Core–shell Ag@polypyrrole for synchronous pre-enrichment and immobilization of iodine (I−, IO3−) from liquid radioactive wastes†
Received
23rd September 2023
, Accepted 13th November 2023
First published on 15th November 2023
Abstract
Radioiodine is of great concern owing to its high mobility in the environment and long-term radiotoxicity, and effective techniques for the simultaneous removal of iodide (I−) and iodate (IO3−) from aqueous solutions remain a significant challenge. Here, Ag@polypyrrole core–shell nanoparticles (Ag@PPy) were synthesized in situ and constructed by synergistic enrichment and immobilization as a desirable iodine nano-adsorbent. Its performance benefits from the ability for anions to be pre-enriched on the surface of Ag@PPy via electrostatic force through the positively charged polypyrrole with nitrogen-containing groups and then immobilized in the core–shell nanostructure with nanosilver as a reactive center. These efforts gave rise to Ag@PPy exhibiting an ultrahigh iodide adsorption capacity (788.7 mg g−1) and desirable iodate uptake capacity (133.9 mg g−1). More importantly, in the low-concentration region, Ag@PPy is able to almost completely remove I− and IO3− from aqueous solution even in the presence of competitive anions such as Cl−, SO42−, NO3− and CO32−, with a distribution coefficient Kd of up to 3.90 × 105 and 2.30 × 105 mL g−1, respectively. Unexpectedly, this core–shell nanostructure endows silver-based materials with high stability under acid–base conditions, and reduces the leaching rate approximately 10-fold compared to silver powder at near-neutral pH. This work highlights the feasibility of using Ag-containing nanomaterials to separate radioiodine from liquid environments.
Environmental significance
The efficient removal of iodide (I−) and iodate (IO3−) from aqueous solution is considered to be of strategic importance for radioactive waste disposal and environmental remediation due to its extremely high solubility and mobility in contaminated water. Herein, highly well-dispersed silver nanoparticles were encapsulated within positively charged polypyrrole (Ag@PPy) to form a stable core–shell nanostructure, which consequently exhibited simultaneous efficient I− and IO3− removal performance. Pre-enrichment was achieved by electrostatic interaction, followed by further immobilization within the core–shell structure via metal-based reaction with a high removal rate of up to 98.98%. Ag@PPy exhibited superior selectivity and stability, making it suitable for application in iodine-contaminated groundwater.
|
1. Introduction
Radioactive iodine (129I), a fission product of uranium-235 during used nuclear fuel reprocessing, is an environmental concern due to its extremely long half-life (15.7 million years), toxicity, and high mobility in the environment.1–3 A great deal of radioactive iodine has been released into our environment, not only during used nuclear fuel reprocessing, nuclear accidents and nuclear weapon testing, but also during the normal operation of nuclear power plants or the discharge of medical wastewater from radionuclide therapeutic applications. In particular, the Fukushima nuclear accident released massive amounts of radioiodine into the atmosphere and oceans, which has attracted great global attention.4,5 Iodine species mainly exist in the form of iodide (I−), iodate (IO3−), elemental iodine (I2) and organo-iodine in liquid environments, depending on the redox conditions of a given aqueous solution, and exhibit complex biogeochemical behavior in the environment.6,7 Additionally, the extremely high solubility and mobility of these iodine species cause a long-term threat to human health and the environment.8–10 Thus, the effective separation and immobilization of radioiodine is of great significance for used fuel reprocessing and contaminated water remediation.
Extensive efforts have been devoted to removing radioiodine species from wastewater through methods such as co-precipitation, membrane separation, adsorption, ion exchange, etc., which have been applied to the separation and enrichment of iodine.11,12 Adsorption is suitable for iodine removal in water treatment owing to its low cost, easy maintenance and operation. Various adsorbent materials can be deployed for the removal of aqueous iodine, including Ag-based materials,13,14 Bi-based materials,9,15 resins,5 aerogels,16 metal oxides,17,18 layered double hydroxides19 and metal organic frameworks (MOFs).20 The calcined Mg/Al LDHs showed the maximum adsorption capacity of 439 mg g−1, and their uptake was dependent on the initial interlayer anion.21 Novel mesoporous Fe-based MOFs (Bi@MIL) were observed to show an adsorption capacity of as high as 189.6 mg g−1 at an initial I− concentration of 200 mg L−1, which was greatly affected by the solution pH.22 Silver-based materials including silver-exchanged zeolite and silver-impregnated activated carbon have proved to be promising sorbents for iodine removal due to the formation of AgI (Ksp = 8.5 × 10−17) precipitates with I−.14,23 Nanomaterials, with their superior physicochemical stability due to the nano-size effect, large specific surface area and high reactivity, have proven to be potential materials for radioactive wastewater decontamination.24,25 Unfortunately, few studies have focused on the simultaneous removal of iodide and iodate from a wastewater matrix with relatively low iodine concentrations. In practicality, the I− and IO3− species commonly coexist as they are both generated in the used fuel reprocessing.26 Additionally, the introduction of nano-metal is expected to greatly improve iodine removal by metal interactions. However, most metal-incorporated adsorbents are loaded on the surface of the host by the impregnation method, and some exposed active metal components will dissolve under low acidic conditions, resulting in reduced effective utilization and further degrading the adsorption effect. One way to overcome this shortcoming is to encapsulate the metal nanoparticles in a highly stable substrate. Therefore, the construction of a stable matrix with abundant active sites and the ability to simultaneously adsorb I− and IO3− is an urgent problem that needs to be solved.
Polypyrrole (PPy), which has positively charged nitrogen atoms and anion-exchange capacity, represents a suitable candidate for anion elimination.27 Additionally, owing to its low cost, superior chemical stability and simple preparation process, PPy can be used as an ideal substrate for functional materials.28,29 The excellent performance of PPy in the field of anion separation, especially the removal of halogenoxide anions,30–32 also gives it great application prospects in the separation of iodine anion pollutants. Further encapsulating the metal with polypyrrole is expected to solve the disadvantages of poor stability and low adsorption capacity of traditional metal-impregnated materials, which is of great significance for the efficient separation and enrichment of iodide and iodate in waste liquid.
Inspired by the above nanostructures, we designed a silver-encapsulated polypyrrole (Ag@PPy) nanoparticle with a core–shell structure, which was synthesized in situ using a simple one-step reaction at room temperature. Notably, the activity of the nanosilver was maintained as a result of the protection of the polypyrrole shell, thereby maximizing its effective utilization. The admirable electrostatic interaction facilitated the enrichment of I−/IO3− from aqueous solutions into Ag@PPy. The encapsulated nano-silver provided a reactive site as a capture center for I−/IO3− and achieved high iodine removal capacity through an irreversible chemical reaction process. This synthesis strategy exhibited excellent flexibility in stabilizing active nanometals and satisfactory selectivity for iodide and iodate.
2. Materials and methods
2.1. Materials
The pyrrole (Py), silver nitrate (AgNO3), sodium borohydride (NaBH4), polyvinylpyrrolidone (PVP), sodium nitrate (NaNO3), sodium chloride (NaCl), sodium carbonate (Na2CO3), sodium sulfate (Na2SO4), sodium fluoride (NaF), sodium silicate (Na2SiO3), trisodium phosphate (Na3PO4), ethanol, potassium iodide (KI) and potassium iodate (KIO3) used in this work were obtained from Kelong Chemicals Company. All reagents used in this experiment were of analytical grade without further purification.
2.2. Synthesis of Ag@PPy
Typically, NaBH4 solution (50 mL, 4 mmol L−1) was added dropwise to AgNO3 solution (50 mL, 1 mmol L−1) under stirring. 2.3 g of PVP was completely dissolved in the above solution, in which 1 mL Py was added into the mixture and stirred for about 20 min. Subsequently, 1.2 g of AgNO3 as an oxidant as well as a dopant was further added to be sufficiently dissolved and then allowed to stand for 2 days. Finally, the obtained product was filtered, washed with deionized water and ethanol, and dried under vacuum for 24 hours at room temperature.
2.3. Characterizations
The morphologies of the samples were detected using scanning electron microscopy (SEM, UItra55, Germany). Structural and morphological characterizations of the prepared samples were conducted using a spherical-aberration-corrected transmission electron microscope (STEM, Titan G2 60–300, FEI) attached to an energy dispersive X-ray spectrometer unit (EDX). FT-IR spectra of dried samples in potassium bromide discs were recorded at 400–4000 cm−1 using a Nicolet-5700 model FT-IR spectrometer. The crystal texture was identified using X-ray diffraction (XRD, X Pert pro) with Cu-Kα (40 kV, 40 mA) radiation. Raman spectra were measured using an inVia laser Raman spectrometer. A zeta potential analyzer (JS94K) was used to test the zeta potential of the samples. The composition and chemical state of the elements were measured using X-ray photoelectron spectroscopy (XPS, Thermo Escalab 250Xi). The leaching concentration of silver in the solution was measured using inductively coupled plasma mass spectrometry (ICP-MS; iCAP 6500, UK) under different pH conditions. A UV-visible spectrophotometer (UV-vis, DR 6000) and/or inductively coupled plasma optical emission spectrometry (ICP-OES, iCAP 7000) were used to determine the iodine concentration.
2.4. Batch experiments
All experiments were conducted at room temperature under atmospheric conditions using a batch adsorption method. This work explored the effects of different adsorbent doses, contact times, initial concentrations, pH values and competitive anions on the adsorption of iodine anions. For the adsorption kinetics experiment, the resulting solution was stirred for the desired contact time and separated with a 0.22 μm nylon membrane filter. The effect of other competitive anions was examined by adding 1.6 × 102 mM F−, Cl−, SO42−, NO3−, CO32−, SiO32− or PO43− to a 1.6 mM iodide/iodate solution. The concentrations of iodine in aqueous solution were measured using a UV–visible spectrophotometer. The adsorption capacity of the adsorbent at equilibrium qe (mg g−1) was calculated according to the following equation: | 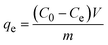 | (1) |
where C0 (mg L−1) and Ce (mg L−1) are the initial and equilibrium concentrations of I−/IO3− anions in solution. Moreover, V (mL) is the solution volume, and m (mg) is the adsorbent mass.
For the removal of iodine at low concentrations, we performed adsorption experiments at four respective concentrations of iodide and iodate: 10 mg L−1, 1 mg L−1, 100 μg L−1 and 10 μg L−1. In addition, iodine removal experiments under simulated wastewater conditions were performed with five solid/liquid ratios of 0.25 g L−1, 0.5 g L−1, 1 g L−1, 2 g L−1 and 5 g L−1 to evaluate the adsorption performance of the sample in iodine-containing wastewater solution. The accurate concentrations of iodide and iodate were determined using ICP-OES.
3. Results and discussion
3.1. Characterization
Ag@PPy core–shell nanoparticles were hydrothermally synthesized using pyrrole and silver nitrate as the reducing agent and oxidant, respectively.33,34 The core–shell structure was confirmed directly using SEM and STEM (Fig. 1a and b). The as-synthesized Ag@PPy exhibited well-shaped and relatively homogeneous spherical nanoparticles. The prepared nanospheres with a core diameter of 60–90 nm and a shell thickness of 5–30 nm were uniform in size and well dispersed. The high-resolution STEM image provides additional detail about the encapsulated nanosilver. The lattice fringes had a spacing of 2.33 Å, which matches well with the spacing of the (111) planes of Ag fcc (Fig. 1b). The elemental distribution of C, N and Ag (Fig. 1c) and the EDX spectrum (Fig. S1†) further confirm that the silver nanoparticles are encapsulated in PPy polymers. Fig. 1d shows the EDX line profile of silver along the red line through the particle in the STEM-HAADF image. The EDX counts of silver in the Ag line profile increase with increasing depth in the particle, but gradually reach a steady plateau, consistent with the core–shell structure of Ag@PPy.
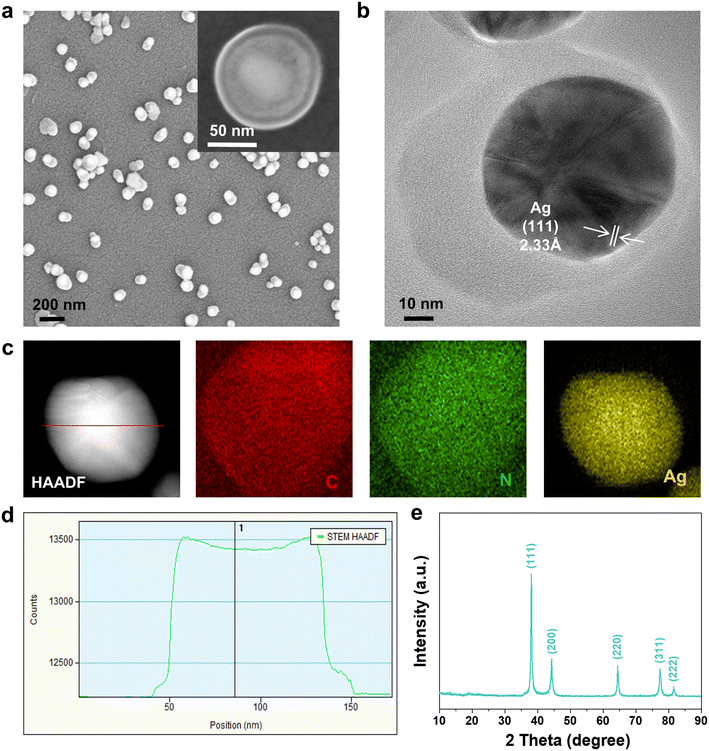 |
| Fig. 1 Structure and morphology of Ag@PPy material. (a) SEM images, (b) high-resolution STEM image, (c) HAADF image and STEM-EDS elemental mapping, (d) Ag line profiles, and (e) XRD patterns. | |
The XRD results for Ag@PPy reveals its phase composition. As shown in Fig. 1e, the weak diffraction peak with a 2θ value of around 20–25° is characteristic of amorphous polypyrrole, consistent with pure PPy in Fig. S2.†35,36 The other five diffraction peaks at 2θ = 38.1°, 44.3°, 64.4°, 77.5°, and 81.5° correspond to the (111), (200), (220), (311) and (222) planes of the Ag0 phase, indicating the presence of Ag nanoparticles in the Ag@PPy composite material.37 The molecular structures of the nanocomposite were further characterized using Raman and FTIR spectra. The peaks at 1582 and 1411 cm−1 were attributed to the C
C stretching of the PPy backbone and an antisymmetric C–N stretching band, respectively (Fig. S3†).38 The band at 1332 cm−1 was associated with the bipolaron ring stretching, while two small peaks at 1049 and 985 cm−1 were assigned to symmetrical C–H in-plane bending and C–H polaron in-plane deformation, respectively.39–41 The FTIR spectrum of Ag@PPy is shown in Fig. S4;† the peak at 3433 cm−1 is associated with the N–H stretching vibration, while the characteristic peak located at 1552 cm−1 corresponds to the pyrrole ring-stretching.42 The peaks at 1186 and 1035 cm−1 are associated with the C–N stretching mode and the in-plane vibration of
C–H, respectively; that at 920 cm−1 is attributable to the ring deformation.39 In addition, the band at 1646 cm−1 is related to the C
O stretching vibration, which indicates the presence of a small amount of unwashed PVP in the resulting composite.43 Furthermore, a very strong absorption band assignable to NO3− is observed at 1384 cm−1, suggesting that the obtained PPy in the Ag@PPy composite is doped by NO3−.44 The results were in good agreement with the reported data, which demonstrated that PPy had been successfully synthesized.
3.2. Adsorption kinetics and isotherms
The influence of different adsorbent dosages on the removal of iodide (200 mg L−1, 20 mL) was initially investigated (Fig. S5†). It was observed that the removal efficiency increases with increasing adsorbent dosage, which may be due to the increase in surface area and adsorption active sites. When the amount of adsorbent reached 0.5 g L−1, a removal efficiency of more than 90% was achieved. Conversely, the adsorption capacity (qe) decreased with increasing adsorbent dose, which may be attributed to the unsaturation of the adsorption sites. Therefore, we chose a solid–liquid ratio of 0.25 g L−1 to further investigate the effects of different factors such as kinetics, initial concentration, pH value, and coexisting ions on I−/IO3− removal by Ag@PPy. As shown in Fig. 2a, the adsorption of iodide and iodate by Ag@PPy increased gradually with increasing contact time. The sorption process reached equilibrium within 40 h, at which the adsorption capacity was 703.3 mg g−1 for I−. The uptake capacity of Ag@PPy for IO3− was limited, with only 100.7 mg g−1 within 2 h. This was primarily due to the relatively high solubility of AgIO3, which makes silver-based materials inefficient in removing IO3−. The adsorption process of I− and IO3− on Ag@PPy follows the pseudo-second-order kinetics model, which means that chemisorption dominated the adsorption process,5,45 involving valency forces and ion exchange through the sharing or exchange of electrons between the adsorbent and adsorbate in the form of covalent bonds, and the maximum adsorption capacities were calculated to be 751.88 and 116.01 mg g−1, respectively (Fig. S6 and Table S1†).
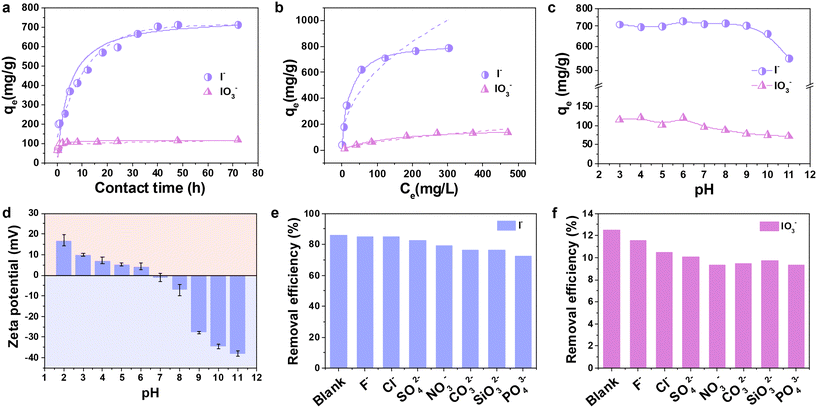 |
| Fig. 2 (a) Kinetics and (b) isotherm adsorption of I−/IO3− by Ag@PPy. (c) Effect of pH on adsorption of I−/IO3− and (d) the zeta potential of the Ag@PPy at different pH. Effect of competing anions on adsorption of (e) I− and (f) IO3− by Ag@PPy. | |
To ensure complete sorption, the isotherm experiments were conducted for 48 h (Fig. 2b). The saturated adsorption capacity of Ag@PPy for I− and IO3− reached 788.7 and 133.9 mg g−1, respectively. The Langmuir and Freundlich isotherms were calculated to evaluate the maximum sorption capacity; the full datasets are given in Fig. S7 and Table S2.† According to the correlation coefficient R2 of the two isotherm models, the Langmuir isotherm was suitable for both I− and IO3−, indicating that the removal process of I−/IO3− can be described as homogeneous monolayer adsorption. The calculated maximum sorption capacity is 826.4 mg g−1 for I− and 175.1 mg g−1 for IO3−, demonstrating that Ag@PPy was more conducive to I− removal. The developed material, Ag@PPy, combines highly active silver nanoparticles and positively charged nitrogen groups and exhibits a record-high I− adsorption capacity, much higher than those of a reported Ag@SOF@Co–Fe PBs composite (388.39 mg g−1),46 silver-doped zeolite 4A (160 mg g−1)47 and ZnO@Ag@Cu2O nanocomposite (217.4 mg g−1),48etc. (Table S3†). Additionally, its adsorption capacity for IO3− was significantly more remarkable than that of a Prussian-blue-functionalized CoCr layered double hydroxide (91 mg g−1),49 purolite A530E resin (53.34 mg g−1),5 and diatomite/nano-titanium dioxide composite (1.31 mg g−1)50 (Table S4†). In fact, all the Ag-containing materials had similar properties with high adsorption capacity for iodide, while iodate removal was limited, likely because of the low chemisorption affinity of iodate with silver and the large ionic size, resulting in the poor removal of iodate.51 More importantly, these core–shell nanomaterials greatly reduce the leaching rate of encapsulated silver, which is approximately 10 times lower than that of silver powder at near-neutral pH (Table 1 and Fig. S8†). The highly stable structure of the PPy shell protects the encapsulated nanosilver from being eroded by acid–base solutions. The XRD patterns of the immersed samples confirm that Ag@PPy fully retains its structure after immersion in solutions with different pH values for 3 days (Fig. S9†). Obviously, this further improves the effective utilization rate of the active silver nanoparticles to obtain higher adsorption capacity and more stable immobilization capacity for the targeted removal of radioactive iodine. Moreover, this core–shell Ag@PPy outperforms traditional silver-impregnated materials, such as silver-impregnated activated carbon, which may leach silver into the solution under acidic conditions.23 The continuous release of Ag and AgI/AgIO3 particles would require purification and present potential disposal challenges due to the toxicity of Ag. Only in the case of improved sorbent stability should silver-containing media be considered for iodine removal from the liquid phase.
Table 1 Ag leaching percentage (%) of silver powder and Ag@PPy soaked at pH = 3–11 after 2 days
pH |
Ag powder (%) |
Ag@PPy (%) |
3 |
9.311 ± 0.014 |
3.018 ± 0.020 |
4 |
5.679 ± 0.023 |
1.117 ± 0.013 |
5 |
3.416 ± 0.030 |
0.460 ± 0.018 |
6 |
1.915 ± 0.020 |
0.157 ± 0.021 |
7 |
1.279 ± 0.008 |
0.106 ± 0.015 |
8 |
0.915 ± 0.034 |
0.095 ± 0.006 |
9 |
0.826 ± 0.026 |
0.087 ± 0.010 |
10 |
0.773 ± 0.015 |
0.079 ± 0.009 |
11 |
0.768 ± 0.007 |
0.083 ± 0.011 |
3.3. Effect of pH
The effect of the solution pH value on the removal of I−/IO3− by Ag@PPy was investigated in the pH range of 3–11. The adsorption capacity of Ag@PPy for I− showed negligible change in the pH range of 3–9, while a decrease was observed under conditions of pH > 9 (Fig. 2c). The same is true for the removal of IO3−, which dropped slightly at pH > 7. I−/IO3− removal decreased because of competing anions such as OH− and the surface deprotonation of Ag@PPy under alkaline conditions. Generally, the pH-dependent surface charge of the adsorbent may also be responsible for pH-dependent I−/IO3− adsorption. The zeta potential of Ag@PPy as a function of pH is also shown in Fig. 2d. The surface of Ag@PPy is positively charged under acidic conditions, and electrostatic interaction enhances its enrichment of I−/IO3−. This result clearly reveals the important role of the presence of nitrogen atoms in polypyrrole for anion adsorption.27 However, as the solution pH increased, especially at pH > 9, the higher OH− concentrations enabled Ag@PPy to exhibit a stronger negative charge due to deprotonation effects.49 This enhanced the electrostatic repulsion with I− and IO3− anions, resulting in reduced adsorption performance. Additionally, the pH changed before and after adsorption. As shown in Fig. S10,† in acidic solutions, iodine adsorption resulted in an increase in the equilibrium pH relative to the initial pH, which confirms a mechanism of chemical reaction between silver and iodine ions.52 However, this mechanism is inhibited by high concentrations of OH− in alkaline solutions, and OH−, as a competing anion, could also be adsorbed on the adsorbent surface, resulting in a slight decrease in the pH after adsorption in this pH range.53 The large amount of OH− in solution as well as the significantly increased surface negative charge of Ag@PPy as the pH was increased to 10 lead to a sharp decrease in adsorption capacity. Notably, an uptake of more than 500 mg g−1 was achieved at pH = 11, which is still superior to most inorganic materials, such as Cu2O and Ag2O@Mg(OH)2.54–56 The Ag@PPy core–shell nanosorbent exhibits high removal ability for I− anions over a wide pH range, which can overcome the drawbacks of previously reported Ag-based adsorbents used only in a limited pH range,13,46,48 and thus, it has the potential to be applied to practical radioactive wastewater treatment.
3.4. Effects of competitive anions
Notably, since nuclear wastewater typically contains multiple anions, selective removal undoubtedly becomes even more problematic. Considering that various competing anions are present in low-activity wastes (LAW) of the liquid wastes at the Hanford site,57 the selective adsorption of I− and IO3− by Ag @PPy in the presence of high concentrations of F−, Cl−, SO42−, NO3−, CO32−, SiO32− and PO43− was conducted. As shown in Fig. 2e, an excess of F−, Cl− and SO42− did not significantly affect the adsorption of I− anions; the removal percentage of I− was still higher than 80%, which was comparable to that of the blank. In the presence of NO3−, CO32−, SiO32− and PO43−, the removal efficiency decreases slowly. Generally, anions with higher charge densities often successfully outcompete I− or IO3− during the exchange process owing to stronger host–guest electrostatic interactions.58 With the higher charge densities of the anions, the detrimental effect of the anions on I− and IO3− is greater. As mentioned previously, an alkaline environment is also not conducive to the adsorption of iodide and iodate, and high concentrations of CO32−, SiO32− and PO43− may increase the solution pH, which will also adversely inhibit I− removal.59 In the presence of a 100-fold excess of NO3−, the removal rate of I− by Ag@PPy decreased from 85.97% to 79.20%, indicating that a large excess of NO3− inhibited the anion exchange behavior with I−, but the effect was not significant. Under the same conditions with a NO3− to I− molar ratio of 100
:
1, purolite A530E resin only removed nearly 25% of I−.5 Unexpectedly, Ag@PPy exhibited high selectivity for I− adsorption because it maintained a removal percentage of more than 75% in all cases, even when the molar ratio of coexisting ions was 100 times that of I−. Apparently, although Ag@PPy showed much less affinity toward IO3− than I−, the high concentration of competitive anions slightly reduced the uptake of IO3− compared to the removal of 12.52% of IO3− under blank conditions (Fig. 2f). The underlying reason that this IO3− uptake differs from that of I− is well elucidated in the mechanism section below.
3.5. Iodine removal at low concentration
In addition to determining whether it can maintain high adsorption capacity, it is also necessary to assess whether Ag@PPy can remove iodine-containing contaminants at low concentrations to meet the current standards for iodine discharge into water. In general, iodine-containing wastewater has iodine contents ranging from 0.068 μg L−1 to 2 mg L−1 (Table S5†). Therefore, we performed adsorption experiments at concentrations of 10 mg L−1, 1 mg L−1, 100 μg L−1 and 10 μg L−1 iodine (I−/IO3−) in near-neutral pH solutions. Comparing the initial and final iodine concentrations, both the I− and IO3− removal percentages reach as high as 92% (Table 2). The distribution coefficients are in the range of 5.31 × 104 to 3.90 × 105 mL g−1. The ability of Ag@PPy to remove iodine is much higher than that of silver-impregnated granular activated carbon (Ag-GAC), with maximum distribution coefficients of 4423 and 612 mL g−1 for I− and IO3−, respectively.60 Furthermore, even in the presence of one equivalent of competing anions, including Cl−, SO42−, NO3− and CO32−, the uptake of iodide and iodate are still higher than 96% with distribution coefficients of >1.06 × 105 mL g−1. The residual iodine concentrations were <4 μg L−1; thus, the solution treated with Ag@PPy can fully meet the standards of China for drinking water (GB 5749-2022) of below 100 μg L−1 iodine and for the USA, for which the range of iodine levels is from 4 to 18 μg L−1 in drinking water.61,62 These excellent properties make it possible to truly remove iodine contaminants in the environment.
Table 2 Uptake of I− and IO3− onto Ag@PPy material. Experimental conditions: m/V = 0.25 g L−1, contact time: 48 h
Sample |
I− |
IO3− |
pH |
Initial (μg L−1) |
Final (μg L−1) |
Removal (%) |
K
d (mL g−1) |
pH |
Initial (μg L−1) |
Final (μg L−1) |
Removal (%) |
K
d (mL g−1) |
I |
6.75 |
10 |
0.10 |
98.98 |
3.90 × 105 |
6.40 |
10 |
0.29 |
97.14 |
1.36 × 105 |
I |
6.70 |
100 |
1.87 |
98.13 |
2.10 × 105 |
6.48 |
100 |
1.71 |
98.29 |
2.30 × 105 |
I |
6.69 |
1000 |
21.45 |
97.86 |
1.83 × 105 |
6.27 |
1000 |
36.78 |
96.32 |
1.05 × 105 |
I |
6.61 |
10 000 |
219.89 |
97.80 |
1.78 × 105 |
6.34 |
10 000 |
700.72 |
92.99 |
5.31 × 104 |
I + NO3− |
6.74 |
100 |
2.64 |
97.36 |
1.48 × 105 |
6.33 |
100 |
3.62 |
96.38 |
1.06 × 105 |
I + CO32− |
9.66 |
100 |
2.49 |
97.51 |
1.57 × 105 |
9.59 |
100 |
2.55 |
97.45 |
1.53 × 105 |
I + SO42− |
7.06 |
100 |
2.77 |
97.23 |
1.41 × 105 |
6.28 |
100 |
2.06 |
97.94 |
1.90 × 105 |
I + Cl− |
6.59 |
100 |
1.91 |
98.09 |
2.06 × 105 |
6.91 |
100 |
1.82 |
98.18 |
2.15 × 105 |
3.6. Iodine removal in the simulated Hanford wastewater system
To further validate the potential application of Ag@PPy in actual iodine-containing waste solutions, we performed adsorption experiments using simulated Hanford groundwater. As listed in Table S6,† the simulated solution was composed of four competitive anions, SiO32−, Cl−, SO42−, and CO32−, in addition to I− and IO3−. In this type of solution, the concentration of CO32− is 150 times higher than that of I− and IO3−, making selective removal of I− and IO3− a huge challenge. Notably, Ag@PPy can capture 64.29% of I− from the simulated Hanford wastewater with a solid–liquid ratio of 1 g L−1 (Table S7†). When the solid–liquid ratio was increased to 5 g L−1, as much as 86.43% of I− was removed, whereas the removal rate of IO3− increased to 52.87%. Therefore, it is assumed that when there is sufficient adsorbent, the adsorption efficiency is favorable. In addition, Ag@PPy showed a high Kd value (224 mL g−1) for iodate removal at a solid–liquid ratio of 5 g L−1, while the distribution coefficients of CeO2 and FeA were 45 and 14 mL g−1, respectively, under the same simulated Hanford wastewater conditions.2 In contrast, using Ag@PPy to remove iodine can reach deeper levels. The results showed the promising practical applicability of Ag@PPy in the treatment of iodine-containing wastewater.
3.7. Sorption mechanisms
In order to understand the uptake mechanism of Ag@PPy, XRD, Raman, FT-IR, XPS and STEM analysis of the adsorbents after the uptake of iodine were performed. For the adsorption of iodide, characteristic peaks at 2θ = 22.32°, 23.71°, 25.35°, 32.76°, 39.20°, 42.63° and 46.31° attributed to AgI (JCPDS No. 09-0374) were observed, and strong chemical interaction occurred (Fig. 3a). Conversely, only a small amount of the AgIO3 phase was formed after iodate adsorption, which means that the Ag@PPy sorbent was partially involved in the chemical adsorption process due to the low affinity of Ag with IO3−. According to the results of STEM and XRD analysis described above, it was revealed that silver was originally present as metallic silver (Ag0) in the interior of the polypyrrole. Therefore, the I− or IO3− removal might have occurred according to the following chemical reactions with the involvement of dissolved oxygen in solution:14,52 | 4Ag0 + 4I− + O2(aq) + 2H+ → 4AgI + 2OH− | (2) |
| 4Ag0 + 4IO3− + O2(aq) + 2H+ → 4AgIO3 + 2OH− | (3) |
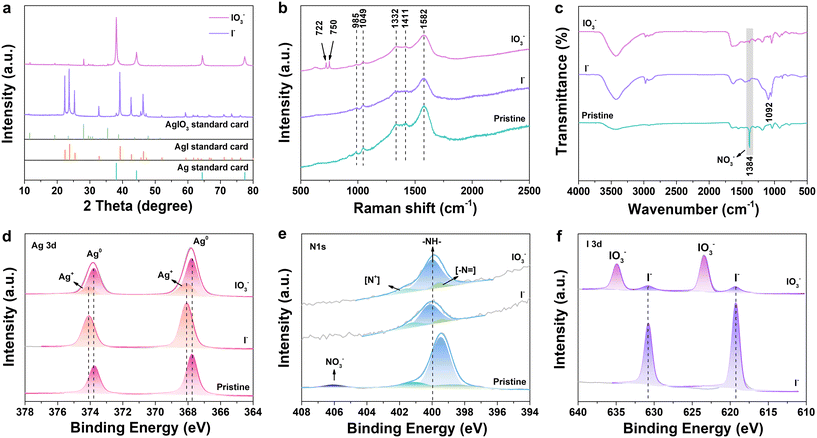 |
| Fig. 3 (a) XRD patterns, (b) Raman spectrum and (c) FTIR spectroscopy of Ag@PPy after I− and IO3− adsorption. XPS spectra: (d) Ag 3d, (e) N 1s and (f) I 3d of Ag@PPy after I− and IO3− adsorption. | |
The adsorption of iodine on Ag@PPy nanoparticles was further investigated using the Raman technique, as shown in Fig. 3b. The new peaks at 750 and 722 cm−1 are attributed to the in-plane vibration of IO3−,15,63 while there was no significant Raman band of I− in this wavenumber region. The FT-IR spectrum shows that a very strong absorption band at 1384 cm−1 that can be assigned to NO3− was observed to decrease after I−/IO3− adsorption (Fig. 3c), probably due to ion exchange of the I− and IO3− anions with NO3−. In addition, an increase in the intensity of the bands in the range of 1200–900 cm−1 could possibly be ascribed to the vibration of the pyrrole ring caused by the strong interaction between Ag@PPy and I−.27
X-ray photoelectron spectroscopy analysis was conducted on the pristine Ag@PPy and on Ag@PPy after iodide and iodate adsorption to explore the iodine species distribution on Ag@PPy and the mechanisms involved. After the adsorption of iodide and iodate, two characteristic peaks of elemental iodine between 600 and 650 eV can be detected on Ag@PPy (Fig. S11†), which proved the existence of iodine anions after adsorption. Fig. 3d–f show the high-resolution XPS spectrum of the elements Ag, N and I after the reaction between Ag@PPy and I−/IO3−. For the pristine Ag@PPy materials, the binding energy peaks at 373.78 and 367.78 eV corresponding to Ag 3d3/2 and Ag 3d5/2 indicated the presence of Ag0 (Fig. 3d).37 In the N 1s spectrum (Fig. 3e), the peaks at 401.04, 399.44 and 398.49 eV could be attributed to the positively charged nitrogen atoms (N+), amine (–NH–) and imine (–N
), respectively, while the peak at 406.06 eV was assigned to NO3− in PPy.30,41,64 Positively charged nitrogen functional groups from PPy were considered to be the most favorable for adsorbing anions. After the adsorption of I− anions, the peaks at 374.08 and 368.08 eV can be attributed to the Ag+ in AgI.13 However, most of the Ag0 phase was retained after removal of IO3−, indicating that a portion of the metallic silver participated in the reaction to form the AgIO3 phase. Impressively, the three peaks of the N functional groups all shifted slightly to higher binding energy, indicating the strong interaction between N atoms and I−/IO3−. Additionally, the peak of NO3− that disappeared after adsorption of the two iodine species also provided evidence for a possible ion exchange mechanism. The characteristic peak of I− was observed at 630.78 and 619.28 eV and that of IO3− was seen at 634.88 and 623.38 eV (Fig. 3f).9 Notably, trace amounts of I− were detected after IO3− removal, indicating the reduction of IO3− to I− on the Ag@PPy surface in addition to its adsorption. It has been reported that IO3− can be reduced to I−via electron transfer of positively charged nitrogen atoms and the reduction potential of Ag+.65 Considering that the AgI phase was not detected by XRD analysis after IO3− adsorption (Fig. 3a), it is guessed that the reduced I− was released back into solution or adsorbed on the PPy surface. To confirm this hypothesis, the concentration of I− in the solution after the adsorption of IO3− was determined using UV–vis. As shown in Fig. S12,† I− was not detected or was below the minimum detection limit. These effects enable the reduced I− to be adsorbed on the Ag@PPy surfaces. Therefore, the primary absorption mechanism of IO3− is the same as that of the removal of I− by Ag@PPy, i.e., electrostatic interaction and chemisorption, while a small portion of IO3− was reduced to I−.
Moreover, the corresponding STEM (Fig. 4) results provide further structural information for the adsorbed Ag@PPy. After the removal of I−/IO3−, the positively charged nitrogen atoms in the polymer matrix enriched the I−/IO3− from the solution into the polypyrrole by electrostatic interaction. For I−, large amounts of iodide were attracted to the surface of the silver particles due to the strong nucleophilic interaction between I− and Ag. At the same time, the binding of the strong nucleophile I− on silver nanoparticles will contribute to the surface charge separation of the nano-silver and promote its oxidation by dissolved oxygen in solution.66 The Ag+ produced on the silver surface will immediately combine with the adjacent I− to form AgI precipitate to remove iodide from the system. It is also confirmed from the elemental distribution that the Ag nanocrystals are converted into AgI nanocrystals inside the polypyrrole, as shown in Fig. 4d–f, and a small amount of I− ions were enriched in the outer polypyrrole through electrostatic interaction. This means that AgI was the main adsorbed product, consistent with the XRD results (Fig. 3a). Compared with iodide, a lower uptake efficiency for iodate by the Ag@PPy was observed owing to low affinity in this study (Fig. 2a). Additionally, the different uptake rates of iodide and iodate on Ag@PPy may be related to the diffusion process of the active binding site. These results were also supported by molecular size and spatial effects, since iodate has a triangular pyramidal molecular geometry with a molecular size (>4 Å) that is approximately twice as large as iodide.67 Therefore, it was observed that the adsorption of IO3− by Ag@PPy can be attributed to the major external surface reactions, such as electrostatic interaction and reduction reactions. The high-resolution TEM image for iodate removal also detected a distinct Ag phase rather than an AgIO3 phase, with iodine distributed mainly on the outer surface and in small amounts within the shell (Fig. 4g–l), corresponding with the XRD. More importantly, the used adsorbent still maintained the core–shell structure after absorbing iodide and iodate, which is strongly consistent with the adsorption mechanism of pre-enrichment followed by stable immobilization.
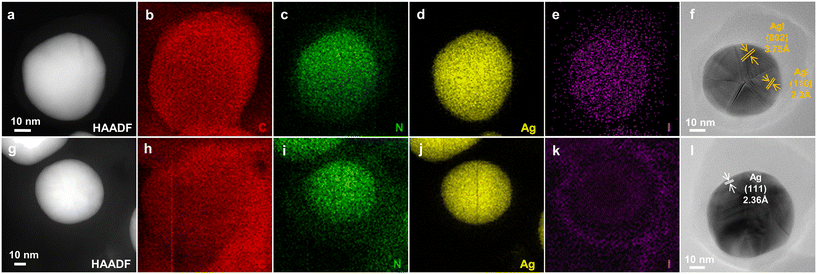 |
| Fig. 4 STEM images and elemental mapping of Ag@PPy after I−/IO3− adsorption. (a and g) High-resolution HAADF images and (b–e and h–k) STEM-XEDS elemental mappings of C, N, Ag, and I, respectively. (f and l) High-resolution TEM images of Ag@PPy after I− and IO3− adsorption, respectively. | |
Combining the above discussions, these excellent features originated from unique sorption mechanisms; first, the polypyrrole shell provided electrostatic interaction as a key driving force to pre-enrich iodide and iodate anions, and then encapsulated silver nanoparticles as a reactive center to achieve immobilization of I− and IO3− (Fig. 5). In comparison, the reaction pathway of silver with I− was different from that of IO3−, in that Ag was almost entirely converted to the AgI phase after I− adsorption, while a small portion of AgIO3 and an extremely small amount of reduced I− were identified after IO3− capture. Moreover, since the powder properties of Ag@PPy would limit further large-scale column test, we propose several strategies to prepare micron-scale bead-like composites appropriate to actual field situations. Polyethersulfone, polyacrylonitrile and sodium alginate have been chosen as support hosts for composite granulation,15,68 and cross-scale macro-assembled composites are expected to be applied to iodine removal in actual liquid waste systems. These future challenges also provide us a new opportunity to fully explore the benefits of silver-based polymers in the remediation of iodine-contaminated water.
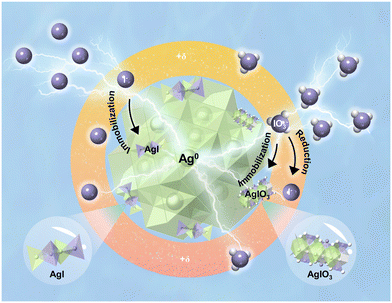 |
| Fig. 5 A conceptual model for iodide and iodate removal with Ag@PPy. | |
4. Conclusions
Radioactive iodide and iodate coexist in contaminated wastewater systems at low concentrations, but few adsorbents can effectively adsorb low-concentration I− and IO3− simultaneously. Here, we present a design philosophy for the efficient removal of I− and IO3− and obtain core–shell silver nanoparticles encapsulated within polypyrrole. The developed Ag@PPy exhibits not only a record-high value of 788.7 mg g−1 for I−, surpassing all reported inorganic adsorbents, but also a desirable uptake capacity of 133.9 mg g−1 for IO3−. A synergetic pre-enrichment and immobilization mechanism was confirmed, in which chemical reaction gives rise to the formation of AgI and AgIO3. Importantly, coupled with the electrostatic interaction and high reaction affinity for iodine, Ag@PPy possesses a marvelous purification capacity of up to 98.98% for I− and 98.29% for IO3− at low iodine concentration. The removal rates of I− and IO3− can reach 98.09% and 98.18%, respectively, even in the presence of competing ions. Moreover, its potential application is also demonstrated by the selective removal of I− and IO3− from a simulated Hanford wastewater system in the presence of a large excess of SiO32−, Cl−, SO42−, and CO32−. Ag@PPy combines the features of high adsorption capacity, excellent selectivity and stability, demonstrating the powerful potential for removing and immobilizing I− and IO3−via heterogeneous synergistic effects, and thus provides a new approach to design efficient adsorbents for in-depth removal from liquid radioactive waste.
Conflicts of interest
There are no conflicts of interest to declare.
Acknowledgements
This work was supported by the National Natural Science Foundation of China (22076155, 21976148, 11905177); the Project of Spent Fuel Reprocessing (KY20007); Sichuan Science and Technology Program (2020JDRC0068); the Project of Science and Technology Department of Sichuan Province (No. 2021JDTD0019); the Project of State Key Laboratory of Environment-friendly Energy Materials, Southwest University of Science and Technology (No. 18fksy0215, 20fksy12).
References
- L. He, L. Chen, X. Dong, S. Zhang, M. Zhang, X. Dai, X. Liu, P. Lin, K. Li, C. Chen, T. Pan, F. Ma, J. Chen, M. Yuan, Y. Zhang, L. Chen, R. Zhou, Y. Han, Z. Chai and S. Wang, A nitrogen-rich covalent organic framework for simultaneous dynamic capture of iodine and methyl iodide, Chem, 2021, 7, 699–714 CAS.
- E. A. Cordova, V. Garayburu-Caruso, C. I. Pearce, K. J. Cantrell, J. W. Morad, E. C. Gillispie, B. J. Riley, F. C. Colon, T. G. Levitskaia, S. A. Saslow, O. Qafoku, C. T. Resch, M. J. Rigali, J. E. Szecsody, S. M. Heald, M. Balasubramanian, P. Meyers and V. L. Freedman, Hybrid Sorbents for 129I Capture from Contaminated Groundwater, ACS Appl. Mater. Interfaces, 2020, 12, 26113–26126 CrossRef CAS PubMed.
- Y. Zhang, L. He, T. Pan, J. Xie, F. Wu, X. Dong, X. Wang, L. Chen, S. Gong, W. Liu, L. Kang, J. Chen, L. Chen, L. Chen, Y. Han and S. Wang, Superior Iodine Uptake Capacity Enabled by an Open Metal-Sulfide Framework Composed of Three Types of Active Sites, CCS Chem., 2023, 5, 1540–1548 CrossRef CAS.
- G. Steinhauser, Fukushima's Forgotten Radionuclides: A Review of the Understudied Radioactive Emissions, Environ. Sci. Technol., 2014, 48, 4649–4663 CrossRef CAS.
- Y. Zhao, J. Li, L. Chen, Q. Guo, L. Li, Z. Chai and S. Wang, Efficient removal of iodide/iodate from aqueous solutions by Purolite A530E resin, J. Radioanal. Nucl. Chem., 2023, 332, 1193–1202 CrossRef CAS.
- X. Hou, V. Hansen, A. Aldahan, G. R. Possnert, O. C. Lind and G. Lujaniene, A review on speciation of iodine-129 in the environmental and biological samples, Anal. Chim. Acta, 2009, 632, 181–196 CrossRef CAS.
- T. Nagata and K. Fukushi, Prediction of iodate adsorption and surface speciation on oxides by surface complexation modeling, Geochim. Cosmochim. Acta, 2010, 74, 6000–6013 CrossRef CAS.
- C. I. Pearce, E. A. Cordova, W. L. Garcia, S. A. Saslow, K. J. Cantrell, J. W. Morad, O. Qafoku, J. Matyas, A. E. Plymale, S. Chatterjee, J. Kang, F. C. Colon, T. G. Levitskaia, M. J. Rigali, J. E. Szecsody, S. M. Heald, M. Balasubramanian, S. Wang, D. T. Sun, W. L. Queen, R. Bontchev, R. C. Moore and V. L. Freedman, Evaluation of materials for iodine and technetium immobilization through sorption and redox-driven processes, Sci. Total Environ., 2020, 716, 136167 CrossRef CAS PubMed.
- N. Wang, R. Xiong, G. Zhang, R. Liu, X. He, S. Huang, H. Liu and J. Qu, Species transformation and removal mechanism of various iodine species at the Bi2O3@MnO2 interface, Water Res., 2022, 223, 118965 CrossRef CAS PubMed.
- R. J. Konings, T. Wiss and O. Benes, Predicting material release during a nuclear reactor accident, Nat. Mater., 2015, 14, 247–252 CrossRef CAS.
- K. Tokunaga, Y. Takahashi, K. Tanaka and N. Kozai, Effective removal of iodate by coprecipitation with barite: Behavior and mechanism, Chemosphere, 2021, 266, 129104 CrossRef CAS PubMed.
- S. Mushtaq, S.-J. Yun, J. E. Yang, S.-W. Jeong, H. E. Shim, M. H. Choi, S. H. Park, Y. J. Choi and J. Jeon, Efficient and selective removal of radioactive iodine anions using engineered nanocomposite membranes, Environ. Sci.: Nano, 2017, 4, 2157–2163 RSC.
- H. Li and Y. Li, Ag-doped silicon-based nanospheres for the efficient capture and remove of iodide anions from solutions, Appl. Surf. Sci., 2019, 496, 143707 CrossRef CAS.
- Z. Tauanov and V. J. Inglezakis, Removal of iodide from water using silver nanoparticles-impregnated synthetic zeolites, Sci. Total Environ., 2019, 682, 259–270 CrossRef CAS PubMed.
- Q. Zhao, G. Chen, Z. Wang, M. Jiang, J. Lin, L. Zhang, L. Zhu and T. Duan, Efficient removal and immobilization of radioactive iodide and iodate from aqueous solutions by bismuth-based composite beads, Chem. Eng. J., 2021, 426, 131629 CrossRef CAS.
- Y. Xiong, B. Dang, C. Wang, H. Wang, S. Zhang, Q. Sun and X. Xu, Cellulose Fibers Constructed Convenient Recyclable 3D Graphene-Formicary-like δ-Bi2O3 Aerogels for the Selective Capture of Iodide, ACS Appl. Mater. Interfaces, 2017, 9, 20554–20560 CrossRef CAS.
- N. Wang, G. Zhang, R. Xiong, R. Liu, H. Liu and J. Qu, Synchronous Moderate Oxidation and Adsorption on the Surface of γ-MnO2 for Efficient Iodide Removal from Water, Environ. Sci. Technol., 2022, 56, 9417–9427 CrossRef CAS.
- T. J. Robshaw, J. Turner, S. Kearney, B. Walkley, C. A. Sharrad and M. D. Ogden, Capture of aqueous radioiodine species by metallated adsorbents from wastestreams of the nuclear power industry: a review, SN Appl. Sci., 2021, 3, 843 CrossRef CAS.
- J. Kang, F. Cintron-Colon, H. Kim, J. Kim, T. Varga, Y. Du, O. Qafoku, W. Um and T. G. Levitskaia, Removal of iodine (I− and IO3−) from aqueous solutions using CoAl and NiAl layered double hydroxides, Chem. Eng. J., 2022, 430, 132788 CrossRef CAS.
- C. Copeman, H. A. Bicalho, M. W. Terban, D. Troya, M. Etter, P. L. Frattini, D. M. Wells and A. J. Howarth, Adsorptive Removal of Iodate Oxyanions from Water using a Zr-based Metal-Organic Framework, Chem. Commun., 2023, 59, 3071–3074 RSC.
- L. Iglesias, M. G. Álvarez, R. J. Chimentão, J. L. Leganés and F. Medina, On the role of ultrasound and mechanical stirring for iodide adsorption by calcined layered double hydroxides, Appl. Clay Sci., 2014, 91–92, 70–78 CrossRef CAS.
- W. Xu, W. Zhang, J. Kang and B. Li, Facile synthesis of mesoporous Fe-based MOFs loading bismuth with high speed adsorption of iodide from solution, J. Solid State Chem., 2019, 269, 558–565 CrossRef CAS.
- J. S. Hoskins, T. Karanfil and S. M. Serkiz, Removal and Sequestration of Iodide Using Silver-Impregnated Activated Carbon, Environ. Sci. Technol., 2002, 36, 784–789 CrossRef CAS PubMed.
- X. Zhang and Y. Liu, Nanomaterials for radioactive wastewater decontamination, Environ. Sci.: Nano, 2020, 7, 1008–1040 RSC.
- M. A. Ruiz Fresneda, J. Delgado Martín, J. Gómez Bolívar, M. V. Fernández Cantos, G. Bosch-Estévez, M. F. Martínez Moreno and M. L. Merroun, Green synthesis and biotransformation of amorphous Se nanospheres to trigonal 1D Se nanostructures: impact on Se mobility within the concept of radioactive waste disposal, Environ. Sci.: Nano, 2018, 5, 2103–2116 RSC.
- R. M. Asmussen, A. Westesen, E. A. Cordova, A. L. Fujii Yamagata, P. P. Schonewill, A. C. Moore, A. Bourchy, S. A. Saslow, G. L. Smith, B. J. Riley and R. S. Skeen, Iodine Removal from Carbonate-Containing Alkaline Liquids Using Strong Base Resins, Hybrid Resins, and Silver Precipitation, Ind. Eng. Chem. Res., 2023, 62, 3271–3281 CrossRef CAS.
- C. Li, N. Chen, Y. Zhao, R. Li and C. Feng, Polypyrrole-grafted peanut shell biological carbon as a potential sorbent for fluoride removal: Sorption capability and mechanism, Chemosphere, 2016, 163, 81–89 CrossRef CAS.
- V. Chandra and K. S. Kim, Highly selective adsorption of Hg2+ by a polypyrrole-reduced graphene oxide composite, Chem. Commun., 2011, 47, 3942–3944 RSC.
- Y. Wang, C. Liang, C. Fan, J. Chen, Z. Zhang and H. Liu, Composite modification of carbon fiber cathode with tree-like branched polypyrrole-microwires and polyaniline-nanorods for enhancing hexavalent chromium reduction, Environ. Sci.: Nano, 2023, 10, 891–901 RSC.
- S. Hong, S. Deng, X. Yao, B. Wang, Y. Wang, J. Huang and G. Yu, Bromate removal from water by polypyrrole tailored activated carbon, J. Colloid Interface Sci., 2016, 467, 10–16 CrossRef CAS.
- Y. Lei, X. Qian, J. Shen and X. An, Integrated Reductive/Adsorptive Detoxification of Cr(VI)-Contaminated Water by Polypyrrole/Cellulose Fiber Composite, Ind. Eng. Chem. Res., 2012, 51, 10408–10415 CrossRef CAS.
- S. Zhang, Y. Shao, J. Liu, I. A. Aksay and Y. Lin, Graphene-polypyrrole nanocomposite as a highly efficient and low cost electrically switched ion exchanger for removing ClO4− from wastewater, ACS Appl. Mater. Interfaces, 2011, 3, 3633–3637 CrossRef CAS.
- A. Chen, K. Kamata, M. Nakagawa, T. Iyoda, H. Wang and X. Li, Formation process of silver-polypyrrole coaxial nanocables synthesized by redox reaction between AgNO3 and pyrrole in the presence of poly(vinylpyrrolidone), J. Phys. Chem. B, 2005, 109, 18283–18288 CrossRef CAS PubMed.
- A. Chen, H. Wang and X. Li, One-step process to fabricate Ag–polypyrrole coaxial nanocables, Chem. Commun., 2005, 1863–1864 RSC.
- P. Dallas, D. Niarchos, D. Vrbanic, N. Boukos, S. Pejovnik, C. Trapalis and D. Petridis, Interfacial polymerization of pyrrole and in situ synthesis of polypyrrole/silver nanocomposites, Polymer, 2007, 48, 2007–2013 CrossRef CAS.
- S. Jing, S. Xing, L. Yu and C. Zhao, Synthesis and characterization of Ag/polypyrrole nanocomposites based on silver nanoparticles colloid, Mater. Lett., 2007, 61, 4528–4530 CrossRef CAS.
- Z. Tian, T. S. Chee, L. Zhu, T. Duan, X. Zhang, L. Lei and C. Xiao, Comprehensive comparison of bismuth and silver functionalized nickel foam composites in capturing radioactive gaseous iodine, J. Hazard. Mater., 2021, 417, 125978 CrossRef CAS.
- J. Duchet, R. Legras and S. Demoustier-Champagne, Chemical synthesis of polypyrrole: structure-properties relationship, Synth. Met., 1998, 98, 113–122 CrossRef CAS.
- Y. Wei, Y. Zhao, L. Li, X. Yang, X. Yu and G. Yan, Magnetic ionic liquid-assisted synthesis of polypyrrole/AgCl nanocomposites, Polym. Adv. Technol., 2010, 21, 742–745 CrossRef CAS.
- Y. C. Liu, B. J. Hwang, W. J. Jian and R. Santhanam,
In situ cyclic voltammetry-surface-enhanced Raman spectroscopy: Studies on the doping-undoping of polypyrrole film, Thin Solid Films, 2000, 374, 85–91 CrossRef CAS.
- Y. Han, T. Wang, T. Li, X. Gao, W. Li, Z. Zhang, Y. Wang and X. Zhang, Preparation and electrochemical performances of graphene/polypyrrole nanocomposite with anthraquinone-graphene oxide as active oxidant, Carbon, 2017, 119, 111–118 CrossRef CAS.
- J. Luo, X. Du, F. Gao, Y. Yang, X. Hao, S. Li, X. Hao, K. Tang and G. Guan, Iodide ion trapping polypyrrole film: Selective capture of
iodide ions by electrochemically switched ion extraction (ESIE) process, Chem. Eng. J., 2020, 380, 122529 CrossRef CAS.
- Z. Shi, H. Wang, T. Dai and Y. Lu, Room temperature synthesis of Ag/polypyrrole core–shell nanoparticles and hollow composite capsules, Synth. Met., 2010, 160, 2121–2127 CrossRef CAS.
- J. Liu, J. Wang, X. Yu, L. Li and S. Shang, One-pot synthesis of polypyrrole/AgCl composite nanotubes and their antibacterial properties, Micro Nano Lett., 2015, 10, 50–53 CrossRef.
- H. Li, Y. Li, B. Li, D. Liu and Y. Zhou, Highly selective anchoring silver nanoclusters on MOF/SOF heterostructured framework for efficient adsorption of radioactive iodine from aqueous solution, Chemosphere, 2020, 252, 126448 CrossRef CAS PubMed.
- Z. Xu, P. Zhang, W. Teng, M. He, S. Liu, K. Li and H. Wang, Anchoring of Ag into binary PBs-SOF heterostructure composite enhances selective radioactive iodine adsorption: Intrinsic affinity and interconnected framework, J. Environ. Chem. Eng., 2023, 11, 110139 CrossRef CAS.
- M. Cheng, Y. Luo, J. Geng, R. Cui, Y. Qu, L. Sun, Q. Dou and H. Fu, Adsorption behavior of iodide ion by silver-doped zeolite 4A in LiCl-KCl molten salt, Adv. Powder Technol., 2022, 33, 103415 CrossRef CAS.
- J. Chen, A. Gu, E. Djam Miensah, Y. Liu, P. Wang, P. Mao, C. Gong, Y. Jiao, K. Chen, Z. Zhang and Y. Yang, Core-shell ZnO@Cu2O encapsulated Ag NPs nanocomposites for photooxidation-adsorption of iodide anions under visible light, Sep. Purif. Technol., 2021, 262, 118328 CrossRef CAS.
- J. Kim, J. Kang and W. Um, Simultaneous removal of cesium and iodate using prussian blue functionalized CoCr layered double hydroxide (PB-LDH), J. Environ. Chem. Eng., 2022, 10, 107477 CrossRef CAS.
- P. Liu, T. Chen and J.-G. Zheng, Removal of iodate from aqueous solution using diatomite/nano titanium dioxide composite as adsorbent, J. Radioanal. Nucl. Chem., 2020, 324, 1179–1188 CrossRef CAS.
- S. Han, W. Um and W. S. Kim, Development of bismuth-functionalized graphene oxide to remove radioactive iodine, Dalton Trans., 2019, 48, 478–485 RSC.
- J. Li, M. Wang, G. Liu, L. Zhang, Y. He, X. Xing, Z. Qian, J. Zheng and C. Xu, Enhanced Iodide Removal from Water by Nano-Silver Modified Anion Exchanger, Ind. Eng. Chem. Res., 2018, 57, 17401–17408 CrossRef CAS.
- X. Zhang, P. Gu, X. Li and G. Zhang, Efficient adsorption of radioactive iodide ion from simulated wastewater by nano Cu2O/Cu modified activated carbon, Chem. Eng. J., 2017, 322, 129–139 CrossRef CAS.
- P. Mao, J. Jiang, Y. Pan, C. Duanmu, S. Chen, Y. Yang, S. Zhang and Y. Chen, Enhanced Uptake of Iodide from Solutions by Hollow Cu-Based Adsorbents, Materials, 2018, 11, 769 CrossRef.
- P. Mao, L. Qi, X. Liu, Y. Liu, Y. Jiao, S. Chen and Y. Yang, Synthesis of Cu/Cu2O hydrides for enhanced removal of iodide from water, J. Hazard. Mater., 2017, 328, 21–28 CrossRef CAS PubMed.
- Y.-Y. Chen, S.-H. Yu, Q.-Z. Yao, S.-Q. Fu and G.-T. Zhou, One-step synthesis of Ag2O@Mg(OH)2 nanocomposite as an efficient scavenger for iodine and uranium, J. Colloid Interface Sci., 2018, 510, 280–291 CrossRef CAS PubMed.
- R. M. Asmussen, J. J. Neeway, A. R. Lawter, A. Wilson and N. P. Qafoku, Silver-based getters for 129I removal from low-activity waste, Radiochim. Acta, 2016, 104, 905–913 CrossRef CAS.
- L. Zhu, D. Sheng, C. Xu, X. Dai, M. A. Silver, J. Li, P. Li, Y. Wang, Y. Wang, L. Chen, C. Xiao, J. Chen, R. Zhou, C. Zhang, O. K. Farha, Z. Chai, T. E. Albrecht-Schmitt and S. Wang, Identifying the Recognition Site for Selective Trapping of 99TcO4− in a Hydrolytically Stable and Radiation Resistant Cationic Metal–Organic Framework, J. Am. Chem. Soc., 2017, 139, 14873–14876 CrossRef CAS PubMed.
- J. Seon and Y. Hwang, Cu/Cu2O-immobilized cellulosic filter for enhanced iodide removal from water, J. Hazard. Mater., 2021, 409, 124415 CrossRef CAS.
- D. Li, D. I. Kaplan, K. A. Price, J. C. Seaman, K. Roberts, C. Xu, P. Lin, W. Xing, K. Schwehr and P. H. Santschi, Iodine immobilization by silver-impregnated granular activated carbon in cementitious systems, J. Environ. Radioact., 2019, 208–209, 106017 CrossRef CAS.
- J. Li, Y. Wang, W. Guo, X. Xie, L. Zhang, Y. Liu and S. Kong, Iodine mobilization in groundwater system at Datong basin, China: evidence from hydrochemistry and fluorescence characteristics, Sci. Total Environ., 2014, 468–469, 738–745 CrossRef CAS PubMed.
- F. Yu, Y. Chen, Y. Wang, C. Liu and W. Ma, Enhanced removal of iodide from aqueous solution by ozonation and subsequent adsorption on Ag-Ag2O modified on Carbon Spheres, Appl. Surf. Sci., 2018, 427, 753–762 CrossRef CAS.
- E. J. Liang, X. L. Ye and W. Kiefer, Interaction of halide and halate ions with colloidal silver and their influence on surface-enhanced Raman scattering of pyridine with near-infrared excitation, Vib. Spectrosc., 1997, 15, 69–78 CrossRef CAS.
- Y. Li, R. Qian, K. Imaeda and H. Inokuchi, Behavior of the High Temperature Conductivity of Polypyrrole Nitrate Films, Polym. J., 1994, 26, 535–538 CrossRef CAS.
- R. M. Asmussen, J. Matyas, N. P. Qafoku and A. A. Kruger, Silver-functionalized silica aerogels and their application in the removal of iodine from aqueous environments, J. Hazard. Mater., 2019, 379, 119364 CrossRef CAS.
- P. Mulvaney, T. Linnert and A. Henglein, Surface chemistry of colloidal silver in aqueous solution: observations on chemisorption and reactivity, J. Phys. Chem., 1991, 95, 7843–7846 CrossRef CAS.
- S. Choung, W. Um, M. Kim and M. G. Kim, Uptake mechanism for iodine species to black carbon, Environ. Sci. Technol., 2013, 47, 10349–10355 CAS.
- R. Liu, G. Chen, Z. Wang, Q. Zhao, L. Wu, Q. Li, R. Tian, X. Chen, X. Li, Z. Chen, L. Zhu, J. Chen and T. Duan, Calcium-Intercalated Zirconium Phosphate by Granulation: A Strategy for Enhancing Adsorption Selectivity of Strontium and Cesium from Liquid Radioactive Waste, Inorg. Chem., 2023, 62, 5799–5809 CrossRef CAS PubMed.
|
This journal is © The Royal Society of Chemistry 2024 |