Defect-engineered Zr-MOFs with enhanced O2 adsorption and activation for photocatalytic H2O2 synthesis†
Received
8th October 2023
, Accepted 25th November 2023
First published on 27th November 2023
Abstract
Defect engineering has recently received much attention as an effective approach for improving photocatalytic performances. Herein, UiO-66-NH2 with missing ligand defects (DUiO-66-NH2) wrapped by ZnIn2S4 was investigated for photocatalytic H2O2 production under visible light. The defects in DUiO-66-NH2 enable efficient charge separation and O2 capture, which are the key factors in accelerating H2O2 production. Specifically, the O2 adsorption capacity over ZnIn2S4/DUiO-66-NH2 was improved by a factor of 3.1 as compared to its counterpart without defects. As a result, in ambient air and pure water without any sacrificial agents, the H2O2 yield of ZnIn2S4/DUiO-66-NH2 was 340 μmol L−1, which was significantly higher than that of ZnIn2S4/UiO-66-NH2 (150 μmol L−1). The main H2O2 formation pathway over ZnIn2S4/DUiO-66-NH2 is an indirect oxygen reduction reaction with ·O2− as the intermediate, and the defects in UiO-66-NH2 could act as active sites to adsorb and activate O2 to produce ·O2−. However, the H2O2 generation over ZnIn2S4/UiO-66-NH2 undergoes both indirect and direct oxygen reduction reactions. This work could provide new insights and inspire further research into defect engineering of MOFs and photocatalytic H2O2 synthesis.
1. Introduction
H2O2 is a green, versatile and high-energy oxidant, and has great application values in the fields of bleaching, pollutant removal and chemical synthesis.1,2 Moreover, H2O2 also has the ability to store hydrogen.3 Under such a background, the global demand for H2O2 will reach a staggering level in the next decades.4 In industry, the anthraquinone process is used to produce H2O2, which is complicated and would produce a large amount of inorganic salt waste.5,6 It is therefore of great significance to realize the green production of H2O2. Photocatalytic H2O2 synthesis through the O2 reduction reaction or the water oxidation reaction has been verified as a promising strategy,7,8 in which the O2 reduction reaction is readily conducted and has been broadly explored. Since O2 is a major reactant, its capture and activation will inevitably affect the formation of H2O2, especially when the reactions take place in ambient air. Meanwhile, few studies have considered the effect of O2 adsorption and activation,9 and increasing O2 adsorption and activation would kinetically and thermodynamically accelerate H2O2 production.
Metal–organic frameworks (MOFs) are multifunctional materials constructed by coordination bonds between inorganic nodes (metal ions/metal clusters) and organic ligands,10,11 which have a broad range of applications.12–14 Particularly, due to their semiconducting behavior and tunable optical properties, MOFs are in the spotlight in photocatalysis.15–17 Nonetheless, photocatalytic performances of pristine MOFs are usually poor owing to the rapid recombination of photo-induced charge carriers.18–20 To address the above problem, defect engineering on MOFs has recently emerged as a viable countermeasure by modulating the electronic energy band structure.21 Investigations of defective MOFs mainly focus on Zr-MOFs (e.g. UiO-66 and UiO-66-NH2) because of their excellent structural stability.22,23 Jiang et al. found that moderate defects in UiO-66-NH2 enabled an energy decrease of the unoccupied d orbitals of Zr atoms, leading to improved charge separation and transfer.24 For the photocatalytic production of H2O2, the Yamashita group found that the defects could also restrain the decomposition of H2O2 (ref. 25) and the nonradiative relaxation of ligands.7 Additionally, defects in MOFs may result in a high porosity and large surface area, as well as coordination-unsaturated metal sites that can act as catalytically active centers.26,27 Based on these, it is an essential task to elucidate the correlation between MOF defects and O2 adsorption/activation in the reaction of H2O2 generation from O2 reduction.
Herein, UiO-66-NH2 with missing ligand defects (DUiO-66-NH2) was prepared and then decorated by two-dimensional ZnIn2S4 for photocatalytic H2O2 production in pure water and ambient air under visible light. The introduction of ZnIn2S4 boosts the visible-light response and constructs a heterojunction with MOFs for optimizing charge separation. In comparison with ZnIn2S4/UiO-66-NH2, ZnIn2S4/DUiO-66-NH2 exhibited a much higher H2O2 yield. Based on a series of experiments and characterization, the effects of defects in DUiO-66-NH2 on the textural properties and H2O2 synthesis reactions were clarified. It is noted that the defects could accelerate the charge separation and O2 adsorption and activation. And interestingly, the H2O2 formation pathway changed from two modes (direct and indirect O2 reduction reactions) on ZnIn2S4/UiO-66-NH2 to one mode (indirect O2 reduction reaction) on ZnIn2S4/DUiO-66-NH2. This study could offer a novel insight into defective MOFs in the green synthesis of H2O2.
2. Experimental
2.1. Materials
Thioacetamide (TAA, ≥99%), N,N-dimethylformamide (DMF), formic acid and ethanol were purchased from Sinopharm. Zirconium chloride (ZrCl4, 98%) and p-benzoquinone were bought from Aladdin. Zinc chloride (ZnCl2, ≥98%) and anhydrous acetone (≥98%) were obtained from Nanjing Chemical Reagent. Indium chloride tetrahydrate (InCl3·4H2O, >99%) was obtained from Macklin. 2-Aminoterephthalic acid (ATA, ≥98%) was purchased from Sigma-Aldrich.
2.2. Preparation of UiO-66-NH2 and DUiO-66-NH2
UiO-66-NH2 was synthesized by a solvothermal method at 120 °C for 24 h in a 100 mL autoclave with 40 mL of DMF, 190 mg of ZrCl4 and 146 mg of ATA. The resultant solid was obtained by centrifugation, rinsed with DMF, anhydrous acetone and ethanol, and dried at 80 °C overnight. DUiO-66-NH2 was prepared with the same processes, but extra formic acid (3 mL) was added to the precursor solution. In addition, the final defective samples were gained after heat treatment at 270 °C for 2 h to remove the coordinated formic acid to form MOFs with missing ligand defects. The preparation illustrations of UiO-66-NH2 and DUiO-66-NH2 are displayed in Fig. 1a.
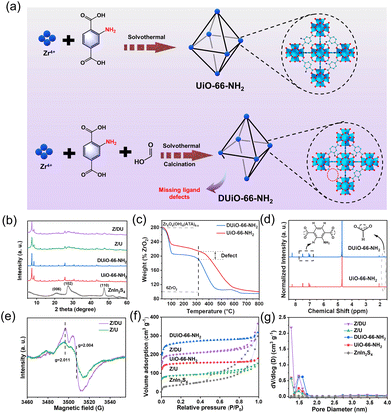 |
| Fig. 1 Preparation illustrations of UiO-66-NH2 and DUiO-66-NH2 (a), XRD patterns of UiO-66-NH2, DUiO-66-NH2, ZnIn2S4, Z/U and Z/DU (b), TG plots (c) and 1H NMR spectra (d) of UiO-66-NH2 and DUiO-66-NH2, EPR spectra of Z/U and Z/DU after 10 min illumination (e), N2 adsorption–desorption isotherms (f) and pore size distribution curves (g) of UiO-66-NH2, DUiO-66-NH2, ZnIn2S4, Z/U and Z/DU. | |
2.3. Preparation of ZnIn2S4/UiO-66-NH2 and ZnIn2S4/DUiO-66-NH2
In general, 282 mg of Zr-MOFs was dispersed in 100 mL of deionized water and sonicated for 30 min, after which 587 mg of InCl3·4H2O, 137 mg of ZnCl2 and 451 mg of TAA were added and stirred for another 30 min, and the resultant suspension was sealed and stirred for 2 h at 80 °C in an oil bath. Then, the solid was obtained after centrifugation and washed three times with water and ethanol, and finally dried at 80 °C. Notably, ZnIn2S4 growth on DUiO-66-NH2 and UiO-66-NH2 were named Z/DU and Z/U, respectively.
2.4. Characterization
The crystal structures of the prepared samples were studied by X-ray diffraction (XRD, Rigaku Ultima IV). N2 adsorption–desorption isotherms were acquired using a Micromeritics TriStar II apparatus. Their microstructures were observed by scanning electron microscopy (SEM, Hitachi Regulus 8100) and transmission electron microscopy (TEM, JEOL JEM-2100). Energy dispersive X-ray spectrometry (EDS, Hitachi Regulus 8100) was used for elemental analyses of samples. UV-vis diffuse reflectance spectra (DRS) were documented using a Shimadzu UV-2600 photometer. Fourier transform infrared spectroscopy (FTIR) was carried out on a Thermo Electron Nicolet-360 instrument. X-ray photoelectron spectroscopy (XPS) was conducted using an AXIS UltraDLD machine. Photocurrent and electrochemical impedance spectra (EIS), linear sweep voltammetry (LSV) curves and Mott–Schottky plots were tested on a CHI-760E electrochemical workstation. Steady-state photoluminescence (PL) and time-resolved photoluminescence decay (TRPD) spectra were measured using a FluoroMax-4. Electron paramagnetic resonance (EPR) was conducted on a Bruker EMXPLUS spectrometer. Thermogravimetric analysis (TGA) was carried out on a STA2500 thermal analyzer. Nuclear magnetic resonance (NMR) spectra were monitored on an AVANCE III HD equipment.
2.5. Photocatalytic experiments
Typically, 20 mg of photocatalyst was scattered in 40 mL of deionized water. After 30 min stirring, the photocatalytic reaction was triggered through exposure under a 300 W xenon lamp (400–780 nm). At specific time points, a certain amount of the suspension was pipetted and filtered. The production of H2O2 was detected at 350 nm based on iodometry (the corresponding standard curve is displayed in Fig. S1†). The apparent quantum yield (AQY) was studied using the following equation at different irradiation wavelengths:
3. Results and discussion
3.1. Material characterization
XRD patterns indicate the high crystallinity of both UiO-66-NH2 and DUiO-66-NH2 (Fig. 1b),28 suggesting that the proper missing ligand defects do not destroy the crystal structure. After ZnIn2S4 growth, the characteristic peaks of ZnIn2S4 emerge on the patterns of Z/U and Z/DU, indicating the successful decoration of ZnIn2S4 to Zr-MOFs. The FTIR spectra of UiO-66-NH2, DUiO-66-NH2, Z/U and Z/DU are similar overall (Fig. S2†), but the bands at 1257 (C–N), 1501 (C
C) and 1624 (N–H) cm−1 for DUiO-66-NH2 and Z/DU29 are weaker than those of UiO-66-NH2 and Z/U, respectively, implying the partial loss of ATA ligands. To further determine the defects, TGA, 1H NMR and EPR spectra were employed to explore the defects in DUiO-66-NH2. TGA plots of Zr-MOFs show three weight loss segments: the loss of water of crystallization in 25–100 °C, the removal of solvent DMF from 100 to 320 °C and the combustion and fracture of NH2-BDC ligands after 320 °C (Fig. 1c).30 It is worth noting that the final phase of weight loss marks the defective extent of ligands. From the fracture of ligands to the complete transformation into ZrO2, the weight loss ratio of DUiO-66-NH2 is obviously smaller than that of UiO-66-NH2, suggesting the existence of ligand defects in DUiO-66-NH2.31 For 1H NMR measurements, a D2O solution of NaOH was used to digest UiO-66-NH2 and DUiO-66-NH2 (Fig. 1d).32 The chemical shifts at 6.99, 7.06 and 7.49 ppm show the benzene ring structures of ATA,33 and the peak at 2.06 ppm is ascribed to the formation of Zr–OH. Notably, DUiO-66-NH2 shows an extra but small H peak from HCOO− at the chemical shift of 1.71 ppm,34 which is triggered by the residual HCOO– in the structure of DUiO-66-NH2, indicating that ATA was partially substituted by HCOOH to coordinate with Zr–O clusters. In addition, the EPR spectrum of Z/DU shows more apparent peaks at g = 2.011 and 2.004 than Z/U after 10 min illumination (Fig. 1e). The two peaks are allocated to Zr(III) produced from ligand to metal charge transfer in UiO-66-NH2, which implies a higher charge transfer efficiency due to the existence of ligand defects.35 It is known that the ligand-missing defects have an impact on the specific surface area and porosity of MOFs. As illustrated in Fig. 1f, the specific surface areas of DUiO-66-NH2 and UiO-66-NH2 are 814 and 467 m2 g−1, respectively. After integration with ZnIn2S4, the specific surface areas still remain as 624 and 271 m2 g−1 for Z/DU and Z/U, respectively. Accordingly, the pore volumes of UiO-66-NH2, DUiO-66-NH2, Z/U and Z/DU are 0.204, 0.379, 0.127 and 0.302 cm3 g−1, respectively (Fig. 1g). The boosted specific surface areas and pore volumes of DUiO-66-NH2 and Z/DU are attributed to more pore space formed in the presence of missing ligand defects.35 By now, the missing ligand defects in DUiO-66-NH2 and Z/DU are confirmed indubitably.
The construction of defects may influence the crystal growth of UiO-66-NH2 and the subsequent growth of ZnIn2S4. The SEM image of pristine UiO-66-NH2 shows an irregular structure and a rough surface (Fig. 2a),36 while DUiO-66-NH2 possesses a regular and uniform octahedral structure with a size of ∼260 nm (Fig. 2b). This is due to the addition of monocarboxylic acid (formic acid) competing with the ligands (ATA, dicarboxylic acid) for coordination and timely cutting of the crystal cell intergrowth.37 ZnIn2S4 presents dense flower-shaped microspheres with interlaced layered structures (Fig. S3†). Interestingly, with ZnIn2S4in situ growth, UiO-66-NH2 was thoroughly buried by ZnIn2S4 nanosheets (Fig. 2c). However, ZnIn2S4 nanosheets can be exfoliated by DUiO-66-NH2 and coated on the surface of DUiO-66-NH2 uniformly (Fig. 2d). TEM and high-resolution TEM images (Fig. 2e and f) of Z/DU further distinguish the surface ZnIn2S4 lamellas and the interface between ZnIn2S4 and DUiO-66-NH2. Such a structure of Z/DU is more beneficial for the mass and charge transfer compared with that of Z/U. The above results indicate that the defects in DUiO-66-NH2 have a huge impact on the growth of ZnIn2S4 and could effectively inhibit the aggregation of ZnIn2S4 nanosheets. EDS mapping images shed light on the even distribution of the elements S, In, Zn, Zr, C, N and O in Z/DU (Fig. S4†) and valence states of these elements were determined by XPS (Fig. S5†), where the valence state of each element is in line with the compositions of Z/DU and Z/U.
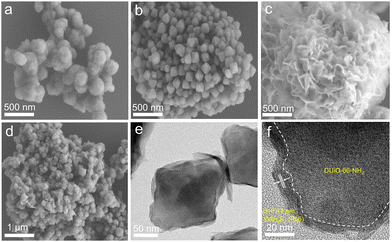 |
| Fig. 2 SEM images of UiO-66-NH2 (a), DUiO-66-NH2 (b), Z/U (c) and Z/DU (d), TEM images of Z/DU (e and f). | |
3.2. Photocatalytic performances of H2O2 production and mechanism
UiO-66-NH2 with different HCOOH/ATA molar ratios was prepared for photocatalytic H2O2 production first (Fig. S6†) and the results demonstrate that DUiO-66-NH2 with a HCOOH/ATA molar ratio of 100
:
1 (3 mL of HCOOH addition) has the highest H2O2 yield of 57 μmol L−1, but the performance is still far from ideal due to the rapid recombination of photo-excited charge carriers. ZnIn2S4 also has poor activity (52 μmol L−1, Fig. 3a), which is because of the limited active sites and fast charge recombination as well. Z/DU and Z/U possess an appreciably enhanced H2O2 yield of 340 and 150 μmol L−1, respectively. Additionally, different weight ratios of DUiO-66-NH2 in Z/DU were tested for photocatalytic H2O2 production (Fig. S7†) and Z/DU with a 40% weight ratio of DUiO-66-NH2 is the best sample. The H2O2 production rate of Z/DU is competitive to the related MOF-based photocatalysts (Table S1†). Subsequently, the performance reproducibility of H2O2 production over Z/DU was tested. A slight decrease in the H2O2 yields over Z/DU was detected after four-time cycling reactions (Fig. S8†), and the crystal structure, morphology, valence states and chemical structure of Z/DU have no obvious changes (Fig. S9–S11†), indicating the favorable recyclability and stability. To investigate the dynamic process of photocatalytic H2O2 production over Z/DU and Z/U, the rate constants of H2O2 formation (Kf, μmol L−1 min−1) and decomposition (Kd, min−1) were estimated based on [H2O2] = (Kf/Kd)(1 − exp(−Kdt)) (Fig. S12†).38 Compared with Z/U, Z/DU possesses a higher Kf but a lower Kd, which is conducive to the H2O2 generation. Furthermore, AQYs of Z/DU for photocatalytic H2O2 production at 360, 400, 550 and 650 nm wavelengths were tested (Fig. 3b). The results were consistent with the trend of UV-vis DRS, indicating the excellent utilization of light in the process of generating H2O2 over Z/DU.
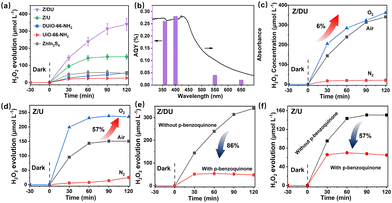 |
| Fig. 3 Photocatalytic H2O2 production over ZnIn2S4, UiO-66-NH2, DUiO-66-NH2, Z/U and Z/DU (a), AQYs of Z/DU for H2O2 production and UV-vis DRS of Z/DU (b), photocatalytic H2O2 production over Z/DU (c and e) and Z/U (d and f) in various gas atmospheres, with and without p-benzoquinone. | |
Photocatalytic production of H2O2 in different gas atmospheres (O2 and N2) is studied to elucidate the formation pathway of H2O2. For both Z/DU and Z/U (Fig. 3c and d), in comparison with reactions in air, reactions in O2 accelerate the H2O2 generation but the H2O2 generation in N2 has a pronounced shrinkage, proving that the H2O2 formation mainly stems from O2 reduction rather than water oxidation. Of note, the H2O2 yield in O2 merely has a slight enhancement by 6% compared with that in air over Z/DU, but this enhancement is 57% over Z/U, implying that Z/DU has a quite higher utilization of O2 in air than that of Z/U. Since the O2 reduction reaction to produce H2O2 is distinguished as direct (O2 + 2H+ + 2e− → H2O2) and indirect pathways (O2 + e− → ·O2−, ·O2− + e− + 2H+ → H2O2),39,40 to determine this, ·O2− trapping experiments using p-benzoquinone as a scavenger were conducted over Z/DU and Z/U. As displayed in Fig. 3e, after addition of p-benzoquinone, the H2O2 yield over Z/DU has a sharp decrease by 86%, which demonstrates that the formation of H2O2 over Z/DU mainly undergoes an indirect O2 reduction reaction with ·O2− as intermediates. Nevertheless, the H2O2 yield only decreases by 57% over Z/U with the existence of p-benzoquinone, suggesting that H2O2 production over Z/U experiences both indirect and direct O2 reduction reactions. The above difference in H2O2 formation pathways is probably due to missing ligand defects weakening the proton-coupled capacity of Z/DU, because the abatement of amino groups on ligands may decrease the hydrogen-bond interaction between Z/DU and water. In addition, to determine the average electron transfer number (n) of the O2 reduction reaction, the rotating disk electrode test was performed. The n values of both Z/DU and Z/U are close to 2, indicating that they have mainly undergone a process of two-electron reduction of O2 to H2O2 (Fig. S13†).
From the results of photocatalytic H2O2 production, it can be seen that there is an increase in the H2O2 yield by 2.3 fold over Z/DU compared to Z/U, where defects play a vital role in this process. On the one hand, defects in DUiO-66-NH2 would expedite charge separation and transportation.41 To explore this, behaviors of charge separation and transfer over Z/U and Z/DU were characterized by a series of characterization techniques including photocurrent spectroscopy, EIS, LSV, PL and TRPD. On account of the heterostructure formation, Z/U and Z/DU exhibited stronger photocurrent signals than their monomers (Fig. 4a). Additionally, the photocurrent signal over Z/DU is more obvious than that over Z/U. Meanwhile, the resistance on Z/DU is also smaller (Fig. S14†), and the LSV curves imply that the overpotential on Z/DU is lower than that on Z/U (Fig. 4b).42,43 Furthermore, the weaker photoluminescence intensity of Z/DU suggests a low recombination frequency of photo-induced electrons and holes (Fig. 4c), and the charge lifespan on Z/DU (0.91 ns) is also longer than that on Z/U (0.73 ns, Fig. 4d). The above results undoubtedly confirm that more efficient charge transportation and separation over Z/DU are achieved in comparison with those over Z/U.44
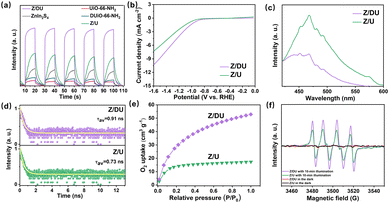 |
| Fig. 4 Photocurrent spectra of ZnIn2S4, UiO-66-NH2, DUiO-66-NH2, Z/U and Z/DU (a), LSV curves (b), PL (c) and TRPL (d) spectra and O2 adsorption isotherms (e) of Z/U and Z/DU, and EPR spectra of DMPO–·O2− adducts in methanol over Z/U and Z/DU in the dark and 10 min irradiation (f). | |
On the other hand, defects in DUiO-66-NH2 can prompt O2 adsorption and activation to convert into ·O2− since the H2O2 formation over Z/DU mainly experiences an indirect O2 reduction reaction. The O2 adsorption capacities of Z/DU and Z/U were measured using O2 adsorption isotherms. The maximum O2 adsorption capacity of Z/DU is 53 cm3 g−1, which is 3.1 fold larger than that of Z/U (Fig. 4e), suggesting that defects could promote the O2 adsorption. The promoting effect is likely to be aroused by the boosted surface area and coordination-unsaturated metal sites in the presence of missing ligand defects. The generation of ·O2− was detected by EPR (Fig. 4f). No corresponding signals appeared in the absence of light illumination, and evident characteristic peaks of ·O2− are present with light illumination, suggesting that ·O2− comes from light excitation.45 In comparison, the ·O2− signal over Z/DU is stronger than that over Z/U, mainly due to the more efficient charge separation and O2 capture, indirectly confirming that defects in DUiO-66-NH2 are conducive to O2 activation to convert into ·O2−.
To disclose the effects of missing ligand defects on light absorption and band configuration, and finally define the charge transfer routes for photocatalytic H2O2 production over Z/DU, UV-vis DRS, XPS valence band (VB) spectra and Mott–Schottky plots were detected. The light absorption edge of DUiO-66-NH2 has a slight blue shift compared to that of UiO-66-NH2 (Fig. 5a), which is caused by the reduction of amino groups along with the ligand defects. It is known that amino substituents can act as auxochromic and bathochromic groups in aromatic rings that is the reason for UiO-66-NH2 with visible-light absorption. After combination with ZnIn2S4, both Z/DU and Z/U have good visible-light absorption. Based on the curves of (αhv)2versus hv, bandgaps of DUiO-66-NH2, UiO-66-NH2 and ZnIn2S4 were determined as 2.80, 2.75 and 2.42 eV, respectively (Fig. 5b). Moreover, the highest occupied molecular orbital (HOMO)/VB potentials of DUiO-66-NH2, UiO-66-NH2 and ZnIn2S4 were estimated as 2.34, 2.20 and 1.40 eV, respectively, according to their XPS VB/HOMO spectra (Fig. 5c). To determine their lowest unoccupied molecular orbital (LUMO)/conduction band (CB) potentials, Mott–Schottky plots of DUiO-66-NH2, UiO-66-NH2 and ZnIn2S4 were assayed at 500, 1000 and 1500 Hz (Fig. 5d–f), and all samples were proved to be n-type semiconductors. Since the Ag/AgCl electrode is the reference electrode, the flat-band potentials of DUiO-66-NH2, UiO-66-NH2 and ZnIn2S4 were −0.363, −0.473 and −0.803 V vs. NHE (ENHE = EAg/AgCl + 0.197 V), respectively. For n-type semiconductors, the LUMO/CB potentials are 0.1 V less than the corresponding flat-band potentials.46 Hence, the LUMO potentials of DUiO-66-NH2 and UiO-66-NH2 and the CB potential of ZnIn2S4 are −0.463, −0.573 and −0.903 V, respectively. In accordance with the equation of Ebandgap = EVB/HOMO − ECB/LUMO,47 the bandgaps of DUiO-66-NH2, UiO-66-NH2 and ZnIn2S4 were calculated as 2.803, 2.773 and 2.303 eV, respectively, which are close to the results obtained from the corresponding curves of (αhv)2versus hv.
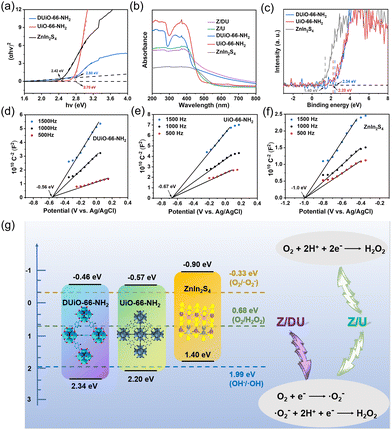 |
| Fig. 5 (αhv)2versus hv curves of ZnIn2S4, UiO-66-NH2 and DUiO-66-NH2 (a), UV-vis DRS of ZnIn2S4, UiO-66-NH2, DUiO-66-NH2, Z/U and Z/DU (b), VB/HOMO XPS spectra of ZnIn2S4, UiO-66-NH2 and DUiO-66-NH2 (c), Mott–Schottky plots of DUiO-66-NH2 (d), UiO-66-NH2 (e) and ZnIn2S4 (f) at 500, 1000 and 1500 Hz, band configurations of DUiO-66-NH2, UiO-66-NH2 and ZnIn2S4 (g). | |
Now, the band configurations of DUiO-66-NH2, UiO-66-NH2 and ZnIn2S4 are affirmed and presented in Fig. 5g. All of them are easy to stimulate to produce electrons and holes under visible light due to their narrow bandgaps, and their electrons on the CB are able to reduce O2 to generate H2O2via either indirect or direct pathway. As a summary, H2O2 production pathways over Z/DU and Z/U were also exhibited. To infer the charge transfer behavior on Z/DU and Z/U, the presence or absence of ·OH generation is an important index (E(OH−/·OH) = 1.99 eV).48 However, the EPR characteristic peaks of ·OH over both Z/DU and Z/U are not obvious after illumination (Fig. S15 and S16†), suggesting that little ·OH is produced and holes on the VB of DUiO-66-NH2 or UiO-66-NH2 are migrated to the VB of ZnIn2S4. Hence, a type II heterostructure would be formed between DUiO-66-NH2 (UiO-66-NH2) and ZnIn2S4, which facilitates the charge transfer. In addition, the presence of defects in DUiO-66-NH2 could further promote the charge separation, and, significantly, O2 adsorption and activation are accelerated as well, which finally improves the H2O2 production.
4. Conclusions
In summary, DUiO-66-NH2 and UiO-66-NH2 were prepared and modified by ZnIn2S4 for photocatalytic H2O2 synthesis in pure water and ambient air under visible light. Z/DU exhibited a higher H2O2 yield of 350 μmol L−1 than Z/U (150 μmol L−1). Z/DU and Z/U produce H2O2 in different O2 reduction modes. Z/DU primarily experiences an indirect O2 reduction reaction with ·O2− as an intermediate product, while Z/U has both indirect and direct O2 reduction modes. The performance enhancement over Z/DU is allocated to the efficient charge separation and O2 capture initiated by the missing ligand defects, and finally facilitating the activation of O2 to transform into ·O2−. This study provides a new perspective on defect engineering of MOFs and we hope that it will shed light on the photocatalytic H2O2 synthesis from oxygen reduction reactions.
Conflicts of interest
There are no conflicts of interest.
Acknowledgements
The authors are grateful for the financial support from the Natural Science Foundation of Jiangsu Province Youth Fund (BK20210628) and the Scientific Research Foundation from Nanjing Forestry University. We also thank the Advanced Analysis & Testing Center, Nanjing Forestry University for sample tests.
References
- G. Xia, J. Qiu, D. Dai, L. Zhang, Y. Tang and J. Yao, AIChE J., 2023, 69, e18192 CrossRef CAS.
- Q. Yuan, M. Fan, Y. Zhao, J. Wu, J. Raj, Z. Wang, A. Wang, H. Sun, X. Xu, Y. Wu, K. Sun and J. Jiang, Appl. Catal., B, 2023, 324, 122195 CrossRef CAS.
- M. Kaur, S. Kumar, S. A. Younis, M. Yusuf, J. Lee, S. Weon, K.-H. Kim and A. K. Malik, Chem. Eng. J., 2021, 423, 130230 CrossRef CAS.
- A. Gopakumar, P. Ren, J. Chen, B. V. Manzolli Rodrigues, H. Y. Vincent Ching, A. Jaworski, S. V. Doorslaer, A. Rokicinska, P. Kustrowski, G. Barcaro, S. Monti, A. Slabon and S. Das, J. Am. Chem. Soc., 2022, 144, 2603–2613 CrossRef CAS PubMed.
- F. Sandelin, P. Oinas, T. Salmi, J. Paloniemi and H. Haario, Ind. Eng. Chem. Res., 2006, 45, 986–992 CrossRef CAS.
- M. Fan, J. Xu, Y. Wang, Q. Yuan, Y. Zhao, Z. Wang and J. Jiang, Chem. – Eur. J., 2022, 28, e202201996 CrossRef CAS PubMed.
- Y. Kondo, K. Honda, Y. Kuwahara, K. Mori, H. Kobayashi and H. Yamashita, ACS Catal., 2022, 12, 14825–14835 CrossRef CAS.
- Y. Yang, Y. Li, X. Ma, L. Xie, D. Lv, L. Jiang, J. He, D. Chen and J. Wang, Catal. Sci. Technol., 2023, 13, 5599–5609 RSC.
- Y. Li, F. Ma, L. Zheng, Y. Liu, Z. Wang, P. Wang, Z. Zheng, H. Cheng, Y. Dai and B. Huang, Mater. Horiz., 2021, 8, 2842–2850 RSC.
- S. Kato, J. Jung, T. Suenobu and S. Fukuzumi, Energy Environ. Sci., 2013, 6, 3756 RSC.
- Z. Ma, B. Guan, J. Guo, X. Wu, Y. Chen, J. Zhang, X. Jiang, S. Bao, L. Chen, K. Shu, H. Dang, Z. Guo, Z. Li, S. Yao and Z. Huang, Catal. Sci. Technol., 2023, 13, 4285–4347 RSC.
- N. A. A. Qasem, R. Ben-Mansour and M. A. Habib, Appl. Energy, 2018, 210, 317–326 CrossRef CAS.
- J. Tang, C. Huang, Y. Liu, T. Wang, M. Yu, H. Hao, W. Zeng, W. Huang, J. Wang and M. Wu, Coord. Chem. Rev., 2023, 490, 215211 CrossRef CAS.
- X. Wang, W. Xu, J. Gu, X. Yan, Y. Chen, M. Guo, G. Zhou, S. Tong, M. Ge, Y. Liu and C. Chen, Nanoscale, 2019, 11, 17782–17790 RSC.
- J. Qiu, X. Zhang, Y. Feng, X. Zhang, H. Wang and J. Yao, Appl. Catal., B, 2018, 231, 317–342 CrossRef CAS.
- J. Qiu, X.-F. Zhang, X. Zhang, Y. Feng, Y. Li, L. Yang, H. Lu and J. Yao, J. Hazard. Mater., 2018, 349, 234–241 CrossRef CAS PubMed.
- X. Chen, Y. Cai, R. Liang, Y. Tao, W. Wang, J. Zhao, X. Chen, H. Li and D. Zhang, Appl. Catal., B, 2020, 267, 118687 CrossRef CAS.
- S. Tasleem, M. Tahir and W. A. Khalifa, Int. J. Hydrogen Energy, 2021, 46, 14148–14189 CrossRef CAS.
- D. Dai, J. Qiu, G. Xia, Y. Tang, Z. Wu and J. Yao, Sep. Purif. Technol., 2023, 316, 123819 CrossRef CAS.
- Q. Yang, S. Li, R. Liang, L. Gao, S. Zhang, J. Jia, Y. Liu, R. Lyv, G. Li, S. Xiao and D. Zhang, J. Environ. Sci., 2023, 134, 126–137 CrossRef PubMed.
- Y. Gu, B. A. Anjali, S. Yoon, Y. Choe, Y. G. Chung and D.-W. Park, J. Mater. Chem. A, 2022, 10, 10051–10061 RSC.
- X. Chen, Y. Lyu, Z. Wang, X. Qiao, B. C. Gates and D. Yang, ACS Catal., 2020, 10, 2906–2914 CrossRef CAS.
- A. K. Kar, R. Sarkar, A. K. Manal, R. Kumar, S. Chakraborty, R. Ahuja and R. Srivastava, Appl. Catal., B, 2023, 325, 122385 CrossRef CAS.
- X. Ma, L. Wang, Q. Zhang and H. L. Jiang, Angew. Chem., Int. Ed., 2019, 58, 12175–12179 CrossRef CAS PubMed.
- Y. Kondo, Y. Kuwahara, K. Mori and H. Yamashita, J. Phys. Chem. C, 2021, 125, 27909–27918 CrossRef CAS.
- W. Wang, T. Sheng, S. Chen, Z. Xiang, F. Zhou, W. Zhu and H. Wang, Chem. Eng. J., 2023, 453, 139711 CrossRef CAS.
- W. Xiang, J. Ren, S. Chen, C. Shen, Y. Chen, M. Zhang and C.-j. Liu, Appl. Energy, 2020, 277, 115560 CrossRef CAS.
- S.-W. Lv, X. Wang, X. Wei, Y. Zhang, Y. Cong and L. Che, Sep. Purif. Technol., 2022, 292, 121018 CrossRef CAS.
- C. Zhao, Y. Li, H. Chu, X. Pan, L. Ling, P. Wang, H. Fu, C.-C. Wang and Z. Wang, J. Hazard. Mater., 2021, 419, 126466 CrossRef CAS PubMed.
- X. Zhang, X. Shi, J. Chen, Y. Yang and G. Lu, J. Environ. Eng., 2019, 7, 103405 CAS.
- Z.-D. Wang, Y. Zang, Z.-J. Liu, P. Peng, R. Wang and S.-Q. Zang, Appl. Catal., B, 2021, 288, 119941 CrossRef CAS.
- H. Saleem, U. Rafique and R. P. Davies, Microporous Mesoporous Mater., 2016, 221, 238–244 CrossRef CAS.
- Y. He, Y. Tan, M. Song, Q. Tu, M. Fu, L. Long, J. Wu, M. Xu and X. Liu, J. Hazard.
Mater., 2022, 430, 128468 CrossRef CAS PubMed.
- W. Jin, Y. Wang, W. Zhao, X. Du, Y. Tian, T. Ding and X. Li, Ind. Eng. Chem. Res., 2020, 59, 13491–13501 CrossRef CAS.
- S.-Q. Wang, X. Wang, X.-M. Cheng, J. Ma and W.-Y. Sun, J. Mater. Chem. A, 2022, 10, 16396–16402 RSC.
- T. Qian, Y. Zhang, J. Cai, W. Cao, T. Liu, Z. Chen, J. Liu, F. Li and L. Zhang, J. Colloid Interface Sci., 2021, 603, 582–593 CrossRef CAS PubMed.
- J. Qiu, Y. Feng, X. Zhang, M. Jia and J. Yao, J. Colloid Interface Sci., 2017, 499, 151–158 CrossRef CAS PubMed.
- Y. Yang, Z. Zeng, G. Zeng, D. Huang, R. Xiao, C. Zhang, C. Zhou, W. Xiong, W. Wang, M. Cheng, W. Xue, H. Guo, X. Tang and D. He, Appl. Catal., B, 2019, 258, 117956 CrossRef CAS.
- C. Liu, T. Bao, L. Yuan, C. Zhang, J. Wang, J. Wan and C. Yu, Adv. Funct. Mater., 2021, 32, 2111404 CrossRef.
- Y.-Y. Yang, C.-G. Niu, D.-W. Huang, H. Guo, H.-P. Feng, L. Li, H.-Y. Liu, Q.-Q. Fan and M.-Z. Qin, J. Hazard. Mater., 2023, 445, 130481 CrossRef CAS PubMed.
- J. Zhou, J. Zhao and R. Liu, Appl. Catal., B, 2020, 278, 119265 CrossRef CAS.
- H. Feng, J. Yu, J. Tang, L. Tang, Y. Liu, Y. Lu, J. Wang, T. Ni, Y. Yang and Y. Yi, J. Hazard. Mater., 2022, 430, 128464 CrossRef CAS PubMed.
- H. Feng, J. Yu, L. Tang, J. Wang, H. Dong, T. Ni, J. Tang, W. Tang, X. Zhu and C. Liang, Appl. Catal., B, 2021, 297, 120478 CrossRef CAS.
- A. De Vos, K. Hendrickx, P. Van Der Voort, V. Van Speybroeck and K. Lejaeghere, Chem. Mater., 2017, 29, 3006–3019 CrossRef CAS PubMed.
- Y.-Y. Yang, H.-P. Feng, C.-G. Niu, D.-W. Huang, H. Guo, C. Liang, H.-Y. Liu, S. Chen, N. Tang and L. Li, Chem. Eng. J., 2021, 426, 131902 CrossRef CAS.
- J. Qiu, L. Zhang, G. Xia, D. Dai, Y. Tang and J. Yao, Catal. Sci. Technol., 2023, 13, 2101–2107 RSC.
- J. Qiu, D. Dai, L. Zhang, G. Xia and J. Yao, Sep. Purif. Technol., 2022, 301, 121990 CrossRef CAS.
- J. Shi, S. Li, F. Wang, Y. Li, L. Gao, X. Zhang and J. Lu, Catal. Sci. Technol., 2018, 8, 6458–6467 RSC.
|
This journal is © The Royal Society of Chemistry 2024 |