DOI:
10.1039/D3CS00723E
(Viewpoint)
Chem. Soc. Rev., 2024,
53, 2258-2263
Hydrogen energy futures – foraging or farming?
Received
9th October 2023
First published on 7th February 2024
Abstract
Exploration for commercially viable natural hydrogen accumulations within the Earth's crust, here compared to ‘foraging’ for wild food, holds promise. However, a potentially more effective strategy lies in the in situ artificial generation of hydrogen in natural underground reservoirs, akin to ‘farming’. Both biotic and abiotic processes can be employed, converting introduced or indigenous components, gases, and nutrients into hydrogen. Through studying natural hydrogen-generating reactions, we can discern pathways for optimized engineering. Some reactions may be inherently slow, allowing for a ‘seed and leave’ methodology, where sites are infused with gases, nutrients, and specific bacterial strains, then left to gradually produce hydrogen. However, other reactions could offer quicker outcomes to harvest hydrogen. A crucial element of this strategy is our innovative concept of ‘X’ components—ranging from trace minerals to bioengineered microbes. These designed components enhance biotic and/or abiotic reactions and prove vital in accelerating hydrogen production. Drawing parallels with our ancestors' transition from hunter-gathering to agriculture, we propose a similar paradigm shift in the pursuit of hydrogen energy. As we transition towards a hydrogen-centric energy landscape, the amalgamation of geochemistry, advanced biology, and engineering emerges as a beacon, signalling a pathway towards a sustainable and transformative energy future.
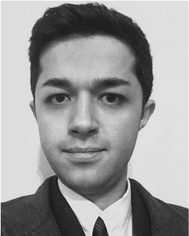
Aliakbar Hassanpouryouzband
| Aliakbar Hassanpouryouzband is Chancellor's Fellow in Net Zero Technologies (since 2023) at the School of Geosciences, University of Edinburgh. He started his journey there in 2019 as a postdoctoral research associate. His research focuses on CCUS and large-scale sustainable energy generation, transport, and storage with particular focus on hydrogen. He completed his PhD at Heriot-Watt University in 2019, specializing in low-carbon energy production and CO2 capture and storage, a part of which was conducted at MIT's Molecular Engineering Laboratory. Aliakbar received his BSc (2013) and MSc (2015) in Petroleum Engineering from Sharif University of Technology. |
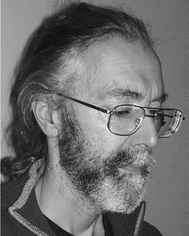
Mark Wilkinson
| Mark Wilkinson studied geology at the University of Oxford, UK, followed by a PhD at the University of Leicester, UK, about geochemistry of sedimentary rocks. He is Senior Lecturer in Geological CO2 Storage at the University of Edinburgh, UK. Recent papers focus on geological carbon storage and underground hydrogen storage, to decarbonise energy and industrial activity. Mark co-created the Masters in GeoEnergy degree at the University of Edinburgh, a World-first at the time. He teaches field geology and energy-related applied geology, and has co-created a Massive Open Online Course (MOOC) about CCS and climate change. |
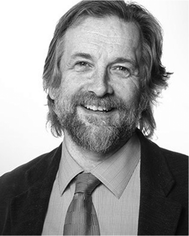
R. Stuart Haszeldine
| R. Stuart Haszeldine is Professor of Carbon Capture and Storage at the University of Edinburgh, Scotland. He is the Director of SCCS. He has 45 years experience working with subsurface information from hydrocarbon extraction to waste disposal. His University group focusses on CO2 storage geology, with a particular emphasis on natural analogues, containment processes and subsurface pressures. Stuart was awarded the Scottish Science Prize in 1999, elected Fellow of the Royal Society of Edinburgh in 2003, appointed OBE for services to climate change technologies in 2012, and in 2021 he was awarded the Energy Medal by the Geological Society of London. |
Navigating the hydrogen energy landscape
The aim of keeping global warming within 2 °C of pre-industrial levels must be supported by robust short-term actions.1 This challenge calls for innovative energy strategies, where hydrogen's crucial role in reducing carbon emissions and advancing sustainable energy practices becomes apparent. Our previous work has spotlighted hydrogen as a viable, large-scale, low-carbon, acceptable cost solution for combating climate change, with its potential for terawatt-hour scale storage in suitable geological formations.2 Yet, significant hurdles remain in incorporating hydrogen into our energy systems.3 Despite extensive research in this field, scalable and low-cost production of hydrogen remain key obstacles, highlighting the need for innovative approaches explored in this paper. The primary challenges include the considerable electricity use required to produce renewable hydrogen, or the carbon dioxide emissions linked to hydrogen production by the reforming of fossil hydrocarbons. To effectively tackle these challenges, a dual approach is essential: enhancing existing hydrogen production methods while exploring innovative techniques for hydrogen generation. Hydrogen can be produced through various methods, each characterized by a distinct 'colour' nomenclature based on the process employed (see Fig. 1, Comprehensive details on additional hydrogen production methods are available in other dedicated sources4). Currently, grey and black hydrogen account for over 95% of the engineered global output.5 These methods are relatively low cost, but have significant CO2 emissions and minimal climate benefit. Production is gradually shifting towards green hydrogen, with rising output and concurrently decreasing costs.5 This transition might also carry a secondary benefit – the decrease of carbon dioxide emissions from grey hydrogen production, through grey hydrogen utilisation as feedstock for generation of carbon by-products.6 Thus, a future can be envisioned where our energy systems are supplemented by both green hydrogen and carbon-mitigated grey hydrogen production, promising pathways that do not contribute to further global warming. The broadening of hydrogen production methodologies marks a significant step towards a sustainable energy future, moving beyond traditional approaches. However, such a promising transition demands careful planning of improved processes at large scale and low cost, reliability – and time.7
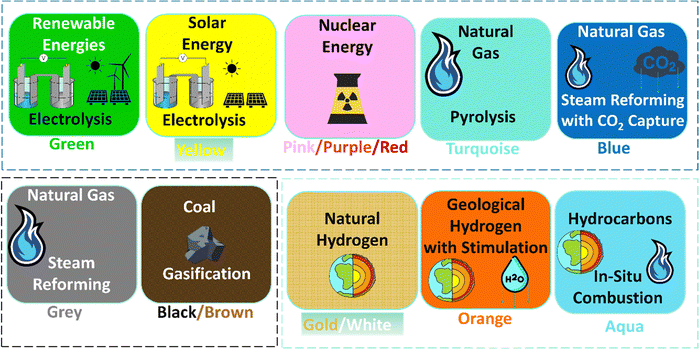 |
| Fig. 1 Classification of Hydrogen Production Techniques: This schematic provides a systematic overview of hydrogen production methodologies, each distinguished by a specific colour based on source and process. The utilization of these colour designations is reflective of an evolving categorization in the field, summarizing the technological and environmental implications associated with each production route. As the quest for sustainable hydrogen intensifies, a clear understanding of these diverse production pathways becomes crucial for both research and application. | |
As we consider the technological advancements in hydrogen production, it is equally important to recognize the critical role of policy frameworks. These policies are instrumental in shaping the hydrogen economy, driving technological innovation, and guiding the transition towards more sustainable practices. Europe's pioneering efforts in implementing carbon taxes8 offer potential for green hydrogen to be economically competitive, because costs are levied on the emissions of fossil carbon. Yet, it is critical to scrutinize such strategies. While they may incentivize the market towards greener alternatives, they do not inherently improve energy efficiency. Green hydrogen production remains energy-intensive, heavily dependent on renewable electricity, which might otherwise be directly supplied to the grid. A notable exception could be the utilization of surplus renewable electricity that would otherwise be wasted, at times of weather related over-production, or gaps in transmission due to engineering outages. In such scenarios, despite lower efficiencies, producing green hydrogen might be a more favourable action than spilled electricity, or zero output for a national energy network.9 However, this situation represents a niche scenario which is unlikely to last into future decades
Hydrogen exploration (foraging)
The cost of hydrogen production must be reduced. But is the remedy already known, and hiding in plain sight? A recent survey of natural hydrogen-containing gases worldwide10 lists hundreds of known natural hydrogen sites, and makes the case that many analyses of natural gases have failed to detect hydrogen, due to the inappropriate methods deployed. The highest natural hydrogen concentrations reported are 99%11 (Oman) and over 97%12 (Mali). However, high maximum figures can disguise much lower averages – many of the reported numbers are around 10%. Moreover, a resource must be present in sufficiently large volumes to be exploitable. The flow rates of natural hydrogen-rich gas seeps are generally low. Though dispersed seepage may sum to a large quantity it may be impossible to exploit resources commercially. For example, the hydrogen flux at Kryvyi Rih, Ukraine, was estimated to be 120
000 m3 per day13 but at only 10–120 cm3 per year per m2
14 is far too dispersed to be commercially exploitable. The number of known high concentration, high volume hydrogen sites is very small, with Zgonnik (2020)10 listing only 16 hydrocarbon fields with more than 10% hydrogen worldwide, compared to thousands of oil and gas fields. The limited availability of high-concentration, high-volume hydrogen sites presents a significant challenge, underscoring the need for advanced exploration techniques and innovative strategies to identify and capitalize on these resources. The low concentrations of hydrogen will also provide a barrier – removing the 10–90% non-hydrogen gas may be commercially unfeasible, and if the non-hydrogen gas is methane or other hydrocarbons, will this really be disposed of (e.g. re-injected into the subsurface) rather than burned, with the associated carbon emissions?
Hydrogen engineering (farming)
The natural production of hydrogen is substantial, with estimates of worldwide production of around 23 Tg per year10 but with high uncertainty.15,16 The true extent of this natural resource remains to be thoroughly mapped, but preliminary investigations already highlight the importance of continued exploration. If substantial reserves are identified, they could serve as a valuable bridge,17 providing energy for many decades. This potential boon, however, should be seen in conjunction with the ever-present imperative to also prioritize and develop sustainable and renewable hydrogen production methods. This ensures we have a robust, long-term energy strategy in place. Effecting this transformative shift calls for strategic foresight, innovative thinking, and time. This leads us to propose that subsurface geological formations could be engineered and exploited for artificial hydrogen production. Leveraging these natural repositories could significantly offset the high costs associated with current production methods, paving the way towards a more sustainable energy future.
Understanding this transition can be aided by drawing parallels with a historical precedent, notably the evolution of human culture from hunter-gathering to agriculture; or more recently the transition from nomadic fishing of wild salmon, to farmed salmon in captivity provided with added nutrients. Just as our ancestors discerned which wild grasses were nutritious during their hunter-gathering phases, we find ourselves at a similar crossroads with natural hydrogen. This quest for natural hydrogen reserves mirrors the ancient act of 'hunting' – searching for what nature spontaneously provides. In the annals of human history, the shift from nomadic hunting to settled agriculture is a seminal turning point, underscoring humanity's propensity for innovation. Millennia ago, instead of just reaping what nature spontaneously provided, we began planting, nurturing, and harvesting. This journey, marked by innovations from refined irrigation techniques to the introduction of fertilizers and genetically modified crops, speaks to a profound symbiosis of understanding nature and harnessing its potential. Today, as we aim to tap into the bounties of the subsurface for hydrogen production, a similar synthesis of nature's offerings and scientific ingenuity is essential.
Fig. 2(i) shows that a wide range of feedstocks, including minerals, fluids and gases, are involved within the Earth's crust in a wide range of processes that produce hydrogen. Some of these processes could be harnessed in engineered scenarios, whereby suitable crustal rocks are drilled into, additional feedstocks are injected, and left for the hydrogen generating reactions to occur. Detailed kinetic studies under high-pressure and high-temperature conditions—simulating the subsurface environments where these reactions occur—are vital. Such studies not only elucidate the dynamics of these reactions but also enable us to identify potential bottlenecks and opportunities for enhancement. Through a blend of geochemical analyses, we can grasp how trace elements and minerals influence reaction pathways. By understanding these nuances, introducing or modifying specific compounds to optimize natural processes might be feasible. Moreover, there is the possibility of using hydrocarbon reservoirs for in situ hydrogen production.18 In these underground formations, the CO2 generated during the process may naturally remain trapped, minimizing environmental impact. A case in point is in situ hydrocarbon gasification, wherein controlled thermal or oxidizing conditions could facilitate targeted subsurface reactions; however, managing heat effectively within these deep reservoirs, especially addressing potential issues with thermal dispersion, remains a challenge. At present, the issue of thermal dispersion in the context of in situ hydrogen production remains under-explored. Current literature provides limited insights into effectively managing these challenges, indicating a clear need for more focused research in this area.
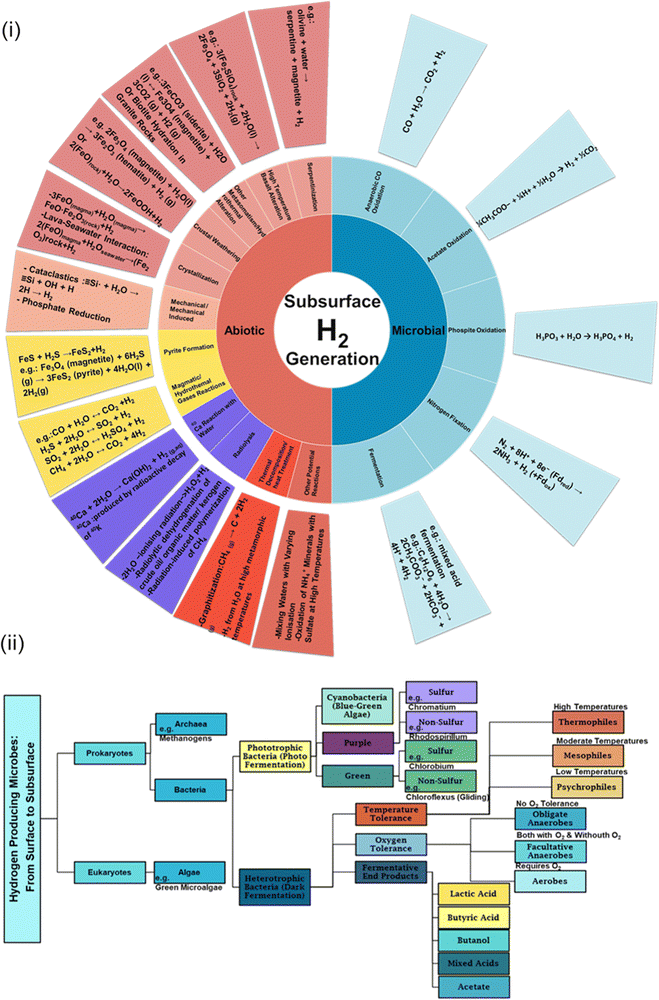 |
| Fig. 2 Comprehensive Exploration of Hydrogen Production Pathways in Nature: (i) This burst chart illustrates the diverse reactions occurring within the subsurface that lead to hydrogen production. They range from abiotic reactions such as serpentinization, high-temperature basalt alteration, and crustal weathering to microbial processes like fermentation and nitrogen fixation. The complexity and richness of these processes underscore the potential of the subsurface as a significant source of sustainable hydrogen. (ii) The diverse microbial community involved in hydrogen production, ranging from surface to subsurface environments. The chart systematically categorizes prokaryotes, such as Archaea and Bacteria, detailing further into phototrophic and heterotrophic bacterial types and their specific attributes. The eukaryotes section shines a spotlight on algae. Together, this chart underscores the breadth and depth of microbial involvement in hydrogen production across varied ecological niches. | |
Natural hydrogen generation rates are slow compared to industrial production,19e.g., minimum estimated rates in Oman range from 73 to 1300 m3 per km2 per day.10 Can we increase this, or will it be necessary to inject feedstocks and leave for years or decades, a ‘seed and leave’ approach? Although this is radically different to current approaches to industrial gas production, it is not so different from, for example, commercial forestry where the interval between planting and harvesting is measured in decades. Even in the oil industry, a large field may take years to pay back the investment costs before reaching a net profit, so again the delay in pay-back is not unprecedented.
Microbial and catalytic improvements to production rates (X to hydrogen)
Beyond this, the hydrogen producing microbial world contains a wide spectrum of organisms from the surface to the subsurface.20,21 The presence of surface microbes within Fig. 2(ii) depicts the complete spectrum, emphasizing the richness of microbial diversity and its potential in hydrogen production. Emerging within this context and the abiotic reactions mentioned above is a novel concept, as illustrated in Fig. 3: the ‘X’ to hydrogen approach. This innovative methodology involves introducing components directly into the subsurface to enhance hydrogen production rates. The ‘X’ factor, encompassing a spectrum of potential feedstocks or catalysts, could vary from specific trace minerals that act as reaction mediators to strains of microbes optimized for subsurface hydrogen production. In this paradigm, even the introduction of water could kick-start the desired reactions. The feasibility and efficiency of this approach hinges on a comprehensive understanding of subsurface geochemistry and kinetics. Variables such as pressure, temperature, and geological composition, which influence reaction pathways, become paramount in determining the optimal selection and application of the ‘X’ component. By strategically tailoring the nature and concentration of ‘X’, there exists the potential to optimise the rate and yield of hydrogen production. Furthermore, in the context of ‘X to Hydrogen,’ it is crucial to consider the interplay of geochemical principles and microbial engineering. While ‘X’ encompasses a variety of components, the strategic manipulation of geochemical conditions can significantly enhance the efficiency of microbial processes in hydrogen production. This approach demonstrates the innovative merging of geochemistry with synthetic biology, offering new pathways to optimize hydrogen generation. While this method is in its infancy, it presents an ambitious fusion of nature's geochemical complexities with human-engineered solutions. A deeper metagenomic and metatranscriptomic exploration is required to reveal the genetic intricacies of suitable microbial communities, potentially offering insights into pathways that can be harnessed or enhanced. The field of synthetic biology offers tantalizing possibilities. Imagine microbes engineered to optimize specific metabolic pathways for hydrogen production or even to thrive under conditions previously deemed unproductive. But the microbes could also be engineered to be non-viable in atmospheric oxygen – in case of leakage. Exploring techniques such as microbial electrolysis to augment microbial hydrogen production is equally paramount. Furthermore, interdisciplinary collaborations between geochemists, microbiologists, and engineers could foster innovative solutions, from developing bioreactors mimicking subsurface conditions to devising novel storage and delivery methodologies for the produced hydrogen. All these activities need to develop in parallel, to help defer, or help solve, the climate emergency. Our world needs climate action on all fronts – everything, everywhere, all at once.22
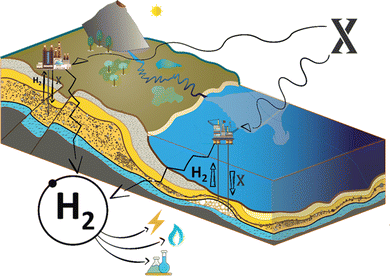 |
| Fig. 3 Subterranean Conversion Mechanisms of feedstock ‘X’ to Hydrogen: This illustration shows the process of hydrogen generation utilizing the novel “X” component within a subsurface reservoir context. The feedstock “X” may be a composite of specific materials, microbial entities, or catalytic agents. The interaction of these elements has the potential to optimize the in situ reactions essential for hydrogen production. | |
Future vision
We are poised to chart a sustainable, hydrogen-fuelled trajectory. In the modern quest for sustainable hydrogen sources, the significance of investigating subsurface geological formations for hydrogen production becomes paramount. These subsurface formations offer not only an alternative energy source; they represent a scientific frontier, laden with opportunities for advanced investigation and discovery. Integrating the principles of geochemistry with cutting-edge microbial engineering may present a paradigm shift in our approach to energy, bolstering sustainability. The potential innovations lie within the depths of terrestrial strata and intricate microbial pathways. By championing interdisciplinary collaborations, we position ourselves on the brink of a transformative energy era, harmonizing nature's riches with scientific innovation.
Conflicts of interest
There are no conflicts to declare.
Acknowledgements
A. H. extends sincere thanks to the University of Edinburgh for the generous support through the Chancellor's Fellowship and associated funding.
References
- N. H. A. Bowerman, D. J. Frame, C. Huntingford, J. A. Lowe, S. M. Smith and M. R. Allen, Nat. Clim. Change, 2013, 3, 1021–1024 CrossRef CAS
.
- A. Hassanpouryouzband, E. Joonaki, K. Edlmann and R. S. Haszeldine, ACS Energy Lett., 2021, 6, 2181–2186 CrossRef CAS
.
- A. Odenweller, F. Ueckerdt, G. F. Nemet, M. Jensterle and G. Luderer, Nat. Energy, 2022, 7, 854–865 CrossRef
.
- F. Dawood, M. Anda and G. M. Shafiullah, Int. J. Hydrogen Energy, 2020, 45, 3847–3869 CrossRef CAS
.
- IEA, Global Hydrogen Review 2023, Paris, 2023, https://www.iea.org/reports/global-hydrogen-review-2023
.
- R. M. Navarro, M. A. Pena and J. L. G. Fierro, Chem. Rev., 2007, 107, 3952–3991 CrossRef CAS PubMed
.
- N. Armaroli and V. Balzani, ChemSusChem, 2011, 4, 21–36 CrossRef CAS PubMed
.
- M. Linsenmeier, A. Mohommad and G. Schwerhoff, Nat. Clim. Change, 2023, 13, 679–684 CrossRef
.
- M. Chatenet, B. G. Pollet, D. R. Dekel, F. Dionigi, J. Deseure, P. Millet, R. D. Braatz, M. Z. Bazant, M. Eikerling and I. Staffell, Chem. Soc. Rev., 2022, 51, 4583–4762 RSC
.
- V. Zgonnik, Earth-Sci. Rev., 2020, 203, 103140 CrossRef CAS
.
- C. Neal and G. Stanger, Earth Planet. Sci. Lett., 1983, 66, 315–320 CrossRef CAS
.
- A. Prinzhofer, C. S. T. Cissé and A. B. Diallo, Int. J. Hydrogen Energy, 2018, 43, 19315–19326 CrossRef CAS
.
- G. I. Voitov, Geochemistry, 1971, 1324 CAS
.
- G. I. Voitov, Nature, 1975, 975, 90–98 Search PubMed
.
- S. L. Worman, L. F. Pratson, J. A. Karson and W. H. Schlesinger, Proc. Natl. Acad. Sci. U. S. A., 2020, 117, 13283–13293 CrossRef CAS
.
- S. L. Worman, L. F. Pratson, J. A. Karson and E. M. Klein, Geophys. Res. Lett., 2016, 43, 6435–6443 CrossRef CAS
.
- E. M. Yedinak, Joule, 2022, 6, 503–508 CrossRef
.
- P. R. Kapadia, M. S. Kallos and I. D. Gates, Fuel, 2011, 90, 2254–2265 CrossRef CAS
.
- T. M. McCollom and W. Bach, Geochim. Cosmochim. Acta, 2009, 73, 856–875 CrossRef CAS
.
- R. Nandi and S. Sengupta, Crit. Rev. Microbiol., 1998, 24, 61–84 CrossRef CAS PubMed
.
- K. Takai, T. Gamo, U. Tsunogai, N. Nakayama, H. Hirayama, K. H. Nealson and K. Horikoshi, Extremophiles, 2004, 8, 269–282 CrossRef CAS PubMed
.
-
A. Guterres, UN Secretary General opening of IPCC AR5 Report launch conference, 2023.
|
This journal is © The Royal Society of Chemistry 2024 |