DOI:
10.1039/D4DT01218F
(Paper)
Dalton Trans., 2024,
53, 11088-11093
Multidentate polyoxometalate modification of metal nanoparticles with tunable electronic states†
Received
25th April 2024
, Accepted 12th June 2024
First published on 13th June 2024
Abstract
To respond to the increasing demands for practical applications, stabilization and property modulation of metal nanoparticles have emerged as a key research subject. Herein, we present a viable protocol for preparing small metal nanoparticles (<5 nm; Ag, Pd, Pt, and Ru) via multidentate polyoxometalate (POM, [SiW9O34]10−) modification. In addition to enhancing stability, the POMs can modulate the electronic states of metal nanoparticles. Moreover, immobilization of the POM-modified metal nanoparticles on solid supports enables further tuning of the electronic states via a cooperative effect between the POMs and the supports without altering the particle size. Notably, POM-modified Pd nanoparticles on carbon support exhibited superior catalytic activity and selectivity in hydrogenation reactions in comparison with the catalyst without the POM modification.
Introduction
Metal nanoparticles have emerged as attractive materials in various research fields ranging from fundamental science to industrial applications.1 Their large surface area and abundant surface sites endow small metal nanoparticles with superior reactivity; however, their stability is still insufficient.2 To address this issue, intense research efforts have been devoted to stabilize small metal nanoparticles by employing organic molecules, surfactants, halides, and polymers.3 Unfortunately, the negative effect of such approaches on the reactivity of metal nanoparticles and the vulnerability of conventional organic ligands have limited the application scope.4 Moreover, toward the realization of practical applications, protecting ligands have recently been proposed to contribute to the modulation of the properties of metal nanoparticles, e.g., structures, morphologies, and electronic states, rather than simply providing stability.2,4,5
Polyoxometalates (POMs), a series of metal oxide clusters,6 have been used to stabilize metal nanoparticles in recent decades.7,8 Apart from offering stronger protecting effects than halides, phosphates, citrate, and surfactants,7a POMs exhibit adaptable structures and properties, enabling synergistic effects with metal nanoparticles in diverse fields, such as energy conversion, catalysis, materials science, and biochemistry.7,8 We recently confirmed that multidentate POMs, a specific series of POMs with high negative charges and multiple coordination sites, can be employed to prepare Au nanoparticles exhibiting exceptional stability under practical catalytic conditions and superior performance in several catalytic aerobic oxidation reactions compared with Au nanoparticles without this modification.9 Experimental characterizations and theoretical calculations have revealed the presence of robust electronic interactions between multidentate POMs and Au nanoparticles, enabling the activation of molecular oxygen by anionic Au species for an enhanced catalytic activity.9a This method essentially approached the trade-off between reactivity and stability for Au nanoparticles. In recent years, numerous metal(−oxo) clusters templated by multidentate POMs have been reported,10 which has prompted us to further explore the modification of a wide range of metal nanoparticles with multidentate POMs. Moreover, immobilization of POM-modified metal nanoparticles on solid supports has proven conducive to stability.9a,11 However, this research field remains largely unexplored, although exploring additional functions of supports is essential to further tailor the properties of metal nanoparticles. Thus, addressing these issues represents an important step toward the precise design of metal nanoparticles with desired functions.
Herein, we report the preparation of various metal nanoparticles of Ag, Pd, Pt, and Ru modified with a multidentate POM ([SiW9O34]10−, SiW9) simply by mixing the corresponding metal precursors, sodium borohydride (NaBH4), and SiW9 in aqueous media (Fig. 1). These metal nanoparticles exhibited small sizes of less than five nanometers. Remarkably, stable small Ag nanoparticles were obtained using this method, in contrast with the agglomeration observed when using other protecting ligands and fully occupied POMs. The as-obtained metal nanoparticles retained their small particle size even after immobilization on solid supports, and SiW9-modified palladium nanoparticles on carbon support (Pd-SiW9/C) exhibited superior catalytic activity and selectivity in hydrogenation reactions in comparison with the catalyst without this modification. Moreover, SiW9 can tune the electronic states of the metal nanoparticles and cooperate with the carbon supports to achieve further property modulation.
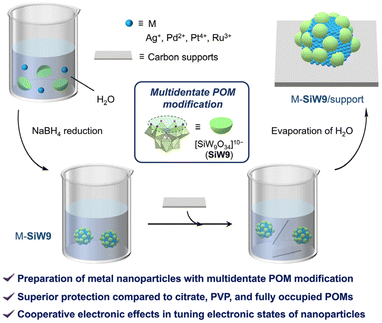 |
| Fig. 1 Schematic of the preparation of metal nanoparticles via modification with the multidentate POM SiW9 and their immobilization on carbon supports. | |
Results and discussion
Preparation of POM-modified colloidal metal nanoparticles
Colloidal metal nanoparticles modified with the multidentate POM SiW9 (M-SiW9) were prepared following our recently reported aqueous-based synthesis method for Au nanoparticles9a with slight modifications (Fig. 1). For example, SiW9-modified Ag nanoparticles (Ag-SiW9) were prepared as follows: AgNO3 was mixed with a sodium salt of SiW9 (Na10SiW9O34, NaSiW9) in water in an ice bath, and the resulting solution was stirred for 30 min. Then, an aqueous solution of NaBH4 (0.1 M) was added dropwise as a reducing agent, resulting in the formation of Ag-SiW9. In a similar manner, Pd-SiW9, Pt-SiW9, and Ru-SiW9 were prepared using Na2PdCl4, K2PtCl6, and K2RuCl5, respectively, as metal sources instead of AgNO3.
The characteristic pale yellow solution and the appearance of a surface plasmon resonance (SPR) band at 405 nm in the ultraviolet–visible (UV–vis) spectrum indicated the formation of small Ag nanoparticles (Fig. 2a). Conversely, for other metal nanoparticles, the absence of a SPR band hindered the estimation of the particle size from the UV–vis spectra of the solutions (Fig. 2b–d). Consequently, transmission electron microscopy (TEM) and dynamic light scattering (DLS) analyses were performed to confirm the formation of metal nanoparticles and determine their particle sizes. The TEM images revealed the presence of metal nanoparticles with average diameter (dav) of several nanometers, i.e., dav = 3.4 nm for Ag-SiW9, dav = 2.4 nm for Pd-SiW9, dav = 2.0 nm for Pt-SiW9, and dav = 3.8 nm for Ru-SiW9 (Fig. 3). Meanwhile, relatively larger hydrodynamic sizes by ca. 2 nm were observed in the DLS analysis, indicating the presence of 1 nm–sized POM ligands surrounding the metal nanoparticles (Fig. S1†).8c,9a
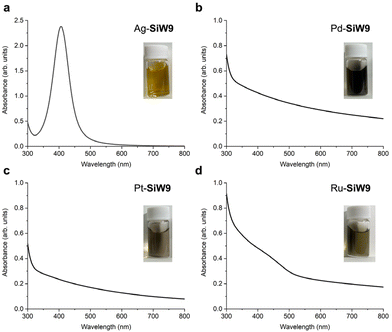 |
| Fig. 2 UV–vis spectra and photos of the solutions of SiW9-modified metal nanoparticles: (a) Ag-SiW9, (b) Pd-SiW9, (c) Pt-SiW9, and (d) Ru-SiW9. | |
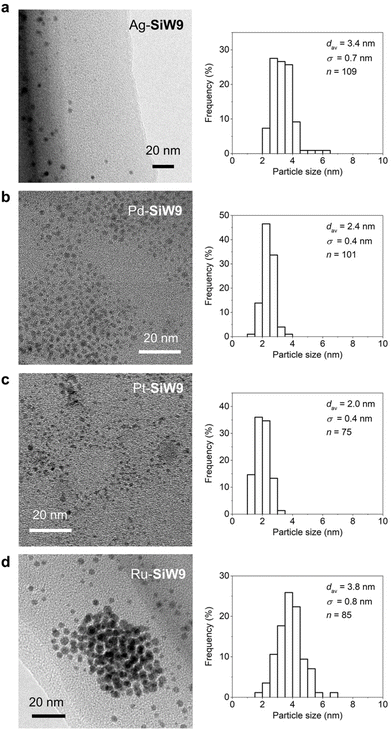 |
| Fig. 3 TEM images and the corresponding size distribution histograms of colloidal SiW9-modified Ag, Pd, Pt, and Ru nanoparticles (a, Ag-SiW9; b, Pd-SiW9; c, Pt-SiW9; d, Ru-SiW9). | |
Next, zeta potential measurements were performed to investigate the surface states of the anionic POM-modified metal nanoparticles (Table 1). Notably, highly negative zeta potentials were observed for M-SiW9 (Ag-SiW9, −50.2 mV; Pd-SiW9, −41.6 mV; Pt-SiW9, −46.3 mV; Ru-SiW9, −47.0 mV) compared with those for metal nanoparticles protected with polyvinylpyrrolidone (PVP) or citrate as ligands (Ag-PVP, −12.1 mV; Pt-PVP, +1.7 mV; Ru-PVP, −10.3 mV; Ru-citrate, −33.9 mV). These results suggested that M-SiW9 possessed anionic POM layers surrounding the metal nanoparticles.8h,i,9b
Table 1 Zeta potentials of metal nanoparticles modified with SiW9 and organic protecting ligands in aqueous solution
Entry |
Metal nanoparticles |
Zeta potential (mV) |
Commercial samples of PVP-protected metal nanoparticles.
|
1 |
Ag-SiW9 |
−50.2 |
2 |
Pd-SiW9 |
−41.6 |
3 |
Pt-SiW9 |
−46.3 |
4 |
Ru-SiW9 |
−47.0 |
5 |
Ag-PVPa |
−12.1 |
6 |
Pt-PVPa |
+1.7 |
7 |
Ru-citrate |
−33.9 |
8 |
Ru-PVP |
−10.3 |
The structure of the multidentate POM was examined via Raman and infrared (IR) spectroscopy using solid samples of M-SiW9 prepared by freeze-drying their synthesis solutions. The IR spectra of M-SiW9 and NaSiW9 showed similar absorption bands in the range of 400–1200 cm−1 (Fig. S2†). Moreover, the Raman spectra of M-SiW9 exhibited the characteristic W
Od band at 940 cm−1 similar to that of NaSiW9 (Fig. S3†). These results suggested that the structure of SiW9 was maintained in M-SiW9.
Typically, Ag nanoparticles tend to agglomerate into large-sized ones during their synthesis.12 Despite their good electrical conductivity, antimicrobial properties, and optical reflectivity, this stability issue limits the applications of Ag nanoparticles.5c,12 Previous reports on POM modification have described Ag nanoparticles with relatively large sizes (>10 nm).8b,e,f,g,j In contrast, our method enabled the preparation of small Ag nanoparticles protected by SiW9 (dav = 3.4 nm, Fig. 2a). Therefore, we studied the stability of small-sized Ag-SiW9 compared with that of Ag nanoparticles prepared using conventional protecting ligands, i.e., citrate, PVP, and a fully occupied POM (K4SiW12O40 as a potassium salt of SiW12) with a similar structure to that of SiW9 but without coordination sites.8j,13,14 These Ag nanoparticles were prepared by mixing AgNO3 and the corresponding protecting ligands in water, followed by addition of an aqueous solution of NaBH4. In the case of Ag-SiW12, a redshifted broad SPR band to 411 nm in the UV–vis spectrum indicated the occurrence of nanoparticle agglomeration (Fig. S4†). When using citrate and PVP as protecting ligands, partial and complete precipitation of Ag was observed, respectively (Fig. S4†). Precipitation was also observed for Pd and Pt nanoparticles when using citrate as a protecting ligand (Fig. S5†). These findings confirm that multidentate POM modification can provide comparable and even superior protection effects compared with conventional protecting ligands for preparing metal nanoparticles.
Immobilization of M-SiW9 on carbon supports
Toward practical applications, immobilization of metal nanoparticles on solid supports is typically important, where maintaining a small particle size is a pivotal concern.1,2 Thus, considering the potential of supported Pd and Ag nanoparticle-based materials,1,2,15 we immobilized the SiW9-modified Ag and Pd nanoparticles on carbon supports. The corresponding M-SiW9/C materials were prepared via an impregnation method involving the addition of carbon supports to the synthesis solution of M-SiW9, followed by removing the solvent (water) using a rotary evaporator (Fig. 1). TEM images revealed that the Ag and Pd nanoparticles in Ag-SiW9/C (dav = 3.2 nm) and Pd-SiW9/C (dav = 2.7 nm) maintained their particle size (Fig. 4a and S6†). In contrast, when using fully occupied SiW12, considerable agglomeration of Ag nanoparticles was observed (Ag-SiW12/C, dav = 5.7 nm), confirming the importance of using multidentate POMs to stabilize small nanoparticles during their synthesis and immobilization (Fig. S4 and S7a†).
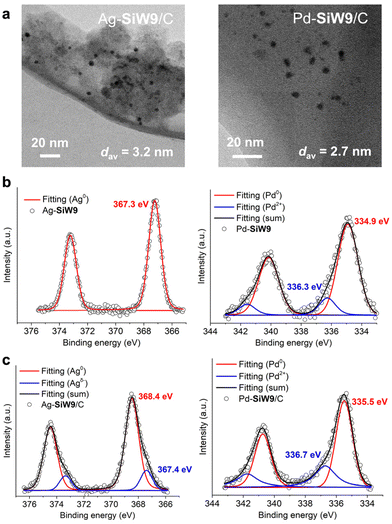 |
| Fig. 4 (a) TEM images of carbon-supported SiW9-modified Ag and Pd nanoparticles (Ag-SiW9/C and Pd-SiW9/C, respectively). (b and c) XPS spectra of SiW9-modified Ag and Pd nanoparticles: (b) before immobilization (Ag-SiW9 and Pd-SiW9) and (c) after immobilization on carbon supports (Ag-SiW9/C and Pd-SiW9/C). | |
Next, we investigated the catalysis of Pd-SiW9/C in hydrogenation reactions based on our recent engagement in the catalysis of POM-modified metal nanoclusters.16 Pd-SiW9/C exhibited superior catalytic activity for the hydrogenation of ethynylbenzene (1a) using H2 (1 atm) in comparison with Pd/C without the SiW9 modification (Table 2). We further validated that, for the hydrogenation of cinnamaldehyde, Pd-SiW9/C afforded 3-phenylpropanal in higher selectivity in comparison with Pd/C (Table S1†). These findings revealed that besides providing stabilizing effect in synthesis and catalysis, multidentate POM modification can contribute to catalytic performance of metal nanoparticle catalysts as well.
Table 2 Hydrogenation of ethynylbenzene (1a) using Pd nanoparticle catalystsa

|
Catalyst |
Time (h) |
Conversion of 1a (%) |
Yield (%) |
2a
|
3a
|
Reaction conditions: 1a (0.5 mmol), catalyst (Pd: 0.5 mol %), diglyme (2 mL), room temperature (∼25 °C), H2 (1 atm). Conversions and yields were determined by GC using biphenyl as an internal standard (n.d. = not detected).
|
Pd-SiW9/C |
1 |
>99 |
32 |
65 |
3 |
>99 |
n.d. |
99 |
18 |
>99 |
n.d. |
99 |
Pd/C |
1 |
68 |
66 |
n.d. |
3 |
89 |
84 |
2 |
18 |
>99 |
n.d. |
99 |
Modulation of the electronic states of metal nanoparticles
In addition to enhancing the nanoparticle stability and catalytic activity, we envisioned that multidentate POM modification could help modulate the properties of metal nanoparticles, such as the electronic states, because the presence of negatively charged active sites on metal nanoparticles can promote the activation of absorbed molecules.17 Accordingly, Weinstock and our group focused on the electronic interactions between POMs and Au nanoparticles to obtain anionic Au nanoparticles as superior catalysts for aerobic oxidation of CO8h and various organic molecules.9 Notably, using various POM ligands, we demonstrated that the electronic states of Au nanoparticles can be modulated within a range of 0.4 eV in terms of the X-ray photoelectron spectroscopy (XPS) binding energies, enabling the activity control in the catalytic oxidative dehydrogenation of piperidone derivatives.9a Therefore, we were interested in confirming the generality of such effects.
To this aim, we studied the electronic interactions between SiW9 and the Ag and Pd nanoparticles via XPS. The binding energy of the main Ag 3d5/2 peak of Ag-SiW9 (367.3 eV) was lower than that of bulk Ag (368 eV) (Fig. 4b). A similar difference was observed between the Pd 3d5/2 peaks of Pd-SiW9 (334.9 eV) and bulk Pd (335.5 eV). These results indicated that multidentate POM modification induced the modulation of the electronic states. The presence of minor Pd 3d5/2 peaks of Pd-SiW9 (336.3 eV) and Pd-SiW9/C (336.74 eV) can be ascribed to the formation of Pd oxide stemming from the oxidation of Pd(0) in air.
The effect of the immobilization on carbon supports on the electronic states of the metal nanoparticles was notable. Thus, immobilization of Ag-SiW9 on carbon supports resulted in a substantial shift of the main Ag 3d5/2 peak to higher binding energies, i.e., from 367.3 eV for Ag-SiW9 to 368.4 eV for Ag-SiW9/C (Fig. 4b and c). Moreover, a minor peak was observed for Ag-SiW9/C (367.4 eV), which could be attributed to Agδ– species, whereas such a peak was not observed for Ag-SiW12/C (Fig. S7b†). Similarly, an obvious shift of the main Pd 3d5/2 peak from 334.9 eV (Pd-SiW9) to 335.5 eV (Pd-SiW9/C) occurred upon immobilization on carbon supports (Fig. 4b and c). A similar shift of the Au 4f7/2 peak from 82.9 eV (Au-SiW9) to 84.0 eV (Au-SiW9/C) was observed for our previously reported Au-SiW9/C material with similar particle sizes (Fig. S8†).9a In contrast, bulk Au and unmodified Au nanoparticles on carbon supports (Au/C) exhibited similar binding energies (84.3 eV).9a In addition to validating the electronic effects of multidentate POM modification, these results revealed the occurrence of a synergistic effect between multidentate POM modification and immobilization on the modulation of the electronic states of metal nanoparticles.
Conclusions
We have demonstrated that multidentate POMs can be used to fabricate small Ag, Pd, Pt, and Ru nanoparticles with diameters of several nanometers in colloidal and supported forms. The superior protection effects of multidentate POMs in the synthesis of metal nanoparticles compared with traditional organic protecting compounds and fully occupied POMs were confirmed. This modification method can be extended to the immobilization of metal nanoparticles while maintaining the particle size, among which POM-modified Pd nanoparticles on carbon supports were proved as efficient catalysts for several hydrogenation reactions in comparison with a commonly utilized Pd/C catalyst. Additionally, multidentate POMs can modulate the electronic states of metal nanoparticles and potentially cooperate with solid supports to achieve a systematic modulation. This work provides not only a versatile approach for producing small metal nanoparticles with high stability but also an efficient tool to tune the electronic states and properties of metal nanoparticles, paving the way for the design of novel metal nanoparticle–based materials with application in the fields of nanoscience, surface chemistry, and material chemistry.
Conflicts of interest
There are no conflicts to declare.
Acknowledgements
We gratefully acknowledge financial supports from JST FOREST (JPMJFR213M), JSPS KAKENHI (22H04971), and JSPS Core-to-Core program. A part of this work was supported by Advanced Research Infrastructure for Materials and Nanotechnology in Japan (ARIM) of the Ministry of Education, Culture, Sports, Science and Technology (MEXT), Grant Number JPMXP1223UT0029 and JPMXP1224UT0038. We thank Ms Umi Shin and Mr Rui Xi for their help with preliminary experiments.
References
-
(a) S. Linic, U. Aslam, C. Boerigter and M. Morabito, Nat. Mater., 2015, 14, 567 CrossRef CAS PubMed
;
(b) Q. Yang, Q. Xu and H. Jiang, Chem. Soc. Rev., 2017, 46, 4774 RSC
;
(c) X. Yang, M. Yang, B. Pang, M. Vara and Y. Xia, Chem. Rev., 2015, 115, 10410 CrossRef CAS PubMed
;
(d) L. Liu and A. Corma, Chem. Rev., 2018, 118, 4981 CrossRef CAS PubMed
.
-
(a) A. Heuer-Jungemann, N. Feliu, I. Bakaimi, M. Hamaly, A. Alkilany, I. Chakraborty, A. Masood, M. F. Casula, A. Kostopoulou and E. Oh, Chem. Rev., 2019, 119, 4819 CrossRef CAS PubMed
;
(b) P. Liu, R. Qin, G. Fu and N. Zheng, J. Am. Chem. Soc., 2017, 139, 2122 CrossRef CAS PubMed
.
-
(a) Z. B. Shifrina, V. G. Matveeva and L. M. Bronstein, Chem. Rev., 2020, 120, 1350 CrossRef CAS PubMed
;
(b) C. Gao, F. Lyu and Y. Yin, Chem. Rev., 2021, 121, 834 CrossRef CAS PubMed
.
-
(a) Z. Niu and Y. Li, Chem. Mater., 2014, 26, 72 CrossRef CAS
;
(b) L. Lu, S. Zou and B. Fang, ACS Catal., 2021, 11, 6020 CrossRef CAS
.
-
(a) Y. Chen, Y. Xianyu and X. Jiang, Acc. Chem. Res., 2017, 50, 310 CrossRef CAS PubMed
;
(b) L. M. Rossi, J. L. Fiorio, M. A. S. Garcia and C. P. Ferraz, Dalton Trans., 2018, 47, 5889 RSC
;
(c) H. Kang, J. T. Buchman, R. S. Rodriguez, H. L. Ring, J. He, K. C. Bantz and C. L. Haynes, Chem. Rev., 2019, 119, 664 CrossRef CAS PubMed
;
(d) B. Zhang, J. Chen, Y. Cao, O. J. H. Chai and J. Xie, Small, 2021, 17, 2004381 CrossRef CAS PubMed
;
(e) M. L. Ermini and V. Voliani, ACS Nano, 2021, 15, 6008 CrossRef CAS PubMed
.
-
(a)
M. T. Pope, Heteropoly and Isopoly Oxometalates, Springer, Berlin, 1983 CrossRef
;
(b) M. Sadakane and E. Steckhan, Chem. Rev., 1998, 98, 219 CrossRef CAS PubMed
;
(c) H. N. Miras, J. Yan, D.-L. Long and L. Cronin, Chem. Soc. Rev., 2012, 41, 403 RSC
;
(d) Y. Ji, L. Huang, J. Hu, C. Streb and Y. Song, Energy Environ. Sci., 2015, 8, 776 RSC
.
-
(a) R. G. Finke and S. Özkar, Coord. Chem. Rev., 2004, 248, 135 CrossRef CAS
;
(b) Y. Wang and I. A. Weinstock, Chem. Soc. Rev., 2012, 41, 7479 RSC
;
(c) S. G. Mitchell and J. M. Fuente, J. Mater. Chem., 2012, 22, 18091 RSC
;
(d) K. Xia, K. Yamaguchi and K. Suzuki, Angew. Chem., Int. Ed., 2023, 62, e202214506 CrossRef CAS PubMed
;
(e) S. Zhang, R. Liu, C. Streb and G. Zhang, Polyoxometalates, 2023, 2, 9140037 CrossRef
.
-
(a) Y. Lin and R. G. Finke, J. Am. Chem. Soc., 1994, 116, 8335 CrossRef CAS
;
(b) A. Troupis, A. Hiskia and E. Papaconstantinou, Angew. Chem., Int. Ed., 2002, 41, 1911 CrossRef CAS
;
(c) A. Neyman, L. Meshi, L. Zeiri and I. A. Weinstock, J. Am. Chem. Soc., 2008, 130, 16480 CrossRef CAS PubMed
;
(d) S. Martín, Y. Takashima, C. Lin, Y. Song, H. N. Miras and L. Cronin, Inorg. Chem., 2019, 58, 4110 CrossRef PubMed
;
(e) G. Zhang, B. Meita, A. Dolbecq, P. Mialane, F. Sécheresse, F. Miserque and L. Nadjo, Chem. Mater., 2007, 19, 5821 CrossRef CAS
;
(f) R. Liu, Z. Xian, S. Zhang, C. Chen, Z. Yang, H. Li, W. Zheng, G. Zhang and H. Cao, RSC Adv., 2015, 5, 74447 RSC
;
(g) H. K. Daima, P. R. Selvakannan, A. E. Kandjani, R. Shukla, S. R. Bhargava and V. Bansal, Nanoscale, 2014, 6, 758 RSC
;
(h) M. Zhang, J. Hao, A. Neyman, Y. Wang and I. A. Weinstock, Inorg. Chem., 2017, 56, 2400 CrossRef CAS PubMed
;
(i) Y. Wang, O. Zeiri, S. Sharet and I. A. Weinstock, Inorg. Chem., 2012, 51, 7436 CrossRef CAS PubMed
;
(j) A. Umapathi, N. P. Nagaraju, H. Madhyastha, D. Jain, S. P. Srinivas, V. M. Rotello and H. K. Daima, Colloids Surf., B, 2019, 184, 110522 CrossRef CAS PubMed
;
(k) Y. Wang, O. Zeiri, M. Raula, B. L. Ouay, F. Stellacci and I. A. Weinstock, Nat. Nanotechnol., 2017, 12, 170 CrossRef CAS PubMed
;
(l) C. Streb, R. Tsunashima, D. A. MacLaren, T. McGlone, T. Akutagawa, T. Nakamura, A. Scandurra, B. Pignataro, N. Gadegaard and L. Cronin, Angew. Chem., Int. Ed., 2009, 48, 6490 CrossRef CAS PubMed
;
(m) S. Repp, A. I. Lopez-Lorente, B. Mizaikoff and C. Streb, ACS Omega, 2020, 5, 25036 CrossRef CAS PubMed
;
(n) C. Martin, K. Kastner, J. M. Cameron, E. Hampson, J. A. Fernandes, E. K. Gibson, D. A. Walsh, V. Sans and G. N. Newton, Angew. Chem., Int. Ed., 2020, 59, 14331 CrossRef CAS PubMed
.
-
(a) K. Xia, T. Yatabe, K. Yonesato, T. Yabe, S. Kikkawa, S. Yamazoe, A. Nakata, K. Yamaguchi and K. Suzuki, Angew. Chem., Int. Ed., 2022, 61, e202205873 CrossRef CAS PubMed
;
(b) K. Xia, T. Yatabe, K. Yonesato, S. Kikkawa, S. Yamazoe, R. Ishikawa, N. Shibata, S. Ikuhara, A. Nakata, K. Yamaguchi and K. Suzuki, Nat. Commun., 2024, 15, 851 CrossRef CAS PubMed
.
-
(a) Y. Sunada, K. Yamaguchi and K. Suzuki, Coord. Chem. Rev., 2022, 469, 214673 CrossRef CAS
;
(b) M. Wang, J. Pang, J. Wang and J. Niu, Coord. Chem. Rev., 2024, 508, 215730 CrossRef CAS
;
(c) L. Liu, L. Wang, X. Xiao, P. Yang, J. Zhao and U. Kortz, Coord. Chem. Rev., 2024, 508, 215687 CrossRef
;
(d) I. Colliard, J. R. I. Lee, C. A. Colla, H. E. Mason, A. M. Sawvel, M. Zavarin, M. Nyman and G. J.-P. Deblonde, Nat. Chem., 2022, 14, 1357 CrossRef CAS PubMed
.
-
(a) G. Maayan and R. Neumann, Chem. Commun., 2005, 4595 RSC
;
(b) G. Maayan and R. Neumann, Catal. Lett., 2008, 123, 41 CrossRef CAS
;
(c) S. Kikkawa, S. Fukuda, J. Hirayama, N. Shira, R. Takahata, K. Suzuki, K. Yamaguchi, T. Teranishi and S. Yamazoe, Chem. Commun., 2022, 58, 9018 RSC
.
-
(a) S. Chernousova and M. Epple, Angew. Chem., Int. Ed., 2012, 52, 1636 CrossRef PubMed
;
(b) L. Rizzello and P. P. Pompa, Chem. Soc. Rev., 2014, 43, 1501 RSC
;
(c) X. Dong, Z. Gao, K. Yang, W. Zhang and L. Xu, Catal. Sci. Technol., 2015, 5, 2554 RSC
.
- H. Tsunoyama, N. Ichikuni, H. Sakurai and T. Tsukuda, J. Am. Chem. Soc., 2009, 131, 7086 CrossRef CAS PubMed
.
- J. Turkevich, P. Stevenson and J. Hiller, Discuss. Faraday Soc., 1951, 11, 55 RSC
.
-
(a) X. Chen, Z. Zheng, X. Ke, E. Jaatinen, T. Xie, D. Wang, C. Guo, J. Zhao and H. Zhu, Green Chem., 2010, 12, 414 RSC
;
(b) B. Yuan, Y. Pan, Y. Li, B. Yin and H. Jiang, Angew. Chem., Int. Ed., 2010, 49, 4054 CrossRef CAS PubMed
.
-
(a) K. Yonesato, D. Yanai, S. Yamazoe, D. Yokogawa, T. Kikuchi, K. Yamaguchi and K. Suzuki, Nat. Chem., 2023, 15, 940–947 CrossRef CAS PubMed
;
(b) Y. Koizumi, K. Yonesato, S. Kikkawa, S. Yamazoe, K. Yamaguchi and K. Suzuki, J. Am. Chem. Soc., 2024, 146, 13658–13665 CrossRef PubMed
.
-
(a) M. Kitano, Y. Inoue, Y. Yamasaki, F. Hayashi, S. Kanbara, S. Matsuishi, T. Yokoyama, S. Kim, M. Hara and H. Hosono, Nat. Chem., 2012, 4, 934 CrossRef CAS PubMed
;
(b) T. Ye, Y. Lu, Z. Xiao, J. Li, T. Nakao, H. Abe, Y. Niwa, M. Kitano, T. Tada and H. Hosono, Nat. Commun., 2019, 10, 5653 CrossRef CAS PubMed
;
(c) J. Guo, Y. Zhang, L. Shi, Y. Zhu, M. F. Mideksa, K. Hou, W. Zhao, D. Wang, M. Zhao, X. Zhang, J. Lv, J. Zhang, X. Wang and Z. Tang, J. Am. Chem. Soc., 2017, 139, 17964 CrossRef CAS PubMed
.
|
This journal is © The Royal Society of Chemistry 2024 |