DOI:
10.1039/D4DT02237H
(Paper)
Dalton Trans., 2024,
53, 16367-16376
Luminescence of nanocrystalline BaFCl codoped with Eu2+/3+ and Tb3+†
Received
5th August 2024
, Accepted 17th September 2024
First published on 24th September 2024
Abstract
BaFCl:Eu,Tb nanocrystals were synthesized via hot-injection thermolysis of metal chlorodifluoroacetates and their luminescence response characterized between 80 and 430 K. Though unintended, partial reduction of Eu3+ to Eu2+ occurred during the synthesis stage, leading to luminescent nanocrystals coactivated by Eu2+, Eu3+, and Tb3+. Their emission was dominated by Eu2+, specifically by radiative transitions 4f7 (6P7/2) → 4f7 (8S7/2) and 4f65d1 (lowest level) → 4f7 (8S7/2). Owing to the thermal coupling between 4f7 (6P7/2) and 4f65d1 (lowest) levels, line-like emission from this manifold at 80 K evolved into band-like emission at 430 K. The change in the shape of the emission profile of Eu2+ was used to demonstrate that BaFCl:Eu,Tb may serve to realize bandshape luminescence thermometry as a more straightforward alternative to the usual ratiometric approach. The energy gap between 4f7 (6P7/2) and 4f65d1 (lowest) levels and their radiative transition probabilities were estimated from time-resolved variable-temperature decays.
Introduction
Alkaline-earth fluorohalides of formula MFX (M = Ca, Sr, Ba; X = Cl, Br, I) have been extensively used as hosts for divalent (e.g., Eu2+) and trivalent rare-earth ions (e.g., Sm3+, Eu3+, Er3+, Tm3+). Isovalent and aliovalent doping of these wide bandgap insulators render them functional materials on account of their photoluminescence and photochemical response. In this way, pressure1–4 and temperature5–7 luminescent sensors, photostimulable X-ray storage phosphors,8–10 and photoexcitable storage phosphors sensitive to X-ray and UV-C radiation have been realized.11–15 The crystal structure of fluorohalides and coordination polyhedra of rare-earth dopants (RE) are shown in Fig. 1. Fluorohalides display the archetypical matlockite structure. The unit cell is tetragonal and MF4X5 capped square antiprisms of C4v symmetry serve as building blocks (Fig. 1a and b). The alkaline-earth metal has four fluoride and five heavy halide anions as nearest neighbors. The assembly of MF4X5 polyhedra leads to –F–M–X–X–M–F– double layers stacked along the c axis. Divalent rare-earth ions such as Eu2+ substitute for the alkaline-earth metal giving rise to REIIF4X5 polyhedra (Fig. 1b).16 In fact, EuFCl and EuFBr are well-known matlockite structures. By contrast, the local atomic environment of trivalent rare-earth dopants is still under debate. Knowledge of the symmetry of the site(s) occupied by these dopants ions was first gained via electron paramagnetic resonance16–19 and later through fluorescence spectroscopy.13,14,20,21 Recently, our group conducted X-ray absorption studies on Yb3+-doped MFX nanocrystals and demonstrated that trivalent rare-earth ions do not sit in the matlockite structure as REIIIF4X5 polyhedra.22 Instead, their first coordination shell consists of anionic donors of identical or similar radii. Two alternative models were proposed to rationalize experimental radial distribution functions. In the first model, the rare-earth coordination was described by a distorted capped square antiprism of formula REIIIF9 and Cs symmetry (Fig. 1c). The second model entailed distorted capped trigonal prisms of formula REIIIO4F3 and C1 symmetry (Fig. 1d). Insight into the local structure of rare-earth-doped fluorohalides is relevant to delineate energy-transfer pathways that directly impact photoluminescence response (e.g., host-to-activator charge-transfer energies) and to establish the structural bases of photochemical reactions (e.g., spatial correlation between electron and hole traps).
 |
| Fig. 1 (a) Unit cell of matlockite MFX. (b) Coordination polyhedra of divalent metals in the matlockite structure. (c and d) Possible coordination polyhedra of trivalent rare-earth metals.22 Point group symmetries are indicated for each polyhedron. | |
A distinct trait of alkaline-earth fluorohalides is their tunability. Chemical substitution of the metal (M) or of the heavy halogen (X) provides a lever to systematically tune structural and electronic features directly relevant to photoluminescence and photochemistry. On the structural side, chemical substitutions enable fine tuning of metal–halogen bond distances and covalency.23 This tuning enables adjustment of, for example, the position of the 4fN−15d1 emissive levels of rare-earth dopants such as Sm2+ (4f6) and Eu2+ (4f7) relative to their ground states.24–33 On the electronic side, chemical substitutions serve to tune band gaps,34–36 which in turn impact the energy separation between rare-earth levels and band edges. The placement of the rare-earth activator ground and excited states relative to each other and to the host's band edges governs both photoluminescence (e.g., charge-transfer excitation energies)37,38 and photochemistry (e.g., photo(thermal)ionization of RE2+ electron traps into the conduction band).39 In parallel to achieving compositional control of structural and electronic features, using the phosphor's grain size to tailor functionality has gained traction over the past two decades. Transitioning from micron- to nano-sized crystals is obviously required in biophotonics.40 When it comes to optical data storage, studies that unveiled the potential of rare-earth-doped alkaline-earth fluorides and fluorohalides as photoexcitable storage phosphors have indicated that size reduction positively impacts a number of aspects, including higher storage density (due to a higher concentration of oxygen defects), faster read out speed (due to shorter luminescence decays), and reduced energy consumption (due to lower bleaching thresholds).11,13,41–43 As a result, advances have been made in the field of rare-earth-doped alkaline-earth fluorohalide nanophosphors. These advances encompass both synthetic developments and understanding of photoluminescence and photochemical response.
As a part of that effort, our group initiated an investigation of the luminescence of BaFCl:Eu3+,Tb3+ nanocrystals synthesized via hot-injection. At first, we aimed to exploit the well-known temperature-dependent Tb3+-to-Eu3+ energy transfer44–46 for luminescence thermometry purposes. Unexpectedly, we observed partial reduction of Eu3+ to Eu2+ during the synthesis stage, leading to BaFCl coactivated by Eu2+, Eu3+, and Tb3+. More importantly, Eu2+ emission dominated the nanocrystals’ response. To the best of our knowledge, reports on BaFCl codoped with mixed-valence europium are scarce.47 In this article, we present a study of the luminescence of BaFCl:Eu2+/3+,Tb3+ nanocrystals. Excitation, emission, and energy-transfer pathways are probed at room temperature and the temperature-dependent emission profile of Eu2+ is characterized between 80 and 430 K. The possibility of exploiting it for bandshape thermometry rather than ratiometric thermometry is discussed.
Experimental
Synthesis of BaFCl:Eu,Tb nanocrystals
Nanocrystal synthesis was carried out under nitrogen atmosphere using standard Schlenk techniques. BaCO3 (99.98%), Eu2O3 (99.9%), Tb(acac)3 (99.9%), CF2ClCOOH (98%), oleic acid (90%), 1-octadecene (90%), and trioctylphosphine (97%) were used as reagents. All chemicals were purchased from Sigma Aldrich and used without further purification. CF2ClCOOH and trioctylphosphine were stored under nitrogen. BaFCl:Eu,Tb nanocrystals (3.0 mol% of each codopant) were synthesized via hot-injection thermolysis of metal chlorodifluoroacetate precursors.48 BaCO3 (0.940 mmol), Eu2O3 (0.015 mmol), and Tb(acac)3 (0.030 mmol) were added to a solution containing 3 mL of double-deionized water and 3 mL of CF2ClCOOH. The resulting suspension was heated at 65 °C for 12 h until a colorless, optically transparent solution was obtained. Polycrystalline chlorodifluoroacetates were obtained after solvent evaporation at 65 °C for 48 h under a constant nitrogen flow rate of 200 mL min−1. Once evaporation was completed, 4 mL of oleic acid and 1 mL of 1-octadecene were added to the flask containing metal precursors (flask A). 4 mL of oleic acid and 7 mL of 1-octadecene were added to a second flask (flask B). Flasks A and B were heated to 115 °C under vacuum (≈2 mTorr); magnetic stirring was employed throughout. After 45 min, flask B was switched to a nitrogen atmosphere, a thermocouple was placed inside the flask in direct contact with the solution, and 8 mL of trioctylphosphine were injected. Then, the temperature of flask B was increased to 275 °C and the solution in flask A was quickly injected. The reaction proceeded for 90 min at 275 °C and was quenched to room temperature using a stream of air. BaFCl:Eu,Tb nanocrystals were isolated by adding 40 mL of EtOH (200 proof) and centrifuging at 8000 rpm for 10 min. The resulting precipitate was resuspended using 5 mL of toluene, reprecipitated using 7 mL of EtOH and 2 mL of MeOH, centrifuged at 8000 rpm for 10 min, and dried in vacuum at room temperature. Polycrystalline BaFCl:Eu,Tb thus obtained was employed for chemical, morphological, structural, and luminescence analyses.
Powder X-ray diffraction (PXRD)
PXRD patterns were collected using a Bruker D2 Phaser diffractometer operated at 30 kV and 10 mA. Cu Kα radiation (λ = 1.5418 Å) was employed. A nickel filter was used to remove Cu Kβ. Diffractograms were collected in the 10–70° 2θ range using a step size of 0.012° and a step time of 1.5 s.
Transmission electron microscopy (TEM)
TEM images were obtained using a Thermo Scientific Talos F200X G2 S/TEM microscope operated at 200 kV. Nanocrystals were dispersed in methanol and sonicated for 30 min. Then, a drop of the resulting suspension was deposited on a 200 mesh Cu grid coated with a Lacey carbon film (Ted Pella Inc.).
Rietveld analysis
Rietveld analysis49,50 of PXRD data was performed using the General Structure Analysis System II (GSAS-II).51 The average crystal structure of BaFCl:Eu,Tb nanocrystals was refined using the tetragonal P4/nmm space group. The following parameters were refined: (1) scale factor; (2) background, which was modeled using a shifted Chebyschev function; (3) instrument parameters, including diffractometer constant and contributions to peak profile; (4) lattice constants (a and c); (5) fractional atomic coordinates of barium (zBa) and chlorine (zCl); (6) an isotropic displacement parameter for each of the atoms in the structure (UBa, UF, and UCl, constrained to UF = 3UBa and UCl = 2UBa); and (7) crystallite size and microstrain. Difference curve and Rw residual were employed to assess the quality of the refinement. Crystal structure and metal coordination polyhedra were visualized using VESTA.52
X-ray photoelectron spectroscopy (XPS)
Nanocrystals were analyzed using a Thermo Scientific Nexsa X-ray photoelectron spectrometer equipped with a hemispherical analyzer and monochromatic Al Kα sources (1486.7 eV). ≈10 mg of the sample were mounted on a regular sample holder using conductive copper tapes; care was taken to ensure a flat surface. The holder was then loaded into the entry-lock chamber and held under vacuum for more than 30 min. Once the vacuum level reached 4 × 10−7 mbar, the sample was transferred from the entry-lock to the analysis chamber, which was kept at a base pressure of ≈1.8 × 10−7 mbar throughout data acquisition. High-resolution spectra were collected for C 1s, Eu 3d, and Ba 3p core lines using a pass energy of 50 eV, an energy step size of 0.1 eV, and 100 ms per step as the dwell time. Qualitative and quantitative spectral analyses were performed using Thermo Avantage. The C 1s core line at 284.8 eV was employed for charge referencing. All peaks were fitted using pseudo-Voigt functions.
Spectrofluorometry
Luminescence analyses were conducted using a Fluorolog 3–222 fluorometer (Horiba Scientific). A xenon lamp and a 265 nm SpectraLED (Horiba Scientific) were used as excitation sources for steady-state and time-resolved measurements, respectively. A photomultiplier tube R928 (Hammamatsu Photonics) served as the detector. Steady-state spectra and time-resolved decays were first collected under ambient conditions and then variable-temperature measurements were conducted. For the latter, BaFCl:Eu,Tb nanocrystals were loaded into a VPF–800 variable-temperature stage (Lake Shore Cryotronics) and degassed at 450 K for 2 h under vacuum (≈50 mTorr) prior to data collection. Heating to 450 K (177 °C) had no effect on the chemical and structural integrity of the nanocrystals as shown by X-ray diffraction and thermal analysis (see ESI, Fig. S1†). Temperature control was provided by a Lake Shore 335–3060 controller. Spectra and decays were collected in the 80–430 K temperature window using slit widths ranging between 1 and 2 nm. A heating rate of 10 K min−1 was employed throughout. Nanocrystals were allowed to dwell for ≈20 min at the target temperature prior to data collection. All spectrofluorometric analyses were conducted using BaFCl:Eu,Tb polycrystalline solids that had not been exposed to X-ray radiation.
Results and discussion
A PXRD pattern and a representative TEM image of BaFCl:Eu,Tb nanocrystals are given in Fig. 2. Rietveld analysis confirmed the phase purity of polycrystalline BaFCl:Eu,Tb as all diffraction maxima in the pattern were indexed to the fluorochloride phase (PDF No. 024–0096); no secondary crystalline phases were observed (Fig. 2a). Refined unit cell constants, atomic coordinates, and isotropic displacement parameters are given in the ESI (see Table S1†). Diffraction maxima exhibited anisotropic microstrain broadening which complicated adequate modeling of some reflection profiles, notably that of (101) (2θ ≈ 23.7°). From a morphological standpoint, nanocrystals showed irregular spherical shape with an average diameter of 26.5 nm (Fig. 2b). These results were in line with those previously reported by our group for BaFCl:Yb,Er nanocrystals synthesized via hot-injection thermolysis of metal chlorodifluoroacetates.48 For completeness, we note that rare-earth solubility curves for BaFCl nanocrystals synthesized using the procedure described herein had shown rare-earth doping efficiencies of 90% up to a total concentration of 6 mol%.48
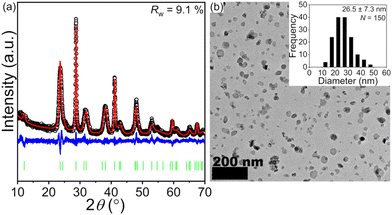 |
| Fig. 2 (a) Rietveld analysis of the PXRD pattern of BaFCl:Eu,Tb nanocrystals. Experimental data (black circles), calculated pattern (red line), difference curve (blue line, offset for clarity), and tick marks corresponding to the calculated diffraction maxima are shown (green vertical bars). (b) Electron microscopy image and nanocrystal size distribution histogram (inset). | |
We then proceeded to screen the room-temperature luminescence response of the nanocrystals. Results from these studies are summarized in Fig. 3. An emission spectrum collected under 275 nm excitation revealed the presence of three emitting centers: Eu2+, Eu3+, and Tb3+ (Fig. 3a). The spectrum was dominated by a broad band peaking at 387 nm arising from radiative relaxation of the lowest level of the Eu2+ 4f65d1 excited state (hereafter referred to as 4f65d1 (ll)) to the 4f7 (8S7/2) ground state. In addition, line emission resulting from the 4f7 (6P7/2) → 4f7 (8S7/2) zero-phonon transition of Eu2+ was observed at 363 nm. Emissions from 4f–4f transitions of Eu3+ and Tb3+ were much weaker and appeared at 486 (Tb3+, 5D4 → 7F6), 544 (Tb3+, 5D4 → 7F5), 584 (Tb3+, 5D4 → 7F4), 592 (Eu3+, 5D0 → 7F1), 612 (Eu3+, 5D0 → 7F2), and 620 nm (Tb3+, 5D4 → 7F3). Excited-state lifetimes extracted from time-resolved luminescence decays were consistent with the presence of Eu2+, Eu3+, and Tb3+ activators (Fig. 3b). Intensity-weighted average lifetimes (〈τ〉) equal to 26 μs (Eu2+, 4f65d1 (ll) → 4f7), 3.3 ms (Eu3+, 5D0 → 7F2), and 3.5 ms (Tb3+, 5D4 → 7F5) were estimated using eqn (1), where I(t) is the baseline-corrected
|  | (1) |
intensity at time
t. Similar average lifetimes were extracted
via multiexponential fits (see ESI, Fig. S2
†). The average lifetime obtained for Eu
2+ in our BaFCl:Eu,Tb nanocrystals is in line with values previously reported for Eu
2+-doped BaFCl.
24,53 All decays were nonexponential, pointing to multisite distribution of each activator within the nanocrystals and/or energy transfer between activators. While emissions from Eu
3+ and Tb
3+ were obviously expected, the presence of Eu
2+ was rather surprising considering that Eu
III2O
3 was used as the europium precursor and that no reducing agent was deliberately introduced at any point during nanocrystal synthesis. Incorporation of Eu
2+ into micro- and nanocrystalline BaFX has been extensively reported but solids were invariably synthesized under a reducing atmosphere (
e.g., N
2/H
2)
24,27,54 or using a divalent europium precursor (
e.g., EuF
2).
55–57 Alternatively, Eu
2+ may be generated
via X-ray induced photoreduction of Eu
3+ doped into BaFCl and BaFBr; however, this was not the case here. As mentioned earlier, luminescence analyses were conducted using BaFCl:Eu,Tb nanocrystals that had not been exposed to X-ray radiation. Thus, partial reduction of Eu
3+ to Eu
2+ occurred during the synthesis stage. At the present time, we are unable to pinpoint the reducing species responsible for this transformation. Control experiments showed that partial reduction of Eu
3+ also took place during the preparation of singly-doped BaFCl:Eu nanocrystals and of nanocrystals codoped with different Eu
![[thin space (1/6-em)]](https://www.rsc.org/images/entities/char_2009.gif)
:
![[thin space (1/6-em)]](https://www.rsc.org/images/entities/char_2009.gif)
Tb ratios (see ESI, Fig. S3
†).
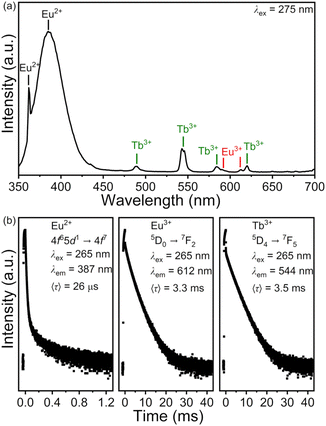 |
| Fig. 3 Room-temperature emission spectrum (a) and time-resolved luminescence decays (b) of BaFCl:Eu,Tb nanocrystals. Decays are plotted in logarithmic scale. | |
We attempted to estimate the Eu3+
:
Eu2+ ratio in BaFCl:Eu,Tb nanocrystals using X-ray photoelectron spectroscopy. However, a europium concentration of 3 mol% was too low to obtain a reliable estimate of that ratio. Thus, we performed XPS analyses on BaFCl:Eu nanocrystals featuring a 4 mol% europium concentration. Results from these analyses are shown in Fig. 4. The high-resolution Eu 3d spectrum exhibited peaks in the 3d5/2 and 3d3/2 regions and a complex shape due to the presence of shake-up and shake-down features. Nonetheless, well-established peak-fit guidelines58–60 enabled peak deconvolution and extraction of qualitative and quantitative information. The spectrum was dominated by an intense peak at ≈1136.6 eV. This peak was deconvoluted into Ba 3p1/2 and Eu 3d5/2 contributions while maintaining the area ratio of 1
:
2 between the Ba 3p3/2 and Ba 3p1/2 spin–orbit components. Deconvolution resulted in two peaks centered at ≈1135.6 and ≈1137.0 eV assigned to Eu 3d5/2 (Eu3+) and Ba 3p3/2 core lines, respectively. Though the Eu 3d5/2 peak arising from Eu3+ showed a strong overlap with the Ba 3p1/2 peak, the Eu 3d3/2 peak centered at ≈1164.7 eV unequivocally confirmed the presence of Eu3+. The presence of Eu2+ was confirmed by Eu 3d5/2 and Eu 3d3/2 peaks centered at ≈1125.9 and ≈1155.6 eV, respectively. The areas of the Eu 3d5/2 peaks corresponding to Eu3+ and Eu2+ were computed and translated to atomic percentages, which were subsequently used to estimate the Eu3+
:
Eu2+ ratio. A value of 0.8 was thus obtained, indicating that ≈56% of the Eu3+ in the initial reaction mixture had been reduced to Eu2+ (n.b., assuming that the calculated ratio was representative of the entire nanocrystal volume). Su and Sun observed Eu3+ → Eu2+ reduction in bulk solids synthesized under nitrogen or air atmosphere, but the extent of the reaction was significantly lower (<2%).47
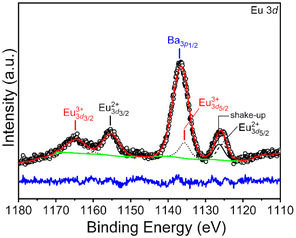 |
| Fig. 4 High-resolution Eu 3d XPS spectrum of BaFCl:Eu (4 mol%) nanocrystals. Experimental data (black circles), calculated spectrum (red line), background (green line), and difference curve (blue line, offset for clarity) are shown. Also shown are deconvoluted peaks corresponding to Eu2+ (short-dashed black line), Eu3+ (dotted black line), Ba 3p core (dashed black line), and shake-up features (solid black line). | |
Room-temperature excitation spectra of BaFCl:Eu,Tb, BaFCl:Tb, and BaFCl:Eu nanocrystals were collected to gain insight into energy-transfer pathways between activators. Results from these studies are presented in Fig. 5. The excitation spectrum of the Eu2+ 387 nm emission in BaFCl:Eu,Tb was dominated by a broad band with maxima at 260 and 272 nm (Fig. 5a), which we assign to excitation from the 4f7 (8S7/2) ground state of Eu2+ to the upper levels of the 4f65d1 excited state.61–63 A very similar excitation profile was observed for the 544 nm emission of Tb3+, although in this case maxima were located at 265 and 275 nm. Additionally, a weak peak arising from the 4f–4f excitation of Tb3+ was observable at 377 nm (7F6 → 5L10). The similarity between the excitation spectra of Eu2+ and Tb3+ and the observation of emission from the latter upon excitation of the former pointed to the occurrence of Eu2+ → Tb3+ energy transfer. Comparison of the excitation spectra of BaFCl:Eu,Tb and BaFCl:Tb nanocrystals gave further support to this conclusion. The spectrum of BaFCl:Tb featured a broad band around 250 nm, which we assign to the 4f8 → 4f75d1 transition of Tb3+, as well as a number of weak peaks arising from 4f–4f transitions (Fig. 5b). Codoping BaFCl:Tb with europium led to the appearance of the band peaking at 275 nm, i.e., of a new excitation pathway in which the 4f65d1 excited state of Eu2+ fed Tb3+ emission. In the case of the 612 nm emission of Eu3+ in BaFCl:Eu,Tb, the excitation spectrum displayed a broad band around 250 nm (Fig. 5a). Moreover, a peak corresponding to the 4f–4f excitation of Eu3+ appeared at 393 nm (7F0 → 5L6). The excitation profile is similar to that observed in singly-doped BaFCl:Eu nanocrystals (Fig. 5c). Based on previous studies of Eu3+-doped BaFCl,20,47,64 we assign the band around 250 nm to a charge-transfer state involving the top of the valence band (vb) and the ground state of Eu3+ (i.e., evb + Eu3+ (7F6) → hvb+ + Eu2+ (8S7/2)). The position of the 8S7/2 ground state of the transient Eu2+ generated during this electron transfer ought to be distinguished from that of the Eu2+ centers generated in the synthesis stage because they sit in different crystallochemical environments, as mentioned in the Introduction. Excitation of BaFCl:Eu nanocrystals using 240 nm light (≈5.2 eV) led to excitation of both Eu3+ (via charge transfer, ≈5.0 eV) and Eu2+ (via 4f7 (8S7/2) → 4f65d1, ≈4.5 eV) and subsequent emissions at 612 and 387 nm (Fig. 5d). Decreasing the excitation energy favored excitation of Eu2+ in detriment of Eu3+ as shown by a decrease in the intensity of Eu3+ emission relative to that of Eu2+. Similar to what was observed for BaFCl:Eu, excitation of BaFCl:Eu,Tb nanocrystals using 275 nm light led to very weak Eu3+ emission (Fig. 5a). In summary, excitation of BaFCl:Eu,Tb at 275 nm populated the upper levels of the 4f65d1 excited state of Eu2+. Subsequently, this led to Eu2+ emissions at 363 and 387 nm via radiative relaxation of the 4f7 (6P7/2) and 4f65d1 (ll) levels, respectively. The 4f65d1 excited state of Eu2+ also served to feed Tb3+ 4f8 (5D4) → 4f8 (7FJ) emissions via Eu2+ → Tb3+ energy transfer. Excitation at 275 nm also induced Eu3+ 4f6 (5D0) → 4f6 (7FJ) emissions via a host → Eu3+ charge-transfer state. For completeness we note that excitation of the terbium-centered 7F6 → 5D4 transition at 486 nm led to Eu3+ emission, indicating the occurrence of Tb3+ → Eu3+ energy transfer (see ESI, Fig. S4†). Tb3+ emission bands were not observed upon excitation of the 7F0 → 5D2 transition of Eu3+ at 463 nm, suggesting that Eu3+ → Tb3+ energy transfer did not take place.
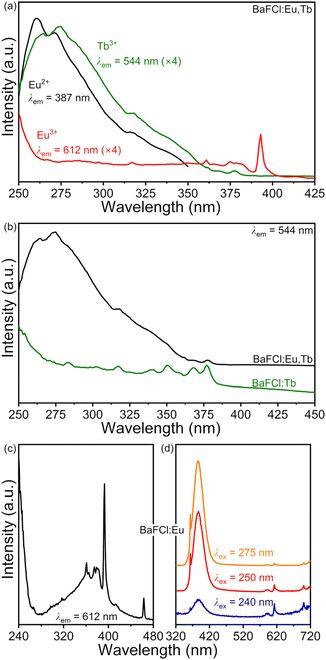 |
| Fig. 5 (a) Excitation spectra of BaFCl:Eu,Tb nanocrystals. (b) Excitation spectrum of BaFCl:Tb (6 mol%) nanocrystals. The spectrum of BaFCl:Eu,Tb is also shown to facilitate comparison. (c) and (d) show the excitation and emission spectra of BaFCl:Eu (6 mol%) nanocrystals. Spectra were collected at room temperature. | |
Emission spectra excited at 275 nm were collected between 80 and 430 K to assess the potential of BaFCl:Eu,Tb nanocrystals as luminescence thermometers. Results from these studies are compiled in Fig. 6. Spectra featured the expected emissions from Eu2+, Eu3+, and Tb3+ (Fig. 6a). At first, the temperature dependence of four major bands was of interest to us; these were 4f7 (6P7/2) → 4f7 (8S7/2) of Eu2+ at 27
550 cm−1, 4f65d1 (ll) → 4f7 (8S7/2) of Eu2+ at ≈25
800 cm−1, 5D4 → 7F5 of Tb3+ at 18
334 cm−1, and the red emission band including the 5D0 → 7F2 of Eu3+ at 16
334 cm−1 and the 5D4 → 7F3 of Tb3+ at 16
139 cm−1. However, visual inspection of the spectra led us to focus on the emissions from Eu2+. Indeed, the most noticeable change was the decrease in the intensity of the f–f line emission at 27
550 cm−1 and the simultaneous increase of the fd–f broad band emission at ≈25
800 cm−1 upon increasing temperature. This change was simply the result of the thermal coupling between the 4f7 (6P7/2) and 4f65d1 (ll) levels of Eu2+, with the former sitting a few hundred wavenumbers below the latter (vide infra). Thermal coupling between these two levels is well-documented for Eu2+ doped into a number of hosts, including BaFCl.24,25,27,53,56,63 The temperature dependence of the integrated intensities of the four bands of interest further confirmed the potential of the 4f65d1 (ll)/4f7 (6P7/2) manifold for temperature sensing. The emission intensity from the lower thermally coupled level dropped by ≈2 orders of magnitude upon going from 80 to 430 K whereas that of the upper level showed a threefold increase up to 230 K and plateaued afterwards (Fig. 6b). Relative intensity changes between the green (Tb3+) and red band (Eu3+ and Tb3+) were significantly less pronounced. Nonetheless, using the 4f65d1 (ll)/4f7 (6P7/2) manifold for ratiometric thermometry is not straightforward due to the strong overlap between their emission bands, which complicates extraction of accurate integrated intensities and of the corresponding luminescence intensity ratios.27,65 Eu2+ intensities plotted in Fig. 6b were extracted by fitting spectra between 20
000 and 30
000 cm−1 with a varying number of gaussians (13 at 80 K, 7 at 430 K). We thought of two alternatives to the luminescence intensity ratio. First, we considered computing the geometric centroid of the Eu2+ emission. However, this involves integration limits that change with temperature, thus compromising accuracy and repeatability. Next, we attempted using a peak-intensity-weighted centroid
as the thermometric parameter. We defined this centroid using eqn (2). Here,
and
are the peak intensities of the emissions from the 4f7 (6P7/2) and 4f65d1 (ll) levels measured at wavenumbers
and
|  | (2) |

, respectively. The use of

as the thermometric parameter does not require setting arbitrary integration limits nor performing peak fit. Further, it can be computed on the fly once wavenumbers

and

are set. In this work, we set

(363 nm) and
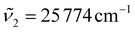
(388 nm) and computed

using the corresponding peak intensities at each temperature (see ESI, Table S2
†).

decreased from 27
![[thin space (1/6-em)]](https://www.rsc.org/images/entities/char_2009.gif)
316 to 26
![[thin space (1/6-em)]](https://www.rsc.org/images/entities/char_2009.gif)
338 cm
−1 upon going from 80 to 430 K (
Fig. 6c). The sigmoidal dependence of

was adequately modeled using a Boltzmann function given by
eqn (3). This phenomenological model reflected the transition from line emission at low temperature
|  | (3) |
(4f
7 (
6P
7/2) → 4f
7 (
8S
7/2)) to broad band emission at high temperatures (4f
65d
1 (
ll) → 4f
7 (
8S
7/2)). The corresponding fit parameters were
A1 = 27
![[thin space (1/6-em)]](https://www.rsc.org/images/entities/char_2009.gif)
437(13) cm
−1,
A2 = 26
![[thin space (1/6-em)]](https://www.rsc.org/images/entities/char_2009.gif)
331(11) cm
−1,
T0 = 166(2) K, and
σ = 41(1) K. The temperature sensitivity of the centroid was maximum at 165 K, with a shift of 6.7 cm
−1 K
−1 (
Fig. 6c). Altogether, results from these studies demonstrated that the temperature-dependent change in the emission profile of Eu
2+ may be exploited to realize bandshape luminescence thermometry as a straightforward alternative to the traditional ratiometric approach.
 |
| Fig. 6 (a) Variable-temperature emission spectra of BaFCl:Eu,Tb nanocrystals. Four emissions of interest are depicted with dotted lines. The temperature dependence of their integrated intensities is given in (b). Intensities are normalized with respect to the broad band of Eu2+ at 230 K. The integrated intensity of Eu2+ line emission corresponds to that of the zero-phonon line at 27 550 cm−1; the one-phonon line at 27 315 cm−1 (depicted with an asterisk) was not included in the integration. (c) Experimental (black squares, eqn (2)) and calculated (red line, eqn (3)) temperature dependence of the peak-intensity-weighted centroid of Eu2+ emission. The derivative of the calculated dependence is also plotted (blue line). | |
Finally, we aimed to gain quantitative insight into the thermosensitive 4f65d1 (ll)/4f7 (6P7/2) manifold of Eu2+ doped into BaFCl:Eu,Tb nanocrystals. Specifically, we sought to estimate the energy gap between these two thermally coupled levels (ΔE), their radiative transition probabilities (kfd→f, kf→f), and compare these values to those reported for micron-sized Eu2+-doped BaFCl.24,27,53 Previous studies used eqn (4) to
| 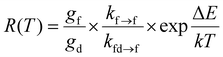 | (4) |
fit the temperature dependence of the luminescence intensity ratio to estimate the energy gap. Here,
gf and
gd are the degeneracies of the emissive levels (
gf/
gd = 4).
27 These fits, however, ran into problems due to the challenge of extracting accurate integrated intensities from emission spectra. Sytsma and Blasse explicitly acknowledged this issue.
27 Liu and coworkers estimated the energy gap using just three experimental
R(
T) values between 10 and 216 K. Some
R(
T) values computed using
eqn (4) deviated more than 20% from their experimental counterparts.
53 Sommerdijk and coworkers used just two experimental data points,
R(77 K) and
R(300 K).
24 Further, authors did not provide enough details to assess the quality of the fits (
e.g., fit plot and residual and/or a complete set of fit parameters). On this basis, we decided to estimate photophysical parameters of the 4f
65d
1 (
ll)/4f
7 (
6P
7/2) manifold using the temperature dependence of its radiative constant (
kEu2+) as given by
eqn (5). Here 〈
τ〉 is the
|  | (5) |
intensity-weighted average lifetime of Eu
2+ emission computed using
eqn (1) (
vide supra). Luminescence decays were excited at 265 nm and emission was monitored at 387 nm between 80 and 325 K, a temperature range comparable to that employed in previous studies of Eu
2+-doped BaFCl.
24,27,53 The resulting temperature-dependence of
kEu2+ is plotted in
Fig. 7; experimental decays are given in the ESI (see Fig. S5
†).
Eqn (5) provided a good fit to experimental
kEu2+ values. The corresponding fit parameters were Δ
E = 205(27) cm
−1,
kf→f = 5212(2643) s
−1, and
kfd→f = 5.1 × 10
5 (4.4 × 10
4) s
−1. Though slightly smaller, the 4f
65d
1 (
ll)/4f
7 (
6P
7/2) energy gap estimated for our nanocrystals is in the same range of that previously reported for BaFCl:Eu
2+ single crystals and bulk solids (393, 400, and 592 cm
−1).
24,27,53 A similar remark holds for radiative transition probability
kf→f (5212 s
−1vs. 4000 s
−1).
24,27,53 In the case of
kfd→f, our value was smaller than those reported in the literature (5.1 × 10
5 s
−1vs. 1.5 × 10
6, 3.5 × 10
6, and 1.6 × 10
7 s
−1).
24,27,53kfd→f and
kf→f differed by two orders of magnitude, as expected for the probabilities of parity-allowed
vs. parity-(and spin)-forbidden transitions, respectively.
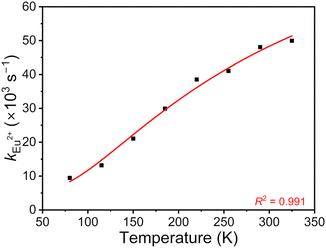 |
| Fig. 7 Experimental (black squares) and calculated (red line, eqn (5)) temperature-dependence of the radiative constant of Eu2+ in BaFCl:Eu,Tb nanocrystals. | |
Conclusions
The luminescence response of BaFCl:Eu2+/3+,Tb3+ nanocrystals was characterized between 80 and 430 K. Although trivalent europium was used as a reagent and no reducing agent was deliberately incorporated in the reaction mixture, partial reduction to divalent europium occurred during the synthesis stage. The resulting Eu3+/Eu2+ ratio was estimated to be ca. 0.8, corresponding to a reduction of over half of the starting Eu3+ ions. The room-temperature emission spectrum of BaFCl:Eu2+/3+,Tb3+ was dominated by near UV emissions from the 4f7 (6P7/2) and 4f65d1 (ll) thermally coupled levels of Eu2+. Emission from this manifold transitioned from line-like to band-like upon increasing temperature, a feature that could be exploited for bandshape luminescence thermometry. We introduced the peak-intensity-weighted centroid as an alternative thermometric parameter that circumvents a number of issues arising in the context of ratiometric thermometry. Photophysical parameters of the 4f65d1 (ll)/4f7 (6P7/2) manifold were estimated from time-resolved variable-temperature decays and should help to establish composition–luminescence relationships in nanocrystalline alkaline-earth fluorohalides as the nanocrystal library expands.
Data availability
All the data used are provided in the article and the ESI.†
Conflicts of interest
There are no conflicts to declare.
Acknowledgements
The authors would like to acknowledge the financial support of the National Science Foundation (DMR-2003118) and of Wayne State University (Department of Chemistry and Office of the Vice President for Research). They also thank the Lumigen Instrument Center at Wayne State University for the use of the X-ray diffraction (National Science Foundation MRI-1427926), electron microscopy (National Science Foundation MRI-2018587), and photoelectron spectroscopy facilities (National Science Foundation MRI-1849578).
References
- Y. R. Shen, T. Gregorian and W. B. Holzapfel, Progress in Pressure Measurements with Luminescence Sensors, High Pressure Res., 1991, 7, 73–75 CrossRef.
- P. Comodi and P. F. Zanazzi, Improved Calibration Curve for the Sm2+-BaFCl Pressure Sensor, J. Appl. Crystallogr., 1993, 26, 843–845 CrossRef CAS.
- Y. R. Shen, U. Englisch, L. Chudinovskikh, F. Porsch, R. Haberkorn, H. P. Beck and W. B. Holzapfel, A Structural Study on the PbFCl-Type Compounds MFCl (M = Ba, Sr, and Ca) and BaFBr Under High-Pressure, J. Phys.: Condens. Matter, 1994, 6, 3197–3206 CrossRef CAS.
- B. Lorenz, Y. R. Shen and W. B. Holzapfel, Characterization of the New Luminescence Pressure Sensor SrFCl:Sm2+, High Pressure Res., 1994, 12, 91–99 CrossRef.
- S. S. Perera, K. T. Dissanayake and F. A. Rabuffetti, Alkaline-Earth Fluorohalide Nanocrystals for Upconversion Thermometry, J. Lumin., 2019, 207, 416–423 CrossRef CAS.
- B. D. Dhanapala, H. N. Munasinghe, K. T. Dissanayake, L. Suescun and F. A. Rabuffetti, Expanding the Synthetic Toolbox to Access Pristine and Rare-Earth-Doped BaFBr Nanocrystals, Dalton Trans., 2021, 50, 16092–16098 RSC.
- B. D. Dhanapala, H. N. Munasinghe and F. A. Rabuffetti, Temperature-Dependent Luminescence of CaFCl:Yb,Er Upconverting Nanocrystals, J. Lumin., 2021, 235, 117974 CrossRef CAS.
- M. Sonoda, M. Takano, J. Miyahara and H. Kato, Computed Radiography Utilizing Scanning Laser Stimulated Luminescence, Radiology, 1983, 148, 833–838 CrossRef CAS.
- K. Takahashi, J. Miyahara and Y. Shibahara, Photostimulated Luminescence (PSL) and Color-Centers in BaFCl-Eu2+, BaFBr-Eu2+, BaFI-Eu2+ Phosphors, J. Electrochem. Soc., 1985, 132, 1492–1494 CrossRef CAS.
- K. Takahashi, K. Kohda, J. Miyahara, Y. Kanemitsu, K. Amitani and S. Shionoya, Mechanism of Photostimulated Luminescence in BaFX:Eu2+ (X = Cl, Br) Phosphors, J. Lumin., 1984, 31–32, 266–268 CrossRef CAS.
- H. Riesen and W. A. Kaczmarek, Efficient X-ray Generation of Sm2+ in Nanocrystalline BaFCl/Sm3+: A Photoluminescent X-ray Storage Phosphor, Inorg. Chem., 2007, 46, 7235–7237 CrossRef CAS.
- N. Riesen, A. François, K. Badek, T. M. Monro and H. Riesen, Photoreduction of Sm3+ in Nanocrystalline BaFCl, J. Phys. Chem. A, 2015, 119, 6252–6256 CrossRef CAS PubMed.
- H. Riesen, K. Badek, T. M. Monro and N. Riesen, Highly Efficient Valence State Switching of Samarium in BaFCl:Sm Nanocrystals in the Deep UV for Multilevel Optical Data Storage, Opt. Mater. Express, 2016, 6, 3097–3108 CrossRef CAS.
- J. Zhang and H. Riesen, Controlled Generation of Tm2+ Ions in Nanocrystalline BaFCl:Tm3+ by X-ray Irradiation, J. Phys. Chem. A, 2017, 121, 803–809 CrossRef CAS PubMed.
- N. Riesen, C. Priest, D. G. Lancaster, K. Badek and H. Riesen, Ultra-High-Resolution Greyscale Fluorescence Images via UV-Exposure of Thin Flexible Phosphor Films, Nanoscale, 2023, 15, 4863–4869 RSC.
- D. Nicollin and H. Bill, Gd3+, Eu2+ in SrFCl and BaFCl Single Crystals: EPR Results, Solid State Commun., 1976, 20, 135–137 CrossRef CAS.
- D. Zevenhuijzen, J. A. Vanwinsum and H. W. D. Hartog, S-State, Ions in Tetragonal SrFCl, J. Phys. C: Solid State Phys., 1976, 9, 3113–3119 CrossRef CAS.
- D. Nicollin and H. Bill, Experimental Contribution to the Study of the S-State Ions in Ionic Single-Crystals, J. Phys. C: Solid State Phys., 1978, 11, 4803–4814 CrossRef CAS.
- M. Falin, H. Bill and D. Lovy, EPR of Sm3+ in BaFCl Single Crystals, J. Phys.: Condens. Matter, 2004, 16, 1293–1298 CrossRef CAS.
- Q. Ju, Y. S. Liu, R. F. Li, L. Q. Liu, W. Q. Luo and X. Y. Chen, Optical Spectroscopy of Eu3+-Doped BaFCl Nanocrystals, J. Phys. Chem. C, 2009, 113, 2309–2315 CrossRef CAS.
- Z. Liu, M. A. Stevens-Kalceff and H. Riesen, Effects of Postannealing on the Photoluminescence Properties of Coprecipitated Nanocrystalline BaFCl:Sm3+, J. Phys. Chem. A, 2013, 117, 1930–1934 CrossRef CAS.
- D. K. Amarasinghe, K. T. Dissanayake, B. D. Dhanapala and F. A. Rabuffetti, Local Atomic Environment of Yb3+ in Alkaline-Earth Fluorohalide Nanocrystals, CrystEngComm, 2022, 24, 5317–5323 RSC.
- K. T. Dissanayake and F. A. Rabuffetti, Multicolor Emission in Chemically and Structurally Tunable Er:Yb:SrFX (X = Cl, Br) Upconverting Nanocrystals, Chem. Mater., 2018, 30, 2453–2462 CrossRef CAS.
- J. L. Sommerdijk, J. M. P. J. Verstegen and A. Bril, Luminescence of MeFX:Eu2+ (Me = Sr, Ba; X = Cl, Br), J. Lumin., 1974, 8, 502–506 CrossRef CAS.
- T. Kobayasi, S. Mroczkowski, J. F. Owen and L. H. Brixner, Fluorescence Lifetime and Quantum Efficiency for 5d → 4f Transitions in Eu2+ Doped Chloride and Fluoride Crystals, J. Lumin., 1980, 21, 247–257 CrossRef CAS.
- R. Jaaniso and H. Bill, f–f and f–d Transition Interference in Sm2+:SrFCl, Phys. Rev. B: Condens. Matter Mater. Phys., 1991, 44, 2389–2392 CrossRef CAS.
- J. Sytsma and G. Blasse, Comparison of the Emission of Eu2+ in MFCl (M = Sr, Ba) and Gd3+ in YOCl, J. Lumin., 1992, 51, 283–292 CrossRef CAS.
- Y. R. Shen and W. B. Holzapfel, Effect of Pressure on Energy Levels of Sm2+ in BaFCl and SrFCl, Phys. Rev. B: Condens. Matter Mater. Phys., 1995, 51, 15752–15762 CrossRef CAS.
- Y. Shen and K. L. Bray, Effect of Pressure and Temperature on 4f–4f, Luminescence Properties of Sm2+ ions in MFCl crystals (M = Ba, Sr, and Ca), Phys. Rev. B: Condens. Matter Mater. Phys., 1998, 58, 11944–11958 CrossRef CAS.
- P. Pal, T. Penhouet, V. D'Anna and H. Hagemann, Effect of Temperature and Pressure on Emission Lifetime of Sm2+ Ion Doped in MFX (M = Sr, Ba; X = Br, I) Crystals, J. Lumin., 2013, 142, 66–74 CrossRef CAS.
- P. Pal, H. Hagemann, H. Bill and J. Zhang, Temperature and Host Dependence of the Transition Interference between f–f and f–d Transitions of Sm2+ in Matlockites, J. Lumin., 2015, 161, 323–329 CrossRef CAS.
- J. Afshani, T. Delgado, G. Paveliuc, P. Pal and H. Hagemann, Optical Properties of Sm2+ Doped in BaFI Crystals, J. Lumin., 2023, 257, 119648 CrossRef CAS.
- C. van Aarle, K. W. Krämer and P. Dorenbos, Lengthening of the Sm2+ 4f55d → 4f6 Decay Time Through Interplay With the 4f,6[5D0] Level and its Analogy to Eu2+ and Pr3+, J. Lumin., 2024, 266, 120329 CrossRef CAS.
- E. Nicklaus, Optical Properties of Some Alkaline Earth Halides, Phys. Status Solidi A, 1979, 53, 217–224 CrossRef CAS.
- V. V. Mikhailin and M. A. Terekhin, Luminescence Excitation of Barium Fluorohalides by Using Synchrotron Radiation, Nucl. Instrum. Methods Phys. Res., Sect. A, 1989, 282, 607–609 CrossRef.
- G. Kalpana, B. Palanivel, I. B. Shameem Banu and M. Rajagopalan, Structural and Electronic Properties of Alkaline-earth Fluorohalides Under Pressure, Phys. Rev. B: Condens. Matter Mater. Phys., 1997, 56, 3532–3535 CrossRef CAS.
- P. Dorenbos, Systematic Behaviour in Trivalent Lanthanide Charge Transfer Energies, J. Phys.: Condens. Matter, 2003, 15, 8417–8434 CrossRef CAS.
- P. Dorenbos, Thermal Quenching of Lanthanide Luminescence via Charge Transfer States in Inorganic Materials, J. Mater. Chem. C, 2023, 11, 8129–8145 RSC.
- J. J. Schuyt and G. V. M. Williams, Quenching of the Sm2+ Luminescence in NaMgF3:Sm via Photothermal Ionization: Alternative Method to Determine Divalent Lanthanide Trap Depths, Appl. Phys. Lett., 2019, 115, 181104 CrossRef.
- X. Zhao, Q. Yu, J. Yuan, N. V. Thakor and M. C. Tan, Biodegradable Rare Earth Fluorochloride Nanocrystals for Phototheranostics, RSC Adv., 2020, 10, 15387–15393 RSC.
- M. A. Stevens-Kalceff, Z. Liu and H. Riesen, Cathodoluminescence Microanalysis of Irradiated Microcrystalline and Nanocrystalline Samarium Doped BaFCl, Microsc. Microanal., 2012, 18, 1229–1238 CrossRef CAS PubMed.
- N. Chowdhury, N. Riesen and H. Riesen, Efficient Generation of Stable Sm2+ in Nanocrystalline BaLiF3:Sm3+ by UV- and X-Irradiation, J. Phys. Chem. C, 2019, 123, 25477–25481 CrossRef CAS.
- E. Aboelezz and B. W. Pogue, Review of Nanomaterial Advances for Ionizing Radiation Dosimetry, Appl. Phys. Rev., 2023, 10, 021312 CAS.
- M. T. Berry, P. S. May and Q. Hu, Calculated and Observed Tb3+ (5D4) → Eu3+ Electronic Energy Transfer Rates in Na3[Tb0.01Eu0.99(oxydiacetate)3]·2NaClO4·6H2O, J. Lumin., 1997, 71, 269–283 CrossRef CAS.
- M. Bettinelli, A. Speghini, F. Piccinelli, J. Ueda and S. Tanabe, Energy Transfer Processes in Sr3Tb0.90Eu0.10(PO4)3, Opt. Mater., 2010, 33, 119–122 CrossRef CAS.
- G. Bao, K.-L. Wong, D. Jin and P. A. Tanner, A Stoichiometric Terbium−Europium Dyad Molecular Thermometer: Energy Transfer Properties, Light: Sci. Appl., 2018, 7, 96 CrossRef PubMed.
- M.-Z. Su and X.-P. Sun, Luminescence and Possible Electron Transfer and Transitions in BaFCl:Eu Crystal, Mater. Res. Bull., 1987, 22, 879–886 CrossRef CAS.
- K. T. Dissanayake, D. K. Amarasinghe, L. Suescun and F. A. Rabuffetti, Accessing Mixed-Halide Upconverters Using Heterohaloacetate Precursors, Chem. Mater., 2019, 31, 6262–6267 CrossRef CAS.
- H. M. Rietveld, Line Profiles of Neutron Powder-Diffraction Peaks for Structure Refinement, Acta Crystallogr., 1967, 22, 151–152 CrossRef CAS.
- H. M. Rietveld, A Profile Refinement Method for Nuclear and Magnetic Structures, J. Appl. Crystallogr., 1969, 2, 65–71 CrossRef CAS.
- B. H. Toby and R. B. Von Dreele, GSAS-II: The Genesis of a Modern Open-Source All-Purpose Crystallography Software Package, J. Appl. Crystallogr., 2013, 46, 544–549 CrossRef CAS.
- K. Momma and F. Izumi, VESTA 3 for Three-dimensional Visualization of Crystal, Volumetric and Morphology Data, J. Appl. Crystallogr., 2011, 44, 1272–1276 CrossRef CAS.
- G. K. Liu, X. Y. Chen and J. Huang, Intensity and Bandwidth of Multiphonon Vibronic Transitions of Rare-earth Ions in Crystals, Mol. Phys., 2003, 101, 1029–1036 CrossRef CAS.
- X. Zhang, Y. Wang, J. Liu, G. Zhang, G. Xiong and X. Xu, Temperature Dependence of the Photostimulated Luminescence of BaFCl:Eu2+ After Ultraviolet Irradiation, J. Appl. Phys., 1995, 78, 1984–1986 CrossRef CAS.
- W. Chen, S. Wang, S. L. Westcott, J. Zhang, K. Dou, A. G. Joly and D. E. McCready, Structure and Luminescence of BaFBr:Eu2+ and BaFBr:Eu2+,Tb3+ Phosphors and Thin Films, J. Appl. Phys., 2005, 97, 083506 CrossRef.
- M. Secu, C. Secu, V. Vasile, D. Predoi and D. Gazdaru, Time-Resolved Luminescence Spectroscopy of Eu2+ in BaFCl:Eu2+ X-ray Storage Phosphor, J. Optoelectron. Adv. Mater., 2007, 9, 1800–1802 CAS.
-
G. Gundiah, E. Bourret-Courchesne, G. Bizarri, S. M. Hanrahan, A. Chaudhry, A. Canning and W. W. Moses, S. E. Derenzo, in Scintillation Properties of Eu2+-Activated Barium Fluoroiodide, IEEE Nuclear Science Symposium Conference, 2009, pp. 1575–1578.
- W.-D. Schneider, C. Laubschat, I. Nowik and G. Kaindl, Shake-up Excitations and Core–Hole Screening in Eu Systems, Phys. Rev. B: Condens. Matter Mater. Phys., 1981, 24, 5422–5425 CrossRef CAS.
- R. Vercaemst, D. Poelman, R. L. Van Meirhaeghe, L. Fiermans, W. H. Laflère and F. Cardon, An XPS Study of the Dopants’ Valence States and the Composition of CaS1−xSex:Eu and SrS1−xSex:Ce Thin Film Electroluminescent Devices, J. Lumin., 1995, 63, 19–30 CrossRef CAS.
- F. Mercier, C. Alliot, L. Bion, N. Thromat and P. Toulhoat, XPS Study of Eu(III) Coordination Compounds: Core Levels Binding Energies in Solid Mixed Oxocompounds EumXxOy, J. Electron Spectrosc. Relat. Phenom., 2006, 150, 21–26 CrossRef CAS.
- T. J. A. Popma, W. F. van der Weg and K. Thimm, Excitation of Luminescent Materials by Synchrotron Radiation, J. Lumin., 1981, 24–25, 289–292 CrossRef CAS.
- A. M. Gurvich and V. V. Mikhailin, Crystal Phosphors for Storage Luminescent Screens, Russ. Chem. Rev., 1992, 61, 576 CrossRef.
- W. Chen, N. Kristianpoller, A. Shmilevich, D. Weiss, R. Chen and M. Su, X-ray Storage Luminescence of BaFCl:Eu2+ Single Crystals, J. Phys. Chem. B, 2005, 109, 11505–11511 CrossRef CAS PubMed.
- M.-Z. Su and X.-P. Sun, The Charge Transfer Excitation of Trivalent Rare Earth Ions Sm3+, Eu3+, Gd3+, Ho3+, Er3+ and Yb3+ Emission in BaFCl Crystals, Mater. Res. Bull., 1987, 22, 89–94 CrossRef CAS.
- M. Sójka, M. Runowski, T. Zheng, A. Shyichuk, D. Kulesza, E. Zych and S. Lis, Eu2+ Emission from Thermally Coupled Levels–New Frontiers for Ultrasensitive Luminescence Thermometry, J. Mater. Chem. C, 2022, 10, 1220–1227 RSC.
Footnote |
† Electronic supplementary information (ESI) available: (1) thermal analysis of BaFCl:Eu,Tb nanocrystals, (2) refined structural parameters, (3) additional spectra and time-resolved decays, and (4) peak intensities used to compute . See DOI: https://doi.org/10.1039/d4dt02237h |
|
This journal is © The Royal Society of Chemistry 2024 |
Click here to see how this site uses Cookies. View our privacy policy here.