Revealing the evolution of local structures in the formation process of alkaline earth metal cation-containing zeolites from glasses†
Received
12th October 2023
, Accepted 23rd November 2023
First published on 7th December 2023
Abstract
Alkaline earth metal cations are ubiquitously present in natural zeolites but less exploited in synthetic zeolites due to their low solubility in water, and hence it remains elusive how they contribute to zeolite formation. Herein, harmotome, a PHI-type zeolite with Ba2+, is readily synthesized from a Ba-containing aluminosilicate glass. This glass-to-zeolite transformation process, in particular the structure-regulating role of Ba2+, is investigated by anomalous X-ray scattering and high-energy X-ray total scattering techniques. The results demonstrate that the steady Ba2+–aluminosilicate interactions not only help prevent the precipitation of barium species under alkaline synthetic conditions, but also dictate the local structures with distinct interatomic distances between the Ba2+ and the surrounding aluminosilicate species throughout the transformation process, which lead to the successful formation of harmotome without detectable impurities. This study highlights the usefulness of the comprehensive X-ray scattering techniques in revealing the formation scheme of the zeolites containing specific metal species. In addition, a promising alternative approach to design and synthesize zeolites with unique compositions and topologies by using well-crafted glasses with suitable metal cation dopants is demonstrated.
1. Introduction
Zeolites are crystalline microporous aluminosilicate minerals constructed by interconnected SiO4 and AlO4 tetrahedra and have been widely utilized as adsorbents, heterogeneous catalysts and ion-exchangers.1 By mimicking the geological conditions where natural zeolites form, i.e., a hydrothermal method, various zeolites with diverse topological structures have been artificially synthesized from a mixture of Si sources, Al sources, mineralizers, and, if necessary, structure-directing agents (SDAs) in water.2,3 However, among the zeolites discovered hitherto, 19 types remain unsynthesized but only exist in nature, and interestingly, most of them contain extra-framework alkaline earth metal cations (Table S1, ESI†).4 Different from the artificial synthesis of zeolites, which usually involves organic SDAs (OSDAs), the crystallization of natural zeolites occurs under OSDA-free conditions, which hints at the potential role of inorganic cations in directing the formation of zeolites with specific framework structures. Several investigations focusing on the role of alkali metal cations have been carried out.5–7 For example, ABW-, CHA-, MER- and ANA-type zeolites could be obtained by hydrothermally converting FAU-type zeolites in the presence of Li+, K+, Rb+ and Cs+, respectively,8 which suggested strong structure-directing effects of these alkali metal cations. Compared with alkali metal cations, alkaline earth metal cations have much lower solubility under alkaline conditions in water (Table S2, ESI†), and thus the applicable methods for synthesizing alkaline earth metal cation-containing zeolites are limited.9,10 By hydrothermal conversion of alkaline earth metal cation-exchanged zeolite Y and zeolite P1 in the reactants containing corresponding hydroxides, a series of zeolites containing alkaline earth metal cations (Ba2+, Ca2+ and Sr2+) with different framework structures was obtained.11,12 Nevertheless, the contents of these alkaline earth metal species in the products were obscured, and their potential structure-directing role was far from being understood.13
Natural zeolites are usually derived from volcanic glasses rather than physically mixed oxides.14 In the case of aluminosilicate glasses, an amorphous matrix of SiO4 and AlO4 tetrahedra is already formed, and their transformation to crystalline zeolites is found viable under relatively lower alkaline conditions, helping to avoid the precipitation of metal species.9 More importantly, alkali and alkaline earth metal cations are usually present in the aluminosilicate glasses, working as charge compensators or network modifiers, and the cations with different cation field strengths (Z/r2, where Z is the cation charge and r is the cation radius in Å) can interact with the framework tetrahedra and modulate the structure of glasses.15,16 Although the syntheses of zeolites from glasses have been reported,14,17,18 to the best of our knowledge, the structural relationship between the starting glass and the resultant zeolite has never been investigated in detail. This may open up new avenues for creating zeolites with unique compositions and topologies by using glass as the starting material, coupled with the selection of suitable inorganic cations as SDAs.
In recent years, high-energy X-ray total scattering (HEXTS) measurement combined with pair distribution function (PDF) analysis has been proven to be an effective approach to track the structural evolution of the products, regardless of their amorphous or crystalline states, during the synthesis of zeolites.19,20 However, it gives the correlations of all the atomic pairs in the zeolites, and therefore it is difficult to extract the structural information related to the specific atoms that may play key roles in the formation processes of zeolites, e.g., the extra-framework alkali metal cations and the transition metal atoms in the framework of metallosilicate zeolites.21,22 Very recently, the anomalous X-ray scattering (AXS) technique was tentatively introduced to explore the formation scheme of zeolites.23 By measuring the scattering patterns using two different X-ray energies near the absorption edge of the target atom, atom-selective PDF analysis can be realized due to the significant change in its scattering factor (details are described in the Experimental section). The complementary use of HEXTS and AXS techniques is expected to help us make a further step in the comprehensive understanding of the formation mechanism of zeolites.
In this study, pure harmotome, a Ba-dominant analogue of zeolite phillipsite (PHI topology, Fig. S1, ESI†) with a typical composition of 1BaO
:
1Al2O3
:
6SiO2,11 is successfully synthesized by hydrothermally treating a pre-prepared Ba-containing aluminosilicate glass (Ba-glass, 1BaO
:
1Al2O3
:
6SiO2) in a NaOH solution (pH 13). With the help of the combined X-ray characterization techniques, i.e., AXS and HEXTS, the structure-regulating role of Ba2+ that dictates the local structures with distinct interatomic distances between the Ba2+ and the surrounding aluminosilicate species throughout the transformation process is discerned, and the glass-to-zeolite transformation scheme is unveiled.
2. Experimental section
2.1. Materials
Silicon dioxide (SiO2), sodium hydroxide (NaOH), and barium oxide (BaO) were purchased from FUJIFILM Wako Pure Chemical Corporation. Aluminum oxide (Al2O3), barium carbonate (BaCO3), and calcium carbonate (CaCO3) were obtained commercially from Sigma-Aldrich Corporation. Fumed silica (SiO2, Cab-O-Sil, M5) was the commercial product of Cabot Corporation.
2.2. Preparation of alkaline earth element-containing glass
The well-grinded mixture of SiO2, Al2O3 and alkaline earth element sources (BaCO3 or CaCO3) was put in a platinum crucible and melted at 1680 °C in the air for 30 min, followed by a quick quenching to room temperature. The obtained glass was coarsely pulverized and then melted again according to the aforementioned procedures. The resultant glass was grinded to a size of less than 40 μm using an agate mortar.
2.3. Synthesis of Ba-containing zeolites
Typically, the prepared glass (40 mg) was added into the NaOH solution (2 g, pH 13), which was transferred into a carbon-coated reactor (Fig. S2, ESI†) and hydrothermally treated at 150 °C for a certain period. In a controlled experiment, the well-grinded mixture (40 mg) of SiO2 (fumed silica), Al2O3 and BaO was alternatively used as the starting material.
2.4. Characterization
X-ray diffraction (XRD) patterns were collected using a Rigaku Ultima IV diffractometer using a Cu Kα radiation source (40 kV, 40 mA). The solid-state magic angle spinning nuclear magnetic resonance (MAS NMR) spectra of 27Al were recorded using a JNM-ECA 500 spectrometer (11.75 T, JEOL) at 130.33 MHz with a π/2 pulse width of 3.2 μs, a relaxation delay of 5 s and a spinning rate of 14 kHz, or using a JNM-ECA 800 spectrometer (18.79 T, JEOL) at 208.49 MHz with a π/10 pulse width of 0.1 μs, a relaxation delay of 2 s and a spinning rate of 20 kHz. The morphologies of the products were observed using a field-emission scanning electron microscope (FE-SEM, S-900, Hitachi). The chemical compositions of the samples were analyzed by inductively coupled plasma atomic emission spectroscopy (ICP-AES, Thermo iCAP 6300). The samples were dissolved in hydrofluoric acid solutions before the measurements.
2.5. X-ray scattering measurements
The HEXTS measurements were performed using the BL04B2 high-energy XRD beamline (SPring-8, Japan) with a horizontal two-axis diffractometer at room temperature. Powdered samples were put in Kapton polyimide film tubes. The energies of the incident X-ray were 112.8 keV and 61.4 keV for the samples with and without Ba, respectively. The maximum Q (Q = 4π
sin
θ/λ) collected here was 25.0 Å−1. The obtained data were processed by well-established analysis procedures, including absorption, background and Compton scattering corrections, and subsequently normalized to give the Faber–Ziman total structure factor, S(Q) (eqn (1)), which was finally used to calculate the reduced PDF, G(r), according to eqn (2), where I(Q) is the coherent scattering intensity per atom, and f(Q) is the atomic scattering factor.24 In addition, the reduced PDF, G(r), is related to the PDF, g(r), through eqn (3), where ρ0 is the atomic density. | 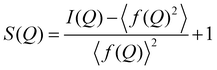 | (1) |
|  | (2) |
| G(r) = 4πρ0r[g(r) − 1] | (3) |
AXS measurements were performed using the BL22XU hard X-ray undulator beamline (SPring-8, Japan) with an imaging plate detector (R-AXIS V, Rigaku) at room temperature. Powdered samples were added into Kapton polyimide film tubes. The energies of the incident X-ray were 36.5 keV and 36.9 keV as the far-edge energy (Efar) and near-edge energy (Enear) of the Ba K-absorption edge (37.4 keV), respectively. The maximum Q collected here was 12.3 Å−1. When the energy of the incident X-ray is close to the absorption edge of element A in the system, the atomic scattering factor becomes energy-dependent due to the dispersion effect (eqn (4)), where f0A(Q) is the atomic scattering factor at the energy sufficiently far from the absorption edge, and
and
are the real and imaginary parts of the anomalous dispersion correction, respectively.25 Accordingly, the differential structure factor, SA(Q), and the differential reduced PDF, GA(r), can be obtained by eqn (5) and (6), respectively.26
|  | (4) |
| 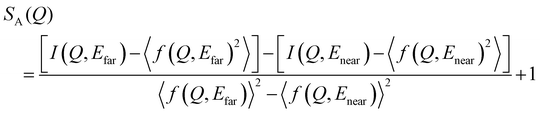 | (5) |
|  | (6) |
The details of the Reverse Monte Carlo (RMC) simulations of the glasses based on the HEXTS and AXS data and the simulations of the reduced PDFs of the zeolites based on the structural models are described in the ESI.†
3. Results and discussion
3.1. Synthesis of harmotome
The synthesis of harmotome was first attempted using a physical mixture of SiO2, Al2O3 and BaO (Ba-PM) as the starting material. According to the XRD patterns (Fig. S3, ESI†), Al2O3 can hardly be dissolved due to the low alkalinity (pH 13), and the presence of BaCO3 as well as other unknown phases is confirmed. The formation of harmotome is not observed even when the hydrothermal synthesis time is prolonged to 7 days.
In contrast, the Ba-glass, consisting of an amorphous aluminosilicate matrix with Ba2+ cations, is a suitable starting material for the synthesis of harmotome. As shown in the XRD patterns (Fig. 1(a)), the formation of harmotome begins within 1 day, and highly crystalline harmotome without detectable impurity can be obtained after 3 days. The local coordination environment of Al atoms in the Ba-glass precursor and the products was monitored by the solid-state 27Al MAS NMR spectroscopy (Fig. 1(b)). Al atoms usually experience a strong quadrupolar interaction in the disordered coordination environment in the amorphous aluminosilicate glass, resulting in the 27Al MAS NMR peak with an asymmetric tailing to the high field side (acquired at 11.75 T).27,28 To alleviate quadrupolar broadening, the 27Al MAS NMR spectrum of the Ba-glass was further acquired at a higher field strength of 18.79 T, and the fitting result obtained by the software dmfit substantiates that almost all the Al atoms (99.5%) are tetrahedrally incorporated in the aluminosilicate network (Fig. S4, ESI†).29,30 Along with the hydrothermal treatment, a new resonance signal appears with a chemical shift of ca. 55 ppm, accompanied by the attenuation of the broad asymmetric signal (Fig. 1(b)), which indicates the transition of Al atoms to a more ordered coordination environment during the crystallization of harmotome. In the spectrum of the crystalline harmotome product (3 d), only a single sharp signal attributed to the tetrahedrally coordinated Al atoms in the zeolite crystals can be detected. The 27Al MAS NMR spectra track well the transformation process from the amorphous glass to the crystalline zeolite.
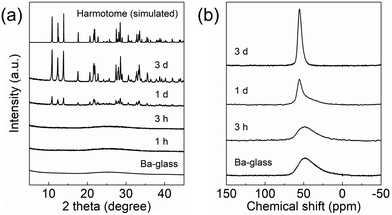 |
| Fig. 1 (a) XRD patterns and (b) 27Al MAS NMR spectra (acquired at 11.75 T) of the Ba-glass and the products synthesized from the Ba-glass for different periods. | |
The FE-SEM images record the changes in the surface morphology of the products synthesized for different periods. Different from the Ba-glass precursor (Fig. 2(a)), which possesses a smooth surface, the surface of the product hydrothermally treated for 3 h becomes uneven (Fig. 2(b)). Further prolongation of the synthesis time to 1 day results in a severely etched surface (Fig. S5, ESI†), and at the same time, the presence of faceted particles is confirmed (Fig. 2(c)), which can be assigned to the newly formed harmotome as verified in the XRD pattern (Fig. 1(a)). The crystalline harmotome product consists of parallel-aligned interpenetrating aggregates of prismatic crystals (Fig. 2(d)), similar to the natural harmotome mineral.31 These observations are consistent with the previous reports that the rapid dissolution of the glass occurs in the alkaline solution with a suitable pH, and the condensation of the aluminosilicate species in the solution leads to the formation and precipitation of zeolites.9,32 During such a dissolution–reprecipitation process in the pH 13 NaOH solution, the release of the Ba2+ cations into the liquid phase is negligible, and the chemical compositions of the products synthesized for different periods are similar according to the results of ICP-AES (Table 1). Because the tetrahedral framework Al atoms are the predominant Al species in the Ba-glass (Fig. S4, ESI†) and there are no cations other than Ba2+ with a molar ratio of Ba/Al = 0.5, the Ba2+ cations must act as the charge compensator,33 and therefore strongly adhere to the negatively charged aluminosilicate network. In contrast, BaCO3, which has a much lower solubility than that of Ba(OH)2, is formed along with the crystallization of harmotome when the Ba-glass is hydrothermally treated with the NaOH solution at a higher pH of 14 (Fig. S6, ESI†). Because of the drastic hydrolysis of the aluminosilicate network, the Ba2+ cations are significantly released into the liquid phase and react with the CO2 dissolved in the alkaline reactant.13
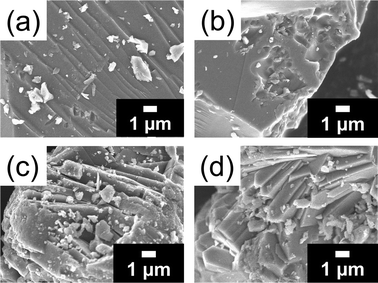 |
| Fig. 2 FE-SEM images of the (a) Ba-glass, and the products synthesized for (b) 3 h, (c) 1 d and (d) 3 d. | |
Table 1 Chemical compositions of the products synthesized from the Ba-glass for different periods determined by ICP-AES
Synthesis time |
Si/Al |
Ba/Al |
0 h (Ba-glass) |
3.0 |
0.52 |
1 h |
3.0 |
0.51 |
3 h |
3.1 |
0.53 |
1 d |
2.8 |
0.51 |
3 d |
2.9 |
0.52 |
3.2. Role of alkaline earth metal cations
To understand the role of Ba2+ cations in this glass-to-zeolite transformation process, the local structures around the Ba2+ cations in the Ba-glass and harmotome were investigated. The differential reduced PDF pattern, GBa(r), of the Ba-glass derived from the AXS measurement near the Ba K-absorption edge (Fig. 3a) displays two legible peaks located at ca. 2.8 Å and 3.7 Å, which can be ascribed to the closest Ba–O and Ba–T (T = Si and Al) correlations, respectively, as corroborated by the RMC simulation of the Ba-glass (Fig. S7(c), ESI†). When the Ba-glass is hydrothermally treated for a short period (1–3 h), GBr(r) remains almost unchanged, except for the broadening of the Ba–T peak toward the longer interatomic distance. The simulated GBa(r) of the harmotome (Fig. 3(a) and Fig. S8(a), ESI†) also shows a similar double-peak pattern in the short interatomic distance region (2.0–4.5 Å). Considering the previously reported structural model34 (Fig. 3(b) and (c)), the Ba2+ cation is coordinated by five framework oxygen (OF) atoms in an eight-membered ring (8R) with a Ba–OF interatomic distance of 2.9–3.1 Å, and the interatomic distances between the Ba2+ cation and the adjacent framework T atoms are regulated in the range of 3.7–4.0 Å. These short-range interatomic distances of the Ba–O and Ba–T correlations do not change significantly throughout the transformation process from the glass to the zeolite. On the other hand, the Ba–Ba pair is noticeably distanced from ca. 5.2 Å in the Ba-glass to above 8.4 Å in the harmotome. Because the Ba2+ cations are individually coordinated by the aluminosilicate species, the elongation of the Ba–Ba pair can be understood by the rearrangement of the Ba-aluminosilicate components and the lower number density of the harmotome possessing the microporous framework compared with that of the Ba-glass.
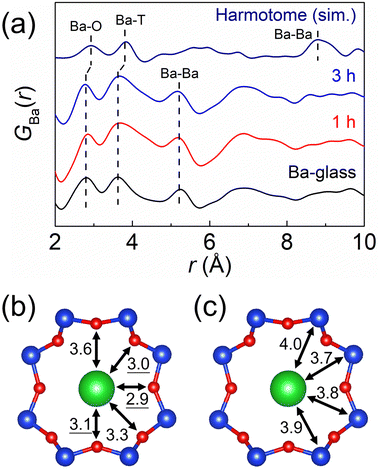 |
| Fig. 3 (a) Differential reduced PDFs, GBa(r), of the Ba-glass and the products synthesized from the Ba-glass for 1 h and 3 h derived from the AXS data obtained near the Ba K-absorption edge, and simulated (sim.) GBa(r) of harmotome. Interatomic distances of (b) Ba–O and (c) Ba–T pairs in the Ba-containing 8R in harmotome (unit: Å). Blue: T (Si or Al); red: O; green: Ba. The underlined values indicate the interatomic distances between the Ba2+ cation and the framework oxygen atoms coordinating to it (<3.2 Å). | |
For comparison, a Ca-containing aluminosilicate glass (Ca-glass, 1CaO
:
1Al2O3
:
6SiO2) was also prepared, with the same composition as that of the Ba-glass except for the alkaline earth metal cation incorporated. Again, the interatomic distances of the closest Ca–O and Ca–T correlations in the Ca-glass (Fig. S9(b), ESI†) are similar to those (ca. 2.5–2.6 Å for Ca–O, the OF atoms coordinating to the Ca2+ cation, and ca. 3.2 Å for Ca–T, the T atoms connecting two OF atoms coordinating to the Ca2+ cation) in the phillipsite-Ca (Fig. S9(c), ESI†), another kind of natural PHI-type zeolite with Ca2+ as the predominant divalent extra-framework cation.35 In this study, we will not discuss the formation process of phillipsite-Ca in detail.
The aforementioned results suggest that the alkaline earth metal cations indeed have a strong ability to arrange the aluminosilicate species surrounding them, no matter in the glass precursors or in the zeolite products, which is assumed to be highly related to their different sizes and cation field strengths.36,37 Because of the larger size and the lower cation field strength of Ba2+ compared with those of Ca2+, the Ba2+ cations are less attractive to the framework oxygen atoms, resulting in the longer interatomic distances between the Ba2+ cations and the oxygen atoms as well as the adjacent Si or Al atoms.37 Furthermore, such specific local distances governed by the alkaline earth metal cations seem to be hardly disturbed, which consequently leads to the different locations of the Ba2+ and Ca2+ sites in the harmotome and the phillipsite-Ca, respectively (Fig. S10, ESI†), accommodating the distinct Ba–O/T and Ca–O/T interatomic distances. It is plausible that the steady and distinctive interactions with the aluminosilicates contribute largely to the structure-regulating ability of the alkaline earth metal cations, which further gives rise to different crystallization pathways to form various zeolites.
3.3. Formation scheme of harmotome from the glass
The structural changes of the aluminosilicate matrix during the transformation process from the Ba-glass to the harmotome were explored by the HEXTS technique (Fig. 4). In the case of the Ba-glass, the contributions of the T–O 2nd (ca. 4.0 Å) and T–T 2nd (ca. 4.0–5.0 Å) correlations (also discerned in the results of the RMC simulation, Fig. S7(c), ESI†) are derived from the deformed small-sized ring structures.38 Accompanying the crystallization of harmotome, ordered four-membered rings (4Rs) are formed, as indicated by the shoulder peak appearing at ca. 4.4–4.5 Å related to the T–T 2nd correlation. In addition, the peak located at ca. 5.4–5.9 Å is mainly due to the T–T 2nd correlation in the ordered 8Rs with and without Ba2+ cations. The intensified peak at ca. 3.7–4.3 Å can be attributed to the integrated contributions of the T–O 2nd correlations in the ordered 4Rs and 8Rs and the Ba–T correlation in the Ba-containing 8Rs.34 The structural evolutions of the local structures around Ba2+ (minor changes in the closest Ba–O and Ba–T interatomic distances) and the aluminosilicate matrix (formation of distinguishable ordered 4Rs and 8Rs) during the glass-to-zeolite transformation process are depicted well by the combined AXS and HEXTS techniques.
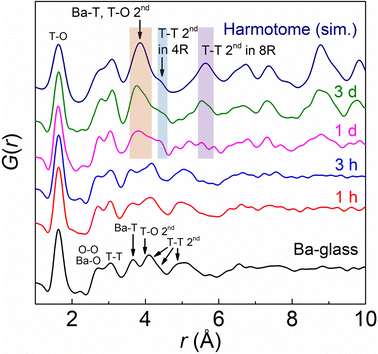 |
| Fig. 4 Total reduced PDFs, G(r), of the Ba-glass and the products synthesized from the Ba-glass for different periods obtained by HEXTS measurements, and simulated (sim.) G(r) of harmotome. | |
Based on the above-mentioned results, a scheme of the transformation process from the Ba-glass to the harmotome is proposed (Fig. 5). In the Ba-glass precursor, the Ba2+ cations are coordinated with the aluminosilicate matrix and regulate the local structures, forming the nearest Ba–O and Ba–T pairs with distinct interatomic distances. Under the suitable alkaline conditions, the bulk Ba-glass is dissolved readily into small fragments, while the local structures of the Ba–aluminosilicate components are preserved due to the steady interactions between the Ba2+ cations and the negatively charged aluminosilicates, which also prevents the formation of precipitates like BaCO3 and Ba(OH)2. Then, the ordered Ba-containing 8Rs, featuring the Ba–O and Ba–T interatomic distances similar to those in the precursor, are derived from these Ba-aluminosilicate components and reconnected with each other through the newly formed ordered 4Rs, which leads to the crystallization of harmotome.
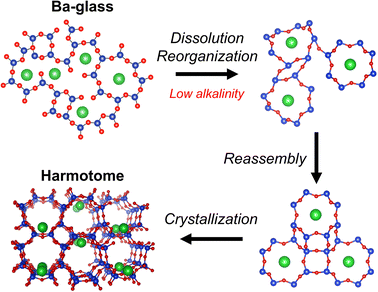 |
| Fig. 5 Schematic illustration of the transformation process from the Ba-glass to harmotome. Blue: T (Si or Al); red: O; green: Ba. The Ba–O bonds are not shown. The ordered Ba-containing 8Rs are derived from the Ba-aluminosilicate components that are dissolved from the Ba-glass, and are reconnected by the newly formed ordered 4Rs, which further leads to the crystallization of harmotome. | |
4. Conclusions
In this study, harmotome, a Ba-containing PHI-type aluminosilicate zeolite, is readily synthesized from the corresponding glass with the same composition. Glass serves as a promising precursor, whose local structures can be tuned by incorporating various alkaline earth metal cations and amorphous nature makes it possible to easily dissolve and reorganize under low-alkalinity conditions. By comprehensively employing the AXS and HEXTS techniques, the role of the Ba2+ cations and the structural evolutions involved in this glass-to-zeolite transformation process are figured out. The key to the successful synthesis can be attributed to the similarity of the local structures between the aluminosilicate matrix and the Ba2+ cations in both the glass precursor and the zeolite product, which is mainly governed by the intrinsic size and cation field strength of Ba2+. This study demonstrates the importance of the simultaneous development of the original synthetic methodology and the advanced characterization technique, and will stimulate the design and synthesis of novel zeolites with unique compositions and topologies under the premise of better understanding of the formation mechanism of zeolites.
Author contributions
Peidong Hu: writing – original draft, visualization, investigation, and data curation. Makiko Deguchi: formal analysis, investigation, and validation. Hiroki Yamada: methodology and funding acquisition. Kentaro Kobayashi: formal analysis. Koji Ohara: formal analysis and data curation. Sohei Sukenaga: investigation. Mariko Ando: investigation. Hiroyuki Shibata: investigation. Akihiko Machida: investigation and resources. Yutaka Yanaba: investigation. Zhendong Liu: supervision and writing – review and editing. Tatsuya Okubo: resources and writing – review and editing. Toru Wakihara: writing – review and editing, visualization, supervision, resources, project administration, funding acquisition, and conceptualization.
Conflicts of interest
There are no conflicts to declare.
Acknowledgements
This work was partially supported by the Japan Society for the Promotion of Science (JSPS), the Grants-in-Aid for Scientific Research (KAKENHI), the Grants-in-Aid for Transformative Research Areas (A) (JP20A206/20H05880), the Scientific Research (S) (JP21H05011 and JP23H05454) and the Early-Career Scientists (JP21K14708). The HEXTS and AXS experiments at SPring-8 were approved by the Japan Synchrotron Radiation Research Institute (Proposal No. 2017A1657, 2017A3751, 2017B3785, 2018A3751, 2018B2095, 2018B3783, 2019A2058, 2019B1572, 2019B2091, 2021A1455, 2022B1368 and 2022B2121) and were partially supported by the QST Advanced Characterization Nanotechnology Platform under the remit of the “Nanotechnology Platform” of Ministry of Education, Culture, Sports, Science and Technology (MEXT) of Japan (Proposal No. JPMXP09A17QS0029 and JPMXP09A18QS0038).
References
- V. Van Speybroeck, K. Hemelsoet, L. Joos, M. Waroquier, R. G. Bell and C. R. Catlow, Advances in theory and their application within the field of zeolite chemistry, Chem. Soc. Rev., 2015, 44, 7044–7111 RSC.
- C. S. Cundy and P. A. Cox, The hydrothermal synthesis of zeolites: history and development from the earliest days to the present time, Chem. Rev., 2003, 103, 663–701 CrossRef CAS PubMed.
- C. S. Cundy and P. A. Cox, The hydrothermal synthesis of zeolites: Precursors, intermediates and reaction mechanism, Microporous Mesoporous Mater., 2005, 82, 1–78 CrossRef CAS.
-
C. Baerlocher and L. B. McCusker, Database of Zeolite Structures, https://www.iza-structure.org/databases/, (accessed August 2023).
- C. Liu, W. Gu, D. Kong and H. Guo, The significant effects of the alkali-metal cations on ZSM-5 zeolite synthesis: From mechanism to morphology, Microporous Mesoporous Mater., 2014, 183, 30–36 CrossRef CAS.
- A. Nearchou and A. Sartbaeva, Influence of alkali metal cations on the formation of zeolites under hydrothermal conditions with no organic structure directing agents, CrystEngComm, 2015, 17, 2496–2503 RSC.
- D. Zhao, R. Szostak and L. Kevan, Role of alkali-metal cations and seeds in the synthesis of silica-rich heulandite-type zeolites, J. Mater. Chem., 1998, 8, 233–239 RSC.
- L. Van Tendeloo, E. Gobechiya, E. Breynaert, J. A. Martens and C. E. Kirschhock, Alkaline cations directing the transformation of FAU zeolites into five different framework types, Chem. Commun., 2013, 49, 11737–11739 RSC.
- S. Khodabandeh and M. E. Davis, Alteration of perlite to calcium zeolites, Microporous Mater., 1997, 9, 161–172 CrossRef CAS.
- U. Wirsching, Experiments on the Hydrothermal Formation of Calcium Zeolites, Clays Clay Miner., 1981, 29, 171–183 CrossRef CAS.
- S. Khodabandeh and M. E. Davis, Zeolites P1 and L as precursors for the preparation of alkaline-earth zeolites, Microporous Mater., 1997, 12, 347–359 CrossRef CAS.
- O. Chiyoda and M. E. Davis, Hydrothermal conversion of Y-zeolite using alkaline-earth cations, Microporous Mesoporous Mater., 1999, 32, 257–264 CrossRef CAS.
- Y. Liang, A. J. Jacobson and J. D. Rimer, Synthesis of PHI-type zeolite harmotome using barium as an inorganic structure-directing agent inspired from natural mineral compositions, Microporous Mesoporous Mater., 2023, 353, 112511 CrossRef CAS.
- M. R. Ghiara and C. Petti, Chemical alteration of volcanic glasses and related control by secondary minerals: Experimental studies, Aquat. Geochem., 1996, 1, 329–354 CrossRef.
- K. Aoki, T. Sakamaki, T. Ohashi, O. Ikeda and A. Suzuki, Effects of alkali and alkaline-earth cations on the high-pressure sound velocities of aluminosilicate glasses, J. Phys. Chem. Minerals, 2020, 47, 28 CrossRef CAS.
- H. Sreenivasan, P. Kinnunen, E. Adesanya, M. Patanen, A. M. Kantola, V.-V. Telkki, M. Huttula, W. Cao, J. L. Provis and M. Illikainen, Field Strength of Network-Modifying Cation Dictates the Structure of (Na–Mg) Aluminosilicate Glasses, Front. Mater., 2020, 7, 267 CrossRef.
- M. Tsujiguchi, T. Kobashi, M. Oki, Y. Utsumi, N. Kakimori and A. Nakahira, Synthesis and characterization of zeolite A from crushed particles of aluminoborosilicate glass used in LCD panels, J. Asian Ceram. Soc., 2014, 2, 27–32 CrossRef.
- H. Ghobarkar, O. Schaf and P. Knauth, Zeolite Synthesis by the High-Pressure Hydrothermal Method: Synthesis of Natural 6-Ring Zeolites with Different Void Systems, Angew. Chem., Int. Ed., 2001, 40, 3831–3833 CrossRef CAS PubMed.
- C.-T. Chen, K. Iyoki, P. Hu, H. Yamada, K. Ohara, S. Sukenaga, M. Ando, H. Shibata, T. Okubo and T. Wakihara, Reaction Kinetics Regulated Formation of Short-Range Order in an Amorphous Matrix during Zeolite Crystallization, J. Am. Chem. Soc., 2021, 143, 10986–10997 CrossRef CAS PubMed.
- A. Minami, P. Hu, Y. Sada, H. Yamada, K. Ohara, Y. Yonezawa, Y. Sasaki, Y. Yanaba, M. Takemoto, Y. Yoshida, T. Okubo and T. Wakihara, Tracking Sub-Nano-Scale Structural Evolution in Zeolite Synthesis by In Situ High-Energy X-ray Total Scattering Measurement with Pair Distribution Function Analysis, J. Am. Chem. Soc., 2022, 144, 23313–23320 CrossRef CAS PubMed.
- N. Hikichi, K. Iyoki, Y. Naraki, Y. Yanaba, K. Ohara, T. Okubo and T. Wakihara, Role of sodium cation during aging process in the synthesis of LEV-type zeolite, Microporous Mesoporous Mater., 2019, 284, 82–89 CrossRef CAS.
- T. Iida, M. Sato, C. Numako, A. Nakahira, S. Kohara, T. Okubo and T. Wakihara, Preparation and characterization of Silicalite-1 zeolites with high manganese contents from mechanochemically pretreated reactants, J. Mater. Chem. A, 2015, 3, 6215–6222 RSC.
- H. Yamada, H. Horikawa, C. Anand, K. Ohara, T. Ina, A. Machida, S. Tominaka, T. Okubo, Z. Liu, K. Iyoki and T. Wakihara, Atom-Selective Analyses Reveal the Structure-Directing Effect of Cs Cation on the Synthesis of Zeolites, J. Phys. Chem. Lett., 2023, 14, 3574–3580 CrossRef CAS PubMed.
- X. Wang, S. Tan, X.-Q. Yang and E. Hu, Pair distribution function analysis: Fundamentals and application to battery materials, Chin. Phys. B, 2020, 29, 028802 CrossRef CAS.
- Y. Waseda, K. Sugiyama and T. Kawamata, Nanometer-Sized Crystalline Clusters of IGZO Films Determined from the Grazing Incidence X-ray Scattering and Anomalous X-ray Scattering Data Combined with Reverse Monte Carlo Simulations, Mater. Trans., 2018, 59, 1691–1700 CrossRef CAS.
- C. Park, M. Saito, Y. Waseda, N. Nishiyama and A. Inoue, Structural Study of Pd-Based Amorphous Alloys with Wide Supercooled Liquid Region by Anomalous X-ray Scattering, Mater. Trans., JIM, 1999, 40, 491–497 CrossRef CAS.
- S. K. Lee and J. F. Stebbins, The Structure of Aluminosilicate Glasses:
High-Resolution 17O and 27Al MAS and 3QMAS NMR Study, J. Phys. Chem. B, 2000, 104, 4091–4100 CrossRef CAS.
- J. B. d'Espinose de Lacaillerie, C. Fretigny and D. Massiot, MAS NMR spectra of quadrupolar nuclei in disordered solids: the Czjzek model, J. Magn. Reson., 2008, 192, 244–251 CrossRef PubMed.
- K. Chen, Z. Gan, S. Horstmeier and J. L. White, Distribution of Aluminum Species in Zeolite Catalysts: 27Al NMR of Framework, Partially-Coordinated Framework, and Non-Framework Moieties, J. Am. Chem. Soc., 2021, 143, 6669–6680 CrossRef CAS PubMed.
- D. R. Neuville, L. Cormier, V. Montouillout, P. Florian, F. Millot, J. C. Rifflet and D. Massiot, Amorphous materials: Properties, structure, and durability: Structure of Mg- and Mg/Ca aluminosilicate glasses: 27Al NMR and Raman spectroscopy investigations, Am. Mineral., 2008, 93, 1721–1731 CrossRef CAS.
- M. Mattioli and M. Cenni, Mineralogical dataset of natural zeolites from Lessini Mounts, Northern Italy: Analcime, natrolite, phillipsite and harmotome chemical composition, Data Brief, 2020, 31, 105791 CrossRef PubMed.
- S. Gin, P. Jollivet, M. Fournier, C. Berthon, Z. Wang, A. Mitroshkov, Z. Zhu and J. V. Ryan, The fate of silicon during glass corrosion under alkaline conditions: A mechanistic and kinetic study with the International Simple Glass, Geochim. Cosmochim. Acta, 2015, 151, 68–85 CrossRef CAS.
- B. Deng, Y. Shi, Q. Zhou and M. Bauchy, Revealing the structural role of MgO in aluminosilicate glasses, Acta Mater., 2022, 222, 117417 CrossRef CAS.
- E. Stuckenschmidt, H. Fuess and Å. Kvick, Investigation of the structure of harmotome by X-ray (293 K, 100 K) and neutron diffraction (15 K), Eur. J. Mineral., 1990, 2, 861–874 CrossRef CAS.
- R. Rinaldi, J. J. Pluth and J. V. Smith, Zeolites of the Phillipsite Family. Refinement of the Crystal Structures of Phillipsite and Harmotome, Acta Cryst., 1974, B30, 2426–2433 CrossRef.
- S. Li, H. Liu, F. Wu, Z. Chang and Y. Yue, Effects of alkaline-earth metal oxides on structure and properties of iron phosphate glasses, J. Non-Cryst. Solids, 2016, 434, 108–114 CrossRef CAS.
- A. Atila, E. M. Ghardi, S. Ouaskit and A. Hasnaoui, Atomistic insights into the impact of charge balancing cations on the structure and properties of aluminosilicate glasses, Phys. Rev. B, 2019, 100, 144109 CrossRef CAS.
- Q. Zhou, Y. Shi, B. Deng, J. Neuefeind and M. Bauchy, Experimental method to quantify the ring size distribution in silicate glasses and simulation validation thereof, Sci. Adv., 2021, 7, eabh1761 CrossRef CAS PubMed.
Footnotes |
† Electronic supplementary information (ESI) available. See DOI: https://doi.org/10.1039/d3cp04954j |
‡ Present address: State Key Laboratory of Chemical Engineering, Department of Chemical Engineering, Tsinghua University, Beijing 100084, China. |
|
This journal is © the Owner Societies 2024 |