DOI:
10.1039/D4CS00761A
(Tutorial Review)
Chem. Soc. Rev., 2024,
53, 10380-10408
Supramolecular and molecular capsules, cages and containers
Received
29th July 2024
First published on 1st October 2024
Abstract
Stemming from early seminal notions of molecular recognition and encapsulation, three-dimensional, cavity-containing capsular compounds and assemblies have attracted intense interest due to the ability to modulate chemical and physical properties of species encapsulated within these confined spaces compared to bulk environments. With such a diverse range of covalent motifs and non-covalent (supramolecular) interactions available to assemble building blocks, an incredibly wide-range of capsular-type architectures have been developed. Furthermore, synthetic tunability of the internal environments gives chemists the opportunity to engineer systems for uses in sensing, sequestration, catalysis and transport of molecules, just to name a few. In this tutorial review, an overview is provided into the design principles, synthesis, characterisation, structural facets and properties of coordination cages, porous organic cages, supramolecular capsules, foldamers and mechanically interlocked molecules. Using seminal and recent examples, the advantages and limitations of each system are explored, highlighting their application in various tasks and functions.
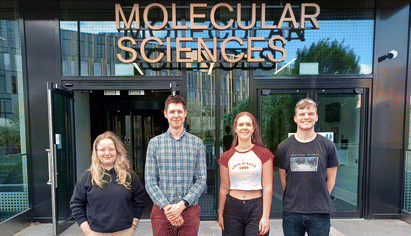
From left to right: Jessica Hale, Jamie Lewis, Paulina Molinska and Cameron Cox
| Cameron Cox (right) is a PhD student under the supervision of Jamie Lewis. He obtained his MChem from the University of Edinburgh in 2023 with a research project at The Australian National University. His research is focused on the synthesis of low-symmetry and template-directed covalent cages. Jessica Hale (left) obtained her MChem (Honors) at Lancaster University completing a research thesis on the enantioselective synthesis of azetidines with Vilius Franckevičius. In 2023, she joined the University of Birmingham to pursue her PhD under the supervision of Jamie Lewis and Chiara Arno on mechanically interlocked molecules in polymeric materials. Paulina Molinska (centre right) obtained her MSci from the University of Birmingham, with her master's project focusing on self-assembly of metal–organic cages in the Lewis Group. She started her PhD work, under the supervision of Jamie Lewis, in 2024. Her research interests include self-assembly and design principles of low-symmetry metal–organic cages. Jamie Lewis (centre left) obtained his PhD (2014) from the University of Otago, New Zealand, under the supervision of Prof. James Crowley. He then joined the group of Prof. Steve Goldup at the University of Southampton, initially as a PDRA and subsequently a Marie-Skłodowska-Curie Fellow. He began his independent career at Imperial College London in 2017. In 2022 Jamie moved to the University of Birmingham, with the award of a Royal Society University Research Fellowship, where he is now an Associate Professor in Supramolecular Chemistry. |
Key learning points
(1) Capsules can be prepared under both thermodynamic and kinetic control that display a wide range of structural, chemical and physical properties.
(2) Through careful design, building blocks can be prepared that self-assemble to form supramolecular capsules using various non-covalent interactions.
(3) The varying properties of different classes of capsules provide a range of systems to choose from depending on the intended application and operating environment.
(4) The host–guest chemistry of capsule-type systems can be exploited for applications in sequestration, sensing, separation, stabilisation and catalysis, amongst others.
|
Introduction
In 1967, Charles J. Pedersen reported the discovery of a new class of macrocyclic compounds, the crown ethers,1 and their ability to bind alkali metal ions within their cavities that would later be described as if the “ion had fallen into the hole in the center of the molecule.”2 Jean-Marie Lehn's subsequent work on bicyclic analogues of the crown ethers – named cryptands – demonstrated the power of extending these host systems into three dimensions (Fig. 1).3 This work would see Pedersen4 and Lehn,5 alongside Donald J. Cram,6 win the 1987 Nobel Prize in Chemistry “for their development and use of molecules with structure-specific interactions of high selectivity.” The concept of host–guest chemistry had been born.7
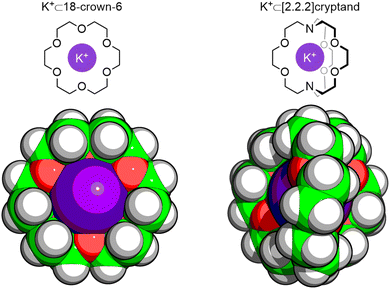 |
| Fig. 1 Single-crystal X-ray diffraction (SCXRD) structures of K+ complexes of Pedersen's macrocyclic 18-crown-6 ether and Lehn's macrobicyclic [2.2.2]cryptand. | |
Since this seminal work (and inspired by increased understanding of natural architectures like enzymes), chemists have been fascinated by the idea and challenges of preparing three-dimensional artificial host systems, often termed capsules, cages or containers. These include covalent systems (of which cryptands are arguably the progenitors), which can incorporate dynamic covalent bonds (such as imines), as well as those assembled using non-covalent (supramolecular) interactions.
Applications, both potential and realised, for molecular capsules are manifold. Encapsulation of guest species8 within the confined cavity spaces of these systems can result in unusual effects on properties and behaviours.9 Reactivity, in particular, can be altered upon encapsulation,10 which can be exploited for both stabilisation11 and promotion of chemical reactions.12
In this Tutorial Review, we introduce the most commonly investigated (supra-)molecular host architectures, outlining their key design principles, structural facets and properties, and link to relevant reviews and articles covering applications for which particular systems have been investigated. In this manner, we aim to provide a broad overview of the various capsule-type hosts and their suitability for use in different environments.
The terms capsule, cage and container are sometimes used interchangeably and sometimes with specific intent to distinguish between systems. In this review we have taken the former route, with no implied comment on the properties of a system from the noun applied. We have also limited our discussion of host–guest chemistry to reversible, supramolecular systems, the assembly of which is driven by non-covalent interactions between the host and guest and/or solvophobic forces.8,13 Such hosts and host–guest complexes are termed hemicarcerands and hemicarceplexes in the nomenclature of Cram, as opposed to kinetically trapped carceplexes in which destruction of the host is required for guest release.14
The systems discussed herein include coordination cages, anion-coordination-directed assemblies, porous-organic cages, assemblies held together by alternative non-covalent interactions (hydrogen, halogen and chalcogen bonds, π–π interactions and hydrophobic effects), foldamers and mechanically interlocked molecules, with selected examples chosen to highlight key principles. For each system, readers are directed to more in-depth reviews to provide greater detail.
Coordination cages
Coordination cages, or metal–organic cages/polyhedra (MOCs/MOPs), are self-assembled container molecules formed through coordination bond interactions between metal ions/nodes and ligand donors, generally under thermodynamic control. We note that these terms are sometimes applied explicitly to architectures assembled from ligands with neutral (often pyridyl) donors (coordination cages) and anionic carboxylate donors (MOCs/MOPs).15 We do not make such a distinction here, but rather view these as different motifs that can be employed for related assemblies depending on the desired properties of the target cage.
The topology of the thermodynamic product is determined by a balance of entropic and enthalpic factors, with the components tending to fulfil Lehn's concept of maximal site occupancy,16i.e. to form the greatest number of coordination bonds according to the denticity of the ligand and coordination number of the metal ion/node. For example, square-planar Pd(II) ions in combination with ditopic, or bis-monodentate, ligands will preferentially form PdnL2n-type assemblies. As demonstrated by extensive ligand engineering studies from the Fujita group, the value of n, in the absence of other factors, is largely controlled by the ligand structure, with the smallest possible assembly forming based on accessible conformations of the ligand (Fig. 2).17 Although structural factors are some of the most important considerations in designing MOCs, guest-induced/-templated transformations, including by counter-anions, can lead to the formation of unexpected species or structural rearrangements.18
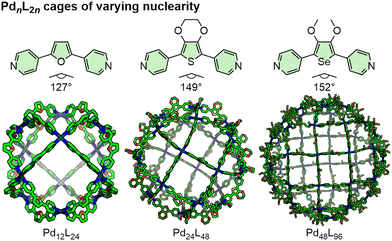 |
| Fig. 2 Different nuclearity PdnL2n coordination cages assembled from bis-monodentate ligands and Pd(II) ions (n = 12, 24 and 48 from left to right). | |
Whilst MOCs are most often formed as the thermodynamic product of self-assembly, there are notable examples of kinetically (meta)stable species. The Mirkin group has pioneered the concept of the weak-link approach (WLA), in which a thermodynamic architecture is used to pre-organise components; subsequent modification of the structure leads to formation of a kinetically trapped assembly that cannot be formed through direct reaction of the building blocks.19
The range of building blocks, and therefore accessible structures, that can be used to assemble MOCs is essentially infinite. Transition metal ions with coordination numbers typically between 3 and 6, as well as lanthanide ions able to coordinate to up to 12 donors,20 and a range of coordination geometries have been used. Additionally, capping ligands can be used to form kinetically stable metal nodes with limited coordination numbers and geometries; the most extensively studied of these is the cis-protected Pd(II) ion, with two coordination sites blocked via a chelating bidentate ligand such as ethylenediamine (en).21 Through selection of ligand denticity and metal ion/node coordination number, topologically related assemblies can be obtained from different building blocks (Fig. 3).22
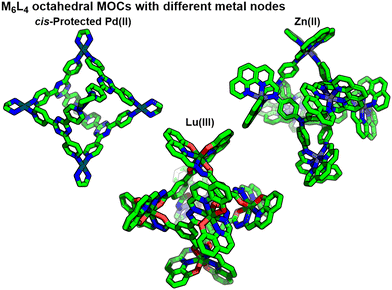 |
| Fig. 3 Octahedral M6L4-type coordination cages assembled from metal ions/nodes with different coordination numbers and ligands with different denticities. | |
With regards to the ligand structure, donor units can be neutral or anionic, with a range of denticities, and arranged in any number of geometries from acyclic or cavitand-based ligand scaffolds. Through the balance of charges between the metal ions and ligands, MOCs can be prepared that are cationic, neutral, or anionic (Fig. 4).23 Myriad studies have also been reported on the appendage of functional groups to both the external24 and internal25 surfaces of cages, endowing them with particular properties and functions. To limit formation of a distribution of products, including polymeric assemblies, relatively rigid ligands are generally used; flexible architectures, however, are not unprecedented.26
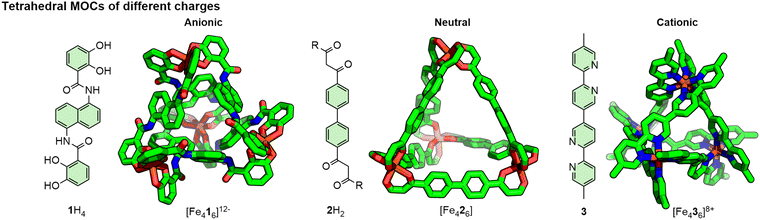 |
| Fig. 4 Tetrahedral Fe4L6 assemblies where the balance of charges between the ligands and metal ions results in anionic, neutral and cationic assemblies. R groups omitted from SCXRD structures for clarity. | |
Various design principles have been explicated for the targeted formation of MOC structures, often based on commonly recognised polyhedra, such as the Platonic solids (tetrahedron, cube, octahedron etc.), that position ligands on the edges, faces or vertices of these shapes.27 These include the symmetry interaction,28 directional bonding29 and molecular panelling30 approaches, with several excellent reviews available that cover these design strategies in detail.31 A subset of these design principles is the concept of sub-component self-assembly,32 in which the complete ligand scaffold is assembled in situ through dynamic covalent chemistry, such as the condensation of an amine building block with 2-formylpyridine to form bidentate pyridylimine moieties (Fig. 5).33
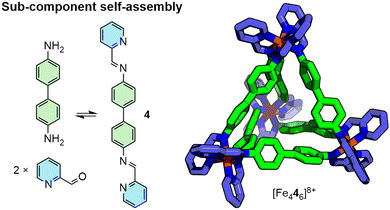 |
| Fig. 5 In sub-component self-assembly strategies, ligands are formed in situ using DCC. | |
It is noted that topologically similar architectures can often be obtained from different building blocks. The tetrapyridyl, square planar Pd(II) motif, for example, is structurally analogous to the tetracarboxylate dicopper(II) paddlewheel node and these can be used to form geometrically isostructural assemblies (Fig. 6).34 Thus, the choice of particular structural features can be dictated by restrictions imposed by the intended application without wholesale redesign of the assembly topology.
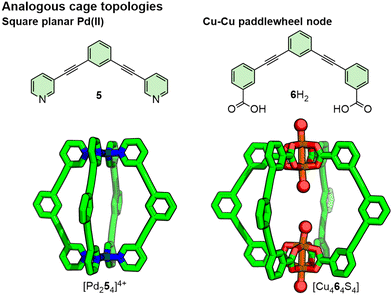 |
| Fig. 6 Topologically analogous architectures can be prepared from ligands and metal nodes with comparable geometries. Axial ligands (S) of [Cu464S4] have been simplified to red balls. | |
Most commonly, MOCs are assembled from a single type of ligand, referred to as homoleptic cages, and a single type of metal ion/node (homometallic or homonuclear). To increase the structural complexity of these systems,35 design principles have been developed to form low-symmetry cages (Fig. 7). These include heteroleptic (mixed-ligand) MOCs36 (Fig. 7a) from the self-assembly of ligand mixtures (a process referred to as integrative self-assembly)37 and heterometallic cages38 incorporating different metal ions (Fig. 7b).39 Additionally, investigations into directing the orientation-selective assembly of low-symmetry ligands,40 that would otherwise form a mixture of isomeric MOCs, have been reported (Fig. 7c).41 Recently, using different design strategies, examples of M2L4-type cages assembled from four different ligands have been reported, representing the state-of-the-art in this area.42
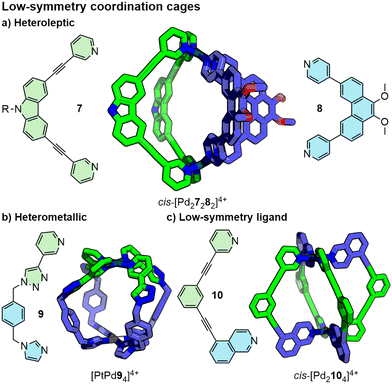 |
| Fig. 7 Low-symmetry coordination cages can be prepared via different strategies. R groups omitted from SCXRD structures for clarity. | |
The range of solvents across which a particular MOC is soluble tends to be quite limited. Cationic MOCs, for instance, are generally only soluble in high polarity solvents, such as acetonitrile and DMSO. The identity of counter-anions, however, can be used to dramatically alter solubility profiles; the highly lipophilic BArF− (tetrakis[3,5-bis(trifluoromethyl)phenyl]borate) anion was demonstrated to render Pd2L4-type MOCs soluble in apolar dichloromethane and chloroform,43 whilst sulfate anions were shown to make Fe(II) MOCs water-soluble.44 For MOCs based on carboxylate paddle-wheel motifs, exchanging axial ligands can be used to similar effect; 4-tert-butylpyridine, 4-trifluoromethylpyridine and L-proline were shown to make a Rh24L24 cuboctahedron soluble in DMF, CH2Cl2/THF and water, respectively (Fig. 8).45 Solubilising groups appended to ligand scaffolds are also commonly used to modify solubility. As the solvent of life, water-solubility is a commonly targeted property of MOCs, with various approaches investigated to achieve this.46
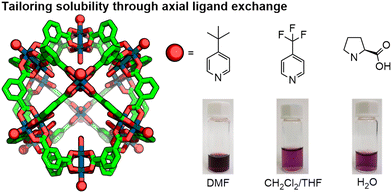 |
| Fig. 8 Through exchange of the exohedral axial ligands of the dirhodium paddlewheel nodes, the Rh24L24 cuboctahedron was rendered soluble in various solvents. Adapted with permission from ref. 45. Copyright 2019 American Chemical Society. | |
Due to the relatively strong nature of metal–ligand bonds, in comparison to other non-covalent interactions, MOCs are often stable under a range of conditions, and can be designed to undergo post-synthetic modifications.47 That being said, paddle-wheel nodes are known to be hydrolytically unstable,48 and metal–ligand bonds are susceptible to competing nucleophiles.49 If well understood, these can be used to prepare stimuli-responsive architectures.50 Attempts have been made to enhance the stability of MOCs51 through increasing the donor strength of the ligand,52 using more inert metal ions (e.g. Pt rather than Pd)53 and steric protection around the coordination sphere.54
The characterisation and study of MOCs in solution is most often carried out by NMR techniques, including diffusion-orientated spectroscopy (DOSY).55 This is generally simpler for systems assembled from diamagnetic metal ions, such as Pd(II) and low-spin Fe(II), although useful information can often be obtained for paramagnetic systems too.56 Mass spectrometry (MS) is also ubiquitous, although often challenging, particularly for larger systems, due to instability of MOCs under MS conditions, although various “softer” techniques have been successfully employed.57 Characterisation of MOCs in the solid-state by SCXRD is often considered the gold-standard for unambiguous structure determination; however, structures that successfully pack to form X-ray diffraction quality crystals are not necessarily representative of what is predominantly in solution. Thus, when used to make conclusions about the structure of metal–organic assemblies, SCXRD data should always be used to support solution-phase data, rather than in lieu of it.
As one of the most commonly explored classes of supramolecular capsules, there is a vast body of literature on the design and molecular engineering of MOCs.58 The relatively high strength and directionality of metal–ligand bonds, predictable coordination geometries of transition metal ions, and innumerable combinations of components that can be incorporated into functional ligand scaffolds make MOCs one of the most versatile and useful classes of molecular capsules. As a result, coordination cages have been investigated for wide-ranging applications in catalysis,59 molecular separations,60 sensing,61 stabilisation of reactive species,11 and in biomedical applications,62 with a multitude of reviews written over the years, a small selection of which the reader is directed to for more detailed discussion.
Anion-coordination-directed assemblies
Conceptually analogous to coordination cages, so-called anion-coordination-directed assemblies (ACDAs) are composed of ligand units bridging between anionic nodes. Rather than the dative covalent, metal–ligand interactions used within coordination cages, ACDAs exploit hydrogen bonding and other electrostatic interactions between anions and ligands to hold the structure together. The notion of anion coordination chemistry has been in discussion since at least the late 1970s,63 however it is only relatively recently that the field has reached sufficient maturity to enable the design and assembly of polyhedral architectures. Detailed historical overviews of ACDAs have been covered in recent reviews.64
In contrast to transition metals, with their often-predictable coordination numbers and geometries, the “coordination” environment around anions is less well-defined and more flexible. Phosphate, PO43−, has become the most intensely studied anion for self-assembly, presumably due to its defined tetrahedral shape and ability to form coordinatively saturated complexes with 12 hydrogen bonds.65 In contrast, sulfate, SO42−, is known to form coordinatively unsaturated assemblies, although capsules analogous to those with phosphate have been reported;66 assemblies formed from tris-carboxylate anions have also been realised.67
In terms of “coordinating groups”, urea moieties are popular choices, with ligands often consisting of bis- or tris-urea units (capable of forming 4 and 6 hydrogen bonds, respectively), and the manner in which these are arranged directing the topology of assembly formed (Fig. 9). In this manner, coordination of three bis-urea units around a PO43− anion is analogous to binding three bidentate ligands around an octahedral metal ion. For example, bis- and tris-(bis-urea) ligands (11 and 12, respectively) have been shown to assemble with PO43− anions to form edge-based A4L668 and face-based A4L4 tetrahedra,69 respectively (Fig. 9). Subtle structural modification of ligands, however, can result in significant changes to the resultant assembly. Whilst ligand 12 with a triphenylamine core assembled with PO43− to form an A4L4 tetrahedron, its phosphine oxide congener, 13, formed an A6L6 trigonal antiprism instead (Fig. 9).70
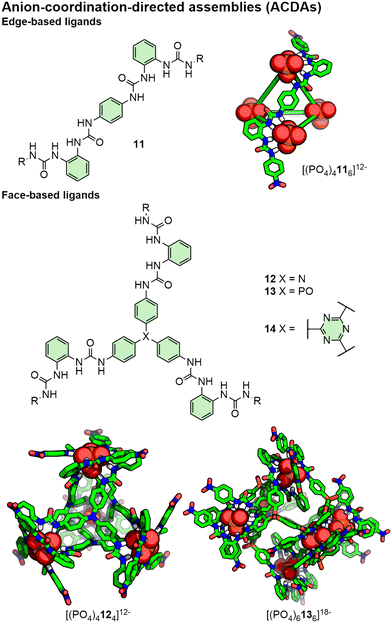 |
| Fig. 9 Ligands incorporating bis-urea “coordinating” groups can be used to assemble ACDAs of different geometries with ligands occupying edge- or face-based positions using design principles analogous to those for coordination cages. R groups omitted from SCXRD structures for clarity. | |
Such structures can be inherently chiral; A4L6 tetrahedra, for example, have vertices composed of anions surrounded by three coordinating units that can adopt Δ or Λ configurations. Chiral induction of ΔΔΔΔ- and ΛΛΛΛ-A4L6 tetrahedra has been demonstrated through the incorporation of chiral auxiliary units into the ligand scaffold71 and through interactions with chiral cations.72
Unsurprisingly, given their anionic nature, ACDAs are prone to binding cationic guests, although encapsulation of neutral molecules including halocarbons73 and P4 and As474 has been demonstrated. As with coordination cages, guest molecules (or lack thereof) can template the formation of different topologies. [(PO4)4116]12−, for example, formed in the presence of a tetramethylammonium guest; with tetrabutylammonium as the countercation, however, which was too large to fit within the cavity of the tetrahedron, an A2L3 helicate was formed.68 In contrast, tetrahedral cage [(PO4)4144]12− was found to form with or without a suitably sized cationic template, and exhibited conformational flexibility to the encapsulation of different sized ammonium cations.75
ACDAs are generally assembled, soluble, and stable in high polarity solvents such as acetonitrile, DMF and DMSO. As such, they can be characterised in solution using standard NMR, DOSY and MS techniques, as well as in the solid-state using SCXRD. The combined effects of multiple hydrogen bonds impart structural stability; the total dissociation energy of [(PO4)4124]12−, assembled from 48 hydrogen bonds, was calculated to be 1709 kJ mol−1.69 Acidic conditions, however, can protonate anions like PO43−, resulting in disassembly of the architecture.69
In summary, the key design principles of ACDAs are similar to those of coordination cages; ligands can be designed to be edge- or face-based, with the anion/ligand ratio controlled by the number of donor moieties, akin to the principal of maximal site occupancy,16 and the assembly stoichiometry controlled by the ligand geometry. The good solubility of ACDAs makes them suitable for study both in solution and the solid-state. Their anionic nature makes them particularly suited to the binding of cationic guests, while the ligand structure can be tailored to promote non-covalent interactions with encapsulated species. Whilst generally stable in the presence of bases, acidic conditions can lead to disassembly through protonation of the anionic nodes. As the first report of an ACDA capsule is little more than a decade old, it is perhaps unsurprising that applications for these systems have not been thoroughly explored. With rapidly growing developments in design principles, however, ACDAs have potential as highly useful supramolecular capsules. For a more detailed overview and analysis of ACDAs, including beyond capsule-type systems, the reader is directed to recent reviews in the area.64
Porous organic cages
The term porous organic cage (POC) is used to refer to capsular molecular systems that contain only covalent organic bonds within their structural scaffold. Several existing reviews provide an historical overview and comprehensive discussion of these systems.76 In this review, we have chosen to specifically distinguish between capsules, typically formed under thermodynamic control, that exploit reversible covalent bonds, and those formed under kinetic control (covalent-organic cages; COCs). It is noted, however, that the reversibility of “dynamic” bonds is dependent upon the conditions. A stark example of this are alkynes; generally considered kinetically robust, alkynes can be rendered dynamic under specific metathesis conditions.77 A prolonged discussion on this will not be provided here, but we simply wish to highlight this blurring of lines between different types of POCs.
Dynamic covalent cages
Dynamic-covalent/combinatorial chemistry (DCC) has become an increasingly important tool in supramolecular chemistry,78 allowing the high-yielding formation of organic molecular architectures from simple precursors in minimal steps and under thermodynamic control. Some of the most widely used dynamic covalent bonds include imines, boronic esters, disulfides, and alkenes and alkynes, each of which has been used to form covalent molecular capsules under thermodynamic control.
As with MOCs and ACDAs, the thermodynamic product(s) formed from a dynamic combinatorial library will be determined by a balance of entropic and enthalpic effects. POCs can, however, be formed as kinetic products,79 although it may not always be obvious when this is the case.80 Regardless of whether cages are kinetic or thermodynamic products, the solvent/conditions in which they are formed can have a significant impact on both the identity of the cage formed81 and its isolation.80 Additional guest species can also affect self-assembly outcomes by acting as templates.82
A significant determinant of what cage(s) can be/is formed will be the relative numbers and positions of the reactive functional groups in the sub-components. While early seminal examples were assembled from condensation of aldehyde-functionalised cavitands with diamine linking units,83 smaller, often commercially available, building blocks are now commonly used.
Jelfs and co-workers have outlined a simple system of nomenclature84 in which building blocks with complementary functionality, depending on the covalent bond being targeted (e.g. aldehyde and amine for imines, boronic acid and diol for boronic esters), are assigned as ditopic (Di), tritopic (Tri), or tetratopic (Tet) based on the number of reactive end groups. The stoichiometry of building blocks in the resultant assembly will be determined by their topicity, following analogous principles to Lehn's maximal site occupancy, e.g. a ditopic unit combined with a tritopic unit will likely give species with a 2
:
3 ratio ([2n+3n]) of Tri and Di units (Tri2nDi3n), with the precise formula dependent on the building block structures and external factors (Fig. 10a). It is noted, however, that there are exceptions to this rule, in which the stoichiometry of building blocks in a POC does not align with the ratio of their reactive groups.81b,85 By careful design of the building blocks, topologically comparable structures can be assembled using different reactions (Fig. 10b). Triformylbenzene, 15, and diamine 16 assemble to form the [4+6] (or Tri4Di6) tetrahedron 17;86 alternatively triamine 18 and dialdehyde 19 also form a [4+6] imine-based tetrahedron (20),87 whilst a boronic ester analogue (23) was formed from the combination of tris-diol 21 and bis-boronic acid 22.88
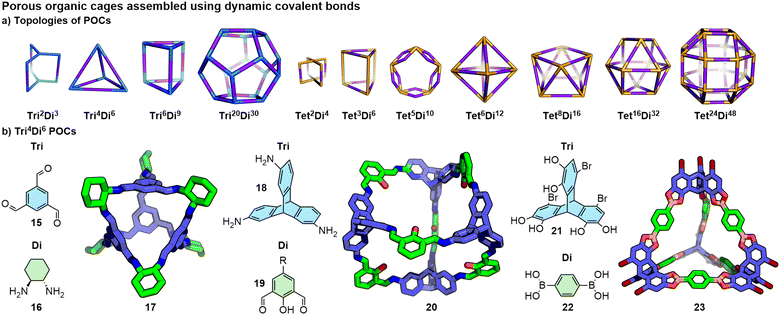 |
| Fig. 10 (a) The topologies of POCs formed through DCCs can be designated formulations based on the number of reactive groups on the building blocks. Reproduced from ref. 84 with permission from the Royal Society of Chemistry. (b) Through careful design, topologically analogous systems can be prepared with functional groups on different building blocks or using alternative reactions. R groups omitted from SCXRD structures for clarity. | |
While imines89 and boronic esters90 are some of the most commonly used dynamic covalent systems, various others have been reported. These include the condensation between resorcinol and dialdehydes forming noria (26)91 and oligoresorcinarene macrocycles,92 alkyne metathesis,93 and formation of disulfides94 and boroxines (Fig. 11).95 Orthogonal dynamic covalent linkages have also been used in concert, including imines and boronic esters,96 and imines and alkynes.97 Using these chemistries, and through careful design of building blocks, impressive architectures have been realised, including a 5.3 nm Tet12Di24 cuboctahedron assembled from a porphyrin tetramine and a dialdehyde.98
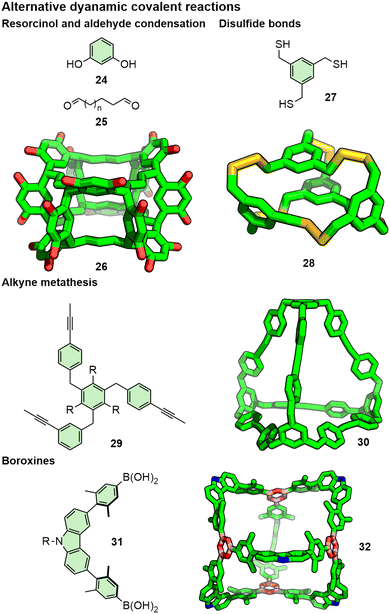 |
| Fig. 11 Alternative dynamic covalent reactions used to generate thermodynamically favoured organic capsules. R groups omitted from SCXRD structures for clarity. | |
Although often characterised and studied in the solid-state as porous materials,99 POCs are usually solution processable and have been used as components of porous liquids,100 and can be engineered to have sufficiently low melting points to render them as neat liquids.101 The conditions under which POCs are stable will depend on the nature of the structure and DCC being used. Imines, for example, are generally susceptible to hydrolysis, although examples of hydrolytically stable systems are known;102 structural tailoring has been used to enhance the stability of imine-based cages,103 or alternatively exploitation of keto-enol tautomerisation104 or use of more robust hydrazones105 provide access to more stable analogous systems. For some systems this is less of a consideration. Alkynes, for example, require a catalyst for metathesis; otherwise, they remain kinetically stable under a wide range of conditions. The dynamic nature of these organic cages can be exploited for stimuli-responsive transformations, such as the exchange of components in cage-to-cage conversions.106
With a wide range of dynamic covalent chemistries to choose from, and with a choice of building blocks only limited by synthetic accessibility,107 dynamic covalent cages are a versatile class of molecular capsules. Systems can be designed of different geometries and sizes with precise control over pore and cavity size108 and solubility,109 and covalent linkages chosen to be stable under a set of required conditions. Indeed, orthogonal DCCs can be combined to enable bond cleavage at specific sites upon application of specific stimuli,97 making them suitable for developing stimuli-responsive architectures. Detailed reviews on dynamic covalent POCs,76 and practical considerations for their synthesis and characterisation,110 providing in-depth information, are available for the reader's consumption.
Covalent-organic cages
Covalent-organic cages (COCs) are differentiated from dynamic POCs by virtue of containing no reversible linkages, making them generally more kinetically robust. This can, however, make their synthesis more challenging as, once an irreversible covalent bond has been formed, no error correction is possible.
COCs can be synthesised via direct covalent-bond forming reactions between components, either via a step-wise approach, or “one-pot” methods. Cryptands, arguably the first COCs, first reported by Lehn and co-workers in the late 1960s, were originally synthesised using the former method; iterative amide bond formation followed by reduction were used to synthesise first a macrocyclic precursor (35) and subsequently the macrobicyclic cryptand in an overall yield of ∼25% (Fig. 12a).3a In a similar vein, the stepwise formation of amide111 and ether-based112 systems (e.g.38) have been demonstrated. Ring-closing of acyclic precursors is also possible: Lee and Moore reported the elegant multi-step synthesis of cage 39 (Fig. 12b) via a series of Sonogashira reactions and use of protecting group strategies, with a final, intramolecular, double Sonogashira step.113
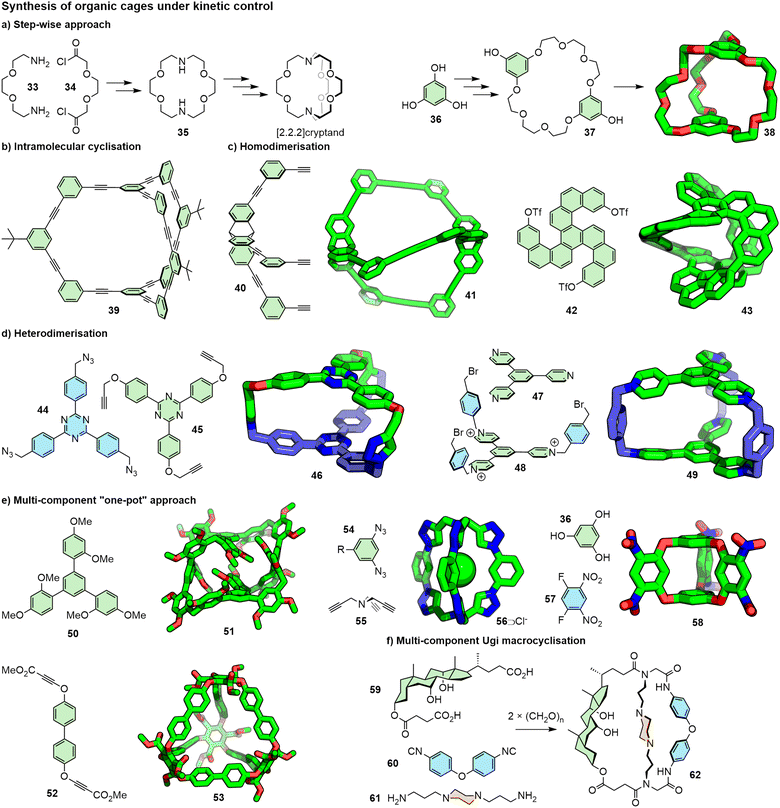 |
| Fig. 12 Kinetically stable organic cages can be prepared via a variety of methodologies and using myriad reactions. R groups omitted from SCXRD structures for clarity. | |
Conversely, “one-pot” approaches, entailing multiple bond-forming reactions between components, have been reported. The simplest of these involve reactions between two complementary building blocks to form [1+1] products. Macrobicyclic systems have been synthesised in this manner through homodimerisation of self-complementary units (Fig. 12c), such as by alkyne homocoupling (e.g.41)114 and Yamamoto coupling (e.g.43),115 and through heterodimerisation (Fig. 12d) using CuAAC chemistry (e.g.46),116 Sonogashira coupling,117 and nucleophilic substitution (e.g.49).118
Likewise, multi-component one-pot approaches have been reported. Such multi-component reactions are advantageous as the building blocks are relatively easy to access; however, yields can often suffer. Vögtle reported the synthesis of a macrotricyclic system through amide condensation: synthesis of the cage directly from a bis-acid chloride and trisamine in a [3 + 2] reaction yielded the desired cage in only 1.5% yield, whilst the [1+1] reaction between a tris-acid chloride, requiring a multi-step synthesis, and trisamine proceeded in 13% yield.119
Capsules have been prepared from oligomerisation of precursors (Fig. 12e), such as tetrameric capsule 51 that was synthesised from the condensation of 50 with paraformaldehyde,120 and cages such as 53 were synthesised through Rh-catalysed [2+2+2] cycloaddition of bis-alkyne precursors.121 Reactions between complementary building blocks have also been used to good effect, such as the [3+2] CuAAC reaction between bis-azide 54 and trialkyne 55122 and bicyclooxacalixarenes (e.g.58) synthesised via nucleophilic aromatic substitution.123 Reactions involving more than two reactive groups, such as the Ugi reaction, have also been used to generate structurally complex COCs (e.g.62; Fig. 12f).124 Whilst far from exhaustive, these examples demonstrate the versatility of chemistries that can be used for the synthesis of COCs.
To enhance the yields of cage-forming reactions, high, or pseudo-high, dilution conditions are often employed, as are relatively rigid precursors. Alternatively, templates can be used to pre-organise components and promote formation of the desired architecture (Fig. 13). The predictable coordination geometry of transition metal ions can make them very useful for such applications. Raymond, for example, demonstrated the efficiency of using an iron(III) template (Fig. 13a) to pre-organise catecholate units that were subsequently linked via amide bond formation with triamine 64, forming the iron complex of the cage (67) in 70% yield; by comparison, the [3+2] condensation of bis-acid chloride 63 with 64 under high dilution yielded cage 65 in 3.5% yield.125 Step-wise formation of a porphyrin nanoball via a macrocyclic intermediate was achieved using sequential coordination templates by Anderson and co-workers.126 Post-synthetic modification of pre-formed coordination cages, followed by demetallation, has also been used.127 Guest templates that interact with the incipient cage framework via alternative interactions, such as π–π118a,b and hydrogen bonding,128 have also been reported. Solvent choice can be critical for the successful formation of desired products,129 including through playing the role of template,116a dramatically affecting the yield of cage formation under kinetic control.
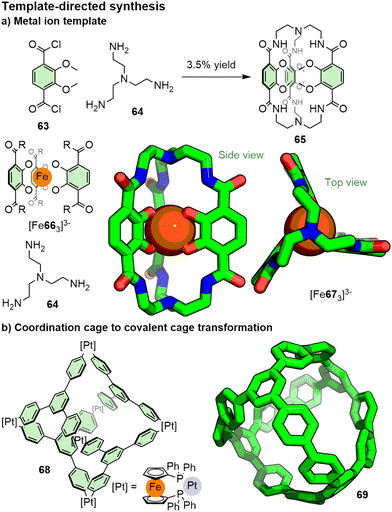 |
| Fig. 13 Templates can be used to increase the yields of covalent cages formed under kinetic control through pre-organisation of a thermodynamic precursor. | |
Alternatively, assemblies can be initially formed under thermodynamic control using dynamic bonds, with subsequent modification used to kinetically “lock” the structure. A number of examples have been reported of transforming POCs into COCs through transformation of imines to amines,130 amides,131 aminals,132 carbamates,133 quinolines,134 hydrocarbons,135 and, through an azedefluorination reaction of a reduced amine with an isocyanate, cyclic ureas.136 In a similar vein, Yamogo and co-workers reported the transformation of a Pt6L4 octahedral coordination cage (68) into a COC (69) through reductive elimination (Fig. 13b).137
With this incredibly wide range of available synthetic strategies and reactions, COCs offer some of the highest levels of structural diversity of molecular capsules. The caveat to this is that the irreversibility of reactions can result in much reduced yields compared to alternative systems. Regardless, the versatility of COCs means they can be designed to be rigid or relatively flexible, of various sizes and geometries. They can also be rendered soluble in various media,138 and porosity in the solid-state can be tailored through substituents.139 In terms of characterising and studying POCs, solid-state analysis by SCXRD is common, as is the use of NMR and MS in solution.
The structural versatility available to POCs, both dynamic and kinetically stable, enables their design for binding a wide range of guests in solution, including anions140 (of particular note, cage 56 exhibited attomolar affinity (Ka ∼1017 M−1) for Cl−,122 and a urea-based cryptand bound SO42− in 100% water)141 and cations142 as well as neutral molecules such as saccharides,143 small aromatics,144 polycyclic aromatic hydrocarbons116d,118 and fullerenes.145 Such host–guest chemistry can be exploited for sensing and separations,146 and the cavity space can be engineered to possess endohedral catalytic sites.147 In the solid-state, many POCs have been explored as porous materials for applications involving the uptake and separation of gases.99 As such, POCs are an incredibly versatile class of molecular capsules that lend themselves to applications as both solution- and solid-phase materials.
Hydrogen, halogen and chalcogen bonding capsules
One of the most commonly encountered non-covalent interactions is the hydrogen bond (HB; Fig. 14). Ubiquitous in nature, the relatively weak nature of HBs (typically <40 kJ mol−1 although estimates vary148) is both a gift and a curse. The low energy barrier to rearrangement allows funnelling of self-assembled mixtures down to their thermodynamic minimum with relative ease. The susceptibility of the HB to unwanted disruption, however, limits the stability of such systems, and makes their design a significant challenge.
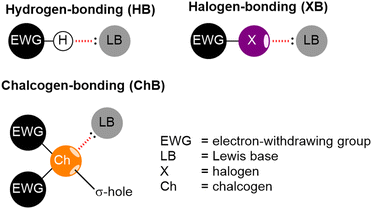 |
| Fig. 14 Cartoon representations of hydrogen-, halogen- and chalcogen-bonding electrostatic interactions. | |
The simplest form of HB capsules would therefore arise from homomeric dimerisation of self-complementary building blocks (Fig. 15). In 1993, Rebek and co-workers introduced curved glycouril-based monomer 70 that was shown to self-assemble into a dimeric capsule in chloroform, forming a molecular tennis-ball held together by a seam of 8 HBs between the glcouril units.149 Encapsulation of small molecule guests (chloroform, dichloromethane, methane, ethylene) was demonstrated by NMR, with slow exchange evidenced by observed signals for both the empty capsule and host–guest adduct.150 Since this seminal work, other examples of hydrogen-bonded dimers have been reported from interactions between various functional groups (Fig. 15), such as peptides,151 oximes,152 amides,153 ureas (e.g.71),154 and resorcinarenes (e.g.72, 73).155,156 For each of these, self-assembly in chloroform proceeded readily, whilst addition of competing solvents (methanol, DMSO) served to disrupt the HB interactions between monomers.
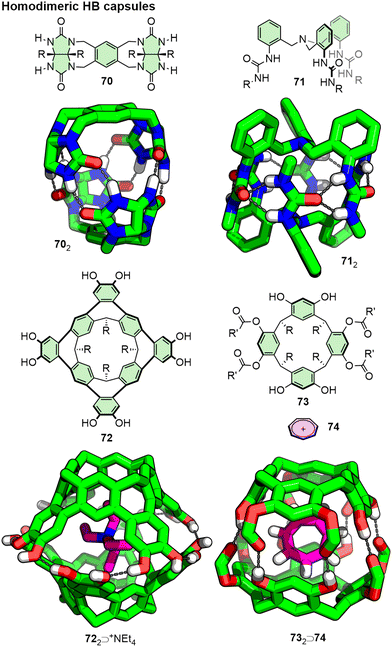 |
| Fig. 15 Homodimeric capsules formed through HB interactions between self-complementary units. R groups omitted from SCXRD structures for clarity. | |
Although apparently forming without an explicit template, subsequent studies suggested that appropriately-sized solvent molecules could take on this role. An extended version of Rebek's glycouril monomer, for example, dimerised in benzene, but only did so in p-xylene in the presence of a suitable guest.157 Likewise, resorcinarene-based cavitands have been shown to cleanly dimerise in chloroform, benzene or toluene, as these were small enough to readily fit within the cavity of the host, whilst bulkier mesitylene as solvent required addition of suitable guests to template the assembly.158 Resocinarene tetraester 73, for example, existed in monomer form in chloroform, but assembled into a dimeric host in the presence of a tropylium cation (74) template.155 Such host–guest interactions can be incredibly powerful in imparting stability to HB capsules: resorcinarene 72 did not spontaneously dimerise in acetone but could be induced to do so upon addition of +NEt4 as a suitable template.155
Larger homomeric HB assemblies beyond dimers have also been investigated. Tetramers,159 hexamers and octamers160 have all been realised, with a large hexameric assembly 2.3 nm in diameter and a cavity volume of 2800 Å3 recently reported.161 Seminally, MacGillivray and Atwood reported the solid-state structure of a hexamer formed from the self-assembly of a resorcinarene with 8 molecules of water through a concerted network of 60 hydrogen bonds.162 The solution-phase persistence of this structure, and its ability to encapsulate guests, in water-saturated chloroform, was subsequently demonstrated.163
In addition to these homomeric systems, heteromeric assemblies have been reported from interactions between complementary building blocks (Fig. 16). Combining resorcinarenes functionalised with HB donors, such as carboxylic acids (e.g.77) and alcohols (e.g.76), and acceptors such as pyridines (e.g.75), has been shown to lead to the formation of heterodimeric capsules (e.g.75.76).164 Related architectures assembled from quite different building blocks, such as acid-functionalised resorcinarenes and tetrapyridyl porphyrins (including double-cavity systems) have also been reported.165 Charge-assisted HBs have been exploited to prepare heterodimeric capsules that have demonstrated enhanced stability in polar media including methanol and water.166
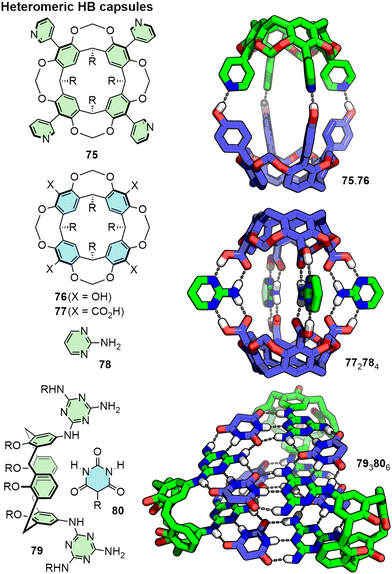 |
| Fig. 16 Heteromeric capsules formed through HB interactions between complementary building blocks. R groups omitted from SCXRD structures for clarity. | |
Through careful design, multi-component heteromeric HB architectures have also been realised. [4+2]-type assemblies have been reported from cavitands assembled with complementary struts (e.g.772784),167 whilst a [6+3] architecture was shown to assemble from functionalised calixarene 79 and barbituric acid derivative 80.168
In summary, supramolecular capsules assembled through cooperative hydrogen bonds between subcomponents can be realised of varying topology, including both homomeric and heteromeric architectures. Perhaps unsurprisingly, these assemblies tend to only be stable in apolar, aprotic media, although there are exceptions.169 Systems held together by charge-assisted HBs, however, have demonstrated enhanced stability in highly competitive solvents, as have hosts that are additionally stabilised by interactions with guest molecules. A wide range of structures for the monomer units have been reported, in addition to the commonly explored calixarene and resorcinarene cavitands, with various HB units that are capable of interacting with themselves or complementary motifs.
Under suitable conditions, HB capsules have been shown to be capable of encapsulating a variety of guest species, both neutral and charged, and to exhibit guest selectivity.158 Such host–guest chemistry has been exploited for applications in catalysis,170 molecular separations,171 and stabilisation of reactive species.172
Conceptually similar to HBs, electrostatic interactions arising from the donation of electron density into electropositive regions, labelled σ-holes, on halogen and chalcogen atoms have been termed halogen bonds (XBs) and chalcogen bonds (ChBs), respectively (Fig. 14). Whilst less well studied than their HB congeners, XBs and ChBs have been investigated as alternatives non-covalent interactions in supramolecular chemistry, including for self-assembled architectures.
Heteromeric XB capsules have been reported from the self-assembly of suitably functionalised cavitands (Fig. 17a). Aakeröy and co-workers observed such a structure in the solid-state from interactions between a calixarene macrocycle with four iodotetrafluorobenzene arms and a tetrapyridyl resorcinarene.173 Subsequently, Diederich and co-workers demonstrated the persistence of related architectures (81.82) in solution by NMR and were able to determine an association constant between the two components. Critically, small quantities of an alcohol were required to stabilise the structures through hydrogen-bonding interactions between the arms of the resorcinarene-based cavitands. The ability of these XB capsules to bind small guest molecules both in the solid state and in solution was also demonstrated.174
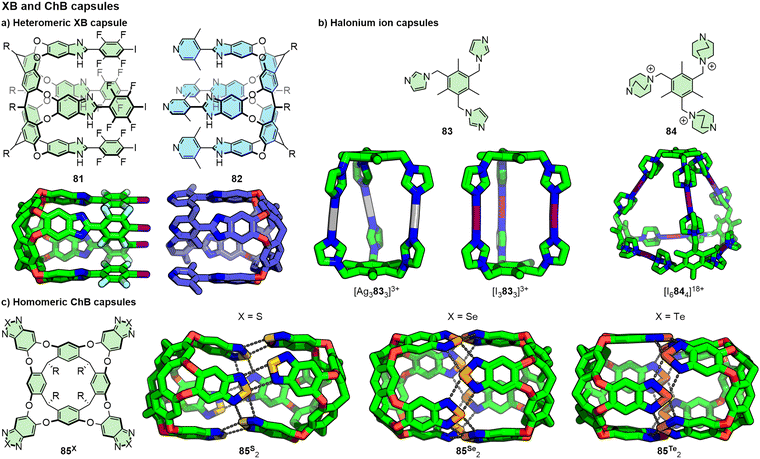 |
| Fig. 17 Halogen- and chalcogen-bond interactions, including with halonium ions, can be used to assemble both homo- and heteromeric capsules. R groups omitted from SCXRD structures for clarity. | |
As an alternative design strategy, homomeric systems have also been reported in which XB acceptor-functionalised cavitands are assembled through interactions with iodonium ions (I+), generally prepared via a pre-organised Ag(I) metallo-capsule intermediate (Fig. 17b). In this way, the halonium ions act as analogues of metal ions in coordination cage structures. Using this approach, Rissanen and co-workers have reported dimeric I3L2175 and I4L2 assemblies,176 I6L4 tetrahedra,177 and a 4 nm I12L6 octahedron.178 As with MOCs, subtle changes to the ligand structure can have a significant impact on the resultant assembly: whilst ligand 83 with imidazole donor units yielded an I3L2 prismatic cage, DABCO ligand 84 formed an I6L4 tetrahedron.177 Aside from characterisation by SCXRD in the solid-state and by MS, the persistence of these species in solution has been demonstrated by NMR and DOSY. Typically, these experiments have been run in low polarity solvents such as dichloromethane or chloroform, although stability in acetonitrile has also been demonstrated.177
Dimeric capsules assembled through chalcogen-bonding interactions have also been reported (Fig. 17c). By incorporating benzotelluradiazole and benzothiadiazole moieties into resorcinarene cavitands, Diederich and co-workers showed that these were capable of self-assembly into [1+1] capsules in solution and the solid-state. Crucially, for the capsule dimers to form, the cavitands were required to adopt a vase conformation. The significantly stronger ChB interactions with tellurium resulted in persistence of 85Te2 across a range of solvents (dichloromethane, benzene and THF) and concentrations (down to 40 μM in THF). In contrast, 85S2 only formed in benzene and THF, resulting from complementary binding of solvent molecules within the cavitand cavity favouring the vase form, whilst in dichloromethane 85S adopted a kite conformation, preventing capsule formation.179 Rebek, Yu and co-workers investigated related benzoselenadiazole systems with water-solubilising substituents attached to the lower rim. Although by itself cavitand 85Se adopted a kite conformation in D2O, addition of suitable guests induced formation of [1+1] capsules templated through incorporating one (e.g. n-nonane) or two (e.g. n-pentane, cyclohexane) guest molecules.180
HB, XB and ChB capsules can be studied in solution by NMR techniques, including DOSY. Of special note, self-assembly of the XB capsules reported by Diederich and co-workers (81.82), that incorporated perfluorinated iodophenyl XB donors, could be followed by 19F NMR, and close spatial proximity of the acceptor and donor cavitands evidenced by 1H,19F HOESY experiments.174 MS is also commonly employed, as is SCXRD in the solid-state, although the relatively weak nature of some HB/XB/ChB interactions can make successful crystallisation of these capsules difficult.
The study of capsules assembled through XB and ChB interactions is still in its infancy, with the first example of a solution-persistent capsule only reported within the last decade. Similarly, preliminary results have shown these systems to be capable of binding guest molecules, although exploitation of this for applications remains very much underexplored. There has been one report, however, of a ChB capsule demonstrating selective encapsulation of oxime isomers and their isomerisation under confinement.181
To date, systems assembled from donor- and acceptor-functionalised build blocks have been reported solely from suitably functionalised cavitands. Despite this limited structural diversity, there is nothing inherent to the design of these capsules that suggests alternative building blocks could not be used. In contrast, those assembled using halonium ions have been demonstrated with a range of ligands of different geometries and denticities. The potential to exhibit stability in various solvents and across a range of concentrations has also been demonstrated, although less robust systems remain predominant. As such, XB and ChB capsules182 at this nascent stage of their study show promise as robust supramolecular architectures.
π–π capsules
π–π, or π–stacking, interactions are relatively weak and poorly directional in comparison to other non-covalent interactions such as dative covalent and hydrogen bonds. This helps to explain the relative paucity of capsules assembled using π–π interactions, as it can be difficult to design systems for controlled self-assembly. Due to their relatively weak nature, the directing effects of π–π interactions are often assisted by solvophobic forces that act as significant drivers of the self-assembly process. In this section we will consider systems that assemble in organic solvents, whilst assemblies that form in aqueous media (i.e. driven specifically by hydrophobic forces) will be discussed in the subsequent section.
Cram and co-workers reported the synthesis of resorcinarene-derived macrocyclic cavitands with extended aromatic units. These were able to adopt conformations referred to as vase,183 in which the aryl arms are axial, i.e. perpendicular to the plane of the resorcinarene base, and kite, in which the arms splay outwards in an equatorial fashion (Fig. 18). By introducing substituents (e.g. methyl group) onto the 2-position of the resorcinol components (X-substituent of 86 in Fig. 18), the structures could be locked into the kite conformation.184 The kite conformers provided a suitable π-surface for dimerisation, observed in the solid-state by SCXRD analysis,185 and in solution by vapour pressure osmometry and NMR in CDCl3 (e.g. evidenced by shielding of methyl groups directed internally in the dimer).186 Such capsule-type dimers were termed velcraplexes.
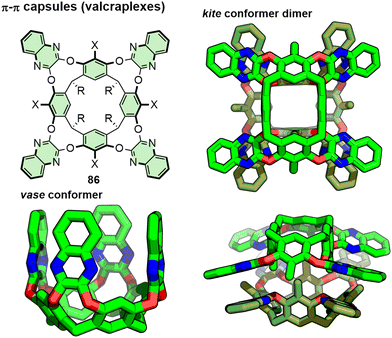 |
| Fig. 18 Cavitands can adopt vase conformations in which the arms are orthogonal to the base, or a splayed kite conformation that allows dimerisation through π–π interactions. R groups omitted from SCXRD structures for clarity. | |
In addition to homodimers, heterodimers were observed to form in some instances from mixtures of cavitands, including when one of the components did not dimerise with itself. Narcissistic self-sorting behaviour of some cavitands was also observed, in which only homodimers self-assembled from mixtures of cavitands.187
Cram observed that dimer formation was highly dependent on both temperature and concentration. The nature of the solvent was also important, with more polar solvents (e.g. acetonitrile, methanol) promoting cavitand dimerisation through solvophobic effects.186b,187 A similar observation was made by Paek and co-workers, who determined the association constant for one homodimer in CDCl3 to be 4.9 × 10−4; increasing the solvent polarity resulted in enhancement of the association constant by two orders of magnitude in 10% CD3OD.185
Within these systems, structural modifications to the cavitand could have profound effects on their self-assembly. Whilst larger resorcinol substituents, e.g. ethyl groups, still promoted the kite conformer, they prevented homodimerisation through steric hindrance.184 Dalcanale and co-workers observed that tethering groups on the lower rim of the cavitand also impeded dimerisation through restricted conformational flexibility.188
Due to the difficulty of their design and fragility with respect to concentration, temperature and solvent, the host–guest chemistry of velcraplexes have not been studied. Although they have been investigated as supramolecular junctions within polymers,189 their use remains extremely limited.
Hydrophobic capsules
Within the context of this discussion, the hydrophobic effect190 is (very) broadly defined as the propensity for apolar moieties to aggregate in aqueous solution due to the sum of interactions between solvent and solute being less energetically favourable than interactions between water molecules. This allows relatively weak, and often ill-defined and poorly directional, non-covalent interactions (van der Waals forces, π–π interactions) to exist between the apolar moieties that might be ineffective at directing any semblance of molecular ordering in alternative solvents. Thus, while polar solvents can be detrimental to the stability of many other supramolecular capsules due to competing interactions between the components and solvent molecules, exploiting the hydrophobic effect can lead to the formation of capsules in one of the most competitive solvents – water (Fig. 19).
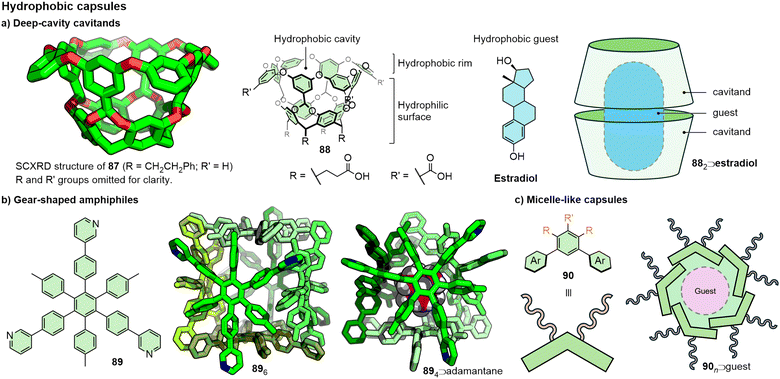 |
| Fig. 19 Hydrophobic capsules assemble from components that contain both hydrophobic moieties, that are forced to interact with themselves via relatively weak and poorly directional interactions, and hydrophilic units capable of externally interacting with water molecules to solubilise the assemblies. | |
Gibb and co-workers have extensively investigated so-called “deep-cavity” cavitands,191 such as 87 and 88, the latter of which consists of a hydrophilic surface functionalised with carboxylic acid groups, and a hydrophobic cavity, the entrance to which is surrounded by a hydrophobic rim (Fig. 19a). By itself, the cavitand was shown to exist in monomeric form in basic aqueous solution; addition of a suitable hydrophobic template (e.g. estradiol), however, led to formation of a dimeric capsule.192 Depending on the size of the guest, one (e.g. estradiol) or two (e.g. butane, naphthalene)193 molecules might be encapsulated within the host dimer. Whilst remarkably stable in water at various concentrations (suggesting a minimum association constant of 108 M−1),192 addition of other solvents (acetonitrile, THF, acetone, DMSO) led to capsule degradation to varying extents.194 Successful deposition of capsule host–guest complexes on silica surfaces has been demonstrated, with retention of the capsular structure.195 Cationic analogues of these have been reported by replacing the carboxylic acid moieties with amine/ammonium functionalities, enabling capsule formation in acidic media.196 Through small structural modifications and choice of guest template, higher-order assemblies including tetramers and hexamers have been reported,197 as have heteromeric assemblies198 and externally functionalised systems.199
Hiraoka, Shionoya and co-workers reported the self-assembly of a remarkable hexameric cube from the gear-shaped monomer, 89, in a water/methanol solvent mixture (Fig. 19b). The solid-state SCXRD structure showed the hexamer to apparently be held together by van der Waals, π–π and CH–π interactions; the delicate balance of these interactions was demonstrated by comparison with an analogous monomer in which the tolyl substituents were replaced with phenyl units, which did not form an analogous assembly.200 Whilst this hexameric structure was able to encapsulate appropriately sized substituted benzenes, adamantane induced a rearrangement of the host to give a tetrameric architecture (894, Fig. 19b).201 Methylation of two of the pyridine rings to cationic pyridinium moieties gave a fully water-soluble monomer that, in the absence of a template, assembled into a hexameric cube,202 the thermal stability of which could be modified by tailoring the electronics and sterics of the non-pyridinium arm of the monomer.203
Detailed reviews on well-defined capsules assembled using the hydrophobic effect are available for perusal.204 For these systems, the precise number of component building blocks incorporated into the self-assembled structures can be determined. For others, there can be a distribution of compositions. Yoshizawa and co-workers have extensively studied micelle-like structures assembled from small-molecule amphiphiles incorporating hydrophobic aromatic shells (Fig. 19c).205 Amphiphiles with the general structure of 90 incorporating anthracene,206 naphthalene, phenanthrene,207 phenothazine208 and even aliphatic adamantane209 have been investigated. NMR spectroscopy was used to confirm the formation of aggregates, whilst dynamic light scattering (DLS) and atomic force microscopy (AFM) reveal the approximate size and narrow size distribution of the assemblies. A U-shaped amphiphile was shown to assemble into a dimeric capsule,210 whilst an anthracene-based amphiphile formed capsules composed of 4-6 sub-components,206 and an adamantly-functionalised monomer formed a larger assembly with approximately 16 sub-components,209 demonstrating that, through structural design, some control over the aggregate size is possible, although formation of different sized aggregates through encapsulation of smaller or larger guests has been observed.209
Given the inherently low directionality of relatively weak forces that contribute to self-assembly under hydrophobic conditions, the rational design of hydrophobically-driven assemblies is somewhat challenging. Structural factors common to the different systems discussed herein include hydrophilic solubilising groups and accessible hydrophobic surfaces that can aggregate together in aqueous media. 1H NMR, NOESY, DOSY and MS have all been used to demonstrate the persistence of structures self-assembled by the hydrophobic effect in solution, with some structures able to be confirmed in the solid-state by SCXRD. Other analytical techniques that have been used include dynamic light scattering (DLS) and atomic force microscopy (AFM), which can also reveal size-distribution of non-uniform assemblies. Although often limited to the binding of guests that are themselves hydrophobic, this class of capsules has demonstrated utility in a number of areas, including as molecular reaction flasks,211 in molecular separations,193a modulation of photophysical/photochemical properties,212 and solubilisation of hydrophobic species.192,206
Mechanically interlocked molecules and foldamers
Although not traditionally considered as molecular capsules per se, mechanically interlocked molecules (MIMs) – species held together through mechanical entanglement of molecular components rather than covalent bonds – and foldamers – oligomers that adopt a defined folded conformation through intramolecular interactions – can possess confined cavities described by the three-dimensional structure of the molecules. For completeness, we include brief discussions of each of these host architectures. Where MIMs are concerned, the discussion will be split into two parts covering (i) the interlocking of components that are capsules in their own right, resulting in the formation of new cavity spaces distinct from the constituents, and (ii) MIMs in which the components are acyclic or macrocyclic, therefore not considered capsules individually, but through their interlocking generate confined spaces.
Interpenetrated capsules
The same non-covalent forces that drive the encapsulation of guests within hosts can also result in interactions between capsules which can give rise to interpenetrated systems (Fig. 20).213 For supramolecular capsules, interlocking can arise spontaneously as a thermodynamic process resulting from interactions ostensibly either directly between cage components or with a template. Kinetically robust interpenetrated capsules have also been prepared through reaction of suitably templated precursors.214
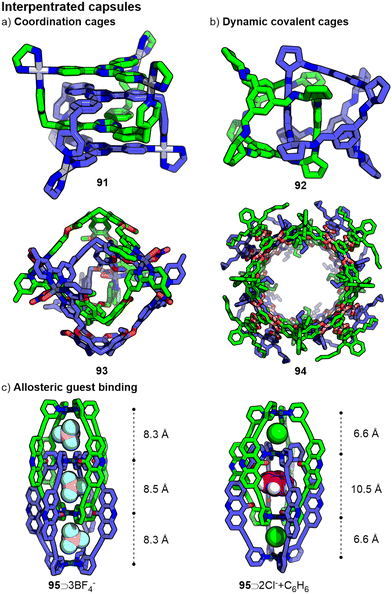 |
| Fig. 20 Examples of metal–organic and covalent interpenetrated cage structures have been reported, some of which retain appreciable cavity space. These systems have the potential to exhibit interesting host–guest chemistry such as allostery. | |
In 1999, Fujita and co-workers reported the spontaneous interlocking of two trinuclear coordination cages in water, driven by π–π interactions between the ligands (91).215 Due to the tight packing between the cage components, any cavity space was occluded, preventing further guest binding. Subsequently, additional examples of interpenetrated coordination216 (Fig. 20a) and dynamic covalent217 cages (Fig. 20b) have been reported via “self-templation”, although accessible cavity space is often severely reduced, limiting potential host–guest chemistry. Exceptions to this include Hardie's catenanted coordination cage (93) based on cyclotriveratrylene ligands218 and Mastalerz's giant dynamic covalent cages (94).219 Perhaps unsurprisingly, the choice of solvent can have a significant impact on the formation of such interpenetrated species.219b
In 2008 Kuroda reported a Pd2L4 coordination cage that, in the presence of suitably sized anions acting as templates, could form a quadruply-interpenetrated catenane.220 Due to the difference between the external cavities and the central one, it was shown to be possible to encapsulate both BF4− and NO3− anions in a site-selective manner.221 Subsequent work on related systems (e.g.95) has shown that guest binding can cause the interlocked cages to move relative to each other, altering the sizes of the cavities and affecting their host–guest chemistry, akin to allosteric binding (Fig. 20c).222
Despite the potentially limited cavity space offered by interpenetrated capsules, there have been reports of these systems being used for molecular recognition223 and separations.224 The targeted synthesis of such interlocked architectures, however, remains a significant challenge, limiting their rational design and use as molecular capsules.
Mechanically interlocked molecules
The two most common classes of MIMs are catenanes (consisting of interlocked macrocycles)225 and rotaxanes (consisting of macrocyclic and dumbbell-shaped components).226 The entanglement and relative orientation of the components in MIMs creates a three-dimensional cavity space between them, enabling encapsulation of guest species (Fig. 21a). The mechanical bond has been exploited to tailor environments for the interlocked components themselves, essentially acting as kinetically stable host–guest assemblies. In this manner, mechanically-enforced proximity of components can alter the reactivity227 of, or interactions228 between, functional units. These effects of the mechanical bond have been investigated for applications in caging the activity of biomolecules,229 catalysts230 and cytotoxic agents,231 just to name a few. The discussion in this review, however, will be limited to MIMs as hosts for guest ions/molecules that are distinct from the interlocked units.
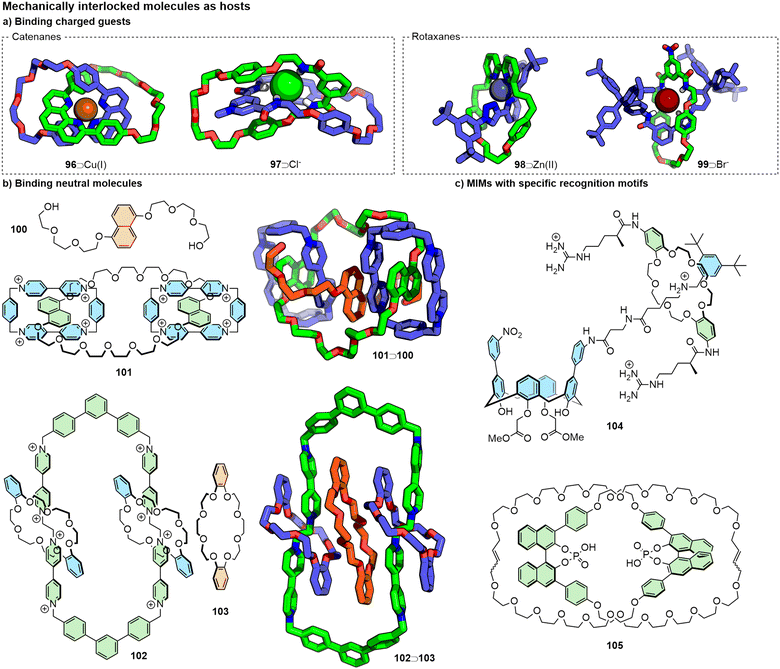 |
| Fig. 21 MIMs are well suited to the binding of small, charged guests such as Cu(I), Cl− and Br− due to the mechanically-enforced proximity of interacting motifs. Less well explored is the binding of neutral guest molecules through interactions between the mechanically interlocked components. | |
Even just within catenanes and rotaxanes there is scope for a huge amount of structural variation dependent upon the number of components, the manner in which they are interlocked, and their chemical structure. The literature on MIMs is vast; reviews detailing synthetic strategies based on anions and metal cations are indicated in the relevant sections below, with alternative template motifs covered in other reviews.232
Perhaps unsurprisingly, given their critical role in the development of high-yielding synthetic methods to access MIMs,233 the binding of transition metal ions has been explored extensively (Fig. 21a).234 The mechanically-enforced proximity of coordinating units on sub-components induces a “mechanical chelate” effect (originally termed the catenand effect by Sauvage).235 In this manner, MIM ligands have been used to stabilise oxidation states or enforce unusual coordination environments of metal ions236 and for selective detection.237
Like transition metal ions, anions have been used to template the formation of MIMs.238 As such, various interlocked molecules have been engineered to possess units capable of interacting with anions (Fig. 21a)239 through non-covalent interactions. In this manner, MIMs have been synthesised that demonstrate binding of various anions, including preferences between halides,240 selective binding of polyatomic anions such as NO3−,241 SO42−,242 and encapsulation in aqueous media.243
Whilst most commonly employed for the binding of charged species, encapsulation of small neutral molecules by MIMs has been reported, albeit often serendipitously (Fig. 21b). Stoddart and co-workers have developed stepwise syntheses of both oligo[n]catenanes and rotacatenanes that proceed through the (transient) formation of a pseudorotacatenane, i.e. an unstoppered axle component bound between the components of a catenane via π–π and hydrogen bonding interactions, the solid-state structure of one of which (101⊃100) was reported.244 Related to this work, the authors were able to generate a redox-active [2]catenane host in which guests could bind within different pockets between the constituent components.245 Loeb and co-workers reported the binding of a dibenzo-24-crown-8 macrocycle between the components of a [3]catenane through π–π and CH–π interactions (102⊃103).246
It is possible to specifically engineer recognition components within the sub-components of MIMs for binding to small-molecule guests (Fig. 21c). Smithrud and co-workers, for example, have designed rotaxane hosts incorporating macrocyclic recognition units into the axle components, with additional host–guest interactions from the macrocyclic components (e.g.104 in Fig. 21c), for the intracellular delivery of various guests.247 Niemeyer and co-workers synthesised [2]catenane 105 incorporating chiral BINOL-phosphate units within each of the macrocycles and demonstrated its ability to bind diamine guests in DMSO, observing stereoselective binding of chiral guests;248 Beer and co-workers showed binding of dicarboxylates within a [3]rotaxane, also reporting chiral discrimination.249 A [3]rotaxane with porphyrin units attached to the two macrocycles was shown by Morin and co-workers to bind fullerenes in a tweezer-like fashion.250
The ability for MIM sub-components to move relative to each other make such cavity spaces highly flexible, particularly for systems large enough to encapsulate polyatomic molecules. Although this could potentially be exploited for adaptive host systems, uncontrolled dynamics might explain the relative paucity of examples reported of binding molecules larger than simple cations and anions.
The diversity of MIM structures that can be accessed make them one of the most tuneable classes of host systems; the type, number and manner of interlocking of the components are all variable, as are their covalent structures, allowing the installation of myriad recognition and solubilising groups. Anion-binding MIMs, for example, have been prepared with recognition groups that interact via hydrogen bonds, halogen bonds, chalcogen bonds and can be charged or neutral.251 Furthermore, so-called “impossible” MIMs have been reported that lack specific binding motifs.252 External functionalisation of MIMs has also allowed their incorporation into polymeric materials, resulting in soft matter with switchable properties dependent on the binding of a guest within the MIM cavity.253 Due to their unusual topology, MIMs have found wide-ranging use for various applications. Specifically related to their ability to bind guest species with the endohedral cavity defined by the intersection of their components, MIMs have been used for sensing,254 transport255 and catalysis.256
MIMs are usually readily characterised using standard techniques for covalent molecular systems: NMR and MS in solution, with NOESY often being useful for identifying co-conformations of the interlocked components, and SCXRD in the solid-state, all of which can also be used to probe host–guest interactions. Mechanical linking of the components means that covalent bonds must be broken to separate them, making MIMs highly robust. As hosts of small, often charged, species such as cations and anions, MIMs are incredibly versatile hosts that can be engineered with high precision. Although the potential for multiple co-conformations has been exploited in the design and synthesis of molecular switches and machines,257 with more complex systems, and for binding of larger guest molecules, this flexibility may be detrimental to strong and selective recognition events.
Foldamers
Foldamers are abiotic oligomers that adopt well-defined folded conformations driven by interactions between components of their primary structure.258 Although foldamers can be very compact, precluding their use as molecular capsules, it is possible to design systems with appreciable cavity spaces, described by the folded conformation of their scaffolds, that can be used to encapsules guests.259
Various monomeric units have been used to construct foldamer scaffolds (Fig. 22a), including peptidomimetics,260 phenylacetylenes261 (e.g.106),262 aromatic amides263 (e.g.108),264 ureas265 (e.g.109)266 and triazoles267 (e.g.107).268 This diversity of building block types and monomer structures, and the quantities and sequences in which they can be combined, allows a wide range of structures to be accessed. Although a detailed discussion of their synthesis will not be included here, we note that solid phase synthetic methods have been applied to a range of foldamer structures,269 allowing automation of what can otherwise be a very tedious process.
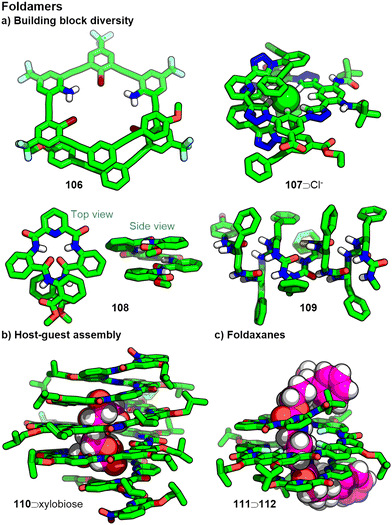 |
| Fig. 22 Foldamers adopt well-defined conformations arising from interactions between units within their scaffold. These can create well-defined cavity spaces that can be used to encapsulate guest species. | |
As the conformation of foldamers is directed by non-covalent interactions such as hydrogen bonding, π–π stacking and solvophobic forces, it is unsurprising that solvents can dramatically affect the conformational state of oligomers;270 the incorporation of additional supramolecular interactions along the backbone has been shown to aid stabilisation of folded conformations.271 The impact of binding anions272 and cations273 on the conformation of foldamers is also well known.
The well-defined folded structure of foldamers allows them to possess a three-dimensional cavity space capable of encapsulating guest species; the binding of solvent/small organic molecules,274 hydrocarbons,275 and saccharides (Fig. 22b)276 have all been reported. Additionally, the helical nature of many foldamers has been exploited in the stereoselective binding of chiral guests.277 A report on the selective binding of a heteromeric pair of saccharides demonstrates the potential for cooperative binding of multiple guests.278 The term foldaxane has been coined to describe assemblies formed from linear, dumbbell-shaped molecules (akin to the axles of rotaxanes) encapsulated within foldamers with the bulky terminal groups located outside of the helical structure (Fig. 22c).279
Although the primary structures of foldamers, being generally covalent, are robust, their folded nature that gives rise to a defined cavity is highly susceptible to external conditions, such as solvent and temperature. While foldamers in general are of interest for their (potential) biomedical280 and catalytic applications,281 their use as capsules has been exploited for selective molecular recognition,282 sensing,283 catalysis,284 separations285 and controlling the second coordination sphere of metal complexes.286
Conclusions
The study of molecular capsules, capable of encapsulating guest species, has grown exponentially since the early work of Pedersen and Lehn. Stemming from these seminal contributions, research in this area has been pushed forward dramatically in terms of both the design of capsules and demonstration of their function, particularly related to chemistry within the confined spaces of their internal cavities. In the design of capsule systems themselves, molecular engineering strategies have been developed to prepare architectures across scale lengths, that are robust, structurally sophisticated, and that incorporate functional units to change physical and chemical properties. In terms of function, the effects of encapsulation on the properties of guest species have been shown to stabilise reactive species or promote reactivity in benign molecules, and to allow their selective recognition or separation from mixtures.
Combined with advances in computational chemistry to aid in their design,287 the chemistry of capsules is moving away from serendipitous discoveries towards the targeted design of specific structures as solutions to scientific challenges. Some of these are clearly pertinent for extant problems, such as the highly selective recognition of glucose128 and extraction of hydrophilic anions from water.122 Others push the boundaries of what can be achieved from a purely fundamental, curiosity-driven perspective, such as the generation of an antiaromatic confined space.288
The breadth of architectures that fall under the umbrella-term capsule is enormous, something that has hopefully been demonstrated in this article. As such, when searching for a class of capsule to achieve a certain task, researchers can be guided by system requirements in terms of stability, chemical compatibility, flexibility etc. Another key consideration is often synthetic accessibility, for which there is usually a trade-off with (i) stability and (ii) structural complexity. Structures formed under thermodynamic control can be assembled from simple building blocks in high yields but are often susceptible to degrading reactions, whilst the synthesis of reduced-symmetry systems and the installation of functional groups can be non-trivial. The essentially infinite diversity of (supra-)molecular capsule structures, however, allows researchers to design systems very specifically to their circumstances.
The study of capsules and their functions is still in a period of maturation. Multiple spectacular examples in the literature have shown what can be achieved within the confined environments of capsule cavities. Building on these, and inspired by nature's use of confinement effects to achieve astounding recognition and reaction selectivity, combined with advances in molecular design, we anticipate that increasingly sophisticated structures and behaviours of capsule systems will emerge over the coming years. Based on current trends, we are sure that (supra-)molecular capsules will become invaluable tools in the development of advanced catalysts, sensors and artificial systems/networks.
Author contributions
All authors contributed to the writing of the manuscript.
Data availability
No primary research results, software or code have been included and no new data were generated or analysed as part of this review.
Conflicts of interest
There are no conflicts of interest to declare.
Acknowledgements
This work was supported by the Royal Society (URF\R1\221740 and RF\ERE\221016) and the University of Birmingham. JEML is a Royal Society University Research Fellow.
Notes and references
-
(a) C. J. Pedersen, J. Am. Chem. Soc., 1967, 89, 2495–2496 CrossRef CAS;
(b) C. J. Pedersen, J. Am. Chem. Soc., 1967, 89, 7017–7036 CrossRef CAS . SCXRD structure of K+ complex of 18-crown-6 from: ;
(c) P. Seiler, M. Dobler and J. D. Dunitz, Acta Crystallogr., Sect. B: Struct. Crystallogr. Cryst. Chem., 1974, 30, 2744–2745 CrossRef.
- C. J. Pedersen, Science, 1988, 241, 536–540 CrossRef CAS PubMed.
-
(a) B. Dietrich, J.-M. Lehn and J.-P. Sauvage, Tetrahedon Lett., 1969, 10, 2885–2888 CrossRef;
(b) B. Dietrich, J.-M. Lehn and J.-P. Sauvage, Tetrahedon Lett., 1969, 10, 2889–2892 CrossRef . SCXRD structure of K+ complex of [2.2.2]cryptand from: ;
(c) R. Alberto, K. Ortner, N. Wheatley, R. Schibli and A. P. Schubiger, J. Am. Chem. Soc., 2001, 123, 3135–3136 CrossRef CAS PubMed.
- C. J. Pedersen, Angew. Chem., Int. Ed. Engl., 1988, 27, 1021–1027 CrossRef.
- J.-M. Lehn, Angew. Chem., Int. Ed. Engl., 1988, 27, 89–112 CrossRef.
- D. J. Cram, Angew. Chem., Int. Ed. Engl., 1988, 27, 1009–1020 CrossRef.
- D. J. Cram and J. M. Cram, Science, 1974, 183, 803–809 CrossRef CAS PubMed.
-
(a) F. Hof, S. L. Craig, C. Nuckolls and J. Rebek, Angew. Chem., Int. Ed., 2002, 41, 1488–1508 CrossRef CAS;
(b) J. Rebek, Angew. Chem., Int. Ed., 2005, 44, 2068–2078 CrossRef CAS PubMed.
- W. Liu and J. F. Stoddart, Chem, 2021, 7, 919–947 CAS.
-
(a) J. Rebek, Acc. Chem. Res., 2009, 42, 1660–1668 CrossRef CAS PubMed;
(b) B. Breiner, J. K. Clegg and J. R. Nitschke, Chem. Sci., 2011, 2, 51–56 RSC;
(c) A. B. Grommet, M. Feller and R. Klajn, Nat. Nanotechnol., 2020, 15, 256–271 CrossRef CAS PubMed.
- A. Galan and P. Ballester, Chem. Soc. Rev., 2016, 45, 1720–1737 RSC.
-
(a) M. Yoshizawa, J. K. Klosterman and M. Fujita, Angew. Chem., Int. Ed., 2009, 48, 3418–3438 CrossRef CAS PubMed;
(b) L. Catti, Q. Zhang and K. Tiefenbacher, Chem. – Eur. J., 2016, 22, 9060–9066 CrossRef CAS PubMed;
(c) C. Gaeta, P. La Manna, M. De Rosa, A. Soriente, C. Talotta and P. Neri, ChemCatChem, 2021, 13, 1638–1658 CrossRef CAS;
(d) G. Olivio, G. Capocasa, D. Del Giudice, O. Lanzalunga and S. Di Stefano, Chem. Soc. Rev., 2021, 50, 7681–7724 RSC.
-
(a) H.-J. Schneider, Angew. Chem., Int. Ed. Engl., 1991, 30, 1417–1436 CrossRef;
(b) S. Sarkar, P. Ballester, M. Spektor and E. A. Kataev, Angew. Chem., Int. Ed., 2023, 62, e202214705 CrossRef CAS PubMed.
-
(a) D. J. Cram, S. Karbach, Y. H. Kim, L. Baczynskyj and G. W. Kalleymeyn, J. Am. Chem. Soc., 1985, 107, 2575–2576 CrossRef CAS;
(b) A. Jasat and J. C. Sherman, Chem. Rev., 1999, 99, 931–968 CrossRef CAS PubMed.
- T. Tateishi, M. Yoshimura, S. Tokuda, F. Matsuda, D. Fujita and S. Furukawa, Coord. Chem. Rev., 2022, 467, 214612 CrossRef CAS.
- R. Krämer, J.-M. Lehn and A. Marquis-Rigault, Proc. Natl. Acad. Sci. U. S. A., 1993, 90, 5394–5398 CrossRef PubMed.
-
(a) K. Harris, D. Fujita and M. Fujita, Chem. Commun., 2013, 49, 6703–6712 RSC . SCXRD structures of Pd12L24, Pd24L48 and Pd48L96 cages taken from the following, respectively: ;
(b) M. Tominaga, K. Suzuki, M. Kawano, T. Kusukawa, T. Ozeki, S. Sakamoto, K. Yamaguchi and M. Fujita, Angew. Chem., Int. Ed., 2004, 43, 5621–5625 CrossRef CAS PubMed;
(c) Q.-F. Sun, J. Iwasa, D. Ogawa, Y. Ishido, S. Sato, T. Ozeki, Y. Sei, K. Yamaguchi and M. Fujita, Science, 2010, 328, 1144–1147 CrossRef CAS PubMed;
(d) D. Fujita, Y. Ueda, S. Sato, N. Mizuno, T. Kumasaka and M. Fujita, Nature, 2016, 540, 563–566 CrossRef CAS PubMed.
- E. G. Percástegui, Eur. J. Inorg. Chem., 2021, 4425–4438 CrossRef.
- J. R. Farrell, C. A. Mirkin, I. A. Guzei, L. M. Liable-Sands and A. L. Rheingold, Angew. Chem., Int. Ed., 1998, 37, 465–467 CrossRef CAS PubMed.
- S. A. Cotton, C. R. Chimie, 2005, 8, 129–145 CrossRef CAS.
- M. Fujita, M. Tominaga, A. Hori and B. Therrien, Acc. Chem. Res., 2005, 38, 371–380 CrossRef PubMed.
- SCXRD structures of Pd(II), Zn(II) and Lu(III) M6L4 ocatahedra, respectively, from:
(a) M. Fujita, D. Oguro, M. Miyazawa, H. Oka, K. Yamaguchi and K. Ogura, Nature, 1995, 378, 469–471 CrossRef CAS;
(b) F. J. Rizzuto, W.-Y. Wu, T. K. Ronson and J. R. Nitschke, Angew. Chem., Int. Ed., 2016, 55, 7958–7962 CrossRef CAS PubMed;
(c) L.-P. Zhou, X.-S. Feng, S.-J. Hu and Q.-F. Sun, J. Am. Chem. Soc., 2023, 145, 17845–17855 CrossRef CAS PubMed.
- SCXRD structures of anionic, neutral and cationic tetrahedra, respectively, from:
(a) D. L. Caulder, R. E. Powers, T. N. Parac and K. N. Raymond, Angew. Chem., Int. Ed., 1998, 37, 1840–1843 CrossRef CAS;
(b) J. K. Clegg, F. Li, K. A. Jolliffe, G. V. Meehan and L. F. Lindoy, Chem. Commun., 2011, 47, 6042–6044 RSC;
(c) C. R. K. Glasson, G. V. Meehan, J. K. Clegg, L. F. Lindoy, P. Turner, M. B. Duriska and R. Willis, Chem. Commun., 2008, 1190–1192 RSC.
-
(a) M. Ikemi, T. Kikuchi, S. Matsumura, K. Shiba, S. Sato and M. Fujita, Chem. Sci., 2010, 1, 68–71 RSC;
(b) T. Kikuchi, S. Sato and M. Fujita, J. Am. Chem. Soc., 2010, 132, 15930–15932 CrossRef CAS PubMed;
(c) J. E. M. Lewis, A. B. S. Elliott, C. J. McAdam, K. C. Gordon and J. D. Crowley, Chem. Sci., 2014, 5, 1833–1843 RSC;
(d) G. A. Taggart, A. M. Antonio, G. R. Lorzing, G. P. A. Yap and E. D. Bloch, ACS Appl. Mater. Interfaces, 2020, 12, 14913–24919 CrossRef PubMed.
-
(a) M. Tominaga, K. Suzuki, T. Murase and M. Fujita, J. Am. Chem. Soc., 2005, 127, 11950–11951 CrossRef CAS PubMed;
(b) T. Murase, S. Sato and M. Fujita, Angew. Chem., Int. Ed., 2007, 46, 1083–1085 CrossRef CAS PubMed;
(c) K. Suzuki, M. Kawano, S. Sato and M. Fujita, J. Am. Chem. Soc., 2007, 129, 10652–10653 CrossRef CAS PubMed;
(d) M. Krick, J. Holstein, C. Würtele and G. H. Clever, Chem. Commun., 2016, 52, 10411–10414 RSC;
(e) Q.-Q. Wang, S. Gonell, S. H. A. M. Leenders, M. Dürr, I. Ivanović-Burmazović and J. N. H. Reek, Nat. Chem., 2016, 8, 225–230 CrossRef CAS PubMed;
(f) A. Platzek, S. Juber, C. Yurtseven, S. Hasegawa, L. Schneider, C. Drechsler, K. E. Ebbert, R. Rudolf, Q.-Q. Yan, J. J. Holstein, L. V. Schäfer and G. H. Clever, Angew. Chem., Int. Ed., 2022, 61, e202209305 CrossRef CAS PubMed;
(g) W.-L. Jiang, Z. Peng, B. Huang, X.-L. Zhao, D. Sun, X. Shi and H.-B. Yang, J. Am. Chem. Soc., 2021, 143, 433–441 CrossRef CAS PubMed.
- A. E. Martín Díaz and J. E. M. Lewis, Front. Chem., 2021, 9, 706462 CrossRef PubMed.
- M. D. Ward, Chem. Commun., 2009, 4487–4499 RSC.
- D. L. Caulder and K. N. Raymond, Acc. Chem. Res., 1999, 32, 975–982 CrossRef CAS.
- R. Chakrabarty, P. S. Mukherjee and P. J. Stang, Chem. Rev., 2011, 111, 6810–6918 CrossRef CAS PubMed.
- M. Fujita, K. Umemoto, M. Yoshizawa, N. Fujita, T. Kusukawa and K. Biradha, Chem. Commun., 2001, 509–518 RSC.
-
(a) A. J. McConnell, Chem. Soc. Rev., 2022, 51, 2957–2971 RSC;
(b) T. R. Cook, Y.-R. Zheng and P. J. Stang, Chem. Rev., 2013, 113, 734–777 CrossRef CAS PubMed;
(c) M. M. J. Smulders, I. A. Riddell, C. Browne and J. R. Nitschke, Chem. Soc. Rev., 2013, 42, 1728–1754 RSC;
(d) S. Lee, H. Jeong, D. Nam, M. S. Lah and W. Choe, Chem. Soc. Rev., 2021, 50, 528–555 RSC.
-
(a) J. R. Nitschke, Acc. Chem. Res., 2007, 40, 103–122 CrossRef CAS PubMed;
(b) A. M. Castilla, W. J. Ramsay and J. R. Nitschke, Acc. Chem. Res., 2014, 47, 2063–2073 CrossRef CAS PubMed;
(c) D. Zhang, T. K. Ronson and J. R. Nitschke, Acc. Chem. Res., 2018, 51, 2423–2436 CrossRef CAS PubMed.
- J. K. Clegg, J. Cremers, A. J. Hogben, B. Breiner, M. M. J. Smulders, J. D. Thoburn and J. R. Nitschke, Chem. Sci., 2013, 4, 68–76 RSC.
- Pd2L4 and Cu4L4 cages reported, respectively, from:
(a) P. Liao, B. W. Langloss, A. M. Johnson, E. R. Knudsen, F. S. Tham, R. R. Julian and R. J. Hooley, Chem. Commun., 2010, 46, 4932–4934 RSC;
(b) M. J. Prakash, M. Oh, X. Liu, K. N. Han, G. H. Seong and M. S. Lah, Chem. Commun., 2010, 46, 2049–2051 RSC.
- S. Pullen, J. Tessarolo and G. H. Clever, Chem. Sci., 2021, 12, 7269–7293 RSC.
- Heteroleptic cage from Fig. 7a from: W. M. Bloch, J. J. Holstein, W. Hiller and G. H. Clever, Angew. Chem., Int. Ed., 2017, 56, 8285–8289 CrossRef CAS PubMed.
-
(a) W. M. Bloch and G. H. Clever, Chem. Commun., 2017, 53, 8506–8516 RSC;
(b) D. Bardhan and D. K. Chand, Chem. – Eur. J., 2019, 25, 12241–12269 CrossRef CAS PubMed;
(c) L. R. Holloway, P. M. Bogie and R. J. Hooley, Dalton Trans., 2017, 46, 14719–14723 RSC.
-
(a) L. K. Moree, L. A. V. Faulkner and J. D. Crowley, Chem. Soc. Rev., 2024, 53, 25–46 RSC;
(b) F. Li and L. F. Lindoy, Aust. J. Chem., 2019, 72, 731–741 CrossRef CAS;
(c) M. Hardy and A. Lützen, Chem. – Eur. J., 2020, 26, 13332–13346 CrossRef CAS PubMed.
- Heteronuclear cage in Fig. 7b from: A. C. Pearcy, L. S. Lisboa, D. Preston, N. B. Page, T. Lawrence, L. J. Wright, C. G. Hartinger and J. D. Crowley, Chem. Sci., 2023, 14, 8615–8623 RSC.
-
(a) J. E. M. Lewis and J. D. Crowley, ChemPlusChem, 2020, 85, 815–827 CrossRef CAS PubMed;
(b) D. Tripathy, N. B. Debata, K. C. Naik and H. S. Sahoo, Coord. Chem. Rev., 2022, 456, 214396 CrossRef CAS.
- Pd2L4 cage assembled from low-symmetry ligand in Fig. 7c from: J. E. M. Lewis, A. Tarzia, A. J. P. White and K. E. Jelfs, Chem. Sci., 2020, 11, 677–683 RSC.
-
(a) D. Preston and J. D. Evans, Angew. Chem., Int. Ed., 2023, 62, e202314378 CrossRef CAS PubMed;
(b) T. Abe, N. Sanada, K. Takeuchi, A. Okazawa and S. Hiraoka, J. Am. Chem. Soc., 2023, 145, 28061–28074 CrossRef CAS PubMed;
(c) K. Wu, E. Benchimol, A. Baksi and G. H. Clever, Nat. Chem., 2024, 16, 584–591 CrossRef CAS PubMed;
(d) Z. T. Avery, J. L. Algar and D. Preston, Trends Chem., 2024, 6, 352–364 CrossRef.
- D. P. August, G. S. Nichol and P. J. Lusby, Angew. Chem., Int. Ed., 2016, 55, 15022–15026 CrossRef CAS PubMed.
- E. G. Percástegui, J. Mosquera and J. R. Nitschke, Angew. Chem., Int. Ed., 2017, 56, 9136–9140 CrossRef PubMed.
- A. Carné-Sánchez, J. Albalad, T. Grancha, I. Imaz, J. Juanhuix, P. Larpent, S. Furukawa and D. Maspoch, J. Am. Chem. Soc., 2019, 141, 4094–4102 CrossRef PubMed.
- E. G. Percástegui, T. Ronson and J. R. Nitschke, Chem. Rev., 2020, 120, 13840 CrossRef PubMed.
- D. A. Roberts, B. S. Pilgrim and J. R. Nitschke, Chem. Soc. Rev., 2018, 47, 626–644 RSC.
- K. Tan, N. Nijem, P. Canepa, Q. Gong, J. Li, T. Thonhauser and Y. J. Chabal, Chem. Mater., 2012, 24, 3153–3167 CrossRef CAS.
- S. M. McNeil, D. Preston, J. E. M. Lewis, A. Robert, K. Knerr-Rupp, D. O. Graham, J. R. Wright, G. I. Giles and J. D. Crowley, Dalton Trans., 2015, 44, 11129–11136 RSC.
-
(a) A. J. McConnell, C. S. Wood, P. P. Neelakandan and J. R. Nitschke, Chem. Rev., 2015, 115, 7729–7793 CrossRef CAS PubMed;
(b) E. Benchimol, B.-N. T. Nguyen, T. K. Ronson and J. R. Nitschke, Chem. Soc. Rev., 2022, 51, 5101–5135 RSC.
- S. Mollick, S. Fajal, S. Mukherjee and S. K. Ghosh, Chem. – Asian J., 2019, 14, 3096–3108 CrossRef CAS PubMed.
- D. Preston, S. M. McNeill, J. E. M. Lewis, G. I. Giles and J. D. Crowley, Dalton Trans., 2016, 45, 8050–8060 RSC.
- A. Ahmedova, D. Momekova, M. Yamashina, P. Shestakova, G. Momekov, M. Akita and M. Yoshizawa, Chem. – Asian J., 2016, 11, 474–477 CrossRef CAS PubMed.
- S. E. Walker, W. Chant, S. Thoonen, K. L. Tuck and D. R. Turner, Chem. – Eur. J., 2024, 30, e202400072 CrossRef CAS PubMed.
- L. Avram and Y. Cohen, Chem. Soc. Rev., 2015, 44, 586–602 RSC.
- M. Lehr, T. Paschelke, E. Trumpf, A.-M. Vogt, C. Näther, F. D. Sönnichsen and A. J. McConnell, Angew. Chem., Int. Ed., 2020, 59, 19344–19351 CrossRef CAS PubMed.
-
(a) J. S. Gardner, R. G. Harrison, J. D. Lamb and D. V. Dearden, New J. Chem., 2006, 30, 1276–1282 RSC;
(b) Z. Qi, T. Heinrich, S. Moorthy and C. A. Schalley, Chem. Soc. Rev., 2015, 44, 515–532 RSC.
- J. E. M. Lewis, Chem. Commun., 2022, 58, 13873–13886 RSC.
-
(a) C. J. Brown, F. D. Toste, R. G. Bergman and K. N. Raymon, Chem. Rev., 2015, 115, 3012–3035 CrossRef CAS PubMed;
(b) I. Sinha and P. S. Mukerjee, Inorg. Chem., 2018, 57, 4205–4221 CrossRef CAS PubMed;
(c) C. Tan, D. Chu, X. Tang, Y. Liu, W. Xuan and Y. Cui, Chem. – Eur. J., 2019, 25, 662–672 CrossRef CAS PubMed;
(d) Y. Xue, X. Hang, J. Ding, B. Li, R. Zhu, H. Pang and Q. Xu, Coord. Chem. Rev., 2021, 430, 213656 CrossRef CAS;
(e) T. K. Piskorz, V. Martí-Centelles, R. L. Spicer, F. Duarte and P. J. Lusby, Chem. Sci., 2023, 14, 11300–11331 RSC.
- D. Zhang, T. K. Ronson, Y.-Q. Zou and J. R. Nitschke, Nat. Rev. Chem., 2021, 5, 168–182 CrossRef CAS PubMed.
- A. Brzechwa-Chodzyńska, W. Drożdż, J. Harrowfield and A. R. Stefankiewicz, Coord. Chem. Rev., 2021, 434, 213820 CrossRef.
-
(a) T. R. Cook, V. Vajpayee, M. H. Lee, P. J. Stang and K.-W. Chi, Acc. Chem. Res., 2013, 46, 2464–2474 CrossRef CAS PubMed;
(b) C.-Y. Zhu, M. Pan and C.-Y. Su, Isr. J. Chem., 2019, 59, 209–219 CrossRef CAS;
(c) H. Sepehrpour, W. Fu, Y. Sun and P. J. Stang, J. Am. Chem. Soc., 2019, 141, 14005–14020 CrossRef CAS PubMed;
(d) Z. Li, W. Huan, Y. Wang and Y.-W. Yang, Small, 2024, 20, 2306245 CrossRef CAS PubMed;
(e) Y.-P. Wang, Y. Zhang, X.-H. Duan, J.-J. Mao, M. Pan, J. Shen and C.-Y. Su, Coord. Chem. Rev., 2024, 501, 215570 CrossRef CAS.
-
(a) J.-M. Lehn, Acc. Chem. Res., 1978, 11, 49–57 CrossRef CAS;
(b) K. Bowman-James, Acc. Chem. Res., 2005, 38, 671–678 CrossRef CAS PubMed.
-
(a) D. Yang, J. Zhao, X.-J. Yang and B. Wu, Org. Chem. Front., 2018, 5, 662–690 RSC;
(b) J. Zhao, D. Yang, X.-J. Yang and B. Wu, Coord. Chem. Rev., 2019, 378, 415–444 CrossRef CAS;
(c) L. Liang, W. Zhao, X.-J. Yang and B. Wu, Acc. Chem. Res., 2022, 55, 3218–3229 CrossRef CAS PubMed.
- C. Jia, B. Wu, S. Li, Z. Yang, Q. Zhao, J. Liang, Q.-S. Li and X.-J. Yang, Chem. Commun., 2010, 46, 5376–5378 RSC.
- W. Zhang, Y. Feng, B. Li, D. Yang, L. Hou, W. Zhao, X.-J. Yang and B. Wu, Chem. – Eur. J., 2022, 28, e202103671 CrossRef CAS PubMed.
- X. Zhao, H. Wang, B. Li, W. Zhang, X. Li, W. Zhao, C. Janiak, A. W. Heard, X.-J. Yang and B. Wu, Angew. Chem., Int. Ed., 2022, 61, e202115042 CrossRef CAS PubMed.
- X. Bai, C. Jia, Y. Zhao, D. Yang, S.-C. Wang, A. Li, Y.-T. Chan, Y.-Y. Wang, X.-J. Yang and B. Wu, Angew. Chem., Int. Ed., 2018, 57, 1851–1855 CrossRef CAS PubMed.
- B. Wu, F. Cui, Y. Lei, S. Li, N. de Sousa Amadeu, C. Janiak, Y.-J. Lin, L.-H. Weng, Y.-Y. Wang and X.-J. Yang, Angew. Chem., Int. Ed., 2013, 52, 5096–5100 CrossRef CAS PubMed.
- B. Li, T. Wei, X. Zhao, Y. Wwang, L. Xu, X.-J. Yang and B. Wu, Angew. Chem., Int. Ed., 2023, 62, e202301300 CrossRef CAS PubMed.
- J. Fu, B. Zheng, H. Zhang, Y. Zhao, D. Zhang, W. Zhang, X.-J. Yang and B. Wu, Chem. Commun., 2020, 56, 2475–2478 RSC.
- B. Li, B. Zheng, W. Zhang, D. Zhang, X.-J. Yang and B. Wu, J. Am. Chem. Soc., 2020, 142, 6304–6311 CrossRef CAS PubMed.
- D. Yang, J. Zhao, Y. Zhao, Y. Lei, L. Cao, X.-J. Yang, M. Davi, N. de Sousa Amadeu, C. Janiak, Z. Zhang, Y.-Y. Wang and B. Wu, Angew. Chem., Int. Ed., 2015, 54, 8658–8661 CrossRef CAS PubMed.
- D. Yang, J. Zhao, L. Yu, X. Lin, W. Zhang, H. Ma, A. Gogoll, Z. Zhang, Y. Wang, X.-J. Yang and B. Wu, J. Am. Chem. Soc., 2017, 139, 5946–5951 CrossRef CAS PubMed.
- W. Zhang, D. Yang, J. Zhao, L. Hou, J. L. Sessler, X.-J. Yang and B. Wu, J. Am. Chem. Soc., 2018, 140, 5248–5256 CrossRef CAS PubMed.
-
(a) M. Mastalerz, Angew. Chem., Int. Ed., 2010, 49, 5042–5053 CrossRef CAS PubMed;
(b) T. Hasell and A. I. Cooper, Nat. Rev. Chem., 2016, 1, 16053 CAS;
(c) M. Mastalerz, Acc. Chem. Res., 2018, 51, 2411–2422 CrossRef CAS PubMed;
(d) H. Wang, Y. Jin, N. Sun, W. Zhang and J. Jiang, Chem. Soc. Rev., 2021, 50, 8874–8886 RSC;
(e) G. Montà-Gonzálex, F. Sancenón, R. Martínez-Máñez and V. Martí-Centelles, Chem. Rev., 2022, 122, 13636–13708 CrossRef PubMed;
(f) X. Yang, Z. Ullah, J. F. Stoddart and C. T. Yavuz, Chem. Rev., 2023, 123, 4602–4634 CrossRef CAS PubMed;
(g) Z. Xu, Y. Ye, Y. Liu, H. Liu and S. Jiang, Chem. Commun., 2024, 60, 2261–2282 RSC.
- A. Fürstner, J. Am. Chem. Soc., 2021, 143, 15538–15555 CrossRef PubMed.
- F. B. L. Cougnon, A. R. Stefankiewicz and S. Ulrich, Chem. Sci., 2024, 15, 879–895 RSC; Y. Jin, Q. Wang, P. Taynton and W. Zhang, Acc. Chem. Res., 2014, 47, 1575–1586 CrossRef CAS PubMed; S. J. Rowan, S. J. Cantrill, G. R. L. Cousins, J. K. M. Sanders and J. F. Stoddart, Angew. Chem., Int. Ed., 2002, 41, 898–952 CrossRef PubMed; Y. Jin, C. Yu, R. J. Denman and W. Zhang, Chem. Soc. Rev., 2013, 42, 6634–6654 RSC; J.-M. Lehn, Chem. – Eur. J., 1999, 5, 2455–2463 CrossRef.
- J. C. Lauer, W.-S. Zhang, F. Rominger, R. R. Schröder and M. Mastalerz, Chem. – Eur. J., 2018, 24, 1739–2019 CrossRef PubMed.
- T. H. G. Schick, F. Rominger and M. Mastalerz, J. Org. Chem., 2020, 85, 13757–13771 CrossRef CAS PubMed.
-
(a) X. Liu and R. Warmuth, J. Am. Chem. Soc., 2006, 128, 14120–14127 CrossRef CAS PubMed;
(b) G.-F. Feng, J. Geng, F.-D. Feng and W. Huang, Sci. Rep., 2020, 10, 4712 CrossRef CAS PubMed.
-
(a) C. Givelet, J. Sun, D. Xu, T. J. Emge, A. Dhokte and R. Warmuth, Chem. Commun., 2011, 47, 4511–4513 RSC;
(b) Z. Lin, J. Sun, B. Efremovska and R. Warmuth, Chem. – Eur. J., 2012, 18, 12864–12872 CrossRef CAS PubMed;
(c) A. R. Stefankiewicz, M. R. Sambrook and J. K. M. Sanders, Chem. Sci., 2012, 3, 2326–2329 RSC;
(d) A. Galán, E. C. Escudero-Adán and P. Ballester, Chem. Sci., 2017, 8, 7746–7750 RSC;
(e) Y. Chen, G. Wu, B. Chen, H. Qu, T. Jiao, Y. Li, C. Ge, C. Zhang, L. Liang, X. Zeng, X. Cao, Q. Wang and H. Li, Angew. Chem., Int. Ed., 2021, 60, 18815–18820 CrossRef CAS PubMed.
-
(a) M. L. C. Quan and D. J. Cram, J. Am. Chem. Soc., 1991, 113, 2754–2755 CrossRef CAS;
(b) X. Liu, Y. Liu, G. Li and R. Warmuth, Angew. Chem., Int. Ed. Engl., 1996, 45, 901–904 CrossRef PubMed.
- V. Santolini, M. Miklitz, E. Berardo and K. E. Jelfs, Nanoscale, 2017, 9, 5280–5298 RSC.
- T. Jiao, G. Wu, L. Chen, C.-Y. Wang and H. Li, J. Org. Chem., 2018, 83, 12404–12410 CrossRef CAS PubMed.
-
(a) P. Skowronek and J. Gawronski, Org. Lett., 2008, 10, 4755–4758 CrossRef CAS PubMed;
(b) T. Tozawa, J. T. A. Jones, S. I. Swamy, S. Jiang, D. J. Adams, S. Shakespeare, R. Clowes, D. Bradshaw, T. Hasell, S. Y. Chong, C. Tang, S. Thompson, J. Parker, A. Trewin, J. Bacsa, A. M. Z. Slawin, A. Steiner and A. I. Cooper, Nat. Mater., 2009, 8, 973–978 CrossRef CAS PubMed.
- M. Mastalerz, M. W. Schneider, I. M. Oppel and O. Presly, Angew. Chem., Int. Ed., 2011, 60, 1046–1051 CrossRef PubMed.
- S. M. Elbert, N. I. Regenauer, D. Schindler, W.-S. Zhang, F. Rominger, R. R. Schröder and M. Mastalerz, Chem. – Eur. J., 2018, 24, 11438–11443 CrossRef CAS PubMed.
-
(a) Y. Jin, Y. Zhu and W. Zhang, CrystEngComm, 2013, 15, 1484–1499 RSC;
(b) K. Acharyya and P. S. Mukherjee, Angew. Chem., Int. Ed., 2019, 58, 8640–8653 CrossRef CAS PubMed;
(c) Y. Chen, H. Tang, H. Chen and H. Li, Acc. Chem. Res., 2023, 56, 2838–2840 CrossRef CAS PubMed.
- N. Iwasawa and K. Ono, Chem. Rec., 2022, 22, e202100214 CrossRef CAS PubMed.
-
(a) H. Kudo, R. Hayashi, K. Mitani, T. Yokozawa, N. C. Kasuga and T. Mishikubo, Angew. Chem., Int. Ed., 2006, 45, 7948–7952 CrossRef CAS PubMed;
(b) F. M. Alexander, S. F. Fonrouge, J. L. Borioni, M. G. Del Pópolo, P. N. Horton, S. J. Coles, B. P. Hutchings, D. E. Crawford and S. L. James, Chem. Sci., 2021, 12, 14230–14240 RSC.
-
(a) H. Yamada, T. Ikeda, T. Mizuta and T. Haino, Org. Lett., 2012, 14, 4510–4513 CrossRef CAS PubMed;
(b) D. Shimoyama, R. Sekiya, H. Kudo and T. Hain, Org. Lett., 2020, 22, 352–356 CrossRef CAS PubMed.
-
(a) S. Lee, A. Yang, T. P. Moneypenny and J. S. Moore, J. Am. Chem. Soc., 2016, 138, 2182–2185 CrossRef CAS PubMed . For a review, see: ;
(b) S. Huang, Z. Lei, Y. Jin and W. Zhang, Chem. Sci., 2021, 12, 9591–9606 RSC.
-
(a) M. S. Collins, M. E. Carnes, B. P. Nell, L. N. Zakharov and D. W. Johnson, Nat. Commun., 2016, 7, 11052 CrossRef CAS PubMed . For reviews, see: ;
(b) S. P. Black, J. K. M. Sanders and A. R. Stefankiewicz, Chem. Soc. Rev., 2014, 43, 1861–1872 RSC;
(c) M. S. Collins, N.-M. Phan, L. N. Zakharov and D. W. Johnson, Inorg. Chem., 2018, 57, 3486–3496 CrossRef CAS PubMed.
-
(a) K. Ono, K. Johmoto, N. Yasuda, H. Uekusa, S. Fujii, M. Kiguchi and N. Iwasawa, J. Am. Chem. Soc., 2015, 137, 7015–7018 CrossRef CAS PubMed . For a review, see: ;
(b) K. Ono and N. Iwasawa, J. Incl. Phenom. Macrocycl. Chem., 2021, 101, 19–29 CrossRef CAS.
- N. Christinat, R. Scopelliti and K. Severin, Angew. Chem., Int. Ed., 2008, 47, 1848–1852 CrossRef CAS PubMed.
- C. C. Pattillo and J. S. Moore, Chem. Sci., 2019, 10, 7043–7048 RSC.
- J. Koo, I. Kim, Y. Kim, D. Cho, I.-C. Hwang, R. D. Mukhopadhyay, H. Song, Y. H. Ko, A. Dhamija, H. Lee, W. Hang, S. Kim, M.-H. Baik and K. Kim, Chem, 2020, 6, 3374–3384 CAS.
-
(a) M. A. Little and A. I. Cooper, Adv. Funct. Mater., 2020, 30, 1909842 CrossRef CAS;
(b) W. Wang, K. Su and D. Yuan, Mater. Chem. Front., 2023, 7, 5247–5262 RSC.
-
(a) N. Giri, M. G. Del Pópolo, G. Melaugh, R. L. Greenaway, K. Rätzke, T. Koschine, L. Pison, M. F. Costa Gomes, A. I. Cooper and S. L. James, Nature, 2015, 527, 216–220 CrossRef CAS PubMed;
(b) B. D. Egleston, A. Mroz, K. E. Jelfs and R. L. Greenaway, Chem. Sci., 2022, 13, 5042–5054 RSC.
- N. Giri, C. E. Davidson, G. Melaugh, M. G. Del Pópolo, J. T. A. Jones, T. Hasell, A. I. Copper, P. N. Horton, M. B. Hursthouse and S. L. James, Chem. Sci., 2012, 3, 2153–2157 RSC.
-
(a) T. Hasell, M. Schmidtmann, C. A. Stone, M. W. Smith and A. I. Cooper, Chem. Commun., 2012, 48, 4689–4691 RSC;
(b) S. Hong, R. Rohman, J. Jia, Y. Kim, D. Moon, Y. Kim, Y. H. Ko, E. Lee and K. Kim, Angew. Chem., Int. Ed., 2015, 54, 13241–13244 CrossRef CAS PubMed.
- S. Bera, K. Dey, T. K. Pal, A. Halder, S. Tothadi, S. Karak, M. Addicoat and R. Banerjee, Angew. Chem., Int. Ed., 2019, 58, 4243–4247 CrossRef CAS PubMed.
-
(a) P. Kieryk, J. Janczak, J. Panek, M. Miklitz and J. Lisowski, Org. Lett., 2016, 18, 12–15 CrossRef CAS PubMed;
(b) S. Bera, A. Basu, S. Tothadi, B. Garai, S. Banerjee, K. Vanka and R. Banerjee, Angew. Chem., Int. Ed., 2017, 56, 2123–2126 CrossRef CAS PubMed;
(c) D.-X. Cui, Y. Geng, J.-N. Kou, G.-G. Shan, C.-Y. Sun, K.-H. Zhang, Z.-L. Wang and Z.-M. Su, Nat. Commun., 2022, 13, 4011 CrossRef CAS PubMed.
- M. Yang, F. Qiu, E.-S. M. El-Sayed, W. Wang, S. Du, K. Su and D. Yuan, Chem. Sci., 2021, 12, 13307–13315 RSC.
- K. Acharyya, S. Mukherjee and P. S. Mukherjee, J. Am. Chem. Soc., 2013, 135, 554–557 CrossRef CAS PubMed.
- S. Bennett, F. T. Szczypiński, L. Turcani, M. E. Briggs, R. L. Greenaway and K. E. Jelfs, J. Chem. Inf. Model., 2021, 61, 4342–4356 CrossRef CAS PubMed.
- B. D. Egleston, K. V. Luzyanin, M. C. Brand, R. Clowes, M. E. Briggs, R. L. Greenaway and A. I. Copper, Angew. Chem., Int. Ed., 2020, 59, 7362–7366 CrossRef CAS PubMed.
- R. L. Greenaway, D. Holden, E. G. B. Eden, A. Stephenson, C. W. Yong, M. B. Bennison, T. Hasell, M. E. Briggs, S. L. James and A. I. Copper, Chem. Sci., 2017, 8, 2640–2651 RSC.
- M. E. Briggs and A. I. Cooper, Chem. Mater., 2017, 29, 149–157 CrossRef CAS PubMed.
-
(a) A. P. Davis and R. S. Wareham, Angew. Chem., Int. Ed., 1998, 37, 2270–2273 CrossRef;
(b) E. Klein, M. P. Crump and A. P. Davis, Angew. Chem., Int. Ed., 2005, 44, 298–302 CrossRef CAS PubMed;
(c) Y. Ferrand, M. P. Crump and A. P. Davis, Science, 2007, 318, 619–622 CrossRef CAS PubMed;
(d) N. P. Barwell and A. P. Davis, J. Org. Chem., 2011, 76, 6548–6557 CrossRef CAS PubMed;
(e) B. Sookcharoenpinyo, E. Klein, Y. Ferrand, D. B. Walker, P. R. Brotherhood, C. Ke, M. P. Crump and A. P. Davis, Angew. Chem., Int. Ed., 2012, 51, 4586–4590 CrossRef CAS PubMed.
- F. Huang, H. W. Gibson, W. S. Bryant, D. S. Nagvekar and F. R. Fronczek, J. Am. Chem. Soc., 2003, 125, 9367–9371 CrossRef CAS PubMed.
- Z. Wu, S. Lee and J. S. Moore, J. Am. Chem. Soc., 1992, 114, 8730–8732 CrossRef CAS.
-
(a) C. Zhang and C.-F. Chen, J. Org. Chem., 2007, 72, 9339–9341 CrossRef CAS PubMed;
(b) A. Avellaneda, P. Valente, A. Burgun, J. D. Evans, A. W. Markwell-Heys, D. Rankine, D. J. Nielsen, M. R. Hill, C. J. Sumby and C. J. Doonan, Angew. Chem., Int. Ed., 2013, 52, 3746–3749 CrossRef CAS PubMed;
(c) A. Burgun, P. Valente, J. D. Evans, D. M. Huang, C. J. Sumby and C. J. Doonan, Chem. Commun., 2016, 52, 8850–8853 RSC.
-
(a) K. Matsui, Y. Segawa, T. Namikawa, K. Kamada and K. Itami, Chem. Sci., 2013, 4, 84–88 RSC;
(b) Y. Ni, T. Y. Gopalakrishna, H. Phan, T. Kim, T. S. Herng, Y. Han, T. Tao, J. Ding, D. Kim and J. Wu, Nat. Commun., 2020, 12, 242–248 CAS;
(c) Y. Ni, F. Gordillo-Gámez, M. P. Alvarez, Z. Nan, Z. Li, S. Wu, Y. Han, J. Casado and J. Wu, J. Am. Chem. Soc., 2020, 142, 12730–12742 CrossRef CAS PubMed;
(d) T. Matsushima, S. Kikkawa, I. Azumaya and S. Watanabe, ChemistryOpen, 2018, 7, 278–281 CrossRef CAS PubMed;
(e) X. Gu, T. Y. Gopalakrishna, H. Phan, Y. Ni, T. S. Herng, J. Ding and J. Wu, Angew. Chem., Int. Ed., 2017, 129, 15585–15589 CrossRef;
(f) S. Cui, G. Zhuang, D. Lu, Q. Huang, H. Jia, Y. Wang, S. Yang and P. Du, Angew. Chem., Int. Ed., 2018, 57, 9330–9335 CrossRef CAS PubMed.
-
(a) J. Morales-Sanfrutos, M. Ortega-Muñoz, J. Lopez-Jaramillo, F. Hernandez-Mateo and F. Santoyo-Gonzalez, J. Org. Chem., 2008, 73, 7772–7774 CrossRef CAS PubMed;
(b) J. Zhang, Y. Li, W. Yang, S.-W. Lai, C. Zhou, H. Liu, C.-M. Che and Y. Li, Chem. Commun., 2012, 48, 3602–3604 RSC;
(c) L. C. Gilday, N. G. White and P. D. Beer, Dalton Trans., 2012, 41, 7092–7097 RSC;
(d) J. Samanta and R. Natarajan, Org. Lett., 2016, 18, 3394–3397 CrossRef CAS PubMed.
- C. M. Thomas and N. G. White, Supramol. Chem., 2024 DOI:10.1080/10610278.2024.2324822.
-
(a) E. J. Dale, N. A. Vermeulen, A. A. Thomas, J. C. Barnes, M. Juríček, A. K. Blackburn, N. L. Strutt, A. A. Sarjeant, C. L. Stern, S. E. Denmark and J. F. Stoddart, J. Am. Chem. Soc., 2014, 136, 10669–10682 CrossRef CAS PubMed;
(b) N. Hafezi, J. M. Holcroft, K. J. Hartlieb, E. J. Dale, N. A. Vermeulen, C. L. Stern, A. A. Sarjeant and J. F. Stoddart, Angew. Chem., Int. Ed., 2015, 54, 456–461 CrossRef CAS PubMed;
(c) H. Duan, Y. Li, Q. Li, P. Wang, X. Liu, L. Cheng, Y. Yu and L. Cao, Angew. Chem., Int. Ed., 2020, 59, 10101–10110 CrossRef CAS PubMed.
- W. Kiggen and F. Vögtle, Angew. Chem., Int. Ed. Engl., 1984, 23, 714–715 CrossRef.
- X. Zhao, Y. Liu, Z.-Y. Zhang, Y. Wang, X. Jia and C. Li, Angew. Chem., Int. Ed., 2021, 60, 17904–17909 CrossRef CAS PubMed.
- Y. Sato, M. Abekura, T. Oriki, Y. Nagashima, H. Uekusa and K. Tanaka, Angew. Chem., Int. Ed., 2023, 62, e202304041 CrossRef CAS PubMed.
- Y. Liu, W. Zhao, C.-H. Chen and A. H. Flood, Science, 2019, 365, 159–161 CrossRef CAS PubMed.
- J. L. Katz, K. J. Selby and R. R. Conry, Org. Lett., 2005, 7, 3505–3507 CrossRef CAS PubMed.
-
(a) D. G. Rivera and L. A. Wessjohann, J. Am. Chem. Soc., 2006, 128, 7122–7123 CrossRef CAS PubMed;
(b) L. A. Wessjohann, O. Kreye and D. G. Rivera, Angew. Chem., Int. Ed., 2017, 56, 3501–3505 CrossRef CAS PubMed.
- T. J. McMurry, S. J. Rodgers and K. N. Raymond, J. Am. Chem. Soc., 1987, 109, 3451–3453 CrossRef CAS.
- J. Cremers, R. Haver, M. Rickhaus, J. Q. Gong, L. Favereau, M. D. Peeks, T. D. W. Claridge, L. M. Herz and H. L. Anderson, J. Am. Chem. Soc., 2018, 140, 5352–5355 CrossRef CAS PubMed.
-
(a) S. Hiraoka, Y. Yamauchi, R. Arakane and M. Shionoya, J. Am. Chem. Soc., 2009, 131, 11646–11647 CrossRef CAS PubMed;
(b) L.-Y. Sun, N. Sinha, T. Yan, Y.-S. Wang, T. T. Y. Tan, L. Yu, Y.-F. Han and F. E. Hahn, Angew. Chem., Int. Ed., 2018, 57, 5161–5165 CrossRef CAS PubMed.
- R. A. Tromans, T. S. Carter, L. Chabanne, M. P. Crump, H. Li, J. V. Matlock, M. G. Orchard and A. P. Davis, Nat. Chem., 2019, 11, 52–56 CrossRef CAS PubMed.
- S.-N. Liu, Q.-X. Ren, Y.-T. Ding, X.-P. Cao, Z.-F. Shi, H.-F. Chow and D. Kuck, J. Org. Chem., 2024, 89, 2127–2137 CrossRef CAS PubMed.
-
(a) S. I. Swamy, J. Bacsa, J. T. A. Jones, K. C. Stylianou, A. Steiner, L. K. Ritchie, T. Hasell, J. A. Gould, A. Laybourn, Y. Z. Khimyak, D. J. Adams, M. J. Rosseinsky and A. I. Cooper, J. Am. Chem. Soc., 2010, 132, 12773–12775 CrossRef CAS PubMed;
(b) Y. Jin, B. A. Voss, R. D. Noble and W. Zhang, Angew. Chem., Int. Ed., 2010, 49, 6348–6351 CrossRef CAS PubMed;
(c) M. Mastalerz, M. W. Schneider, I. M. Oppel and O. Presly, Angew. Chem., Int. Ed., 2011, 50, 1046–1051 CrossRef CAS PubMed.
-
(a) A. S. Bhat, S. M. Elbert, W.-S. Zhang, F. Rominger, M. Dieckmann, R. R. Schröder and M. Mastalerz, Angew. Chem., Int. Ed., 2019, 58, 8819–8823 CrossRef CAS PubMed;
(b) J. C. Lauer, A. S. Bhat, C. Barwig, N. Fritz, T. Kirschbaum, F. Rominger and M. Mastalerz, Chem. – Eur. J., 2022, 28, e202201527 CrossRef CAS PubMed.
- M. Liu, M. A. Little, K. E. Jelfs, J. T. A. Jones, M. Schmidtmann, S. Y. Chong, T. Hasell and A. I. Cooper, J. Am. Chem. Soc., 2014, 136, 7583–7586 CrossRef CAS PubMed.
- X.-Y. Hu, W.-S. Zhang, F. Rominger, I. Wacker, R. R. Schröder and M. Mastalerz, Chem. Commun., 2017, 53, 8616–8619 RSC.
- P.-E. Alexandre, W.-S. Zhang, F. Rominger, S. M. Elbert, R. R. Schröder and M. Mastalerz, Angew. Chem., Int. Ed., 2020, 59, 19675–19679 CrossRef CAS PubMed.
- T. H. G. Schick, J. C. Lauer, F. Rominger and M. Mastalerz, Angew. Chem., Int. Ed., 2019, 58, 1768–1773 CrossRef CAS PubMed.
- T. Pausch, T. David, T. Fleck-Kunde, H. Pols, J. Gurke and B. M. Schmidt, Angew. Chem., Int. Ed., 2024, 63, e202318362 CrossRef CAS PubMed.
- E. Kayahara, T. Iwamoto, H. Takay, T. Suzuki, M. Fujitsuka, T. Majima, N. Yasuda, N. Matsuyama, S. Seki and S. Yamago, Nat. Commun., 2013, 4, 2694 CrossRef PubMed.
- D. Chakraborty and P. S. Mukherjee, Chem. Commun., 2022, 58, 5558–5573 RSC.
- M. W. Schneider, I. M. Oppel, H. Ott, L. G. Lechner, H.-J. S. Hauswald, R. Stoll and M. Mastalerz, Chem. – Eur. J., 2012, 18, 836–847 CrossRef CAS PubMed.
-
(a) K. Bowman-James, Science, 2019, 365, 124–125 CrossRef CAS PubMed;
(b) S. O. Kang, A. Hossain and K. Bowman-James, Coord. Chem. Rev., 2006, 250, 3038–3052 CrossRef CAS;
(c) S. O. Kang, J. M. Llinares, V. W. Day and K. Bowman-James, Chem. Soc. Rev., 2010, 39, 3980–4003 RSC;
(d) G. Alibrandi, V. Amendola, G. Bergamaschi, L. Fabbrizzi and M. Licchelli, Org. Biomol. Chem., 2015, 13, 3510–3524 RSC;
(e) L. K. Macreadie, A. M. Gilchrist, D. A. McNaughton, W. G. Ryder, M. Fares and P. A. Gale, Chem, 2022, 8, 46–118 CrossRef CAS.
- L. Jing, E. Deplazes, J. K. Clegg and X. Wu, Nat. Chem., 2024, 16, 335–342 CrossRef CAS PubMed.
-
(a) W. Kiggen and F. Vögtle, Angew. Chem., Int. Ed. Engl., 1984, 23, 714–715 CrossRef;
(b) T. M. Garrett, T. J. McMurry, M. W. Hosseini, Z. E. Reyes, F. E. Hahn and K. N. Raymond, J. Am. Chem. Soc., 1991, 113, 2965–2977 CrossRef CAS;
(c) G. N. Di Francesco, A. Gaillard, I. Ghiviriga, K. A. Abboud and L. J. Murray, Inorg. Chem., 2014, 53, 4647–4654 CrossRef CAS PubMed;
(d) D. Zhang, K. Jamieson, L. Guy, G. Gao, J.-P. Dutasta and A. Martinez, Chem. Sci., 2017, 8, 789–794 RSC;
(e) S. C. Bete, C. Würtele and M. Otte, Chem. Commun., 2019, 55, 4427–4430 RSC;
(f) G. Qiu, P. Nava, A. Martinez and C. Colomban, Chem. Commun., 2021, 57, 2281–2284 RSC;
(g) M. Hennebelle, Y. Cirillo, A.-D. Manick, D. Nuel, A. Martinez and B. Chatelet, J. Org. Chem., 2024, 89, 4741–4748 CrossRef CAS PubMed.
-
(a) R. A. Tromans, T. S. Carter, L. Chabanne, M. P. Crump, H. Li, J. V. Matlock, M. G. Orchard and A. P. Davis, Nat. Chem., 2019, 11, 52–56 CrossRef CAS PubMed;
(b) W. Liu, Y. Tan, L. O. Jones, B. Song, Q.-H. Guo, L. Zhang, Y. Qiu, Y. Feng, X.-Y. Chen, G. C. Schatz and F. J. Stoddart, J. Am. Chem. Soc., 2021, 143, 15688–15700 CrossRef CAS PubMed.
-
(a) D. Daneilsiek and G. Dyker, Eur. J. Org. Chem., 2021, 1026–1034 CrossRef;
(b) K. Acharyya and P. S. Mukherjee, Chem. Commun., 2014, 50, 15788–15791 RSC.
- Y. Shi, K. Cai, H. Xiao, Z. Liu, J. Zhou, D. Shen, Y. Qiu, Q.-H. Guo, C. Stern, M. R. Wasielewski, F. Diederich, W. A. Goddard and J. F. Stoddart, J. Am. Chem. Soc., 2018, 140, 13835–13842 CrossRef CAS PubMed.
- S. La Cognata and V. Amendola, Chem. Commun., 2023, 59, 13668–13678 RSC.
-
(a) S. C. Bete and M. Otte, Angew. Chem., Int. Ed., 2021, 60, 18582–18586 CrossRef CAS PubMed;
(b) S. C. Bete, L. K. May, P. Woite, M. Roemelt and M. Otte, Angew. Chem., Int. Ed., 2022, 61, e202206120 CrossRef CAS PubMed.
- E. Arunan, G. R. Desiraju, R. A. Klein, J. Sadlej, S. Scheiner, I. Alkorta, D. C. Clary, R. H. Crabtree, J. J. Dannenberg, P. Hobza, H. G. Kjaergaard, A. C. Legon, B. Mennucci and D. J. Nesbitt, Pure Appl. Chem., 2011, 83, 1619–1636 CrossRef CAS.
- R. Wyler, J. de Mendoza and J. Rebek, Angew. Chem., Int. Ed. Engl., 1993, 32, 1699–1701 CrossRef.
- N. Branda, R. Wyler and J. Rebek, Science, 1994, 263, 1267–1268 CrossRef CAS PubMed.
- M. R. Ghadiri, K. Kobayashi, J. R. Granja, R. K. Chadha and D. E. McRee, Angew. Chem., Int. Ed. Engl., 1995, 34, 93–95 CrossRef CAS.
- A. Scarso, L. Pellizzaro, O. De Lucchi, A. Linden and F. Fabris, Angew. Chem., Int. Ed., 2007, 46, 4972–4975 CrossRef CAS PubMed.
- M. R. Ghadiri, K. Kobayashi, J. R. Granja, R. K. Chadha and D. E. McRee, Angew. Chem., Int. Ed. Engl., 1995, 34, 93–95 CrossRef CAS.
-
(a) M. Alajarín, A. López-Lázaro, A. Pastor, J. W. Steed and R. Arakawa, Chem. Commun., 2001, 169–170 RSC;
(b) M. Alajarín, A. Pastor, R.-Á. Orenes and J. W. Steed, J. Org. Chem., 2002, 67, 7091–7095 CrossRef PubMed;
(c) B. C. Hamann, K. D. Shimizu and J. Rebek, Angew. Chem., Int. Ed. Engl., 1996, 35, 1326–1329 CrossRef CAS.
- A. Shivanyuk, E. F. Paulus and V. Böhmer, Angew. Chem., Int. Ed., 1999, 38, 2906–2909 CrossRef CAS PubMed.
- J. N. Smith, C. Ennis and N. T. Lucas, Chem. Sci., 2021, 12, 11858–11863 RSC.
- R. S. Meissner, J. Rebek and J. de Mendoza, Science, 1995, 270, 1485–1488 CrossRef CAS PubMed.
- T. Heinz, D. M. Rudkevich and J. Rebek, Nature, 1998, 394, 764–766 CrossRef CAS.
-
(a) T. Martín, U. Obst and J. Rebek, Science, 1998, 281, 1842–1845 CrossRef;
(b) D. W. Johnson, F. Hof, P. M. Iovine, C. Nuckolls and J. Rebek, Angew. Chem., Int. Ed., 2002, 41, 3793–3796 CrossRef CAS.
-
(a) D. Beaudoin, F. Rominger and M. Mastalerz, Angew. Chem., Int. Ed., 2016, 55, 15599–15603 CrossRef CAS PubMed;
(b) G. Markiewicz, A. Jenczak, M. Kołodziejski, J. J. Holstein, J. K. M. Sanders and A. R. Stefankiewicz, Nat. Commun., 2017, 8, 15109 CrossRef PubMed.
- S. Merget, L. Catti, S. Zev, D. T. Major, N. Trapp and K. Tiefenbacher, Chem. – Eur. J., 2021, 27, 4447–4453 CrossRef CAS PubMed.
- L. R. MacGillivray and J. L. Atwood, Nature, 1997, 389, 469–472 CrossRef CAS.
- A. Shivanyuk and J. Rebek, Chem. Commun., 2001, 2424–2425 RSC.
-
(a) K. Kobayashi, K. Ishi, S. Sakamoto, T. Shirasaka and K. Yamaguchi, J. Am. Chem. Soc., 2003, 125, 10615–10624 CrossRef CAS PubMed;
(b) H. Kitagawa, Y. Kobori, M. Yamanaka, K. Yoza and K. Kobayashi, Proc. Natl. Acad. Sci. U. S. A., 2009, 106, 10444–10448 CrossRef CAS PubMed;
(c) I. Higler, L. Grave, E. Breuning, W. Verboom, F. de Jong, T. M. Fyles and D. N. Reinhoudt, Eur. J. Org. Chem., 2000, 1727–1734 CrossRef CAS.
-
(a) J. Nakazawa, M. Mizuki, Y. Shimazaki, F. Tani and Y. Naruta, Org. Lett., 2006, 8, 4275–4278 CrossRef CAS PubMed;
(b) K. Kishimoto, M. Nakamura and K. Kobayashi, Chem. – Eur. J., 2016, 22, 2629–2633 CrossRef CAS PubMed;
(c) K. Nakabayashi, K. Kishimoto and K. Kobayashi, Chem. – Asian J., 2022, 11, e202100646 CAS.
-
(a) T. Grawe, T. Schrader, M. Gurrath, A. Kraft and F. Osterod, Org. Lett., 2000, 2, 29–32 CrossRef CAS PubMed;
(b) R. Zadmard, T. Schrader, T. Grawe and A. Kraft, Org. Lett., 2002, 4, 1687–1690 CrossRef CAS PubMed;
(c) F. Corbellini, L. Di Costanzo, M. Crego-Calama, S. Germia and D. N. Reinhoudt, J. Am. Chem. Soc., 2003, 125, 9946–9947 CrossRef CAS PubMed;
(d) J. S. Sasine, R. E. Brewster, K. L. Caran, A. M. Bentley and S. B. Shuker, Org. Lett., 2006, 8, 2913–2915 CrossRef CAS PubMed;
(e) T. Kusukawa, C. Katano and C. Kim, Tetrahedron, 2012, 68, 1492–1501 CrossRef CAS;
(f) C. M. Thomas, É. M. Foyle, S. E. Walker and N. G. White, Aust. J. Chem., 2021, 74, 787–794 CrossRef CAS.
-
(a) K. Kobayashi, T. Shirasaka, K. Yamaguchi, S. Sakamoto, E. Horn and N. Furukawa, Chem. Commun., 2000, 41–42 RSC;
(b) L. R. MacGillivray, P. R. Diamente, J. L. Reid and J. A. Ripmeester, Chem. Commun., 2000, 359–360 RSC.
- J. M. C. A. Kerckhoffs, F. W. B. van Leeuwen, A. L. Spek, H. Kooijman, M. Crego-Calama and D. N. Reinhoudt, Angew. Chem., Int. Ed., 2003, 42, 5715–5722 Search PubMed.
- K.-D. Zhang, D. Ajami and J. Rebek, J. Am. Chem. Soc., 2013, 135, 18064–18066 CrossRef CAS PubMed.
-
(a) Q. Zhang, L. Catti and K. Tiefenbacher, Acc. Chem. Res., 2018, 51, 2107–2114 CrossRef CAS PubMed;
(b) C. Gaeta, C. Talotta, M. De Rosa, P. La Manna, A. Soriente and P. Neri, Chem. – Eur. J., 2019, 25, 4899–4913 CrossRef CAS PubMed.
-
(a) E. Huerta, G. A. Metselaar, A. Fragoso, E. Santos, C. Bo and J. de Mendoza, Angew. Chem., Int. Ed., 2007, 46, 202–205 CrossRef CAS PubMed;
(b) E. Huerta, E. Cequier and J. de Mendoza, Chem. Commun., 2007, 5016–5018 RSC.
-
(a) S. K. Körner, F. C. Tucci, D. M. Rudkevich, T. Heinz and J. Rebek, Chem. – Eur. J., 2000, 6, 187–195 CrossRef;
(b) A. Shivanyuk, J. C. Friese, S. Döring and J. Rebek, J. Org. Chem., 2003, 68, 6489–6496 CrossRef CAS PubMed;
(c) A. C. Sather, O. B. Berryman, D. Ajami and J. Rebek, Tetrahedron Lett., 2011, 52, 2100–2103 CrossRef CAS.
- C. B. Aakeröy, A. Rajbanshi, P. Metrangolo, G. Resnati, M. F. Parisi, J. Desper and T. Pilati, CrystEngComm, 2012, 14, 6366–6368 RSC.
-
(a) O. Dumele, N. Trapp and F. Diederich, Angew. Chem., Int. Ed., 2015, 54, 12339–12344 CrossRef CAS PubMed;
(b) O. Dumele, B. Schreib, U. Warzok, N. Trapp, C. A. Schalley and F. Diederich, Angew. Chem., Int. Ed., 2017, 56, 1152–1157 CrossRef CAS PubMed.
- E. Taipale, J. S. Ward, G. Fiorini, D. L. Stares, C. A. Schalley and K. Rissanen, Inorg. Chem. Front., 2022, 9, 2231–2239 RSC.
- L. Turunen, U. Warzok, R. Puttreddy, N. K. Beyeh, C. A. Schalley and K. Rissanen, Angew. Chem., Int. Ed., 2016, 55, 14033–14036 CrossRef CAS PubMed.
- L. Turunen, A. Peuronen, S. Forsblom, E. Kalenius, M. Lahtinen and K. Rissanen, Chem. – Eur. J., 2017, 23, 11714–11718 CrossRef CAS PubMed.
- L. Turunen, U. Warzok, C. A. Schalley and K. Rissanen, Chem, 2017, 3, 861–869 CAS.
- L.-J. Riwar, N. Trapp, K. Root, R. Zenobi and F. Diederich, Angew. Chem., Int. Ed., 2018, 57, 17259–17264 CrossRef CAS PubMed.
- F.-U. Rahman, D. Tzeli, I. D. Petsalakis, G. Theodorakopoulos, P. Ballester, J. Rebek and Y. Yu, J. Am. Chem. Soc., 2020, 142, 5876–5883 CrossRef CAS PubMed.
- K. Kanagaraj, R. Wang, M.-K. Zhao, P. Ballester, J. Rebek and Y. Yu, J. Am. Chem. Soc., 2023, 145, 5816–5823 CrossRef CAS PubMed.
-
(a) K. Twum, K. Rissanen and N. K. Bayeh, Chem. Rec., 2021, 21, 386–395 CrossRef CAS PubMed;
(b) Y.-J. Zhu, Y. Gao, M.-M. Tang, J. Rebek and Y. Yu, Chem. Commun., 2021, 57, 1543–1549 RSC.
- SCXRD structure of cavitand vase conformer shown in Fig. 18 from: E. Dalcanale, P. Soncini, G. Bacchilega and F. Ugozzoli, J. Chem. Soc., Chem. Commun., 1989, 500–502 RSC.
- J. R. Moran, J. L. Ericson, E. Dalcanale, J. A. Bryant, C. B. Knobler and D. J. Cram, J. Am. Chem. Soc., 1991, 113, 5707–5714 CrossRef CAS.
- SCXRD structure of velcraplex shown in Fig. 18 from: H. Ihm, J.-S. Ahn, M. S. Lah, Y. H. Ko and K. Paek, Org. Lett., 2004, 6, 3893–3896 CrossRef CAS PubMed.
-
(a) J. A. Bryant, C. B. Knobler and D. J. Cram, J. Am. Chem. Soc., 1990, 112, 1254–1255 CrossRef CAS;
(b) J. A. Bryant, J. L. Ericson and D. J. Cram, J. Am. Chem. Soc., 1990, 112, 1255–1256 CrossRef CAS.
- D. J. Cram, H.-J. Choi, J. A. Bryant and C. B. Knobler, J. Am. Chem. Soc., 1992, 114, 7748–7765 CrossRef CAS.
- L. Pirondini, A. G. Stendardo, S. Geremia, M. Campagnolo, P. Samorì, J. P. Rabe, R. Fokkens and E. Dalcanale, Angew. Chem., Int. Ed., 2003, 42, 1384–1387 CrossRef CAS PubMed.
-
(a) F. Tancini, E. Rampazzo and E. Dalcanale, Aust. J. Chem., 2010, 63, 646–652 CrossRef CAS;
(b) J. Tellers, J. Vachon, M. Soliman, E. Dalcanale and R. Pinalli, Molecules, 2019, 24, 902 CrossRef PubMed.
- B. C. Gibb, Nat. Chem., 2010, 2, 512–513 CrossRef CAS PubMed.
- C. L. D. Gibb, E. D. Stevens and B. C. Gibb, J. Am. Chem. Soc., 2001, 123, 5849–5850 CrossRef CAS PubMed.
- C. L. D. Gibb and B. C. Gibb, J. Am. Chem. Soc., 2004, 126, 11408 CrossRef CAS PubMed.
-
(a) C. L. D. Gibb and B. C. Gibb, J. Am. Chem. Soc., 2006, 128, 16498–16499 CrossRef CAS PubMed;
(b) L. S. Kaanumalle, C. L. D. Gibb, B. C. Gibb and V. Ramamurthy, J. Am. Chem. Soc., 2005, 127, 3674–3675 CrossRef CAS PubMed.
- S. Liu and B. C. Gibb, Chem. Commun., 2011, 47, 3574–3576 RSC.
- E. Ramasamy, N. Jayaraj, M. Porel and V. Ramamurthy, Langmuir, 2012, 28, 10–16 CrossRef CAS PubMed.
- R. Kulasekharana and V. Ramamurthy, Org. Lett., 2011, 13, 5092–5095 CrossRef PubMed.
- H. Gan and B. C. Gibb, Chem. Commun., 2013, 49, 1395–1397 RSC.
- H. Gan and B. C. Gibb, Chem. Commun., 2012, 48, 1656–1658 RSC.
- M. D. Giles, S. Liu, R. L. Emanuel, B. C. Gibb and S. M. Grayson, J. Am. Chem. Soc., 2008, 130, 14430–14431 CrossRef CAS PubMed.
- S. Hiraoka, K. Harano, M. Shiro and M. Shionoya, J. Am. Chem. Soc., 2008, 130, 14368–14369 CrossRef CAS PubMed.
- S. Hiraoka, K. Harano, T. Makamura, M. Shiro and M. Shionoya, Angew. Chem., Int. Ed., 2009, 48, 7006 CrossRef CAS PubMed.
- S. Hiraoka, T. Makamura, M. Shiro and M. Shionoya, J. Am. Chem. Soc., 2010, 132, 13223–13225 CrossRef CAS PubMed.
-
(a) Y.-Y. Zhan, K. Ogata, T. Kojima, T. Koide, K. Ishii, T. Mashiko, M. Tachikawa, S. Uchiyama and S. Hiraoka, Commun. Chem., 2018, 1, 14 CrossRef;
(b) Y.-Y. Zhan, T. Kojima, T. Koide, M. Tachikawa and S. Hiraoka, Chem. – Eur. J., 2018, 24, 9130–9135 CrossRef CAS PubMed.
-
(a) S. Liu and B. C. Gibb, Chem. Commun., 2008, 3709–3716 RSC;
(b) Z. Laughrey and B. C. Gibb, Chem. Soc. Rev., 2011, 40, 363–386 RSC;
(c) J. H. Jordan and B. C. Gibb, Chem. Soc. Rev., 2015, 44, 547–585 RSC;
(d) Y.-Y. Zhan and S. Hiraoka, Bull. Chem. Soc. Jpn., 2021, 94, 2329–2341 CrossRef CAS.
-
(a) K. Kondo, J. K. Klosterman and M. Yoshizawa, Chem. – Eur. J., 2017, 23, 16710–16721 CrossRef CAS PubMed;
(b) M. Yoshizawa and L. Catti, Proc. Jpn. Acad., Ser. B, 2023, 99, 29–38 CrossRef CAS PubMed.
- K. Kondo, A. Suzuki, M. Akita and M. Yoshizawa, Angew. Chem., Int. Ed., 2013, 52, 2308–2312 CrossRef CAS PubMed.
- Y. Okazawa, K. Kondo, M. Akita and M. Yoshizawa, J. Am. Chem. Soc., 2015, 137, 98–101 CrossRef CAS PubMed.
- Y. Satoh, L. Catti, M. Akita and M. Yoshizawa, J. Am. Chem. Soc., 2019, 141, 12268–12273 CrossRef CAS PubMed.
- Y. Katagiri, Y. Tsuchida, Y. Matsuo and M. Yoshizawa, J. Am. Chem. Soc., 2021, 143, 21492–21496 CrossRef CAS PubMed.
- A. Suzuki, K. Kondo, Y. Sei, M. Akita and M. Yoshizawa, Chem. Commun., 2016, 52, 3151–3154 RSC.
-
(a) L. S. Kaanumalle, C. L. D. Gibb, B. C. Gibb and V. Ramamurthy, J. Am. Chem. Soc., 2004, 126, 14366–14367 CrossRef CAS PubMed;
(b) L. S. Kaanumalle and V. Ramamurthy, Chem. Commun., 2007, 1062–1064 RSC;
(c) A. Natarajan, L. S. Kaanumalle, S. Jockusch, C. L. D. Gibb, B. C. Gibb, N. J. Turro and V. Ramamurthy, J. Am. Chem. Soc., 2007, 129, 4132–4133 CrossRef CAS PubMed;
(d) C. L. D. Gibb, A. K. Sundaresan, V. Ramamurthy and B. C. Gibb, J. Am. Chem. Soc., 2008, 130, 4069–4080 CrossRef CAS PubMed;
(e) R. Kulasekharan, M. V. S. N. Maddipatla, A. Parthasarthy and V. Ramamurthy, J. Org. Chem., 2013, 78, 942–949 CrossRef CAS PubMed;
(f) K. Wang, X. Cai, W. Yao, D. Tang, R. Kataria, H. S. Ashbaugh, L. D. Byers and B. C. Gibb, J. Am. Chem. Soc., 2019, 141, 6740–6747 CrossRef CAS PubMed . For reviews, see: ;
(g) V. Ramamurthy, Acc. Chem. Res., 2015, 48, 2904–2917 CrossRef CAS PubMed;
(h) V. Ramamurthy, Chem. Commun., 2022, 58, 6571–6585 RSC.
- For recent examples, see:
(a) A. Das, A. Danao, S. Banerjee, A. M. Raj, G. Sharma, R. Prabhakar, V. Srinivasan, V. Ramamurthy and P. Sen, J. Am. Chem. Soc., 2021, 143, 2025–2036 CrossRef CAS PubMed;
(b) C. J. Otolski, A. M. Raj, V. Ramamurthy and C. G. Elles, Chem. Sci., 2020, 11, 9513–9523 RSC.
-
(a) M. Frank, M. D. Johnstone and G. H. Clever, Chem. – Eur. J., 2016, 22, 14104–14125 CrossRef CAS PubMed;
(b) R. Zhu, J. Ding, L. Jin and H. Pang, Coord. Chem. Rev., 2019, 389, 119–140 CrossRef CAS;
(c) W.-X. Gao, H.-J. Feng, B.-B. Guo, Y. Lu and G.-X. Jin, Chem. Rev., 2020, 120, 6288–6325 CrossRef CAS PubMed.
-
(a) Y. Li, K. M. Mullen, T. D. W. Claridge, P. J. Costa, V. Felix and P. D. Beer, Chem. Commun., 2009, 7134–7136 RSC;
(b) D. Chakraborty, R. Modak, P. Howlader and P. S. Mukherjee, Chem. Commun., 2021, 57, 3995–3998 RSC;
(c) Y. Wu, Q.-H. Guo, Y. Qiu, J. A. Weber, R. M. Young, L. Bancroft, Y. Jiao, H. Chen, B. Song, W. Liu, Y. Feng, X. Zhao, X. Li, L. Zhang, X.-Y. Chen, H. Li, M. R. Wasielewski and J. F. Stoddart, Proc. Natl. Acad. Sci. U. S. A., 2022, 119, e2118573119 CrossRef CAS PubMed.
- M. Fujita, N. Fujita, K. Ogura and K. Yamaguchi, Nature, 1999, 400, 52–55 CrossRef CAS.
-
(a) A. Mishra, A. Dubey, J. W. Min, H. Kim, P. J. Stang and K.-W. Chi, Chem. Commun., 2014, 50, 7542 RSC;
(b) T. K. Ronson, Y. Wang, K. Baldridge, J. S. Siegel and J. R. Nitschke, J. Am. Chem. Soc., 2020, 142, 10267–10272 CrossRef CAS PubMed;
(c) Y.-W. Zhang, S. Bai, Y.-Y. Wang and Y.-F. Han, J. Am. Chem. Soc., 2020, 142, 13614–13621 CrossRef CAS PubMed;
(d) Y.-Y. Zhang, F.-Y. Qiu, H.-T. Shi and W. Yu, Chem. Commun., 2021, 57, 3010–3013 RSC.
-
(a) T. Hasell, X. Wu, J. T. A. Jones, J. Bacsa, A. Steiner, T. Mitra, A. Trewin, D. J. Adams and A. I. Cooper, Nat. Chem., 2010, 2, 750–755 CrossRef CAS PubMed;
(b) H. Li, H. Zhang, A. D. Lammer, M. Wang, X. Li, W. M. Lynch and J. L. Sessler, Nat. Chem., 2015, 7, 1003–1008 CrossRef CAS PubMed;
(c) Q. Wang, C. Yu, H. Long, Y. Du, Y. Jin and W. Zhang, Angew. Chem., Int. Ed., 2015, 54, 7550–7554 CrossRef CAS PubMed;
(d) D. Wang, L. Zhang and Y. Zhao, J. Org. Chem., 2022, 87, 2767–2772 CrossRef CAS PubMed.
- A. Westcott, J. Fisher, L. P. Harding, P. Rizkallah and M. J. Hardie, J. Am. Chem. Soc., 2008, 130, 2950–2951 CrossRef CAS PubMed.
-
(a) G. Zhang, O. Presly, F. White, I. M. Oppel and M. Mastalerz, Angew. Chem., Int. Ed., 2014, 53, 5126–5130 CrossRef CAS PubMed;
(b) P. Wagner, F. Rominger, J. H. Gross and M. Mastalerz, Angew. Chem., Int. Ed., 2023, 62, e202217251 CrossRef CAS PubMed.
-
(a) M. Fukuda, R. Sekiya and R. Kuroda, Angew. Chem., Int. Ed., 2008, 47, 706–710 CrossRef CAS PubMed;
(b) R. Sekiya, M. Fukuda and R. Kuroda, J. Am. Chem. Soc., 2012, 134, 10987–10997 CrossRef CAS PubMed.
- R. Sekiya, M. Fukuda and R. Kuroda, Org. Biomol. Chem., 2017, 15, 4328–4335 RSC.
-
(a) S. Freye, J. Hey, A. Torras-Galán, D. Stalke, R. Herbst-Irmer, M. John and G. H. Clever, Angew. Chem., Int. Ed., 2012, 51, 2191–2194 CrossRef CAS PubMed;
(b) S. Freye, R. Michel, D. Stalke, M. Pawliczek, H. Frauendorf and G. H. Clever, J. Am. Chem. Soc., 2013, 135, 8476–8479 CrossRef CAS PubMed;
(c) S. Löffler, J. Lübben, L. Krause, D. Stalke, B. Dittrich and G. H. Clever, J. Am. Chem. Soc., 2015, 137, 1060–1063 CrossRef PubMed.
- H.-M. Yu, M.-H. Du, J. Shu, Y.-H. Deng, Z.-M. Xu, Z.-W. Huang, Z. Zhang, B. Chen, P. Braunstein and J.-P. Lang, J. Am. Chem. Soc., 2023, 145, 25103–25108 CrossRef CAS PubMed.
-
(a) D. Chakraborty, R. Saha, J. K. Clegg and P. S. Mukherjee, Chem. Sci., 2022, 13, 11764–11771 RSC;
(b) D. Chakraborty, S. Pradhan, J. K. Clegg and P. S. Mukherjee, Inorg. Chem., 2024, 63, 14924–14932 CrossRef CAS PubMed.
- G. Gil-Ramírez, D. A. Leigh and A. J. Stephens, Angew. Chem., Int. Ed., 2015, 54, 6110–6150 CrossRef PubMed.
- M. Xue, Y. Yang, X. Chi, X. Yan and F. Huang, Chem. Rev., 2015, 115, 7398–7501 CrossRef CAS PubMed.
- For examples of the mechanical bond stabilising species, see:
(a) J. E. H. Buston, J. R. Young and H. L. Anderson, Chem. Commun., 2000, 905–906 RSC;
(b) J. E. H. Bouston, F. Marken and H. L. Anderson, Chem. Commun., 2001, 1046–1047 RSC;
(c) M. R. Craig, M. G. Hutchings, T. D. W. Claridge and H. L. Anderson, Angew. Chem., Int. Ed., 2001, 40, 1071–1074 CrossRef CAS;
(d) E. Arunkumar, C. C. Forbes, B. C. Noll and B. D. Smith, J. Am. Chem. Soc., 2005, 127, 3288–3289 CrossRef CAS PubMed;
(e) E. Arunkumar, N. Fu and B. D. Smith, Chem. – Eur. J., 2006, 12, 4467–4707 CrossRef PubMed;
(f) C. M. S. Yau, S. I. Pascu, S. A. Odom, J. E. Warren, E. J. F. Klotz, M. J. Frampton, C. C. Williams, V. Coropceanu, M. K. Kuimova, D. Phillips, S. Barlow, J.-L. Brédas, S. R. Marder, V. Millar and H. L. Anderson, Chem. Commun., 2008, 2897–2899 RSC;
(g) L. D. Movsisyan, D. V. Kondratuk, M. Franz, A. L. Thompson, R. R. Tykwinski and H. L. Anderson, Org. Lett., 2012, 14, 3424–3426 CrossRef CAS PubMed;
(h) M. Franz, J. A. Januszewski, D. Wendinger, C. Neiss, L. D. Movsisyan, F. Hampel, H. L. Anderson, A. Görling and R. R. Tykwinski, Angew. Chem., Int. Ed., 2015, 54, 6645–6649 CrossRef CAS PubMed;
(i) H. O. Bak, B. E. Nielsen, M. A. Petersen, A. Jeppesen, T. Brock-Nannestad, C. B. O. Nielsen and M. Pittelkow, New J. Chem., 2020, 44, 20930–20934 RSC;
(j) C. W. Patrick, J. F. Woods, P. Gawel, C. E. Otteson, A. L. Thompson, T. D. W. Claridge, R. Jasti and H. L. Anderson, Angew. Chem., Int. Ed., 2022, 61, e202116897 CrossRef CAS PubMed . For examples of the mechanical bond promoting unusual reactivity, see: ;
(k) A. Martinez-Cuezva, C. Lopez-Leonardo, D. Bautista, M. Alajarin and J. Berna, J. Am. Chem. Soc., 2016, 138, 8726–8729 CrossRef CAS PubMed;
(l) F. Modicom, E. M. G. Jamieson, E. Rochette and S. M. Goldup, Angew. Chem., Int. Ed., 2019, 58, 3875–3879 CrossRef CAS PubMed;
(m) B. Leforestier, M. R. Gyton and A. B. Chaplin, Angew. Chem., Int. Ed., 2020, 59, 23500–23504 CrossRef CAS PubMed;
(n) J. de Maria Perez, M. Alajarin, A. Martinez-Cuezva and J. Berna, Angew. Chem., Int. Ed., 2023, 62, e202302681 CrossRef CAS PubMed;
(o) M. J. Power, D. T. J. Morris, I. J. Vitorica-Yrezabal and D. A. Leigh, J. Am. Chem. Soc., 2023, 145, 8593–8599 CrossRef CAS PubMed.
-
(a) A. Garci, Y. Beldjoudi, M. S. Kodaimati, J. E. Hornick, M. T. Nguyen, M. M. Cetin, C. L. Stern, I. Roy, E. A. Weiss and J. F. Stoddart, J. Am. Chem. Soc., 2020, 142, 7956–7967 CrossRef CAS PubMed;
(b) S. Yu, A. Kupryakov, J. E. M. Lewis, V. Martí-Centelles, S. M. Goldup, J.-L. Pozzo, G. Jonusauskas and N. D. McClenaghan, Chem.
Sci., 2021, 12, 9196–9200 RSC;
(c) Y. Deng, S. K.-M. Lai, L. Kong and H. Y. Au-Yeung, Chem. Commun., 2021, 57, 2931–2934 RSC;
(d) A. Garci, J. A. Weber, R. M. Young, M. Kazem-Rostami, M. Ovalle, Y. Beldjoudi, A. Atilgan, Y. J. Bae, W. Liu, L. O. Jones, C. L. Stern, G. C. Schatz, O. K. Farha, M. R. Wasielewski and J. F. Stoddart, Nat. Catal., 2022, 5, 524–533 CrossRef CAS;
(e) N. Hoyas Pérez, P. S. Sherin, V. Posligua, J. L. Greenfield, M. J. Fuchter, K. E. Jelfs, M. K. Kuimova and J. E. M. Lewis, Chem. Sci., 2022, 13, 11368–11375 RSC;
(f) A. Garci, A. H. G. David, L. Le Bras, M. Ovalle, S. Abid, R. M. Young, W. Liu, C. S. Azad, P. J. Brown, M. R. Wasielewski and J. F. Stoddart, J. Am. Chem. Soc., 2022, 144, 23551–23559 CrossRef CAS PubMed;
(g) A. Garci, S. Abid, A. H. G. David, L. O. Jones, C. S. Azad, M. Ovalle, P. J. Brown, C. L. Stern, X. Zhao, L. Malaisrie, G. C. Schatz, R. M. Young, M. R. Wasielewski and J. F. Stoddart, J. Am. Chem. Soc., 2023, 145, 18391–18401 CrossRef CAS PubMed.
- A. Acevedo-Jake, A. T. Ball, M. Galli, M. Kukwikila, M. Denis, D. G. Singleton, A. Tavassoli and S. M. Goldup, J. Am. Chem. Soc., 2020, 142, 5985–5990 CrossRef CAS PubMed.
-
(a) M. Galli, J. E. M. Lewis and S. M. Goldup, Angew. Chem., Int. Ed., 2015, 54, 13545–13549 CrossRef CAS PubMed;
(b) K. Eichstaedt, J. Jaramillo-Garcia, D. A. Leigh, V. Marcos, S. Pisano and T. A. Singleton, J. Am. Chem. Soc., 2017, 139, 9376–9381 CrossRef CAS PubMed;
(c) A. Bessaguet, Q. Blancart-Remaury, P. Poinot, I. Opalinski and S. Papot, Angew. Chem., Int. Ed., 2023, 62, e202216787 CrossRef CAS PubMed.
-
(a) A. Fernandes, A. Viterisi, F. Coutrot, S. Potok, D. A. Leigh, V. Aucagne and S. Papot, Angew. Chem., Int. Ed., 2009, 48, 6443–6447 CrossRef CAS PubMed;
(b) R. Barat, T. Legigan, I. Tranoy-Opalinski, B. Renoux, E. Péraudeau, J. Clarhaut, P. Poinot, A. E. Fernandes, V. Aucagne, D. A. Leigh and S. Papot, Chem. Sci., 2015, 6, 2608–2613 RSC;
(c) T. Kench, P. A. Summers, M. K. Kuimova, J. E. M. Lewis and R. Vilar, Angew. Chem., Int. Ed., 2021, 60, 10928–10934 CrossRef CAS PubMed.
- G. Barin, A. Coskun, M. M. G. Fouda and J. F. Stoddart, ChemPlusChem, 2012, 77, 159–185 CrossRef CAS; N. H. Evans and P. D. Beer, Chem. Soc. Rev., 2014, 43, 4658–4683 RSC; N. H. Evans, Eur. J. Org. Chem., 2019, 3320–3343 CrossRef; N. Hoyas Pérez and J. E. M. Lewis, Org. Biomol. Chem., 2020, 18, 6757–6780 RSC; K. Cai, L. Zhang, R. D. Astumian and J. F. Stoddart, Nat.
Rev. Chem., 2021, 5, 447–465 CrossRef PubMed; Q. Chen and K. Zhu, Chem. Soc. Rev., 2024, 53, 5677–5703 RSC; S. D. P. Fielden, ChemSystemsChem, 2024, 6, e202300048 CrossRef.
-
(a) J. D. Crowley, S. M. Goldup, A.-L. Lee, D. A. Leigh and R. T. McBurney, Chem. Soc. Rev., 2009, 38, 1530–1541 RSC;
(b) J. E. M. Lewis, P. D. Beer, S. J. Loeb and S. M. Goldup, Chem. Soc. Rev., 2017, 46, 2577–2591 RSC;
(c) M. Denis and S. M. Goldup, Nat. Rev. Chem., 2017, 1, 0061 CrossRef CAS;
(d) J.-P. Sauvage, Angew. Chem., Int. Ed., 2017, 56, 11080–11093 CrossRef CAS PubMed.
-
(a) J. E. M. Lewis, M. Galli and S. M. Goldup, Chem. Commun., 2017, 53, 298–312 RSC . SCXRD structure of Cu(I) complex of a [2]catenane from: ;
(b) M. Cesario, C. O. Dietrich-Buchecker, J. Guilhem, C. Pascard and J. P. Sauvage, J. Chem. Soc., Chem. Commun., 1985, 244–247 RSC . SCXRD structure of Zn(II) complex of a [2]rotaxane from: ;
(c) M. Cirulli, A. Kaur, J. E. M. Lewis, Z. Zhang, J. A. Kitchen, S. M. Goldup and M. M. Roessler, J. Am. Chem. Soc., 2019, 141, 879–889 CrossRef CAS PubMed.
-
(a) C. O. Dietrich-Buchecker and J.-P. Sauvage, J. Am. Chem. Soc., 1984, 106, 3043–3045 CrossRef CAS;
(b) A.-M. Albrecht-Gary, Z. Saad, C. O. Dietrich-Buchecker and J.-P. Sauvage, J. Am. Chem. Soc., 1985, 107, 3205–3209 CrossRef CAS.
-
(a) C. O. Dietrich-Buchecker, J.-M. Kern and J.-P. Sauvage, J. Chem. Soc., Chem. Commun., 1985, 760–762 RSC;
(b) C. Dietrich-Buchecker, J.-P. Sauvage and J.-M. Kern, J. Am. Chem. Soc., 1989, 111, 7791–7800 CrossRef CAS;
(c) C. O. Dietrich-Buchecker, J. Guilhem, J.-M. Kern, C. Pascard and J.-P. Sauvage, Inorg. Chem., 1994, 33, 3498–3502 CrossRef CAS;
(d) D. A. Leigh, P. J. Lusby, A. M. Z. Slawin and D. B. Walker, Angew. Chem., Int. Ed., 2005, 44, 4557–4564 CrossRef CAS PubMed;
(e) J. Winn, A. Pinczewska and S. M. Goldup, J. Am. Chem. Soc., 2013, 135, 13318–13321 CrossRef CAS PubMed;
(f) G. Baggi and S. J. Loeb, Chem. – Eur. J., 2017, 23, 14163–14166 CrossRef CAS PubMed.
-
(a) M. J. Langton and P. D. Beer, Acc. Chem. Res., 2014, 47, 1935–1949 CrossRef CAS PubMed;
(b) K. Hiratani, M. Kaneyama, Y. Nagawa, E. Koyama and M. Kanesato, J. Am. Chem. Soc., 2004, 126, 13568–13569 CrossRef CAS PubMed;
(c) P. H. Kwan and T. M. Swager, J. Am. Chem. Soc., 2005, 127, 5902–5909 CrossRef CAS PubMed;
(d) N.-C. Chen, P.-Y. Huang, C.-C. Lai, Y.-H. Liu, Y. Wang, S.-M. Peng and S.-H. Chiu, Chem. Commun., 2007, 4122–4124 RSC;
(e) M. Denis, J. Pancholi, K. Jobe, M. Watkinson and S. M. Goldup, Angew. Chem., Int. Ed., 2018, 57, 5310–5314 CrossRef CAS PubMed;
(f) S. Bej, M. Nandi and P. Ghosh, Dalton Trans., 2021, 50, 294–303 RSC;
(g) S. Bej, M. Nandi and P. Ghosh, Org. Biomol. Chem., 2022, 20, 7284–7293 RSC.
-
(a) P. D. Beer, M. R. Sambrook and D. Curiel, Chem. Commun., 2006, 2105–2117 RSC;
(b) G. T. Spence and P. D. Beer, Acc. Chem. Res., 2013, 46, 571–586 CrossRef CAS PubMed.
- SCXRD structure of Cl− bound in a [2]catenane from:
(a) M. R. Sambrook, P. D. Beer, J. A. Wisner, R. L. Paul and A. R. Cowley, J. Am. Chem. Soc., 2004, 126, 15364–15365 CrossRef CAS PubMed . SCXRD structure of Br− bound in a [2]rotaxane from: ;
(b) N. G. White, A. R. Colaço, I. Marques, V. Félix and P. D. Beer, Org. Biomol. Chem., 2014, 12, 4924–4931 RSC.
- A. Docker, Y. C. Tse, H. M. Tay, A. J. Taylor, Z. Zhang and P. D. Beer, Angew. Chem., Int. Ed., 2022, 61, e202214523 CrossRef CAS PubMed.
- T. A. Barendt, A. Docker, I. Marques, V. Félix and P. D. Beer, Angew. Chem., Int. Ed., 2016, 55, 11069–11076 CrossRef CAS PubMed.
- B. Huang, S. M. Santos, V. Felix and P. D. Beer, Chem. Commun., 2008, 4610–4612 RSC.
-
(a) M. J. Langton, S. W. Robinson, I. Marques, V. Félix and P. D. Beer, Nat. Chem., 2014, 6, 1039–1043 CrossRef CAS PubMed;
(b) C. J. Serpell, A. Y. Park, C. V. Robinson and P. D. Beer, Chem. Commun., 2021, 57, 101–104 RSC.
- R. S. Forgan, J. J. Gassensmith, D. B. Cordes, M. M. Boyle, K. J. Hartlieb, D. C. Friedman, A. M. Z. Slawin and J. F. Stoddart, J. Am. Chem. Soc., 2012, 134, 17007–17010 CrossRef CAS PubMed.
- G. Barin, M. Frasconi, S. M. Dyar, J. Iehl, O. Buyukcakir, A. A. Sarjeant, R. Carmieli, A. Coskun, M. R. Wasielewski and J. F. Stoddart, J. Am. Chem. Soc., 2013, 135, 2466–2469 CrossRef CAS PubMed.
- A. L. Hubbard, G. J. E. Davidson, R. H. Patel, J. A. Wisner and S. J. Loeb, Chem. Commun., 2004, 138–139 RSC.
-
(a) I. Smukste, B. E. House and D. B. Smithrud, J. Org. Chem., 2003, 68, 2559–2571 CrossRef CAS PubMed;
(b) V. Dvornikovs, B. E. House, M. Kaetzel, J. R. Dedman and D. B. Smithrud, J. Am. Chem. Soc., 2003, 125, 8290–8301 CrossRef CAS PubMed;
(c) X. Wang, X. Bao, M. McFarland-Mancini, I. Isaacsohn, A. F. Drew and D. B. Smithrud, J. Am. Chem. Soc., 2007, 129, 7284–7293 CrossRef CAS PubMed;
(d) X. Bao, I. Isaacsohn, A. F. Drew and D. B. Smithrud, J. Org. Chem., 2007, 72, 3988–4000 CrossRef CAS PubMed.
- R. Mitra, M. Thiele, F. Octa-Smolin, M. C. Letzel and J. Niemeyer, Chem. Commun., 2016, 52, 5977–5980 RSC.
- J. Y. C. Lim, I. Marques, V. Félix and P. D. Beer, Angew. Chem., Int. Ed., 2018, 57, 584–588 CrossRef CAS PubMed.
- J.-S. Marois, K. Cantin, A. Desmarais and J.-F. Morin, Org. Lett., 2008, 10, 33–36 CrossRef CAS PubMed.
- J. T. Wilmore and P. D. Beer, Adv. Mater., 2024, 36, 2309098 CrossRef CAS PubMed.
- A. Saura-Sanmartin, Chem. – Eur.
J., 2024, 30, e202304025 CrossRef CAS PubMed.
- M. A. Nosiglia, N. D. Colley, M. K. Danielson, M. S. Palmquist, A. O. Delawder, S. L. Tran, G. H. Harlan and J. C. Barnes, J. Am. Chem. Soc., 2022, 144, 9990–9996 CrossRef CAS PubMed.
-
(a) A. Caballero, F. Zapata and P. D. Beer, Coord. Chem. Rev., 2013, 257, 2434–2455 CrossRef CAS;
(b) M. J. Langton and P. D. Beer, Acc. Chem. Res., 2014, 47, 1935–1949 CrossRef CAS PubMed.
- H. M. Tay, T. G. Johnson, A. Docker, M. J. Langton and P. D. Beer, Angew. Chem., Int. Ed., 2023, 62, e2023312745 Search PubMed.
-
(a) A. Martinez-Cuezva, A. Saura-Sanmartin, M. Alajarin and J. Berna, ACS Catal., 2020, 10, 7719–7733 CrossRef CAS;
(b) C. Kwamen and J. Niemeyer, Chem. – Eur. J., 2021, 27, 175–186 CrossRef CAS PubMed.
-
(a) V. Balzani, A. Credi, F. M. Raymo and J. F. Stoddart, Angew. Chem., Int. Ed., 2000, 39, 3348–3391 CrossRef CAS;
(b) W. R. Browne and B. L. Feringa, Nat. Nanotechnol., 2006, 1, 25–35 CrossRef CAS PubMed;
(c) V. Balzani, A. Credi and M. Venturi, Chem. Soc. Rev., 2009, 38, 1542–1550 RSC;
(d) E. R. Kay and D. A. Leigh, Angew. Chem., Int. Ed., 2015, 54, 10080–10088 CrossRef CAS PubMed;
(e) S. Erbas-Cakmak, D. A. Leigh, C. T. McTernan and A. L. Nussbaumer, Chem. Rev., 2015, 115, 10081–10206 CrossRef CAS PubMed;
(f) S. Kassem, T. van Leeuwen, A. S. Lubbe, M. R. Wilson, B. L. Feringa and D. A. Leigh, Chem. Soc. Rev., 2017, 46, 2592–2621 RSC;
(g) L. Zhang, V. Marcos and D. A. Leigh, Proc. Natl. Acad. Sci. U. S. A., 2018, 115, 9397–9404 CrossRef CAS PubMed;
(h) A. W. Heard and S. M. Goldup, ACS Cent. Sci., 2020, 6, 117–128 CrossRef CAS PubMed;
(i) I. Aprahamian, ACS Cent. Sci., 2020, 6, 347–358 CrossRef CAS PubMed;
(j) Y. Feng, M. Ovalle, J. S. W. Seale, C. K. Lee, D. J. Kim, R. D. Astumian and J. F. Stoddart, J. Am. Chem. Soc., 2021, 143, 5569–5591 CrossRef CAS PubMed.
-
(a) D. J. Hill, M. J. Mio, R. B. Prince, T. S. Hughes and J. S. Moore, Chem. Rev., 2001, 101, 3893–4011 CrossRef CAS PubMed;
(b) I. Saraogi and A. D. Hamilton, Chem. Soc. Rev., 2009, 38, 1726–1743 RSC;
(c) G. Guichard and I. Huc, Chem. Commun., 2011, 47, 5933–5941 RSC.
- H. Juwarker, J. Suk and K.-S. Jeong, Chem. Soc. Rev., 2009, 38, 3316–3325 RSC.
- T. A. Martinek and F. Fülöp, Chem. Soc. Rev., 2012, 41, 687–702 RSC.
- J. C. Nelson, J. G. Saven, J. S. Moore and P. G. Wolynes, Science, 1997, 277, 1793–1796 CrossRef CAS PubMed.
- E. A. John, A. M. S. Riel, L. H. E. Wieske, D. Ray, D. A. Decato, M. Boller, Z. Takacs, M. Erdélyi, V. S. Bryantsev and O. B. Berryman, J. Am. Chem. Soc., 2024, 146, 16419–16427 CrossRef CAS PubMed.
-
(a) I. Huc, Eur. J. Org. Chem., 2004, 17–29 CrossRef CAS;
(b) D.-W. Zhang, X. Zhao, J.-L. Hou and Z.-T. Li, Chem. Rev., 2012, 112, 5271–5316 CrossRef CAS PubMed;
(c) D.-W. Zhang, X. Zhao and Z.-T. Li, Acc. Chem. Res., 2014, 47, 1961–1970 CrossRef CAS PubMed;
(d) Y. Ferrand and I. Huc, Acc. Chem. Res., 2018, 51, 970–977 CrossRef CAS PubMed.
- Y. Hamuro, S. J. Geib and A. D. Hamilton, J. Am. Chem. Soc., 1996, 118, 7529–7541 CrossRef CAS.
- L. Fischer and G. Guichard, Org. Biomol. Chem., 2010, 8, 3101–3117 RSC.
- L. Fischer, P. Claudon, N. Pendem, E. Miclet, C. Didierjean, E. Ennifar and G. Guichard, Angew. Chem., Int. Ed., 2010, 49, 1067–1070 CrossRef CAS PubMed.
-
(a) H. Juwarker, J. M. Lenhardt, D. M. Pham and S. L. Craig, Angew. Chem., Int. Ed., 2008, 47, 3740–3743 CrossRef CAS PubMed;
(b) R. M. Meudtner and S. Hecht, Angew. Chem., Int. Ed., 2008, 47, 4926–4930 CrossRef CAS PubMed.
- Y. Liu, F. C. Parks, E. G. Sheetz, C.-H. Chen and A. H. Flood, J. Am. Chem. Soc., 2021, 143, 3191–3204 CrossRef CAS PubMed.
-
(a) J. K. Murray and S. H. Gellman, Org. Lett., 2005, 7, 1517–1520 CrossRef CAS PubMed;
(b) H. M. König, R. Abbel, D. Schollmeyer and A. F. M. Kilbinger, Org. Lett., 2006, 8, 1819–1822 CrossRef PubMed;
(c) B. Baptiste, C. Douat-Casassus, K. Laxmi-Reddy and I. Huc, J. Org. Chem., 2010, 75, 7175–7185 CrossRef CAS PubMed;
(d) F. Campbell, J. P. Plante, T. A. Edwards, S. L. Warriner and A. J. Wilson, Org. Biomol. Chem., 2010, 8, 2344–2351 RSC;
(e) C. Douat-Casassus, K. Pulka, P. Claudon and G. Guichard, Org. Lett., 2012, 14, 3130–3133 CrossRef CAS PubMed;
(f) N. S. Murphy, P. Prabhakarn, V. Azzarito, J. P. Plante, M. J. Hardie, C. A. Kilner, S. L. Warriner and A. J. Wilson, Chem. – Eur. J., 2013, 19, 5546–5550 CrossRef CAS PubMed;
(g) V. Corvaglia, F. Sanchez, F. S. Menke, C. Douat and I. Huc, Chem. – Eur. J., 2023, 29, e202300898 CrossRef CAS PubMed.
-
(a) D. J. Hill and J. S. Moore, Proc. Natl. Acad. Sci. U. S. A., 2002, 99, 5053–5057 CrossRef CAS PubMed;
(b) T. Qi, V. Maurizot, H. Noguchi, T. Charoenraks, B. Kauffmann, M. Takafuji, H. Ihara and I. Huc, Chem. Commun., 2012, 48, 6337–6339 RSC;
(c) G. N. Vemuri, M. Chu, H. Dong, B. J. Spinello and C. S. Hartley, Org. Biomol. Chem., 2017, 15, 845–851 RSC;
(d) S. Debnath, A. H. Flood and K. Raghavachari, J. Phys. Chem. B, 2024, 128, 1586–1594 CrossRef CAS PubMed.
- J. M. Cary and J. S. Moore, Org. Lett., 2002, 4, 4663–4666 CrossRef CAS PubMed; J. M. Heemstra and J. S. Moore, Chem. Commun., 2004, 1480–1481 RSC; X. Yang, L. Yuan, K. Yamato, A. L. Brown, W. Feng, M. Furukawa, X. C. Zheng and B. Gong, J. Am. Chem. Soc., 2004, 126, 3148–3162 CrossRef PubMed.
-
(a) H. Juwarker and K.-S. Jeong, Chem. Soc. Rev., 2010, 39, 3664–3674 RSC;
(b) E. A. John, C. J. Massena and O. B. Berryman, Chem. Rev., 2020, 120, 2759–2782 CrossRef CAS PubMed.
-
(a) S. R. Rao, S. L. Schettler and W. S. Horne, ChemPlusChem, 2021, 86, 137–145 CrossRef CAS PubMed;
(b) J. L. Algar, J. A. Findlay and D. Preston, ACS Org. Inorg. Au, 2022, 2, 464–476 CrossRef CAS PubMed.
-
(a) J. Garric, J.-M. Léger and I. Huc, Angew. Chem., Int. Ed., 2004, 44, 1954–1958 CrossRef PubMed;
(b) J. Garric, J.-M. Léger and I. Huc, Chem. – Eur. J., 2007, 13, 8454–8462 CrossRef CAS PubMed;
(c) C. Bao, B. Kauffmann, Q. Gan, K. Srinivas, H. Jiang and I. Huc, Angew. Chem., Int. Ed., 2008, 47, 4153–4156 CrossRef CAS PubMed.
- R. B. Prince, S. A. Barnes and J. S. Moore, J. Am. Chem. Soc., 2000, 122, 2758–2762 CrossRef CAS.
-
(a) J.-L. Hou, X.-B. Shao, G.-J. Chen, Y.-X. Zhou, X.-K. Jiang and Z.-T. Li, J. Am. Chem. Soc., 2004, 126, 12386–12394 CrossRef CAS PubMed;
(b) Y. Ohishi, H. Abe and M. Inouye, Eur. J. Org. Chem., 2017, 6975–6979 CrossRef CAS;
(c) J. Y. Hwang, H.-G. Jeon, Y. R. Choi, J. Kim, P. Kang, S. Lee and K.-S. Jeong, Org. Lett., 2017, 19, 5625–5628 CrossRef CAS PubMed . SCXRD structure shown in Fig. 22b from: ;
(d) S. Saha, B. Kauffmann, Y. Ferrand and I. Huc, Angew. Chem., Int. Ed., 2018, 57, 13542–13546 CrossRef CAS PubMed.
-
(a) C. Li, G.-T. Wang, H.-P. Yi, X.-K. Jiang, Z.-T. Li and R.-X. Wang, Org. Lett., 2007, 9, 1797–1800 CrossRef CAS PubMed;
(b) Y. Ferrand, A. M. Kendhale, B. Kauffmann, A. Grélard, C. Marie, V. Blot, M. Pipelier, D. Dubreuil and I. Huc, J. Am. Chem. Soc., 2010, 132, 7858–7859 CrossRef CAS PubMed;
(c) Y. Ferrand, N. Chandramouli, A. M. Kendhale, C. Aube, B. Kauffmann, A. Grélard, M. Laguerre, D. Dubreuil and I. Huc, J. Am. Chem. Soc., 2012, 134, 11282–11288 CrossRef CAS PubMed.
- P. Mateus, N. Chandramouli, C. D. Mackereth, B. Kauffmann, Y. Ferrand and I. Huc, Angew. Chem., Int. Ed., 2020, 59, 5797–5805 CrossRef CAS PubMed.
-
(a) V. Koehler, A. Roy, I. Huc and Y. Ferrand, Acc. Chem. Res., 2022, 55, 1074–1085 CrossRef CAS PubMed;
(b) A. Tanatani, T. S. Hughes and J. S. Moore, Angew. Chem., Int. Ed., 2002, 41, 325–328 CrossRef CAS;
(c) A. Petitjean, L. A. Cuccia, M. Schmutz and J.-M. Lehn, J. Org. Chem., 2008, 73, 2481–2495 CrossRef CAS PubMed . SCXRD structure shown in Fig. 22d from: ;
(d) Q. Gan, Y. Ferrand, C. Bao, B. Kauffman, A. Grélard, H. Jiang and I. Huc, Science, 2011, 331, 1172–1175 CrossRef CAS PubMed.
- R. Gopalakrishnan, A. I. Frolov, L. Knerr, W. J. Drury III and E. Valeur, J. Med. Chem., 2016, 59, 9599–9621 CrossRef CAS PubMed.
- Z. C. Girvin and S. H. Gellman, J. Am. Chem. Soc., 2020, 142, 17211–17223 CrossRef CAS PubMed.
- N. Chandramouli, Y. Ferrand, G. Lautrette, B. Kauffmann, C. D. Mackereth, M. Laguerre, D. Dubreuil and I. Huc, Nat. Chem., 2015, 7, 334–341 CrossRef CAS PubMed.
- P. Mateus, A. Jacquet, A. Méndez-Ardoy, A. Boulloy, B. Kauffmann, G. Pecastaings, T. Buffeteau, Y. Ferrand, D. M. Bassani and I. Huc, Chem. Sci., 2021, 12, 3743 RSC.
-
(a) G. Song and K.-S. Jeong, ChemPlusChem, 2020, 85, 2475–2481 CrossRef CAS PubMed;
(b) Y. Ohishi, T. Takata and M. Inouye, ChemPlusChem, 2020, 85, 2565–2569 CrossRef CAS PubMed;
(c) C. Ma, J. Tang, L. Yu, K. Wen and Q. Gan, Chem. – Eur. J., 2022, 28, e202200834 CrossRef CAS PubMed.
- Y. Ohishi, H. Abe and M. Inouye, Chem. – Eur. J., 2015, 21, 16504–16511 CrossRef CAS PubMed.
- A. Meeunier, M. L. Singleton, B. Kauffmann, T. Granier, G. Lautrette, Y. Ferrand and I. Huc, Chem. Sci., 2020, 11, 12178–12186 RSC.
-
(a) L. Turcani, A. Tarzia, F. Szczypiński and K. E. Jelfs, J. Chem. Phys., 2021, 154, 214102 CrossRef CAS PubMed;
(b) T. A. Young, R. Gheorghe and F. Duarte, J. Chem. Inf. Model., 2020, 60, 3546–3557 CrossRef CAS PubMed . For reviews on computational exploration of chemical space related to capsules, see: ;
(c) A. Tarzia and K. E. Jelfs, Chem. Commun., 2022, 58, 3717–3730 RSC;
(d) T. K. Pizkorz, V. Martí-Centelles, T. A. Young, P. J. Lusby and F. Duarte, ACS Catal., 2022, 12, 5806–5826 CrossRef PubMed;
(e) K. E. Jelfs, Ann. N. Y. Acad. Sci., 2022, 1518, 106–119 CrossRef PubMed.
- M. Yamashina, Y. Tanaka, R. Lavendomme, T. K. Ronson, M. Pittelkow and J. R. Nitschke, Nature, 2019, 574, 511–515 CrossRef CAS PubMed.
Footnote |
† These authors contributed equally and are listed in alphabetical order. |
|
This journal is © The Royal Society of Chemistry 2024 |
Click here to see how this site uses Cookies. View our privacy policy here.