ZnO NPs in potato tissues, including edible tubers (Fig. 2a), consistent with similar studies on wheat8 and cowpea.35 Du et al.29 reached a similar conclusion for wheat seedlings after adding TiO2 and ZnO NPs to the soil. While they reported small amounts of TiO2 NPs in the vacuoles of root tip cortex cells, no ZnO NPs were found. This phenomenon likely occurred because ZnO NPs are more soluble than CeO2 and TiO2 NPs, and their toxicity depends mainly on the dissolved Zn2+ rather than the ZnO NPs themselves.36
Recent research on potato quality has focused on their processing quality, including starch, protein, soluble sugar, and reducing sugar concentrations.37 We found that applying ZnO NPs within a certain concentration range significantly increased the sugar concentration of potato tubers without reducing protein and starch concentrations. This improvement can have significant implications for potato storage and nutritional value.38
Soluble sugars are fundamental components in plant structure and metabolism, and actively participate in various stress responses.39 The ZnO NP treatments increased soluble sugar and protein contents in potato tubers, consistent with the findings of Sun et al.40 Thus, ZnO NPs potentially contribute to the structural stability of proteins, maintain cell homeostasis, and increase the stress and drought resistance of potatoes. This effect may be attributed to Zn′s involvement in maintaining membrane potential and structure while also playing a regulatory role in protein synthesis, increasing sucrose transfer, and decreasing starch content.41,42 Consequently, ZnO NP application to potatoes significantly increased carbohydrate accumulation. Robertson and Alzaabi43 compared potatoes with equal energy and other starch carbohydrate foods. The findings revealed that potatoes induced a greater sense of satiety and, when consumed alone, resulted in lower energy consumption. Therefore, potatoes boasting high carbohydrate content could offer health benefits within a balanced diet, provided that excessive spices or additives are not incorporated.
Furthermore, ZnO NPs had varying impacts on soil microbial communities. Low concentrations significantly increased the number of bacterial OTUs, species abundance in rhizosphere soil, and the diversity of α species (Table 1), consistent with findings in guar bean rhizosphere soil.44 High concentrations significantly reduced soil bacterial abundance and diversity, even killing soil bacteria. Ge et al.18 also found after adding ZnO NPs to the soil and culturing for 60 days without plants, the amount of extractable DNA in the soil decreased exponentially as the ZnO NP concentration increased. Specifically, the microbial biomass significantly decreased at 50 mg kg−1 ZnO NPs. However, we found no significant effect at 50 mg kg−1 ZnO NPs, possibly because the presence of plants alleviated the ZnO NP toxicity.45 Ge et al.17 compared the number of soil bacteria OTUs after 48 days of soybean cultivation and no cultivation in soil containing 50 mg kg−1 ZnO NPs. They found that adding ZnO NPs to pure soil significantly reduced the number of OTUs compared with the CK (no ZnO NPs), whereas soil under soybean cultivation did not change significantly. The interplay between the soil environment and plant growth likely contributes to these shifts.
Rousk et al.46 concluded that the bacterial community structure strongly correlated with the soil Zn2+ concentration, with changes in the bacterial community structure caused by the released Zn2+ rather than ZnO NPs. Moreover, the effect of ZnO NPs on the soil bacterial community structure changes over time. Dıaz-Ravina and Bååth47 showed that the effect of an initial ZnO NP treatment on the soil community structure remained one year after removing Zn stress. After entering the soil, some ZnO NPs form agglomerates and adsorb onto the surface of soil particles, thus continuously dissolving Zn2+.34 Compared with bacteria, ZnO NPs had a smaller effect on the fungal community structure. For the species relative abundance at the genus level, the ZnO NP treatments affected the dominant group's proportion of bacterial groups. This is consistent with the results of Moll et al.,28 who measured the soil bacterial and fungal communities in soil containing 1000 mg kg−1 TiO2 NPs after 12 weeks of planting wheat. While the bacterial community structure in the treated soil significantly changed compared to CK, the fungal community structure did not significantly change. The difference in susceptibility between bacteria and fungi may be related to different interactions between NPs and their surfaces.
Certain bacterial genera, such as Sphingomonas, can repair environmental pollution and promote plant growth, with the degradation of metal compounds playing an intriguing role.48Sphingomonas is suitable for bioaugmentation, an effective strategy for inhibiting critical exogenous compounds.49 In our study, the ZnO NP treatments increased the relative proportion of Sphingomonas, reducing Zn stress in the soil. We found that ZnO NPs significantly increased the abundance of bacterial genera in the soil, such as Sphingomonas, Subgroup_6, Subgroup_7, and Microscillaceae. Many identified taxa are involved in Zn remediation in soils, including members of Bacillus, Sphingomonas, and Pseudomonas.50 Similarly, Liu et al.51 observed increased Sphingomonas, Subgroup_7, and Microscillaceae abundance after ZnO NP supplementation.
5. Conclusion
The application of ZnO NPs positively affected potato growth, nutrient content, and overall quality, including tuber expansion, increased proportion of large tubers, and decreased proportion of small tubers, ultimately improving the overall commodity rate of tubers. Moreover, ZnO NPs increased tissue Zn concentrations in potato in a dose-dependent manner, offering potential biofortification benefits. Notably, we found no evidence that ZnO NPs entered the potato plants, indicating the absence of specific transporters for ZnO NPs, and suggesting that the primary source of ZnO NP phytotoxicity is the release of Zn2+. High ZnO NP concentrations significantly affected the soil physical and chemical properties. While ZnO NPs did not change the dominant microbial groups in potato rhizosphere soil, the relative proportions of these species changed. In summary, low ZnO NP concentrations had high Zn biofortification efficiencies, which improved the potato quality and commercial yield with minimal environmental disturbance and no nano-specific toxicity. However, while the high ZnO NP concentrations had little impact on the plant growth and quality, they disrupted the soil stability with potential environmental consequences. Further investigations are needed to determine the ZnO NP threshold in current agricultural environments to avoid environmental pollution and human health risks associated with short-term high doses of ZnO NPs entering agricultural soils.
Abbreviations used
NPs | Nanoparticles |
XRD | X-Ray diffraction |
OTU | Operational taxonomic units |
TEM | Transmission electron microscopy |
BCFs | Bioconcentration factors |
TF | Translocation factor |
Author contributions
Haoyue Zhang: writing – original draft, writing – review & editing, and formal analysis. Xiaohan Zhao, Junrui Bai, and Mengshan Tang: methodology, resources, supervision, and validation. Wei Du and Zhiyuan Lv: conceptualization, project administration, and data acquisition. Kadambot H. M. Siddique: writing – review, writing & editing. Hui Mao: writing – review & editing, funding acquisition, project administration, and supervision.
Conflicts of interest
We declare that we do not have any commercial or associative interest that represents a conflict of interest in connection with the work submitted.
Acknowledgements
All of the authors gratefully acknowledge the National Key R&D Program of China (2021YFD190070402), the Special Funds for the Science and Technology Innovation of Shaanxi Province (2020zdzx03-02-01), and the China Agriculture Research System of MOF and MARA (CARS-27). We also thank the anonymous reviewers who have helped improve the manuscript's quality.
References
- M. Khan, M. S. A. Khan, K. K. Borah, Y. Goswami, K. R. Hakeem and I. Chakrabartty, The potential exposure and hazards of metal-based nanoparticles on plants and environment, with special emphasis on ZnO NPs, TiO2 NPs, and AgNPs: a review, Environ. Adv., 2021, 6, 100128, DOI:10.1016/j.envadv.2021.100128.
- G. D. Savi, K. C. Piacentini, S. R. de Souza, M. E. Costa, C. M. Santos and V. M. Scussel, Efficacy of zinc compounds in controlling Fusarium head blight and deoxynivalenol formation in wheat (Triticum aestivum L.), Int. J. Food Microbiol., 2015, 205, 98–104, DOI:10.1016/j.ijfoodmicro.2015.04.001.
- J. Hou, Y. Wu, X. Li, B. Wei, S. Li and X. Wang, Toxic effects of different types of zinc oxide nanoparticles on algae, plants, invertebrates, vertebrates and microorganisms, Chemosphere, 2018, 193, 852–860, DOI:10.1016/j.chemosphere.2017.11.077.
- R. Liu and R. Lal, Potentials of engineered nanoparticles as fertilizers for increasing agronomic productions, Sci. Total Environ., 2015, 514, 131–139, DOI:10.1016/j.scitotenv.2015.01.104.
- A. Singh, N. A. Singh, S. Afzal, T. Singh and I. Hussain, Zinc oxide nanoparticles: a review of their biological synthesis, antimicrobial activity, uptake, translocation and biotransformation in plants, J. Mater. Sci., 2018, 53(1), 185–201, DOI:10.1007/s10853-017-1544-1.
- J. H. Priester, Y. Ge, R. E. Mielke, A. M. Horst, S. C. Moritz, K. Espinosa, J. Gelb, S. L. Walker, R. M. Nisbet and Y.-J. An, Soybean susceptibility to manufactured nanomaterials with evidence for food quality and soil fertility interruption, Proc. Natl. Acad. Sci. U. S. A., 2012, 109(37), E2451–E2456, DOI:10.1073/pnas.1205431.
- L. V. Subbaiah, T. N. V. K. V. Prasad, T. G. Krishna, P. Sudhakar, B. R. Reddy and T. Pradeep, Novel effects of nanoparticulate delivery of zinc on growth, productivity, and zinc biofortification in maize (Zea mays L.), J. Agric. Food Chem., 2016, 64(19), 3778–3788, DOI:10.1021/acs.jafc.6b00838.
- T. Zhang, H. Sun, Z. Lv, L. Cui, H. Mao and P. M. Kopittke, Using synchrotron-based approaches to examine the foliar application of ZnSO4 and ZnO nanoparticles for field-grown winter wheat, J. Agric. Food Chem., 2017, 66(11), 2572–2579, DOI:10.1021/acs.jafc.7b04153.
- C. O. Dimkpa, U. Singh, P. S. Bindraban, W. H. Elmer, J. L. G. Torresdey and J. C. White, Zinc oxide nanoparticles alleviate drought-induced alterations in sorghum performance, nutrient acquisition, and grain fortification, Sci. Total Environ., 2019, 688, 926–934, DOI:10.1016/j.scitotenv.2019.06.392.
- V. D. Rajput, T. M. Minkina, A. Behal, S. N. Sushkova, S. Mandzhieva, R. Singh, A. Gorovtsov, V. S. Tsitsuashvili, W. O. Purvis and K. A. Ghazaryan, Effects of zinc-oxide nanoparticles on soil, plants, animals and soil organisms: a review, Environ. Nanotechnol., Monit. Manage., 2018, 9, 76–84, DOI:10.1016/j.enmm.2017.12.006.
- D. Zhou and A. A. Keller, Role of morphology in the aggregation kinetics of ZnO nanoparticles, Water Res., 2010, 44(9), 2948–2956, DOI:10.1016/j.watres.2010.02.025.
- A. A. Keller, S. McFerran, A. Lazareva and S. Suh, Global life cycle releases of engineered nanomaterials, J. Nanopart. Res., 2013, 15(6), 1–17, DOI:10.1007/s11051-013-1692-4.
- F. Wu, B. J. Harper and S. L. Harper, Comparative dissolution, uptake, and toxicity of zinc oxide particles in individual aquatic species and mixed populations, Environ. Toxicol. Chem., 2019, 38(3), 591–602, DOI:10.1002/etc.4349.
- M. Hamidat, M. Barakat, P. Ortet, C. Chanéac, J. R. Rose, J.-Y. Bottero, T. Heulin, W. Achouak and C. Santaella, Design defines the effects of nanoceria at a low dose on soil microbiota and the potentiation of impacts by the canola plant, Environ. Sci. Technol., 2016, 50(13), 6892–6901, DOI:10.1021/acs.est.6b01056.
- M. Hallama, C. Pekrun, H. Lambers and E. Kandeler, Hidden miners–the roles of cover crops and soil microorganisms in phosphorus cycling through agroecosystems, Plant Soil, 2019, 434(1), 7–45, DOI:10.1007/s11104-018-3810-7.
- J. Parada, O. Rubilar, M. A. Fernández-Baldo, F. A. Bertolino, N. Durán, A. Seabra and G. Tortella, The nanotechnology among US: are metal and metal oxides nanoparticles a nano or mega risk for soil microbial communities?, Crit. Rev. Biotechnol., 2019, 39(2), 157–172, DOI:10.1080/07388551.2018.1523865.
- Y. Ge, J. H. Priester, L. C. Van De Werfhorst, S. L. Walker, R. M. Nisbet, Y.-J. An, J. P. Schimel, J. L. Gardea-Torresdey and P. A. Holden, Soybean plants modify metal oxide nanoparticle effects on soil bacterial communities, Environ. Sci. Technol., 2014, 48(22), 13489–13496, DOI:10.1021/es5031646.
- Y. Ge, J. P. Schimel and P. A. Holden, Evidence for negative effects of TiO2 and ZnO nanoparticles on soil bacterial communities, Environ. Sci. Technol., 2011, 45(4), 1659–1664, DOI:10.1021/es103040t.
- Y. Peng, R. Yang, T. Jin, J. Chen and J. Zhang, Risk assessment for potentially toxic metal (loid) s in potatoes in the indigenous zinc smelting area of northwestern Guizhou Province, China, Food Chem. Toxicol., 2018, 120, 328–339, DOI:10.1016/j.fct.2018.07.026.
- A. Lehmann, S. D. Veresoglou, E. F. Leifheit and M. C. Rillig, Arbuscular mycorrhizal influence on zinc nutrition in crop plants–a meta-analysis, Soil Biol. Biochem., 2014, 69, 123–131, DOI:10.1016/j.soilbio.2013.11.001.
- P. C. Ghasal, Y. S. Shivay, V. Pooniya, M. Choudhary and R. K. Verma, Zinc partitioning in basmati rice varieties as influenced by Zn fertilization, Crop J., 2018, 6(2), 136–147, DOI:10.1016/j.cj.2017.09.001.
- N. Kumar, V. Shah and V. K. Walker, Influence of a nanoparticle mixture on an arctic soil community, Environ. Toxicol. Chem., 2012, 31(1), 131–135, DOI:10.1002/etc.721.
- X. Feng, Y. Yan, B. Wan, W. Li, D. P. Jaisi, L. Zheng, J. Zhang and F. Liu, Enhanced dissolution and transformation of ZnO nanoparticles: the role of inositol hexakisphosphate, Environ. Sci. Technol., 2016, 50(11), 5651–5660, DOI:10.1021/acs.est.6b00268.
- Y. Chen, S. Chai, H. Tian, Y. Chai, Y. Li, L. Chang and H. Cheng, Straw strips mulch on furrows improves water use efficiency and yield of potato in a rainfed semiarid area, Agric. Water Manag., 2019, 211, 142–151, DOI:10.1016/j.agwat.2018.09.048.
- D. Šimková, J. Lachman, K. Hamouz and B. Vokál, Effect of cultivar, location and year on total starch, amylose, phosphorus content and starch grain size of high starch potato cultivars for food and industrial processing, Food Chem., 2013, 141(4), 3872–3880, DOI:10.1016/j.foodchem.2013.06.080.
- Q. Liu, Q. Guo, S. Akbar, Y. Zhi, A. El Tahchy, M. Mitchell, Z. Li, P. Shrestha, T. Vanhercke and J. P. Ral, Genetic enhancement of oil content in potato tuber (Solanum tuberosum L.) through an integrated metabolic engineering strategy, Plant Biotechnol. J., 2017, 15(1), 56–67, DOI:10.1111/pbi.12590.
- S. Paul, N. Gogoi, B. Sarma and B. Baroowa, Biochemical changes in potato under elevated temperature, Indian J. Plant Physiol., 2014, 19(1), 36–42, DOI:10.1007/s40502-014-0066-y.
- J. Moll, F. Klingenfuss, F. Widmer, A. Gogos, T. D. Bucheli, M. Hartmann and M. G. van der Heijden, Effects of titanium dioxide nanoparticles on soil microbial communities and wheat biomass, Soil Biol. Biochem., 2017, 111, 85–93, DOI:10.1016/j.soilbio.2017.03.019.
- W. Du, Y. Sun, R. Ji, J. Zhu, J. Wu and H. Guo, TiO 2 and ZnO nanoparticles negatively affect wheat growth and soil enzyme activities in agricultural soil, J. Environ. Monit., 2011, 13(4), 822–828, 10.1039/c0em00611d.
- S. J. Bradfield, P. Kumar, J. C. White and S. D. Ebbs, Zinc, copper, or cerium accumulation from metal oxide nanoparticles or ions in sweet potato: yield effects and projected dietary intake from consumption, Plant Physiol. Biochem., 2017, 110, 128–137, DOI:10.1016/j.plaphy.2016.04.008.
- H. Singh, S. Singh, D. Kumar and S. K. Singh, Impact of foliar application of zinc on potato (Solanum tuberosum L.) CV. Kufri Pukhraj, Plant Arch., 2018, 18(2), 1334–1336 Search PubMed.
- O. M. Elshayb, K. Y. Farroh, H. E. Amin and A. M. Atta, Green synthesis of zinc oxide nanoparticles: fortification for rice grain yield and nutrients uptake enhancement, Molecules, 2021, 26(3), 584, DOI:10.3390/Molecules26030584.
- S. J. Watts-Williams, T. W. Turney, A. F. Patti and T. R. Cavagnaro, Uptake of zinc and phosphorus by plants is affected by zinc fertiliser material and arbuscular mycorrhizas, Plant Soil, 2014, 376(1–2), 165–175, DOI:10.1007/s11104-013-1967-7.
- M. W. Rahman, M. M. Islam, M. M. Sheikh, M. I. Hossain, M. A. Kawochar and M. S. Alam, Effect of foliar application of zinc on the yield, quality and storability of potato in tista meander floodplain soil, Pertanika J. Trop. Agric. Sci., 2018, 41(4), 1779–1793 Search PubMed.
- P. Wang, N. W. Menzies, E. Lombi, B. A. McKenna, B. Johannessen, C. J. Glover, P. Kappen and P. M. Kopittke, Fate of ZnO nanoparticles in soils and cowpea (Vigna unguiculata), Environ. Sci. Technol., 2013, 47(23), 13822–13830, DOI:10.1021/es403466p.
- B. D. Johnston, T. M. Scown, J. Moger, S. A. Cumberland, M. Baalousha, K. Linge, R. V. Aerle, K. Jarvis, J. R. Lead and C. R. Tyler, Bioavailability of nanoscale metal oxides TiO2, CeO2, and ZnO to fish, Environ. Sci. Technol., 2010, 44(3), 1144–1151, DOI:10.1021/es901971a.
- D. Fajardo, K. G. Haynes and S. Jansky, Starch characteristics of modern and heirloom potato cultivars, Am. J. Potato Res., 2013, 90(5), 460–469, DOI:10.1007/s12230-013-9320-5.
- J. Eggadi Ramesh, R. Chatterjee and G. Banik, Effect of foliar application of secondary and micronutrients on quality of potato, Int. J. Chem. Stud., 2019, 7(1), 2189–2192 Search PubMed.
- I. Couée, C. Sulmon, G. Gouesbet and A. El Amrani, Involvement of soluble sugars in reactive oxygen species balance and responses to oxidative stress in plants, J. Exp. Bot., 2006, 57(3), 449–459, DOI:10.1093/jxb/erj027.
- L. Sun, F. Song, J. Guo, X. Zhu, S. Liu, F. Liu and X. Li, Nano-ZnO-induced drought tolerance is associated with melatonin synthesis and metabolism in maize, Int. J. Mol. Sci., 2020, 21(3), 782, DOI:10.3390/ijms21030782.
- N. Taran, V. Storozhenko, N. Svietlova, L. Batsmanova, V. Shvartau and M. Kovalenko, Effect of zinc and copper nanoparticles on drought resistance of wheat seedlings, Nanoscale Res. Lett., 2017, 12, 1–6, DOI:10.1186/s11671-017-1839-9.
- S. Y. R. Sadati, S. J. Godehkahriz, A. Ebadi and M. Sedghi, Zinc oxide nanoparticles enhance drought tolerance in wheat via physio-biochemical changes and stress genes expression, Iran. J. Biotechnol., 2022, 20(1), e3027, DOI:10.30498/ijb.2021.280711.3027.
- T. M. Robertson and A. Z. Alzaabi, Starchy Carbohydrates in a Healthy Diet: The role of the humble potato, Nutrients, 2018, 10(11), 1764, DOI:10.3390/nu10111764.
- R. Raliya and J. C. Tarafdar, ZnO nanoparticle biosynthesis and its effect on phosphorous-mobilizing enzyme secretion and gum contents in clusterbean (Cyamopsis tetragonoloba L.), Agric. Res., 2013, 2(1), 48–57, DOI:10.1007/s40003-012-0049-z.
- V. Rajput, T. Minkina, M. Mazarji, S. Shende and H. Jatav, Accumulation of nanoparticles in the soil-plant systems and their effects on human health, Ann. Agric. Sci., 2020, 65(2), 137–143, DOI:10.1016/j.aoas.2020.08.001.
- J. Rousk, K. Ackermann, S. F. Curling and D. L. Jones, Comparative toxicity of nanoparticulate CuO and ZnO to soil bacterial communities, PLoS One, 2012, 7(3), e34197, DOI:10.1371/journal.pone.0034197.
- A. M. Dıaz-Ravina and E. Bååth, Response of soil bacterial communities pre-exposed to different metals and reinoculated in an unpolluted soil, Soil Biol. Biochem., 2001, 33(2), 241–248, DOI:10.1016/S0038-0717(00)00136-X.
- S. Asaf, M. Numan, A. L. Khan and A. Al-Harrasi, Sphingomonas: from diversity and genomics to functional role in environmental remediation and plant growth, Crit. Rev. Biotechnol., 2020, 40(2), 138–152, DOI:10.1080/07388551.2019.1709793.
- B. M. Coppotelli, A. Ibarrolaza, M. T. Del Panno and I. S. Morelli, Effects of the inoculant strain Sphingomonas paucimobilis 20006FA on soil bacterial community and biodegradation in phenanthrene-contaminated soil, Microb. Ecol., 2008, 55(2), 173–183, DOI:10.1007/s00248-007-9265-7.
- A. Muratova, S. Golubev, V. Romanova, I. Sungurtseva and A. Nurzhanova, Effect of heavy-metal-resistant PGPR inoculants on growth, rhizosphere microbiome and remediation potential of miscanthus× giganteus in Zinc-contaminated soil, Microorganisms, 2023, 11(6), 1516, DOI:10.3390/microorganisms11061516.
- Y. Liu, Y. Li, B. Pan, X. Zhang, H. Zhang, E. W. Steinberg, H. Qiu, M. G. Vijver and W. J. G. M. Peijnenburg, Application of low dosage of copper oxide and zinc oxide nanoparticles boosts bacterial and fungal communities in soil, Sci. Total Environ., 2021, 757, 143807, DOI:10.1016/j.scitotenv.2020.143807.
|
This journal is © The Royal Society of Chemistry 2024 |