Constructing hollow core–shell Z-scheme heterojunction CdS@CoTiO3 nanorods for enhancing the photocatalytic degradation of 2,4-DCP and TC
Received
26th March 2024
, Accepted 23rd April 2024
First published on 24th April 2024
Abstract
Constructing Z-scheme heterojunctions incorporating an exquisite hollow structure is an effective performance regulation strategy for the realization of high quantum efficiency and a strong redox ability over photocatalysts. Herein, we report the delicate design and preparation of a core–shell hollow CdS@CoTiO3 Z-scheme heterojunction with a CdS nanoparticle (NP)-constructed outer shell supported on a CoTiO3 nanorod (NR) inner shell. The in situ growth synthetic method led to a tightly connected interface for the heterojunction between CdS and CoTiO3, which shortened the transport distance of photoinduced charges from the interface to the surface. The promoted charge carrier separation efficiency and the retained strong redox capacity caused by the Z-scheme photoinduced charge-transfer mechanism were mainly responsible for the boosted photocatalytic performance. Additionally, the well-designed core–shell structure afforded a larger interfacial area by the multiple direction contact between CdS and CoTiO3, ensuring sufficient channels for efficient charge transfer, and thus further boosting the photocatalytic activity. As an efficient photocatalyst, the optimized CdS@CoTiO3 nanohybrids displayed excellent 2,4-dichlorophenol (2,4-DCP) and tetracycline (TC) degradation efficiencies of 91.3% and 91.8%, respectively. This study presents a Z-scheme heterojunction based on ecofriendly CoTiO3, which could be valuable for the development of metal perovskite photocatalysts for application in environmental remediation, and also demonstrated the tremendous potential of integrating a Z-scheme heterojunction with the morphology design of photocatalyts.
1. Introduction
The photocatalytic remediation of waste effluent containing toxic organic pollutants is a promising strategy to mitigate the ever-increasing environmental pollution crisis owing to its satisfactory catalytic efficiency and ecofriendly features. However, the practical application of photocatalysis is still limited due to most current photocatalyts suffering from poor visible-light-absorption performance and a fast recombination of photoinduced carriers.1–3 Developing efficient photocatalyts is highly desirable to promote the practical implementation of photocatalysis. Rationally constructing a heterojunction is an effective strategy to accelerate the spatial separation of photoinduced carriers in semiconductors and the supply of more potential catalytic sites compared to in the single-component counterparts.4,5 In particular, by forming a Z-scheme heterojunction, a wide light-absorption range and sufficient redox capacity can be simultaneously achieved, ascribed to the Z-scheme charge-transfer path with the assistance of the built-in electric field in the heterojunction region, which can thus enhance the photocatalytic performance.6–9 Recently, a number of Z-scheme heterojunctions such as g-C3N4/MoS2,10 CdIn2S4/ZnS,11 CoSx/CdS,12 and BiOCl/Bi2S313 have been constructed with the aim of achieving excellent photocatalytic performances. Additionally, assembling low-dimensional nanoscale building blocks to form hierarchical hollow configurations is reported as another commendable method for improving the photocatalytic performance.14,15 This is mainly because hierarchical hollow structures possess multiple intrinsic advantages, including improved light absorption, expedited photoinduced carrier separation and migration, and enhanced surface redox reactions.16–20 So far, numerous nanomaterials with hierarchical hollow configurations have been developed as photocatalysts to catalyze CO2 conversion,21 water splitting reactions,22 and the photocatalytic degradation of pollutants.23 Z-scheme heterojunction photocatalysts can further accelerate the separation and direct transfer of light-induced electron–hole pairs to promote the redox reactions. It is noteworthy that forming core–shell structures with distinct boundaries can not only provide larger active interfaces between the constituent materials by multiple directional contact, but also produce new synergetic effects owing to the ranges of physical and chemical properties of the core and shell influenced by their compositions, structures, and dimensions, making them attractive for applications in catalysis.24,25 For example, Zhu et al.26 prepared a Sn2+-ion self-doping CdS/ZnSnO3 hollow core–shell cubic heterojunction photocatalyst, which demonstrated significantly improved photocatalytic activity for the hydrogen evolution reaction and for ciprofloxacin degradation under visible-light irradiation.
Among the numerous reported photocatalysts, perovskite oxide CoTiO3 has aroused much interest recently owing to its superior photoelectronic properties, visible-light-responsive band gap (Eg ≈ 2.3), and high carrier mobility. In particular, its unique ABO3 structure can increase ionic defects or oxygen vacancies via holding more cations, resulting in improved photocatalytic performances.27,28 Importantly, CoTiO3 possess a high valence band potential (Ev ≈ 2.3) that endow Ev holes with strong oxidizability. CoTiO3-based photocatalytic materials have already been exploited for pollutant degradation and have achieved excellent performances.29–31 Apparently, the introduction of a complementary semiconductor with a strong reduction capacity to construct Z-scheme heterojunctions with CoTiO3 can not only contribute to promoting a strong redox ability but also fast carrier separation and transportation, thus further improving the photocatalytic performance. CdS with a moderate band gap (≈2.4 eV) has been widely reported to be a remarkable photocatalyst.32–34 Moreover, CdS possessing the required conduction band potential (EC) of about −0.5 V is more negative than that of CoTiO3, which makes it one of the potential candidates to construct Z-scheme heterojunctions with CoTiO3. The fabrication of heterostructured nanocomposites of CoTiO3 by combining with CdS can not only endow the hybrids with novel characteristics, but also improve their efficiency due to synergistic effects. The given background supports the feasibility of synergistic photocatalysis by combining CoTiO3 and CdS. Yet, to the best of our knowledge, the use of hybrids of CdS and CoTiO3 as an efficient photocatalyst for the degradation of toxic organic pollutants in waste effluents has not been reported previously.
To realize the ingenious synergistic advantages of a Z-scheme heterojunction and a hollow structure, a hierarchical hollow CdS@CoTiO3 Z-scheme heterojunction was obtained by the in situ growth of CdS NPs on the surface of CoTiO3 NRs, in which the CoTiO3 NRs served as an outstanding matrix that could also greatly prevent the CdS NPs from agglomerating. The unique structure promoted the separation and transfer of photogenerated charges, while also offering a large surface area for target pollutants adsorption, and the exposure of abundant active sites for surface catalysis. Accordingly, the optimized CdS@CoTiO3 hybrids manifested superior activity for the visible-light degradation of 2,4-DCP and TC, achieving remarkable degradation efficiencies of ∼91.3% (2,4-DCP) and 91.8% (TC), respectively. Additionally, CdS@CoTiO3 exhibited remarkable stability over four successive cycles of the degradation process. Based on the experiment results, the enhanced photocatalytic degradation mechanism of CdS@CoTiO3 is proposed.
2. Experimental section
2.1. Materials
The key reagents were Co(CH3COOH)2·4H2O, tetrabutyl titanate, ethylene glycol (EG), diethylenetriamine (DETA), CdSO4·8H2O, thiourea, (Kemiou Chemical Reagent Co. Ltd, Tianjin, China), 2,4-dichlorophenol, and tetracycline (Aladdin Biological Co., Ltd, Shanghai, China). All the reagents were of analytical grade.
2.2. Synthesis
2.2.1. Preparation of hollow CoTiO3 nanorods.
The CoTiO3 was prepared according to a literature method35,36 with some modifications as follows: Co(CH3COOH)2·4H2O (0.244 g) and butyl titanate (0.4 mL) were mixed with ethylene glycol (60 mL) and vigorously stirred at room temperature for 24 h to obtain light-green Co–Ti–ethylene glycol (Co–Ti–EG–). The Co–Ti–EG– was collected by centrifugation. After washing with ethanol three times, the obtained Co–Ti–EG– was dried in an oven at 80 °C and calcined at 600 °C for 2 h under vacuum conditions at a heating rate of 2 °C min−1 to obtain the CoTiO3 nanorod structures as a green powder.
2.2.2. Preparation of the core–shell CdS@CoTiO3 hybrids.
The CdS@CoTiO3 hybrid was synthesized by the in situ growth of CdS on the surface of CoTiO3via a facile solvothermal method, as illustrated in Scheme 1. Here, 10%CdS@CoTiO3, 0.0865 g Cd(NO3)2·4H2O, and 0.0215 g thiourea were added into an 80 mL mixed solvent of 64 mL deionized H2O and 16 mL DETA, and then a certain amount of CoTiO3 (0.3724 g) was added as the substrate, with stirring for 5 min. After that, the above mixture was transferred to a 100 mL Teflon-lined stainless steel autoclave and kept at 160 °C for 5 h. Finally, the product was washed with water and ethanol three times, collected, and dried in an oven at 80 °C for 24 h. The obtained samples were denoted as xCdS@CoTiO3 (where x means the mass ratio (%) of CdS relative to CoTiO3, and x = 5, 10, 15, and 20). For comparison, pure CdS was prepared by the same method in the absence of CoTiO3.
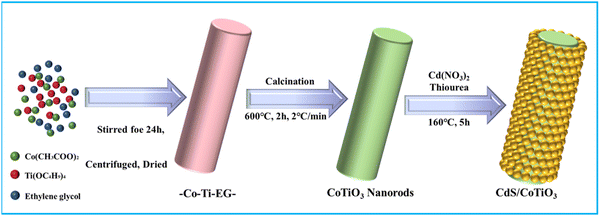 |
| Scheme 1 Illustration of the synthesis of the CdS@CoTiO3 Z- scheme heterojunction. | |
2.2.3. Characterizations.
The morphologies and microstructures of the catalysts were analyzed by SEM (S4800, Hitachi Co., Japan), HRTEM coupled with EDX elemental scanning spectroscopy (S4800, Hitachi Co., Japan and 2100F, JEOL Co. Japan), XRD with Cu Ka radiation (D/max-2200, Rigaku., Japan), and XPS (Thermo Electron, ESCALAB 250 Xi., USA). The UV-visible diffuse reflectance spectra and photoluminescence spectra were acquired using UV2550 and FLS-920T fluorescence spectrophotometers, respectively. The ESR signals were recorded using a Bruker A300 spectrometer. The photoelectrochemical and electrochemical impedance spectra were obtained on a CHI660D electrochemical workstation (Shanghai Chenhua, China).
2.2.4. Evaluation of the photocatalytic degradation activity.
Here, 2,4-DCP (10 mg L−1) and TC (10 mg L−1) were selected to estimate the photocatalytic activities of the obtained materials under visible-light irradiation. A 500 W Xenon lamp with a 420 nm cutoff filter (100 mW cm−2 light intensity) was used as the visible-light source and the mixture was maintained at around 20 cm distance from it. First, 30 mg photocatalyst was added into a photocatalytic reactor containing 50 mL 2,4-DCP/TC solution and then placed in darkness under magnetic stirring for 40 min at 300 rpm to achieve adsorption–desorption equilibrium. After that, the light source was turned on and 2 mL aqueous was withdrawn from the reactor at certain time intervals, and centrifuged to remove the catalysts. The concentration of 2,4-DCP was monitored using an Agilent 1290 Infinity II ultraperformance liquid chromatography (UPLC) system equipped with a C18 column and UV detector (λ = 284 nm) and the mobile phase was HCOOH and CH3OH (50/50, v:v) with a flow rate of 1.0 mL min−1, while TC was analyzed using a Lambda 750S UV/VIS/NIR spectrometer at λ = 358 nm.
The degradation ratio (R) of 2,4-DCP and TC was calculated according to the following equation:
| 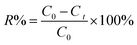 | (1) |
where
C0 and
Ct denote the 2,4-DCP and TC concentrations in mg L
−1 at the initial time and at time
t (min), respectively.
3. Results and discussion
It could be clearly observed that pristine CoTiO3 displayed a rod-like morphology with a length and width of approximately 2 μm and 400 nm, respectively (Fig. 1a), and a definite interior cavity could also be observed (see the inset of Fig. 1a). Meanwhile, the morphology analysis of pure CdS showed it was composed of a great many irregular nanoparticles agglomerated together (Fig. 1b). Following the hydrothermal reaction at 160 °C, CdS NPs uniformly grew on the whole surface of the CoTiO3 NRs to form the shell layer without any agglomeration (Fig. 1c), meaning there was a large contact area between CdS and CoTiO3 to form more heterojunctions. A typical TEM image of the as-derived sample further confirmed the hollow core–shell structure with a thickness of CdS shell layer of about 40 nm, revealing that the incorporation of CdS had no obvious influence on the morphology.
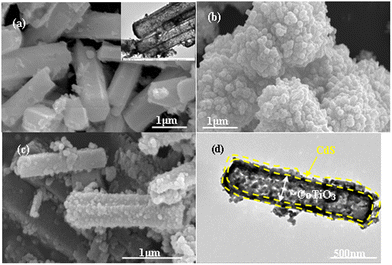 |
| Fig. 1 SEM images: CoTiO3 (inset is the TEM image) (a), CdS (b), CdS@CoTiO3 (c); and TEM image of CdS@CoTiO3 (d). | |
Additionally, HRTEM images for the CdS@CoTiO3 heterojunction are shown in Fig. 2a and b. According to the partially magnified image, a clear heterojunction interface with the intersection of the lattice fringes could be observed, further confirming the forming of the CdS@CoTiO3 heterojunction. The tight anchoring of CdS NPs on the CoTiO3 nanorods favored the formation of intimate interfacial contacts, which is highly beneficial for efficient charge separation/transfer in hybrids.37 The clear lattice fringe with a spacing of 0.37 nm were attributed to the (012) plane of CoTiO3 nanorods (Fig. 2c), while that of 0.34 nm was assigned to the (002) plane of CdS (Fig. 2d).38,39 To further obtain information on the elemental composition and distribution for the CdS@CoTiO3 heterojunction photocatalyst, scanning TEM-energy-dispersive X-ray (STEM-EDX) characterization was conducted. As shown in Fig. 2e, it could be observed that the CdS@CoTiO3 heterojunction contained Co, Ti, O, Cd, and S elements, and their distribution was homogeneous, supporting the results obtained from the TEM and HRTEM studies showing that the CdS nanoparticles were uniformly covered on the CoTiO3 core nanostructures.
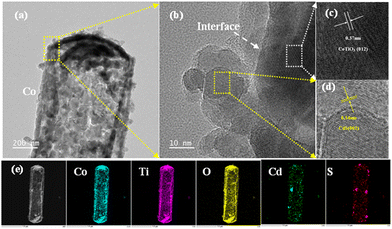 |
| Fig. 2 (a)–(d) HRTEM micrographs and (e) corresponding EDS elemental mapping images of the CdS@CoTiO3 heterojunction. | |
XPS was performed to obtain information on the elements present and on the alteration in the electronic environments of the different samples. The XPS spectra of CoTiO3, CdS, and CdS@CoTiO3 verified the existence of the corresponding elements (Fig. 3a and b). Fig. 3c illustrates the fine spectra of Co in CoTiO3 and CdS@CoTiO3. The two peaks at about 796.18 and 780.18 eV with their distinguishable satellite peaks (at about 802.38 and 785.68 eV, denoted as Sta.) were indexed to Co 2p3/2 and Co 2p1/2. Moreover, the splitting energy between the Co 2p1/2 and Co 2p3/2 peaks of CoTiO3 and CdS@CoTiO3 were consistent with the theoretical value of 16 eV for Co(II), revealing the presence of Co2+ in CoTiO3.27,40 Notably, the binding energies were positively shifted by 0.60 eV for the CdS@CoTiO3 heterojunction. The Ti 2p spectra for CoTiO3 and CdS@CoTiO3 are portrayed in Fig. 3d, where the peaks at about 457.51 and 463.24 eV were from the 2p3/2 and 2p1/2 orbitals of Ti, respectively.41,42 After incorporating CdS NPs, the binding energy was 0.57 eV higher than that of pure CoTiO3. The high-resolution O1s XPS spectrum of the CdS@CoTiO3 heterojunction was divided into three peaks, in which the peak at 532.98 eV originated from the –OH groups adsorbed on the surface (Fig. 3e),43 while the peaks at 530.47 and 529.16 eV represent the oxygen present in the lattice of CoTiO3.44,45 A positive shift by around 0.20 eV was also observed in the CdS@CoTiO3 heterojunction. At the same time, the binding energies for Cd 3d and S 2p for the CdS@CoTiO3 heterojunction were negatively shifted of 0.20 and 0.50 eV with respect to the pristine CdS NPs, respectively (Fig. 3f and g). It is well accepted that the binding energy is negatively correlated with surface electron density.46 The positive shifts of the Co 2p, Ti 2p, and O 1s XPS peaks as well as the negative shifts of the Cd 3d and S 2p regions on CdS@CoTiO3 suggested that the electrons transfer from CoTiO3 to CdS, further manifesting that hybrids with efficient electron-transfer processes were formed in the CdS@CoTiO3 heterojunction.47–49
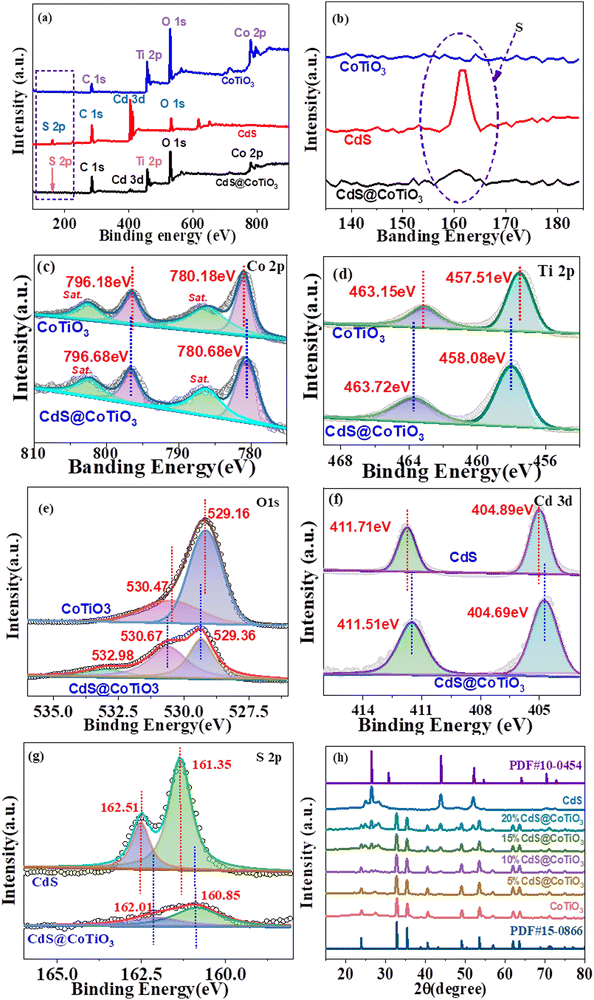 |
| Fig. 3 (a) and (b) XPS survey spectra and high-resolution XPS spectra: (c) Co 2p, (d) Ti 2p, (e) O 1s, (f) Cd 3d, (g) S 2p; and (h) the XRD spectra of different samples. | |
Fig. 3h presents the X-ray diffraction (XRD) patterns for CdS, CoTiO3, and a series of CdS@CoTiO3 heterojunctions. For CdS and CoTiO3, all the diffraction peaks matched the respective standard cards (CdS, PDF#10-0454)50 and (CoTiO3, PDF#15-0866).51 As for the CdS@CoTiO3 heterojunctions, not only were diffraction peaks corresponding to CoTiO3 observed, but also peaks for CdS. Interestingly, the intensity of the peaks for CdS gradually increased with the dosage increase, which unambiguously confirmed the successful synthesis of the CdS@CoTiO3 heterojunction.
The light-absorption property was investigated by the UV-vis-DRS technique for better exploring the photocatalytic mechanism of the CdS@CoTiO3 hybrids. As shown in Fig. 4a, the absorption edge of the CdS NPs was about 550 nm, concurring with related reports.13 The pure CoTiO3 NRs displayed optical adsorption properties both in the UV and visible-light regions. Furthermore, absorption peaks centered at about 538 and 605 nm were observed, which could be indexed to metal-to-metal charge transfer (Co2+ to Ti 4+).52 Notably, after assembling CdS NPs on the CoTiO3 hollow NRs, a stronger absorption intensity was discovered compared to CoTiO3, implying that the CdS@CoTiO3 hybrid enabled efficient light harvesting, which was mainly due to the well-designed core–shell structure, wherein the tightly connected interface accelerated the transmission rate of charge carriers, and thus promoted the photocatalytic performance. According to the transformed Kubelka–Munk function,53 the corresponding bandgaps of CdS, CoTiO3, and CdS@CoTiO3 were estimated to be 2.23, 2.48, and 2.42, respectively (Fig. 4b).
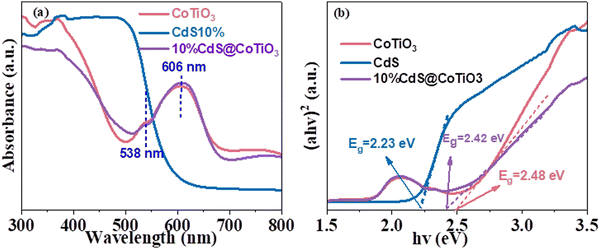 |
| Fig. 4 (a) UV-vis-diffuse reflectance spectra and (b) corresponding (αhν)2versus hν curves of the pure CoTiO3, CdS, and CdS@CoTiO3 heterojunction. | |
Subsequently, the band structures of CoTiO3 and CdS were calculated using the following equations:54,55
where
χ and
Eg are the bulk electronegativity and bandgap of the semiconductors, respectively, and
E0 represents the energy of free electrons
versus NHE (≈ 4.5 eV). The estimated values of
χ for CoTiO
3 and CdS were 5.76 and 5.19 eV, respectively. Hence,
EC and
EV for CoTiO
3 were calculated to be 0.02 and 2.50 eV, respectively, while these of CdS were estimated to be −0.43 and 1.80 eV, respectively.
Various photoelectrochemical techniques were applied to obtain insights into the separation and transfer performance of the carriers between CdS and CoTiO3 in the CdS@CoTiO3 hybrid. Compared to CdS and CoTiO3 alone, CdS@CoTiO3 clearly displayed an enhancement in photoelectric current density (Fig. 5a), implying the lowest recombination rate of photogenerated carriers.56 Moreover, the lower charge-transfer resistance in the CdS@CoTiO3 hybrid was also confirmed by the smaller high-frequency semicircle in the electrochemical impedance spectroscopy results (EIS, Fig. 5b).57 The photoelectrochemical characterizations indicated the accelerated separation and migration of photoinduced charges in the CdS@CoTiO3 hybrid, and thus a prominent activity for photodegrading pollutants. Additionally, an obvious inhibition of the recombination of photogenerated e−/h+ in the CdS@CoTiO3 hybrids was indicated by the PL emission spectrum. Generally, a weaker PL intensity means a higher separation and transfer performance of photogenerated e−/h+ pairs.17,58 As presented in Fig. 5c, the emission intensity of the CdS@CoTiO3 hybrids was remarkably weaker than those of CdS and CoTiO3 alone, signifying the recombination of light-induced charges in the CdS@CoTiO3 hybrids was effectively prohibited.
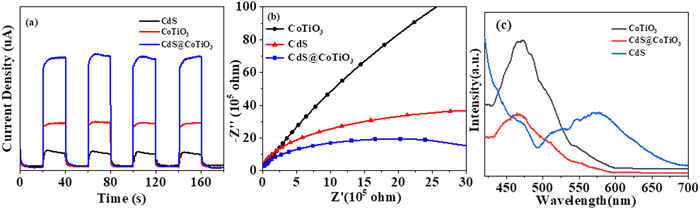 |
| Fig. 5 (a) Visible-light photocurrent responses, (b) EIS Nyquist plots, and (c) PL plots of the pure CoTiO3, CdS, and CdS@CoTiO3 heterojunction. | |
To evaluate the photocatalytic activity of the as-prepared catalysts, the representative organic pollutant 2,4-DCP solution was chosen as a pollution model, as exhibited in Fig. 6. Clearly, all the samples were essentially saturated by adsorption within 40 min in the dark, with only a small decrease in the concentration of 2,4-DCP. Moreover, only a negligible removal of 2,4-DCP was observed in the absence of any photocatalyst, suggesting that 2,4-DCP exhibited excellent stability under visible-light irradiation (Fig. 6a). All the CdS@CoTiO3 hybrids delivered boosted degradation capability toward 2,4-DCP compared with CdS and CoTiO3 alone, which was attributed to the acceleration of the separation of photogenerated carriers and the strength of the redox capacity caused by the formation of a hollow core–shell Z-scheme heterojunction between CdS and CoTiO3. Among the hybrids with different masses of CdS, 10% CdS@CoTiO3 showed the highest activity, with a removal efficiency of approximately 91.3%, while 50% and 46% removal efficiencies were obtained for CdS and CoTiO3 after 120 min visible irradiation. Fig. 6b displays the fitting of the kinetics of the photocatalytic degradation, whereby all the catalysts conformed to the quasi-first-order kinetic model. The kinetic constant (k) of 10% CdS@CoTiO3 (0.018 min−1) was about 4.0- and 4.6-times higher than those of pure CdS (0.0045 min−1) and CoTiO3 (0.0039 min−1), respectively. The concentration changes of 2,4-DCP was detected by UPLC. As depicted in Fig. 6c, the intensity of the peak for 2,4-DCP rapidly decreased with increasing the irradiation time, suggesting that 2,4-DCP was gradually decomposed over the 10% CdS@CoTiO3 heterojunction. Considering its most effective performance, 10% CdS@CoTiO3 was chosen for the subsequent research studies, without special instructions.
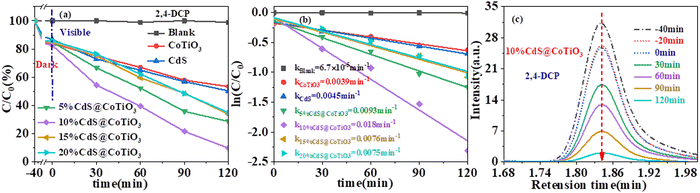 |
| Fig. 6 (a) Photocatalytic degradation of 2,4-DCP aqueous solutions, and the (b) corresponding kinetic fitting curves over different samples under visible-light irradiation; and (c) corresponding UPLC plots of 2,4-DCP during degradation over the 10%CdS@CoTiO3 hybrid. | |
Subsequently, the photocatalytic degradation of a well-known antibiotic (TC) was investigated to confirm the enhancement of the photodegradation performance of the 10% CdS@CoTiO3 hybrids under identical conditions (Fig. 7). Obviously, the removal over the CdS@CoTiO3 hybrids (≈ 91.8%) was much higher than that for CoTiO3 alone (≈ 65.3%) with 80 min visible irradiation (Fig. 7a and b), with a k of 0.025 min−1, which was 2.5-times higher than that of CoTiO3 (0.010 min−1). Furthermore, the photocatalytic degradation of TC was confirmed by recording its UV-visible spectral behavior at λmax = 358 nm (Fig. 7c and d).
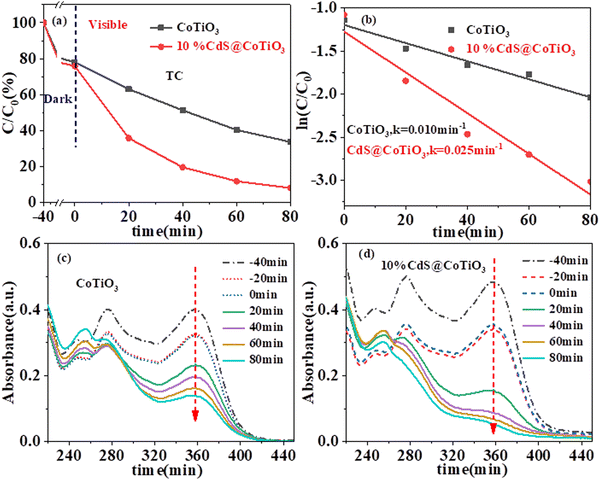 |
| Fig. 7 (a) Photocatalytic degradation of TC aqueous solutions, and the (b) corresponding kinetic fitting curves over pure CoTiO3 and (d) 10% CdS@CoTiO3; time-dependent absorption spectra of TC degradation: (c) pure CoTiO3, (d) 10% CdS@CoTiO3. | |
Generally, cycling stability is a crucial parameter to assess the industrialization potential of photocatalysts. Therefore, the 10% CdS@CoTiO3 hybrid with the best catalytic activity was selected for the cycling tests, as shown in Fig. 8. It could be clearly seen that the photocatalytic performance was not significantly attenuated for 2,4-DCP degradation after four cycling tests (Fig. 8a). Furthermore, the XRD spectrum of 10% CdS@CoTiO3 showed no obvious change after the reaction compared with that before, while the architectural structure of the CdS@CoTiO3 hybrids was also well maintained after the cycles (Fig. 8b and c), further implying the satisfactory reusability and stability of CdS@CoTiO3during the photocatalytic reaction.
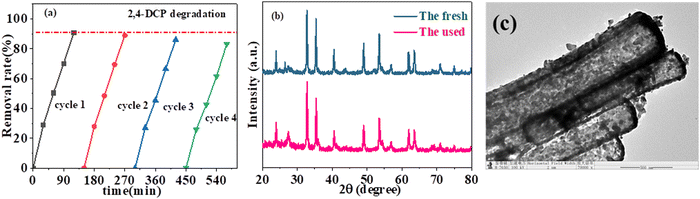 |
| Fig. 8 (a) Reusability of the catalyst for the removal of 2,4-DCP under visible-light irradiation, (b) XRD patterns before and after the photocatalytic tests, and (c) TEM image of the CdS@CoTiO3 hybrid after use. | |
Next, trapping tests were performed to investigate the reactive species taking part in the photodegradation of 2,4-DCP process. Experimentally, isopropanol (IPA), sodium oxalate (Na2C2O4), and benzoquinone (BQ) were used to scavenge hydroxyl radicals (˙OH), superoxide radicals (˙O2−), and holes (h+), respectively.11 As illustrated in Fig. 9a, with the addition of IPA and BQ, the degradation efficiency of 2,4-DCP significantly decreased from 91.0% to 49.5% and 45.1%, indicating that ˙OH and ˙O2− as primary active species participated in 2,4-DCP degradation. Meanwhile, the remove efficiency was also inhibited from 91.0% to 61.9% after introducing Na2C2O4, implying that h+ also play a certain role in the photocatalytic degradation of 2,4-DCP. Furthermore, the generation of ˙OH and ˙O2− in the photocatalytic reaction system was further confirmed by EPR using DMPO as a free radical trapping agent, as exhibited in Fig. 9b. Clearly, no EPR signal was observed under the dark condition, indicating no ˙OH or ˙O2− were produced.59,60 However, four characteristic signals of DMPO-˙OH possessing the intensity ratio of 1
:
2
:
2
:
1 were observed in the EPR spectrum after 5 min of visible-light irradiation. Similarly, typical DMPO-˙O2− adducts (quartet signal relative intensities of 1
:
1
:
1
:
1) were also detected in the CdS@CoTiO3 photocatalytic degradation system, illustrating the formation of ˙O2−. The EPR results unambiguously suggested that ˙OH and ˙O2− radicals were both formed over the CdS@CoTiO3 hybrids, which was well consistent with the active species trapping experimental results.
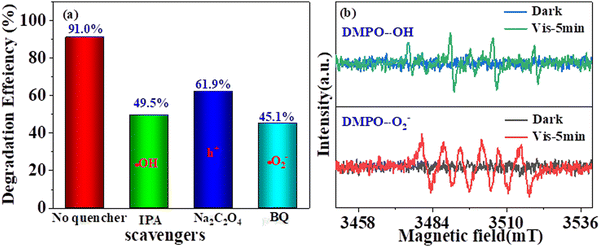 |
| Fig. 9 (a) Influence of various scavengers on the degradation of 2,4-DCP and (b) EPR spectra in the dark and under visible-light irradiation for DMPO-˙OH and DMPO-˙O2−. | |
Based on the energy band positions of CdS and CoTiO3, as well as the results from the radical trapping tests and EPR analysis, a typical Z-scheme mechanism over the core–shell structured CdS@CoTiO3 is proposed, as shown in Scheme 2. In this mechanism, owing to the narrow band gaps, both CdS and CoTiO3 can be excited by visible light to generate electrons (e−) in the conduction band (CB) and positive holes (h+) in the valence band (VB) simultaneously. Generally, e− transfer occurs from the EC,CdS to the EC,CoTiO3, meanwhile h+ accumulate at EV,CdS (Scheme 2a, type-II heterojunctions). In such a case of charge-transfer behavior, the EV,CdS is not sufficient to oxidize OH− (EOH−/˙OH = 2.38 eV vs. NHE) and the EC,CoTiO3 is unable to reduce O2 to generate ˙O2− (EO2/˙O2− = 0.33 eV vs. NHE).61,62 Clearly, this is not consistent with the results of the trapping experiments, where ˙O2− and ˙OH were found to be the major active species in the photocatalytic degradation 2,4-DCP system. For the Z-scheme mechanism, the photoinduced e− in the CB of CoTiO3 can transfer to the VB of CdS and recombine with the photogenerated h+, which not only inhibits the self-recombination of e−/h+ pairs in both CdS and CoTiO3 but also preserves the more negative CB potential of CdS and a more positive VB potential of CoTiO3 for the photocatalytic reaction, thereby resulting in an efficient 2,4-DCP mineralization reaction. Drawing from the above analysis, the possible reaction equations could be elucidated as follows in eqn (4)–(7):
| CdS@CoTiO3 + hv → CdS@CoTiO3(h+ + e−) | (4) |
| CoTiO3(h+) + H2O → ˙OH + H+ | (6) |
| 2,4-DCP/TC + h+/˙O2−/˙OH → CO2 + H2O. | (7) |
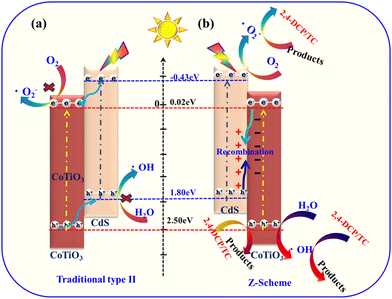 |
| Scheme 2 Illustration of the photocatalytic degradation mechanisms for 3D CdS@CoTiO3 heterojunctions: (a) type II and (b) Z-scheme. | |
From the architectural point of view, the hierarchical hollow core–shell nature of the CdS@CoTiO3 photocatalyst with large internal cavities indeed endow it with sufficient active sites, while also shortening the transport distance of photoinduced charges as well as improving the capture capacity for visible light, simultaneously.
4. Conclusions
In summary, a hierarchical core–shell CdS@CoTiO3 photocatalyst was constructed by uniformly modifying a CdS NPs layer on the surface of CoTiO3 hollow NRs. The experimental investigation and EPR analytical results confirmed the existence of a Z-scheme photoinduced carrier transport path between CdS and CoTiO3. The Z-scheme heterojunction configuration combining this delicate architecture greatly decreased the recombination of photogenerated e−/h+ pairs while still retaining a strong oxidation and reduction ability, resulting in a dramatically improved photocatalytic degradation activity for 2,4-DCP and TC. Additionally, the hollow core–shell nanorod structure could maintain good photocatalytic stability. It is speculated that the strategy adopted in this work could also be expanded to other hollow CoTiO3-based materials for applications not only in photocatalysis but also in other fields, such as energy storage and conversion, and electrochemistry.
Conflicts of interest
There are no conflicts to declare.
Acknowledgements
This project was supported by the National Natural Science Foundation of China (NSFC21906088) and (NSFC52170039), Heilongjiang Youth Innovative Talents Training Program for Universities (UNPYSCT-2020071). The authors also thank their colleagues and other students who participated in this study.
References
- C.-C. Wang, J.-R. Li, X.-L. Lv, Y.-Q. Zhang and G. Guo, Photocatalytic organic pollutants degradation in metal–organic frameworks, Energy Environ. Sci., 2014, 7, 2831–2867 RSC.
- D. Chen, Y. Cheng, N. Zhou, P. Chen, Y. Wang, K. Li, S. Huo, P. Cheng, P. Peng, R. Zhang, L. Wang, H. Liu, Y. Liu and R. Ruan, Photocatalytic degradation of organic pollutants using TiO2-based photocatalysts: A review, J. Cleaner Prod., 2020, 268, 121725 CrossRef CAS.
- Y. Cheng, Y. Zhang, Z. Wang, R. Guo, J. You and H. Zhang, Review of Bi-based catalysts in piezocatalytic, photocatalytic and piezo-photocatalytic degradation of organic pollutants, Nanoscale, 2023, 15, 18571–18580 RSC.
- V. Gadore, S. R. Mishra and M. Ahmaruzzaman, Metal sulphides and their heterojunctions for photocatalytic degradation of organic dyes-A comprehensive review, Environ. Sci. Pollut. Res., 2023, 30, 90410–90457 CrossRef CAS PubMed.
- A. A. Okab, Z. H. Jabbar, B. H. Graimed, A. I. Alwared, S. H. Ammar and M. A. Hussein, A comprehensive review highlights the photocatalytic heterojunctions and their superiority in the photo-destruction of organic pollutants in industrial wastewater, Inorg. Chem. Commun., 2023, 158, 111503 CrossRef CAS.
- J. Low, J. Yu, M. Jaroniec, S. Wageh and A. A. Al-Ghamdi, Heterojunction Photocatalysts, Adv. Mater., 2017, 29, 1601694 CrossRef PubMed.
- S. Cheng, Z. Sun, K. H. Lim, A. A. Wibowo, T. Zhang, T. Du, L. Liu, H. T. Nguyen, G. K. Li, Z. Yin and S. Kawi, Dual-Defective Two-Dimensional/Two-Dimensional Z-Scheme Heterojunctions for CO2 Reduction, ACS Catal., 2023, 13, 7221–7229 CrossRef CAS.
- L. X. Wang, C. B. Bie and J. G. Yu, Challenges of Z-scheme photocatalytic mechanisms, Trends Chem., 2022, 4, 973–983 CrossRef CAS.
- J. Yu, C. Zhang, Y. Yang, T. Su, G. Yi and X. Zhang, 3D chrysanthemum-like g-C3N4/TiO2 as an efficient visible-light-driven Z-scheme hybrid photocatalyst for tetracycline degradation, Phys. Chem. Chem. Phys., 2023, 25, 3848–3858 RSC.
- F. Xing, C. Wang, S. Liu, S. Jin, H. Jin and J. Li, Interfacial Chemical Bond Engineering in a Direct Z-Scheme g-C3N4/MoS2 Heterojunction, ACS Appl. Mater. Interfaces, 2023, 15, 11731–11740 CrossRef CAS PubMed.
- F. Wang, T. Lu, X. Cheng, Y. Zhang and X. Xiao, Fabrication of MoSe2/Bi3O4Br Z-scheme heterojunction with enhanced photocatalytic performance, J. Environ. Chem. Eng., 2024, 12, 111991 CrossRef CAS.
- H. Wang, Q. Liu, T. Ji, R. Zhao, L. Li, J. Han and L. Wang, Preparation of Mn0.8Cd0.2S/NiCo2S4 Z-scheme heterojunction composite for enhanced photocatalytic hydrogen production, Surf. Interfaces, 2024, 45, 103900 CrossRef CAS.
- W. Wang, Z. Liu, H. Nie and B. Kong, The direct Z-scheme character and roles of S vacancy in BiOCl/Bi2S3-(001) heterostructures for superior photocatalytic activity: a hybrid density functional investigation, Phys. Chem. Chem. Phys., 2024, 26, 10723–10736 RSC.
- S. Wang, B. Y. Guan and X. W. D. Lou, Construction of ZnIn2S4–In2O3 Hierarchical Tubular Heterostructures for Efficient CO2 Photoreduction, J. Am. Chem. Soc., 2018, 140, 5037–5040 CrossRef CAS PubMed.
- D. Zheng, X.-N. Cao and X. Wang, Precise Formation of a Hollow Carbon Nitride Structure with a Janus Surface To Promote Water Splitting by Photoredox Catalysis, Angew. Chem., Int. Ed., 2016, 55, 11512–11516 CrossRef CAS PubMed.
- F. Zhao, Y. L. Law, N. Zhang, X. Wang, W. Wu, Z. Luo and Y. Wang, Constructing Spatially Separated Cage-Like Z-scheme Heterojunction Photocatalyst for Enhancing Photocatalytic H2 Evolution, Small, 2023, 19, 2208266 CrossRef CAS PubMed.
- C.-Q. Li, X. Du, S. Jiang, Y. Liu, Z.-L. Niu, Z.-Y. Liu, S.-S. Yi and X.-Z. Yue, Constructing Direct Z-Scheme Heterostructure by Enwrapping ZnIn2S4 on CdS Hollow Cube for Efficient Photocatalytic H2 Generation, Adv. Sci., 2022, 9, 2201773 CrossRef CAS PubMed.
- G.-Q. Zhao, X. Long, J. Zou, J. Hu and F.-P. Jiao, Design of hollow nanostructured photocatalysts for clean energy production, Coord. Chem. Rev., 2023, 477, 214953 CrossRef CAS.
- X. Li, J. Yu and M. Jaroniec, Hierarchical photocatalysts, Chem. Soc. Rev., 2016, 45, 2603–2636 RSC.
- S. Wang, Y. Wang, S.-Q. Zang and X. W. Lou, Hierarchical Hollow Heterostructures for Photocatalytic CO2 Reduction and Water Splitting, Small, Methods, 2020, 4, 1900586 CAS.
- X. Lin, Z. Xie, B. Su, M. Zheng, W. Dai, Y. Hou, Z. Ding, W. Lin, Y. Fang and S. Wang, Well-defined Co9S8 cages enable the separation of photoexcited charges to promote visible-light CO2 reduction, Nanoscale, 2021, 13, 18070–18076 RSC.
- S. Wang, Y. Wang, S. L. Zhang, S.-Q. Zang and X. W. Lou, Supporting Ultrathin ZnIn2S4 Nanosheets on Co/N-Doped Graphitic Carbon Nanocages for Efficient Photocatalytic H2 Generation, Adv. Mater., 2019, 31, 1903404 CrossRef CAS PubMed.
- X. Wang, C. Guo, X. Hu, Y. Xu, C. Zhang, J. Wei, J. Weng, Y. Liu, L. Fu, Q. Wang and T. Yang, Self-assembled oxygen vacancies modified hierarchical hollow tubular Bi2WO6/In2O3 Z-scheme heterojunction for optimized photocatalytic degradation of norfloxacin, J. Environ. Chem. Eng., 2024, 12, 112010 CrossRef CAS.
- S. Das, J. Pérez-Ramírez, J. Gong, N. Dewangan, K. Hidajat, B. C. Gates and S. Kawi, Core–shell structured catalysts for thermocatalytic, photocatalytic, and electrocatalytic conversion of CO2, Chem. Soc. Rev., 2020, 49, 2937–3004 RSC.
- J. Wang, R. Pan, S. Yan, R. Wang, X. Niu, Q. Hao, J. Ye, Y. Wu and H. Ying Yang, Construction of 1D/2D core-shell structured K6Nb10.8O30@Zn2In2S5 as S-scheme photocatalysts for cocatalyst-free hydrogen production, Chem. Eng. J., 2023, 463, 142489 CrossRef CAS.
- X. Li, H. Yang, Q. Peng, M. Zhu and J. Pan, CdS/ZnSnO3 Hollow Core–Shell Nanocubes for Photocatalytic Hydrogen Evolution and Degradation of Ciprofloxacin, ACS Appl. Nano Mater., 2024, 7, 649–660 CrossRef CAS.
- X. Yang, S. Wei, X. Ma, Z. Gao, W. Huang, D. Wang, Z. Liu and J. Wang, Core–shell CoTiO3@MnO2 heterostructure for the photothermal degradation of tetracycline, J. Mater. Sci., 2023, 58, 3551–3567 CrossRef CAS.
- T. Li, X. Wang, Z. Jin and N. Tsubaki, Enhanced kinetics of photocatalytic hydrogen evolution by interfacial Co-C bonded strongly coupled S-scheme inorganic perovskite/organic graphdiyne (CnH2n-2) heterojunction, Chem. Eng. J., 2023, 477, 147018 CrossRef CAS.
- Y. Liu, J. Wu, X. Li, J. Chen, Y. Li, X. Luo, T. Xie, Q. Qiu and T. Liang, Highly efficient CoTiO3/MOF-derived In2S3 photo-electrocatalysts: Degradation kinetics, pathways, and mechanism, J. Alloys Compd., 2024, 975, 172921 CrossRef CAS.
- B. Lin, S. Li, Y. Peng, Z. Chen and X. Wang, MOF-derived core/shell C-TiO2/CoTiO3 type II heterojunction for efficient photocatalytic removal of antibiotics, J. Hazard. Mater., 2021, 406, 124675 CrossRef CAS PubMed.
- R. Dadigala, R. Bandi, M. Alle, B. R. Gangapuram, V. Guttena and J.-C. Kim, In-situ fabrication of novel flower like MoS2/CoTiO3 nanorod heterostructures for the recyclable degradation of ciprofloxacin and bisphenol A under sunlight, Chemosphere, 2021, 281, 130822 CrossRef CAS PubMed.
- L. Zheng, F. Teng, X. Ye, H. Zheng and X. Fang, Photo/Electrochemical Applications of Metal Sulfide/TiO2 Heterostructures, Adv. Energy Mater., 2020, 10, 1902355 CrossRef CAS.
- X. Cong, A. Li, F. Guo, H. Qin, X. Zhang, W. Wang and W. Xu, Construction of CdS@g–C3N4 heterojunction photocatalyst for highly efficient degradation of gaseous toluene, Sci. Total Environ, 2024, 913, 169777 CrossRef CAS PubMed.
- H. Ullah, Z. Haneef, A. Ahmad, I. S. Butler, R. N. Dara and Z. Rehman, MoS2 and CdS photocatalysts for water decontamination: A review, Inorg. Chem. Commun., 2023, 153, 110775 CrossRef CAS.
- S.-R. Kim and W.-K. Jo, Application of a photostable silver-assisted Z-scheme NiTiO3 nanorod/g-C3N4 nanocomposite for efficient hydrogen generation, Int. J. Hydrogen Energy, 2019, 44, 801–808 CrossRef CAS.
- B. Su, H. Huang, Z. Ding, M. B. J. Roeffaers, S. Wang and J. Long, S-scheme CoTiO3/Cd9.51Zn0.49S10 heterostructures for visible-light driven photocatalytic CO2 reduction, J. Mater. Sci. Technol., 2022, 124, 164–170 CrossRef CAS.
- S. Wang, B. Y. Guan, X. Wang and X. W. D. Lou, Formation of Hierarchical Co9S8@ZnIn2S4 Heterostructured Cages as an Efficient Photocatalyst for Hydrogen Evolution, J. Am. Chem. Soc., 2018, 140, 15145–15148 CrossRef CAS PubMed.
- R. Ye, H. Fang, Y.-Z. Zheng, N. Li, Y. Wang and X. Tao, Fabrication of CoTiO3/g-C3N4 Hybrid Photocatalysts with Enhanced H2 Evolution: Z-Scheme Photocatalytic Mechanism Insight, ACS Appl. Mater. Interfaces, 2016, 8, 13879–13889 CrossRef CAS PubMed.
- T. Zhang, W. Wang, X. Jiang, H. Wang, Z.-H. He, Y. Yang, K. Wang, Z.-T. Liu and B. Han, Fabricating CdS/Ag/BiVO4 Z-heterojunction for solvent-free photocatalytic oxidation of amines, Green Chem., 2024, 26, 3338–3345 RSC.
- J. H. Wei, D. D. Han, J. T. Bi and J. B. Gong, Fe-doped ilmenite CoTiO3 for antibiotic removal: Electronic modulation and enhanced activation of peroxymonosulfate, Chem. Eng. J., 2021, 423, 130165 CrossRef CAS.
- X. X. Lu, L. J. Wang, Q. Li, R. Luo, J. F. Zhang and Z. F. Tian, Construction of CoTiO3/BiOI p-n heterojunction with nanosheets-on microrods structure for enhanced photocatalytic degradation of organic pollutions, Adv. Powder Technol., 2022, 33, 103354 CrossRef CAS.
- M. Mousavi and J. B. Ghasemi, Novel visible-light-responsive Black-TiO2/CoTiO3 Z-scheme heterojunction photocatalyst with efficient photocatalytic performance for the degradation of different organic dyes and tetracycline, J. Taiwan Inst. Chem. Eng., 2021, 121, 168–183 CrossRef CAS.
- Y. F. Jia, S. P. Li, H. Ma, J. Z. Gao, G. Q. Zhu, F. C. Zhang, J. Y. Park, S. Cha, J. S. Bae and C. Liu, Oxygen vacancy rich Bi2O4-Bi4O7-BiO2 composites for UV-vis-NIR activated high efficient photocatalytic degradation of bisphenol A, J. Hazard. Mater., 2020, 382, 121121 CrossRef CAS PubMed.
- W. Jin, D. K. Liu, L. Y. Zhang, Q. Sun, Y. S. Wang, E. Z. Liu, X. Y. Hu and H. Miao, TiO2 spatially confined growth of Sb2(S, Se)3@TiO2 NT heterojunction photoanodes and their photoelectrochemical properties, Catal. Sci. Technol., 2023, 13, 7046–7058 RSC.
- Z. R. Zhang, R. T. Guo, C. Xia, C. F. Li and W. G. Pan, B-TiO2/CuInS2 photocatalyst based on the synergistic effect of oxygen vacancy and Z-scheme heterojunction for improving photocatalyst CO2 reduction, Sep. Purif. Technol., 2023, 323, 124416 CrossRef.
- Z. Zhuang, Y. Li, Z. Li, F. Lv, Z. Lang, K. Zhao, L. Zhou, L. Moskaleva, S. Guo and L. Mai, MoB/g-C3N4 Interface Materials as a Schottky Catalyst to Boost Hydrogen Evolution, Angew. Chem., Int. Ed., 2018, 57, 496–500 CrossRef CAS PubMed.
- J. W. Fu, Q. L. Xu, J. X. Low, C. J. Jiang and J. G. Yu, Ultrathin 2D/2D WO3/g-C3N4 step-scheme H2-production photocatalyst, Appl. Catal., B, 2019, 243, 556–565 CrossRef CAS.
- J. G. Yu, S. H. Wang, J. X. Low and W. Xiao, Enhanced photocatalytic performance of direct Z-scheme g-C3N4/TiO2 photocatalysts for the decomposition of formaldehyde in air, Phys. Chem. Chem. Phys., 2013, 15, 16883–16890 RSC.
- J. W. Fu, C. B. Bie, B. Cheng, C. J. Jiang and J. G. Yu, Hollow CoSx Polyhedrons Act as High-Efficiency Cocatalyst for Enhancing the Photocatalytic Hydrogen Generation of g-C3N4, ACS Sustain, Chem. Eng., 2018, 6, 2767–2779 CAS.
- H. Yang, X. Li, T. Zhao, Q. Peng, W. Yang, J. Cao, Y. Zheng, C. Li and J. Pan, The CdS/CaTiO3 cubic core-shell composite towards enhanced photocatalytic hydrogen evolution and photodegradation, Int. J. Hydrogen Energy, 2023, 48, 21788–21798 CrossRef CAS.
- A. Meng, S. Zhou, D. Wen, P. Han and Y. Su, g-C3N4/CoTiO3 S-scheme heterojunction for enhanced visible light hydrogen production through photocatalytic pure water splitting, Chin. J. Catal., 2022, 43, 2548–2557 CrossRef CAS.
- K. Wangkawong, S. Suntalelat, D. Tantraviwat and B. Inceesungvorn, Novel CoTiO3/Ag3VO4 Composite: Synthesis, Characterization and Visible-light-driven Photocatalytic Activity, Mater. Lett., 2014, 133, 119–122 CrossRef CAS.
- J. Zheng and L. Zhang, One-step in situ formation of 3D hollow sphere-like V2O5 incorporated Ni3V2O8 hybrids with enhanced photocatalytic performance, J. Hazard. Mater., 2021, 416, 125934 CrossRef CAS PubMed.
- A. Meng, L. Zhang, B. Cheng and J. Yu, TiO2–MnOx–Pt Hybrid Multiheterojunction Film Photocatalyst with Enhanced Photocatalytic CO2-Reduction Activity, ACS Appl. Mater. Interfaces, 2019, 11, 5581–5589 CrossRef CAS PubMed.
- L. Chen, D. W. Meng, X. L. Wu, A. Q. Wang, J. X. Wang, Y. Q. Wang and M. H. Yu, In Situ Synthesis of V4+ and Ce3+ Self-Doped BiVO4/CeO2 Heterostructured Nanocomposites with High Surface Areas and Enhanced Visible-Light Photocatalytic Activity, J. Phys. Chem. C, 2016, 120, 18548–18559 CrossRef CAS.
- J. Zhang, J. Ma, X. Sun, Z. Yi, T. Xian, X. Wu, G. Liu, X. Wang and H. Yang, Construction of Z-Scheme Ag2MoO4/ZnWO4 Heterojunctions for Photocatalytically Removing Pollutants, Langmuir, 2023, 39, 1159–1172 CrossRef CAS.
- Y. X. Geng, D. Y. Chen, N. J. Li, Q. F. Xu, H. Li, J. H. He and J. M. Lu, Z-scheme 2D/2D α-Fe2O3/g-C3N4 heterojunction for photocatalytic oxidation of nitric oxide, Appl. Catal., B, 2021, 280, 119409 CrossRef CAS.
- L. Tang, C. Y. Feng, Y. C. Deng, G. M. Zeng, J. J. Wang, Y. N. Liu, H. P. Peng and J. J. Wang, Enhanced photocatalytic activity of ternary Ag/g-C3N4/NaTaO3 photocatalysts under wide spectrum light radiation: The high potential band protection mechanism, Appl. Catal., B, 2018, 230, 102–114 CrossRef CAS.
- H. J. Ding, L. P. Bao, Y. Su, Y. Q. Li, G. D. Xu, C. H. Dai and C. Zeng, Core-shell structured Z-scheme Ag2S/AgIO3 composites for photocatalytic organic pollutants degradation, J. Environ. Manag., 2022, 313, 115008 CrossRef CAS PubMed.
- W. J. Wang, Z. T. Zeng, G. M. Zeng, C. Zhang, R. Xiao, C. Y. Zhou, W. P. Xiong, Y. Yang, L. Lei, Y. Liu, D. L. Huang, M. Cheng, Y. Y. Yang, Y. K. Fu, H. Z. Luo and Y. Zhou, Sulfur doped carbon quantum dots loaded hollow tubular g-C3N4 as novel photocatalyst for destruction of Escherichia coli and tetracycline degradation under visible light, Chem. Eng. J., 2019, 378, 122132 CrossRef CAS.
- M. Chen, C. S. Guo, S. Hou, J. P. Lv, Y. Zhang, H. Zhang and J. Xu, A novel Z-scheme AgBr/P- g-C3N4 heterojunction photocatalyst: Excellent photocatalytic performance and photocatalytic mechanism for ephedrine degradation, Appl. Catal., B, 2020, 266, 118614 CrossRef CAS.
- S. H. Cao, Y. Q. Zhao, J. Y. Guo, M. J. Fu, X. D. Yu, L. X. Ren, H. Chen and F. Jiang, Dual role of nickel acetylacetonate in visible light enhanced hydrogen production and antibiotics degradation of nickel/nickel oxides embedded-graphitic carbon nitrides, Int. J. Hydrogen Energy, 2024, 55, 882–892 CrossRef CAS.
|
This journal is © the Owner Societies 2024 |
Click here to see how this site uses Cookies. View our privacy policy here.