DOI:
10.1039/D3AY02014B
(Paper)
Anal. Methods, 2024,
16, 873-883
Synthesis and evaluation of a chitosan nanomaterial as efficient sorbent for determination of fungicide residues in waters and wine by liquid chromatography high resolution mass spectrometry†
Received
13th November 2023
, Accepted 16th January 2024
First published on 19th January 2024
Abstract
In the present study a novel, cost-effective, environmentally friendly, and efficient analytical method was developed to analyze fungicide residues in water and wine. The method relies on the application of a newly developed sorbent nanomaterial named Nano-Cs-NAT, synthesized by modifying chitosan, a naturally occurring, low-cost polysaccharide, through grafting with two acrylic monomers and a cross-linker. Nano-Cs-NAT was introduced as analytical sorbent for Dispersive Micro Solid Phase Extraction (D-μ-SPE) before Liquid Chromatography-Orbitrap High-Resolution Mass Spectrometry (LC-Orbitrap HRMS) analysis of twelve fungicides commonly used in viticulture (among the others, triazoles, strobilurines and N-substituted imidazoles). Characterization of the sorbent was conducted, confirming the successful acrylation of chitosan. A multivariate approach was employed to optimize D-μ-SPE extraction parameters. The material was found to be highly effective in simultaneously purifying and concentrating the target analytes, enhancing overall analytical efficiency and sensitivity. The Nano-Cs-NAT-D-μ-SPE-LC-Orbitrap-HRMS method was thoroughly validated, exhibiting good recoveries (72–104%), reproducibility (average RSD ≤ 6%) and repeatability (average RSD ≤ 7%). It also achieved low limits of detection (LOD) in river water (average LOD of 0.04 μg L−1) and wine (average LOD of 0.72 μg kg−1), highlighting its potential for routine fungicide residue analysis. This developed method addresses environmental and food safety concerns by providing an efficient solution for detecting fungicide residues in waters and wine.
Introduction
Food and environmental safety are deeply intertwined, and this intricate interplay is evident in agriculture. Fungal diseases pose a remarkable challenge to crop growing, and viticulture, the cultivation of grapevine (Vitis vinifera), makes no exception. Fungicide application on grapevine represents a large portion of fungicide use: while viticulture constitutes only 3.3% of the total agricultural area of the EU, it has been proved to account for 67% of all fungicides applied to crops.1,2 The emergence of viticulture related fungicides in surface water and wine has raised significant concerns, with detected concentrations varying from tens to thousands ng L−1, influenced by factors such as the geographical location and the timing of sample collection.3,4 Residue analysis in wine is particularly challenging even when the most advanced spectrometric techniques are employed, due to the complex matrix composition, which includes high levels of anthocyanins, sugars, flavanols, and tannins.5 The pressing need for innovative methods to detect multiple contaminations in both environment and food is clear, and the research on new analytical sorbents and techniques to use in this field is crucial.6–9 Triple-quadrupole mass spectrometers coupled with liquid chromatography (LC) are commonly used in pesticide residue analyses, employing the multiple reaction monitoring (MRM) mode for enhanced specificity. However, the capability to simultaneously monitor compounds is constrained by dwell time, limiting peak data acquisition. In intricate matrices like food samples, despite purification steps, low-resolution mass spectrometry, such as Triple Q instruments, may struggle to distinguish matrix interferents from analytes, resulting in sensitivity loss and false positives. High resolution mass spectrometry (HRMS) instruments such as Orbitrap LC-MS/MS systems are making significant contributions in both environmental and food control laboratories due to their unparalleled resolving capability, overpassing triple quadrupole limitations (i.e. restricted capacity for analysing numerous compounds per assessment, dependency on the accessibility of reference standards, and incapability to screen for unknown substances10). Despite the constant development of instrumental techniques, the stage of sample pre-treatment remains crucial. The application of chitosan (poly(beta-(1,4)-2-amino-2-deoxy-D-glucose)) a cheap, non-toxic, naturally occurring polysaccharide for sample preparation is attracting increasing interest, as recently evidenced by comprehensive reviews.11,12 The use of chitosan as analytical sorbent has been reported in water and food samples, under several configuration: i.e. disk sorptive extraction,13 ultrasound-assisted magnetic solid phase extraction,14 pipette tip-solid phase extraction,15 and dispersive micro-solid phase extraction (D-μ-SPE).16 D-μ-SPE consists in introducing a sorbent directly into a small sample volume. This helps to disperse the sorbent throughout the solution. Because the sorbent is spread out in the sample solution, it significantly boosts the area where the analytes and sorbent meet, differently from other extraction techniques like SPE where the contact between the analyte and the sorbent takes place only during the transit of the sample in the column. The higher surface area raises the chances of interaction between the analyte and sorbent, making the extraction process faster and more effective.17,18 D-μ-SPE, particularly when coupled with innovative nanomaterials, is lately gaining attention due to its simplicity, speed, efficiency, and eco-friendly attributes.19,20 D-μ-SPE procedures has been recently reported for the determination of triazole fungicides in wastewater and fruit juices using respectively carbon nanotubes/metal–organic frameworks,21 and molecularly imprinted polymers (MIPs).22–24 The use of a hyperbranched polyester composite as sorbent for D-μ-SPE of benzoylurea insecticides in water samples has been also reported25 as well as montmorillonite for preconcentration of neonicotinoid insecticides.26
In the present work a sensitive, efficient and high-throughput D-μ-SPE sample preparation method was developed and proposed for analysis of viticulture-related fungicide residues by using UHPLC-Q-Orbitrap HRMS, from surface water and wine samples. This approach relies on the use of a newly developed nanostructured polymer derived from chitosan. The novel material, named Nano-Cs-NAT was specifically designed for D-μ-SPE to enable the extraction of multiclass fungicides relevant to viticulture, and applied for the first time in complex samples treatment prior LC-Orbitrap-HRMS analysis. Medium molecular weight chitosan was grafted with N-isopropylacrylamide, acrylic acid, and secondly crossed-linked with triethylene glycol dimethacrylate, to create a nanostructured sorbent material. The innovative sorbent underwent rigorous characterization through Fourier transform infrared spectroscopy (FT-IR), scanning electron microscopy (SEM), and Brunauer–Emmett–Teller (BET) analysis. Critical extraction parameters such as adsorption/desorption time, sample pH, sorbent amount, desorption solvent, desorption volume, and shaking speed were meticulously screened and optimized using designed experiments (Plackett–Burman, Box–Behnken), culminating in an efficient extraction procedure meeting the criteria required for a green sample pre-treatment technique.27–29 The newly developed analytical method, Nano-Cs-NAT-D-μ-SPE-LC-Orbitrap-HRMS, underwent validation encompassing recovery, reproducibility, repeatability, linearity, matrix effect, limits of detection and limits of quantification, both for fortified wine and river water samples, resulting in satisfactory methodological performance indices. The successful development and validation of the method marks a significant milestone, as it represents the pioneering application of chitosan for intricate sample pre-treatment prior high resolution mass spectrometric determination of multiclass fungicides. This work is a starting point for the employment of Nano-Cs-NAT before LC-Orbitrap analysis, with the future ambition to extend its use to a larger library of target and non-target contaminants.
Experimental
Chemicals and reagents
The reagents required for Nano-Cs-NAT synthesis: medium molecular weight chitosan, N-isopropylacrylamide, acrylic acid, triethylene glycol dimethacrylate, and ammonium persulphate, were all supplied by Sigma-Aldrich (Steinheim, Germany), as well as the analytical standards of penconazole, imazalil, hexaconazole, flusilazole, triticonazole, diniconazole, epoxiconazole, fenbuconazole, bromuconazole, pyraclostrobin, azoxystrobin and difenoconazole. LC-MS grade acetonitrile, methanol and water were purchased by Merck (Darmstadt, Germany). Acetic acid was acquired from Fisher Scientific (Waltham, MA, USA). Working solutions were daily prepared from methanol stock solutions conserved at −20 °C.
Instruments
For the analysis of fungicides, an Orbitrap Q Exactive™ Focus mass spectrometer (Thermo Fisher Scientific Inc., Waltham, MA, USA) coupled with an Ion Max heated electrospray ionization (HESI-II) source and a Dionex Ultimate 3000 system were employed. The Dionex Ultimate 3000 system comprised a binary pump, a temperature-controlled autosampler, and a column compartment. The mass spectrometer operated in positive ionization mode, and the separation of the target analytes was carried out on a Thermo Hypersil GOLD aQ column (50 × 2.1 mm, 1.9 μm particle size). The chromatographic separation of compounds followed a 15 minute program, as previously described in other articles of the group.30,31 The mobile phases consisted of water with 0.1% formic acid (mobile phase A) and methanol with 0.1% formic acid (mobile phase B). The gradient program was as follows: 0 min – 90% A, 1.5 min – 90% A, 4 min – 40% A, 8 min – 30% A, 11 min – 100% A, 12 min – 90% A, 15 min – 90% A. The flow rate was set at 200 μL min−1, and a 5 μL injection volume was used. Analysis was performed in full-scan (FS) MS mode within a scan range of 100–1000 m/z, with a resolution of 70
000. Instrument control, peak detection, and integration were carried out using Thermo Xcalibur 2.2.0.48 software (Thermo Fisher Scientific Inc., Waltham, MA, USA). To achieve comprehensive spectrometric characterization of the fungicides, a solution of each compound at the concentration of 30 μg L−1, was injected into the LC Orbitrap-HRMS system. To elucidate the molecular characteristics of the compounds, full-scan mass spectrometry was conducted, enabling the acquisition of precursor ion ([M + H]+) data for each individual compound, predicted on exact mass and molecular formula. Subsequently, for the purpose of compound identification within the extracted samples, diagnostic ions and fragmentation patterns derived from the standard solutions to those obtained from the samples were compared. Improving chromatographic separation was balanced with efforts to keep the overall sample run time at 15 min.
Sorbent synthesis
The synthesis of Nano-Cs-NAT was performed by free-radical polymerization after activation of chitosan with ammonium persulphate, and subsequent reaction with acrylic compounds. Similar synthetic approaches have been previously reported in literature.32–34 For sorbent synthesis, 0.2 g of medium molecular weight chitosan were dissolved in 20 mL of 1% (v/v) acetic acid ultrapure aqueous solution. The solution was stirred at 1400 rpm at 30 °C for 6 h. After chitosan solubilization, ammonium persulphate was added, and the temperature was raised to 40 °C for 2 hours in order to generate the initiating sites. Secondly the monomers were added to the solution: (a) 3 mmol N-isopropylacrylamide, (b) 3 mmol of acrylic acid, and the solution was stirred for one hour after raising the temperature to 70 °C. Finally, the cross-linker (12 mmol of triethylene glycol dimethacrylate) was added. The temperature of the mixture was kept at 70 °C to promote radical polymerization. After 12 hours the solid white product was collected and washed by subsequent dispersions and centrifugations in an acetonitrile/water mixture (1
:
3 v/v). The clean material was dried to constant weight at the controlled temperature of 38 °C.
Sorbent characterization
Morphology and chemical structure of the sorbent were characterized by FT-IR, SEM and BET analysis. FT-IR-2000 (PerkinElmer, Dresden, Germany) was used for identification of the functional groups. Pellets containing the polymers were created mixing the materials with potassium bromide and pressing the powder. Absorbance for the samples was analysed at wavelengths between 450 and 4000 cm−1 at a resolution of 4 cm−1. Morphological inspection was performed by scanning electron microscopy SEM JEOL JMS-840 (Jeol, Tokyo, Japan), with an accelerating voltage of 5 kV. Before analysis the material was roofed by a carbon coating to enhance conductivity. BET experiments to examine surface area and pore size distribution were performed using the Automatic Volumetric Sorption Analyzer Autosorb-1MP (Quantachrome, Florida, USA).
Nano-Cs-NAT-D-μ-SPE procedure development
Nano-Cs-NAT was tested as sorbent for D-μ-SPE of fungicides from complex matrices. In order to investigate the ability of the developed material to extract the analytes in real conditions, samples of river water and wine were employed. Non-spiked and spiked samples were used for recovery experiments, with a spiking mixture consisting in twelve fungicides belonging to different chemical families (penconazole, imazalil, hexaconazole, flusilazole, triticonazole, diniconazole, epoxiconazole, fenbuconazole, bromuconazole, pyraclostrobin, azoxystrobin and difenoconazole). The optimal extraction procedure required 10 mL of sample volume, 40 mg of Nano-Cs-NAT, an adsorption duration of 40 minutes, and a desorption phase lasting 5 min with 2 mL of acetonitrile. Several extraction parameters were screened by Plackett–Burman design of experiments (PBD),35 considering the average recovery of the twelve analytes as response. Secondly, the factors resulting influent on the recovery were optimized through surface response methodology employing Box–Behnken design (BBD). All experiments were designed with R(software)36 and conducted in a random order, to minimize the effect of uncontrollable variables. After each extraction experiment the eluted volume was filtered through a 22 μm PTFE filter before LC-Orbitrap HRMS injection, without the need for additional pre-concentration steps.
Plackett–Burman design
Through PBD a total of 12 experiments were generated. Seven independent factors were assessed: (A) adsorption time, (B) sample pH, (C) sorbent amount, (D) desorption time, (E) agitation speed, (F) desorption solvent, (G) desorption solvent volume. In a typical experiment Nano-Cs-NAT was added in falcons containing fortified river water or wine. The spiked samples pH was adjusted using NaOH or HCl, the falcons were oscillated in a shaking table at room temperature, and secondly centrifuged to separate the sorbent material from the aqueous supernatant, which was discarded. For analytes desorption from the material the solvent was added in the falcons which were vortexed and then centrifugated. All the quantitative parameters of the experiments were defined by the experimental design. For each experiment the percentage of recovery for the twelve fungicides in water and wine was calculated, and the average recovery was considered as response.
Box–Behnken design
BBD experiments were performed prioritizing ease and procedural efficiency for factors that did not exhibit statistical significance in the preliminary PBD screening. Meanwhile, the factors adsorption time, desorption time, and sorbent amount were subjected to multivariate optimization using BBD methodology. A total of 16 optimization experiments were generated, considering 3 factors and 4 central points as follows. | N(run) = 2K(K − 1) + C | (1) |
where N(run) is the number of experiments in the BBD, K is the number of factors, an C is the number of central points. A response surface was fitted to the experimental data with R(software) using the rsm package,37 considering first-order interactions, two-way interactions, and pure-quadratic interactions. Fitted response-surface contour plots and perspective plots of the responses were generated. Stationary points of maximum response surface were identified and employed, alongside practical consideration, for the development of the optimal Nano-Cs-NAT-D-μ-SPE procedure.
Methodological performance evaluation
Method performance was evaluated in both river water and wine through recovery, reproducibility, repeatability, linearity, matrix effect, LODs and LOQs for all the analytes. Recovery tests were performed under optimal conditions previously determined through experimental designs. Different spiking levels were considered for water and wine (0.2, 0.6, 2 μg L−1 for water and 5, 10, 20 μg kg−1 for wine). For each target analyte, recovery was calculated as the ratio of the signal after extraction to that of a standard at the same theoretical concentration. The analysis of river water and white wine lacking fortification was employed to perform blank correction in the pesticide concentrations, in case already present in the samples. Repeatability (intra-day) and reproducibility (inter-day) precision assessments were quantified in terms of Relative Standard Deviation (RSD), following the guidelines outlined by the European Commission. Intra-day precision values were determined based on the analysis of samples spiked at 3 levels within a single day. Conversely, inter-day precision values were derived from the analysis of samples spiked at 3 levels conducted over a span of 3 consecutive days. Linearity was calculated by linear regression of the calibration curves generated form fortified river water and wine. LODs and LOQs were assessed based on the standard error of the residuals for the instrumental response and the slope of the calibration curves generated from fortified river water and wine. Matrix effect (ME%) was determined by examining calibration curves at various levels of fungicide concentration in both a solvent (acetonitrile) and a blank matrix extract. To evaluate the impact of the matrix, the slopes of these curves were compared as follows. |  | (2) |
Samples collection
River water was sampled from Nestos River, Xanthi, Greece. Containers of poly-propylene were used for water collection. Commercially available white wine was collected at local supermarket in Thessaloniki, Greece. After acquisition all samples were kept refrigerated at 4 °C.
Evaluation of sustainability
Sustainability of the proposed sample preparation technique was evaluated through sample preparation metric of sustainability29 and analytical greenness metric for sample preparation AGREEprep.28
Results and discussion
Sorbent synthesis
Modification of chitosan is generally required when the aim is to use this polysaccharide for analytical purposes.12 Medium molecular weight chitosan was efficiently modified by radical activation, which permits a rapid polymerization with a wide range of monomers and was previously reported in similar syntheses.34,38 Monomers and cross-linker were chosen on the base of their ability to give a stable polymeric material, and on the base of chemical considerations related to their interaction with the target analytes (specifically hydrogen bonds and π–π interactions). After Nano-Cs-NAT synthesis, a precipitate in form of a white powder was obtained. The material was collected, cleaned, and characterized.
Characterization of the sorbent
Characterization of the sorbent was performed by FT-IR, SEM and BET. Schematic synthetic procedure, suggested structure of the sorbent, and characterization results are reported in Fig. 1.
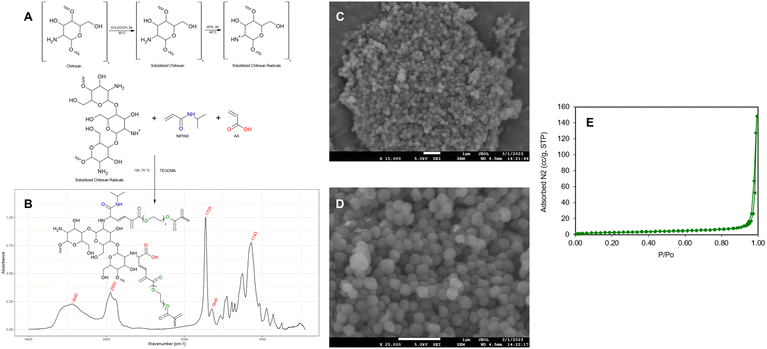 |
| Fig. 1 Schematic synthetic procedure of the sorbent (A), FT-IR spectrum (B), SEM image at ×10 000 magnification (C), SEM image at ×25 000 magnification (D), BET analysis (E). | |
The broad band around 2900–3400 cm−1 in the FT-IR spectrum indicates stretching vibrations of chitosan hydroxyl groups, and it overlaps at 3442 cm−1 with N–H stretching.39 The stretch of the C–H bonds gives a characteristic band at 2950 cm−1, while at 1729 cm−1 it is visible the sharp peak due to the ester groups characteristic of the cross-linker (triethylene glycol dimethacrylate). At 1648 cm−1 it is evident the amide C
O stretching ascribable to N-isopropylacrylamide, and at 1143 cm−1 the signal of C–N stretch, within the range of the non-aromatic amines. FT-IR spectrum indicated an efficient combination between chitosan, acrylic monomers and the cross-linker: characteristic bands of all these molecules were present. Morphological SEM analysis showed Nano-Cs-NAT is characterized by an aggregation of nanoparticles with a well-defined spherical shape and a diameter of approximately 300 nm. BET analysis resulted in a type III isotherm, characteristic of non-porous materials.40 Detailed parameters are reported in Table S1.† Nano-Cs-NAT particles are indeed non-porous, and the low surface area of 10 m2 g−1 can be attributed to the voids between particle aggregates clearly visible in the SEM image. The non-porous nature of nanoparticles containing hydrophobic groups has been reported, as well as their high tendency to aggregate.41 There is limited research on non-porous materials for recovery experiments; however, utilizing non-porous materials as adsorbents has the potential to significantly enhance the adsorption efficiency, due to faster adsorption/desorption rates.42
Interactions between Nano-Cs-NAT and target analytes
The interplay between analytes (12 viticulture-related fungicides) and Nano-Cs-NAT can be attributed to several interaction between the surface of the nanoparticles and the hydrophilic/hydrophobic groups present in the analytes, however π–π interaction is hypothesized to be the major one (Fig. 2). TEGDMA units and the glucosamine backbone of chitosan can interact with the π-electron clouds of the aromatic rings present in the analytes.43 On the other side Nano-Cs-NAT contains abundance of H-bond donor and acceptor groups, ascribable to chitosan, N-isopropylacrylamide, and acrylic acid, which can also interact with the analytes. van der Waals Interactions, including dispersion and dipole–dipole forces, may also play a role. The authors suggest the concurrent action of hydrophobic and hydrophilic interactions results in the selective extraction of the target analytes, which are characterized by both polar and non-polar groups.
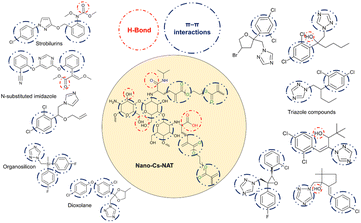 |
| Fig. 2 Interactions between the analytes and Nano-Cs-NAT. Groups responsible for interaction on the material and on the analytes are highlighted. | |
LC Orbitrap HRMS analysis
Full-scan mass spectrometry was employed to gather precursor ion ([M + H]+) data for each compound, based on precise mass and molecular formula are reported in Table 1. For compounds identification in extracted samples, diagnostic ions and fragmentation patterns from the samples were compared with those obtained from standard solutions. Efficient chromatographic separation for all the twelve analytes were obtained. Chromatograms for two analytes and the corresponding fragmentation patterns are presented in Fig. 3, while the chromatograms for all the twelve analytes are reported in Fig. S1.†
Table 1 Features of interest of the analytes
Fungicide |
Molecular formula |
Diagnostic ion |
Retention time |
Penconazole |
C13H15Cl2N3 |
[M + H]+ 284.0716 |
9.75 |
Imazalil |
C14H14Cl2N2O |
[M + H]+ 297.0556 |
7.10 |
Hexaconazole |
C14H17Cl2N3O |
[M + H]+ 314.0821 |
10.04 |
Flusilazole |
C16H15F2N3Si |
[M + H]+ 316.1076 |
9.31 |
Triticonazole |
C17H20ClN3O |
[M + H]+ 318.1368 |
8.67 |
Diniconazole |
C15H17Cl2N3O |
[M + H]+ 326.0821 |
10.49 |
Epoxiconazole |
C17H13ClFN3O |
[M + H]+ 330.0804 |
8.93 |
Fenbuconazole |
C19H17ClN4 |
[M + H]+ 337.1215 |
9.20 |
Bromuconazole |
C13H12BrCl2N3O |
[M + H]+ 375.9614 |
9.44 |
Pyraclostrobin |
C19H18ClN3O4 |
[M + H]+ 388.1059 |
10.35 |
Azoxystrobin |
C22H17N3O5 |
[M + H]+ 404.1241 |
7.64 |
Difenoconazole |
C19H17Cl2N3O3 |
[M + H]+ 406.0720 |
10.82 |
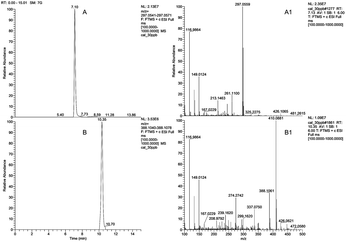 |
| Fig. 3 Q Orbitrap MS chromatograms and spectra: (A and B) extracted ion chromatogram of imazalil [M + H]+m/z 297.0556 and pyraclostrobin [M + H]+m/z 388.1059 with a mass tolerance of 5 ppm; (A1 and B1) mass spectra from chromatograms (A and B). | |
Nano-Cs-NAT for D-μ-SPE
Nano-Cs-NAT was employed as solid sorbent for D-μ-SPE to recover twelve fungicides from fortified river water and wine samples prior LC-Orbitrap HRMS analysis. To devise an optimal D-μ-SPE procedure that balances efficiency, simplicity, environmental sustainability, and cost-effectiveness, a multivariate approach was employed. Initially, a Plackett–Burman design of experiments was used to assess various factors, including adsorption/desorption time, sample pH, sorbent amount, desorption solvent, desorption volume, and shaking speed. PBD is a type of two-level fractional factorial design, known for efficiently screening a large number of variables in a small number of runs.35,44 Factor levels and experiments generated for the PBD are reported in Tables S2 and S3.† In both water and wine, adsorption time, desorption time, sorbent amount and desorption solvent resulted significant on fungicides average recovery. Statistical significance of the seven parameters tested are reported in Tables S4 and S5† respectively for water and wine. Main effects plots are reported in Fig. S2:† interestingly pH did not result statistically significant at the levels tested, this could be explained by the fact that the major interactions between the targeted analytes and the material are π–π (as reported in Fig. 2) which are generally less affected by changes in pH compared to ionic or charged interactions. Acetonitrile resulted in higher recoveries (stronger elution properties of acetonitrile when compared to methanol are well known from literature22). Higher adsorption time, and higher Nano-Cs-NAT amount resulted as well in better recoveries. An opposite behaviour of the sorbent was observed in relation to the desorption time, with higher recoveries obtained at lower desorption times. This phenomenon, despite not common, has been previously reported.45,46 The authors speculate this behaviour may be related to modification occurring in the sorbent when dispersed in an organic solvent for a prolonged time, which may lead to a reuptake of the analytes followed by a hindered desorption of the same. A second explanation may lie in the fact that longer elution could increase the release of interferences adsorbed on the material, causing a decrease in detection sensitivity.
Nano-Cs-NAT-D-μ-SPE procedure optimization
The screening process identified three significant numerical factors: adsorption time, desorption time, and sorbent amount, which were subsequently optimized through Box–Behnken design. BBD is highly effective for response surface methodology, requiring fewer experiments compared to central composite designs.47 Moreover, BBD utilizes practical face points instead of corner points.37 Experiments generated for BBD are reported in Table S6,† contour plots in Fig. S3† and perspective plot in Fig. 4. Best results (>90% of recovery) were obtained at higher adsorption time, lower desorption time, when 40 mg of sorbent were employed, with a high impact of the desorption time.
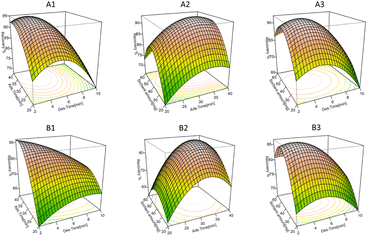 |
| Fig. 4 Perspective plots for optimization of adsorption/desorption time and Nano-Cs-NAT amount in water (A1–A3) and in wine (B1–B3). | |
In water and in wine the same trend was observed. The influence of the studied factors on fungicides recovery from water and wine are reported in Tables S7 and S8.† By backward elimination the best models were selected, the corresponding polynomial equations are reported as follows, respectively for river water and wine.
| RE% = 91.97 − 7.90A + 4.42B + 3.45C − 3.19AB − 10.14A2 − 5.31B2 − 12.86C2 | (3) |
| RE% = 85.22 − 4.28A + 8.80B + 2.45C − 9.44AB − 4.64A2 − 11.81B2 − 9.11C2 | (4) |
With RE% representing the average recovery of the twelve target analytes from the fortified samples after Nano-Cs-NAT-D-μ-SPE, and A, B, C representing respectively the factors desorption time, adsorption time and sorbent amount. Practical considerations concerning both time efficiency and cost-effectiveness were integrated with the findings obtained from Plackett–Burman and Box–Behnken designs to determine the optimal parameters for the extraction procedure based on the newly synthesized chitosan derivative. The selected conditions were the following: (a) 10 mL sample volume with 40 mg of Nano-Cs-NAT, (b) adsorption duration of 40 minutes in shaking table at 100 rpm, (c) desorption phase lasting 5 min in shaking table at 100 rpm, employing 2 mL of acetonitrile. This preparation offers a combination of advantages, including expeditiousness, ease of execution, minimal solvent consumption, and efficiency in terms of sample quantity. Furthermore, it demands only minimal instrumentation, it is operationally straightforward, and possesses the added capability of conducting multiple parallel extractions. These attributes collectively render the Nano-Cs-NAT-D-μ-SPE procedure an attractive and versatile choice for complex sample pre-treatment.
Evaluation of sustainability
According to the sample preparation metric of sustainability a total score of 8.53 was obtained, while based on analytical greenness metric for sample preparation, a total score of 0.55 was obtained, witnessing good sustainability of the method.
Method validation
The applicability of the proposed Nano-Cs-NAT-D-μ-SPE-LC-Orbitrap-HRMS optimized method was verified using river water and wine samples. Two different sets of 3 spiking levels were considered for water and wine. Blank river water was fortified at 0.2, 0.6 and 2 μg L−1, considering the concentrations previously reported in the environment.3 Blank wine samples were spiked at 5, 10 and 20 μg kg−1, considering the Maximum Residue Levels established by the European Union. Recoveries of the analytes from river water were in the range 72–97% (Fig. 5), and for wine in the range 85–104% (Fig. 6), and therefore in compliance with SANTE guidelines.48 In non-spiked samples of both river water and commercially available white wine the targeted analytes resulted not detectable (Table S9†).
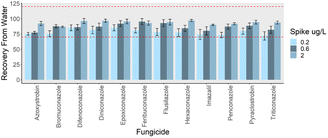 |
| Fig. 5 Recovery values from river water fortified at 3 levels, (n = 3). The range 70–120% provided by SANTE guidelines is reported with dotted red lines. | |
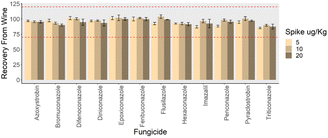 |
| Fig. 6 Recovery values from wine fortified at 3 levels, (n = 3). The range 70–120% provided by SANTE guidelines is reported with dotted red lines. | |
Linearity, LODs, LOQs, average recoveries, repeatability, and reproducibility are reported in Table 2 for river water, and Table 3 for wine. Linearity was assessed in river water (between 0.2 μg L−1 and 2 μg L−1) and in wine (between 5 μg kg−1 and 20 μg kg−1), satisfactory results in terms of determination coefficient (R2) were obtained for all the analytes (R2 > 0.998). Good results were obtained in terms of LODs and LOQs for all the analytes (average LOD of 0.04 μg L−1 for the analytes in river water and average LOD of 0.72 μg kg−1 in wine). Average intra-day relative standard deviations on recovery experiments at the three spiking levels was in the range 3–6% for river water and in the range 2–4% for wine. Average inter-day relative standard deviation in the range 4–7% for river water and 3–7% for wine. Matrix effect was in the range −4% to + 6% for water and −11% to + 8% for wine, and was therefore considered negligible.49,50 Extensive validation results are reported in Table S9.† Validation outcomes underscore the auspicious utility and potential applicability of the developed procedure in environmental and food analysis.
Table 2 Linearity, LODs and LOQs estimations, recovery, intra-day and inter-day variability for the proposed method on river water
Matrix |
Compound |
R
2
|
LOD [μg L−1] |
LOQ [μg L−1] |
Recoverya [%] |
RSD% intra-daya |
RSD% inter-daya |
Average values for the 3 spiking levels are reported.
|
River water |
Penconazole |
0.999 |
0.002 |
0.056 |
85 |
3 |
6 |
Imazalil |
1 |
0.001 |
0.004 |
82 |
5 |
7 |
Hexaconazole |
0.999 |
0.011 |
0.033 |
86 |
4 |
5 |
Flusilazole |
0.998 |
0.022 |
0.067 |
89 |
6 |
7 |
Triticonazole |
1 |
0.012 |
0.036 |
83 |
5 |
7 |
Diniconazole |
1 |
0.011 |
0.035 |
89 |
5 |
7 |
Epoxiconazole |
1 |
0.008 |
0.024 |
91 |
5 |
6 |
Fenbuconazole |
1 |
0.070 |
0.212 |
90 |
4 |
6 |
Bromuconazole |
0.999 |
0.140 |
0.140 |
84 |
3 |
4 |
Pyraclostrobin |
1 |
0.057 |
0.172 |
88 |
4 |
6 |
Azoxystrobin |
0.999 |
0.114 |
0.347 |
83 |
3 |
4 |
Difenoconazole |
1 |
0.016 |
0.049 |
90 |
4 |
5 |
Table 3 Linearity, LODs and LOQs estimations, recovery, intra-day and inter-day variability for the proposed method on wine
Matrix |
Compound |
R
2
|
LOD [μg kg−1] |
LOQ [μg kg−1] |
Recoverya [%] |
RSD% intra-daya |
RSD% inter-daya |
Average values for the 3 spiking levels are reported.
|
Wine |
Penconazole |
0.999 |
1.18 |
3.58 |
94 |
2 |
6 |
Imazalil |
0.998 |
0.46 |
1.38 |
92 |
4 |
7 |
Hexaconazole |
0.998 |
1.51 |
4.58 |
92 |
2 |
4 |
Flusilazole |
0.998 |
1.52 |
4.61 |
99 |
2 |
4 |
Triticonazole |
0.999 |
0.94 |
2.83 |
88 |
2 |
5 |
Diniconazole |
1 |
0.58 |
1.75 |
96 |
2 |
4 |
Epoxiconazole |
1 |
0.29 |
0.88 |
101 |
3 |
4 |
Fenbuconazole |
1 |
0.18 |
0.55 |
101 |
2 |
5 |
Bromuconazole |
1 |
0.19 |
0.56 |
94 |
2 |
3 |
Pyraclostrobin |
0.999 |
1.16 |
3.52 |
98 |
2 |
4 |
Azoxystrobin |
1 |
0.02 |
0.07 |
96 |
2 |
5 |
Difenoconazole |
1 |
0.58 |
1.75 |
99 |
3 |
5 |
Comparison with other methods
In Table 4 a comparison of the developed method with others present in literature is reported.
Table 4 Comparison of Cs-NAT-D-μ-SPE-LC-Orbitrap-HRSM method with other methods for fungicides analysis
Sample preparation |
Matrix |
Analytes |
Analytical instrumentation |
Sample amount |
Extraction time |
Solvent |
Solvent volume |
LODa |
Recovery % |
Linear rangea |
RSD% |
Reference |
μg kg−1 for solid samples μg L−1 for liquid samples.
Deep eutectic solvents matrix solid-phase dispersion liquid–liquid back-extraction.
Thin film microextraction.
Microextraction by packed sorbent.
Effervescent assisted switchable hydrophilicity solvent-based microextraction.
Molecularly imprinted polymer dispersive solid phase extraction.
Molecularly imprinted polymer solid phase extraction.
Cyclodextrin-based dispersive liquid–liquid microextraction.
|
DES-MSPD-LLEb |
Tomatoes |
Triazole fungicides |
HPLC-MS |
0.2 g |
— |
ChCl-EG, EtOAc |
6.5 mL |
≤3.3 |
61–116 |
10–600 |
≤3 |
51
|
TFMEc |
Water |
Chlorpyrifos |
GC-MS |
10 mL |
75 min |
EtOAc |
1 mL |
≤1 |
65–91 |
50–200 |
≤13 |
52
|
MEPSd |
Wheat flour |
Pesticides including fungicides |
UHPLC-MS/MS |
200 mg |
— |
ACN, MeOH |
0.98 mL |
≤5 |
≥87 |
0.01–250 |
≤15 |
53
|
EA-SHS-MEe |
Water, honey |
Triazole fungicides |
HPLC |
10 mL |
15 min |
ACN |
0.1 mL |
≤1 |
60–100 |
3–100 |
<10 |
54
|
MIP-d-SPEf |
Water, fruit juice |
Triazole fungicides |
LC-MS |
6 mL |
10 min |
ACN |
2 mL |
≤9 |
90–10 |
30–150 |
<5 |
22
|
MIP-SPEg |
Tobacco leaves |
Triazole fungicides |
UHPLC-MS/MS |
2 mL |
— |
MeOH, ACN |
18 mL |
≤1.6 |
71–110 |
10–2000 |
≤13 |
55
|
CD-DLLMEh |
Water, juice, vinegar |
Triazole and strobilurin fungicides |
HPLC-DAD |
10 mL |
< 0.02 min |
Undecanol |
0.2 mL |
≤0.3 |
83–103 |
1–100 |
≤7 |
56
|
SPE |
Water |
Pesticides including fungicides |
UHPLC-Orbitrap-MS/MS |
5 mL |
20 min |
Ammonium formate, MeOH |
3.75 mL |
≤0.03 |
80–120 |
0.01–2 |
≤8 |
4
|
QuEChERS |
Vegetables |
Triazole fungicides |
UHPLC-Orbitrap-MS/MS |
10 g |
10 min |
ACN |
10 mL |
≤3 |
70–120 |
5–250 |
≤20 |
57
|
Nano-Cs-NAT-D-μ-SPE |
Water |
Multiclass fungicides |
UHPLC-Orbitrap-MS/MS |
10 mL |
45 min |
ACN |
2 mL |
≤0.14 |
72–97% |
0.2–2 |
<6% |
Present work |
Nano-Cs-NAT-D-μ-SPE |
Wine |
Multiclass fungicides |
UHPLC-Orbitrap-MS/MS |
10 mL |
45 min |
ACN |
2 mL |
≤1.52 |
85–104% |
5–20 |
<4% |
Present work |
For certain parameters the proposed method resulted similar to other methods: sample amount used, pre-treatment time, and recovery. For other parameters the proposed method resulted advantageous: total amount of organic solvent, limits of detection and reproducibility. The amount of total organic solvent employed in the proposed sample preparation (2 mL) was lower compared to the average volume used in the other methods considered (4 mL). The limits of detection of Nano-Cs-NAT-D-μ-SPE-LC-Orbitrap-HRSM method in water and in wine were comparable or, in most cases, lower than the ones of other methods. Relative standard deviations on recovery experiments for the proposed method resulted generally lower than the ones of other methods: this is a major result, especially considering that Nano-Cs-NAT has been in-house synthesized, and so without the standardization protocols that an industrial sorbent undergoes. The developed Nano-Cs-NAT-D-μ-SPE-LC-Orbitrap-HRSM method results therefore a competitive option for multiclass fungicides analysis in complex matrices.
Conclusions
Fungicides represent a threat for the environmental and human health, and the need to develop new efficient methods for fungicide residues analysis in water bodies and dietary samples is current. In the present study a new, inexpensive nanostructured material based on chitosan was developed and employed as efficient analytical sorbent for river water and wine samples pre-treatment before LC-Orbitrap analysis. Utilizing the chitosan derivative (named Nano-Cs-NAT), a multivariate approach enabled the development of a green, fast, and reliable D-μ-SPE procedure for the recovery of twelve fungicides largely used in viticulture. The optimized method has proved to be fast, easy, and cheap, requiring minimal amounts of organic solvent and sorbent material, with satisfactory methodological indices in terms of fungicides recovery, linearity, repeatability, reproducibility, matrix effect, LODs and LOQs, showing the strong potential of Nano-Cs-NAT in sample preparation.
Author contributions
Conceptualization: L. M., D. B., and D. L.; data curation: L. M.; formal analysis: L. M.; funding acquisition: D. L.; investigation: A. R. and L. M.; methodology: L. M., D. B., and D. L.; project administration: D. L.; resources: D. L.; software: L. M.; supervision: D. L. and D. B.; validation: A. R., and L. M.; visualization: L. M.; writing—original draft: L. M.; writing—review & editing: L. M., D. B. and D. L.
Conflicts of interest
There are no conflicts to declare.
Acknowledgements
This project has received funding from the European Union's Horizon 2020 research and innovation programme under the Marie Skłodowska-Curie grant agreement No. 956265 – FoodTraNet. The publication of the article in OA mode was financially supported by HEAL-Link.
References
- W. Qiu, A. Feechan and I. Dry, Current understanding of grapevine defense mechanisms against the biotrophic fungus (Erysiphe necator), the causal agent of powdery mildew disease, Hortic. Res., 2015, 2, 15020, DOI:10.1038/hortres.2015.20.
- A. Nesler, M. Perazzolli, G. Puopolo, O. Giovannini, Y. Elad and I. Pertot, A complex protein derivative acts as biogenic elicitor of grapevine resistance against powdery mildew under field conditions, Front. Plant Sci., 2015, 6, 715, DOI:10.3389/fpls.2015.00715.
- K. Anagnostopoulou, C. Nannou, V. G. Aschonitis and D. A. Lambropoulou, Screening of pesticides and emerging contaminants in eighteen Greek lakes by using target and non-target HRMS approaches: Occurrence and ecological risk assessment, Sci. Total Environ., 2022, 849, 157887, DOI:10.1016/j.scitotenv.2022.157887.
- J. Toth, M. Pineda and V. Yargeau, Fast and simplified quantitative multiresidue analytical method for pesticides in surface waters by UHPLC-MS/MS with online sample preparation, Chemosphere, 2023, 318, 137962, DOI:10.1016/j.chemosphere.2023.137962.
- D. L. Christodoulou, P. Kanari, P. Hadjiloizou and P. Constantinou, Pesticide residues analysis in wine by liquid chromatography–tandem mass spectrometry and using ethyl acetate extraction method: validation and pilot survey in real samples, J. Wine Res., 2015, 26(2), 81–98, DOI:10.1080/09571264.2015.1022255.
- N. Casado, J. Gañán, S. Morante-Zarcero and I. Sierra, New advanced materials and sorbent-based microextraction techniques as strategies in sample preparation to improve the determination of natural toxins in food samples, Molecules, 2020, 25(3), 702, DOI:10.3390/molecules25030702.
- M. Li,
et al., A sensitive and simple competitive nanozyme-linked apta-sorbent assay for the dual-mode detection of ochratoxin A, Analyst, 2022, 147, 2215–2222, 10.1039/d1an02335g.
- P. Baile, E. Fernández, L. Vidal and A. Canals, Zeolites and zeolite-based materials in extraction and microextraction techniques, Analyst, 2019, 144(2), 366–387, 10.1039/c8an01194j.
- Y. Ding, H. Shang, X. Wang and L. Chen, A SERS-based competitive immunoassay for highly sensitive and specific detection of ochratoxin A, Analyst, 2020, 145(18), 6079–6084, 10.1039/d0an01220c.
- A. Fernandez-Alba, Application of Orbitrap Mass Spectrometry in Food Analysis, J. AOAC Int., 2018, 101(2), 335, DOI:10.5740/jaoacint.17-0409.
- A. R. Bagheri, N. Aramesh and H. K. Lee, Chitosan- and/or cellulose-based materials in analytical extraction processes: A review, TrAC, Trends Anal. Chem., 2022, 157, 116770, DOI:10.1016/j.trac.2022.116770.
- M. Sajid, Chitosan-based adsorbents for analytical sample preparation and removal of pollutants from aqueous media: Progress, challenges and outlook, Trends Environ. Anal. Chem., 2022, 36, e00185, DOI:10.1016/j.teac.2022.e00185.
- D. Arismendi, I. Vera, I. Ahumada and P. Richter, A thin biofilm of chitosan as a sorptive phase in the rotating disk sorptive extraction of triclosan and methyl triclosan from water samples, Anal. Chim. Acta, 2023, 1252, 341053, DOI:10.1016/j.aca.2023.341053.
- M. C. Lefatle, L. M. Madikizela, V. E. Pakade and P. N. Nomngongo, Magnetic chitosan-zeolite composite as an adsorbent in ultrasound-assisted magnetic solid phase extraction of tetracyclines in water samples, J. Anal. Sci. Technol., 2023, 14(1) DOI:10.1186/s40543-023-00394-1.
- Q. Wang, T. Wang, Y. Zhang, J. Ma and Y. Tuo, Preparation and evaluation of a chitosan modified biochar as an efficient adsorbent for pipette tip-solid phase extraction of triazine herbicides from rice, Food Chem., 2022, 396, 133716, DOI:10.1016/j.foodchem.2022.133716.
- X. Hai,
et al., Development of magnetic dispersive micro-solid phase extraction of four phenolic compounds from food samples based on magnetic chitosan nanoparticles and a deep eutectic supramolecular solvent, Food Chem., 2023, 410, 135338, DOI:10.1016/j.foodchem.2022.135338.
- M. Ghorbani, M. Aghamohammadhassan, H. Ghorbani and A. Zabihi, Trends in sorbent development for dispersive micro-solid phase extraction, Microchem. J., 2020, 158, 105250, DOI:10.1016/j.microc.2020.105250.
- S. K. Selahle, A. Mpupa, A. Nqombolo and P. N. Nomngongo, A nanostructured o-hydroxyazobenzene porous organic polymer as an effective sorbent for the extraction and preconcentration of selected hormones and insecticides in river water, Microchem. J., 2022, 181, 107791, DOI:10.1016/j.microc.2022.107791.
- W. X. Liu, S. Song, M. L. Ye, Y. Zhu, Y. G. Zhao and Y. Lu, Nanomaterials with Excellent Adsorption Characteristics for Sample Pretreatment: A Review, Nanomaterials, 2022, 12(11), 1845, DOI:10.3390/nano12111845.
- Z. Moradi, E. Alipanahpour Dil and A. Asfaram, Dispersive micro-solid phase extraction based on Fe3O4@SiO2@Ti-MOF as a magnetic nanocomposite sorbent for the trace analysis of caffeic acid in the medical extracts of plants and water samples prior to HPLC-UV analysis, Analyst, 2019, 144(14), 4351–4361, 10.1039/c9an00120d.
- M. Sheykhan, Z. Aladaghlo, S. Javanbakht, A. Fakhari and A. Shaabani, Carbon nanotubes/metal-organic framework based magnetic dispersive micro-solid phase extraction for the determination of triazole fungicides in wastewater and soil samples, Microchem. J., 2023, 193, 109149, DOI:10.1016/j.microc.2023.109149.
- L. Martello, N. M. Ainali, D. N. Bikiaris and D. A. Lambropoulou, Multivariate design and application of novel molecularly imprinted polymers selective for triazole fungicides in juice and water samples, Microchem. J., 2023, 192, 108968, DOI:10.1016/j.microc.2023.108968.
- D. Zelikovich, L. Dery, H. Sagi-Cohen and D. Mandler, Imprinting of nanoparticles in thin films: Quo Vadis?, Chem. Sci., 2023, 14, 9630–9650, 10.1039/d3sc02178e.
- L. A. de Souza Freitas, A. C. Vieira, J. A. F. R. Mendonça and E. C. Figueiredo, Molecularly imprinted fibers with renewable surface for solid-phase microextraction of triazoles from grape juice samples followed by gas chromatography mass spectrometry analysis, Analyst, 2014, 139(3), 626–632, 10.1039/c3an01756g.
- C. Liu,
et al., Dispersive micro-solid-phase extraction of benzoylurea insecticides in water samples with hyperbranched polyester composite as sorbent, New J. Chem., 2018, 42(16), 13978–13984, 10.1039/c8nj02167h.
- K. Moyakao, Y. Santaladchaiyakit, S. Srijaranai and J. Vichapong, Preconcentration of trace neonicotinoid insecticide residues using vortex-assisted dispersive micro solid-phase extraction with montmorillonite as an efficient sorbent, Molecules, 2018, 23(4), 883, DOI:10.3390/molecules23040883.
- H. Musarurwa, L. Chimuka and N. T. Tavengwa, Green pre-concentration techniques during pesticide analysis in food samples, J. Environ. Sci. Health, Part B, 2019, 54(9), 770–780, DOI:10.1080/03601234.2019.1633213.
- W. Wojnowski, M. Tobiszewski, F. Pena-Pereira and E. Psillakis, AGREEprep – Analytical greenness metric for sample preparation, TrAC, Trends Anal. Chem., 2022, 149, 116553, DOI:10.1016/j.trac.2022.116553.
- R. González-Martín, A. Gutiérrez-Serpa, V. Pino and M. Sajid, A tool to assess analytical sample preparation procedures: Sample preparation metric of sustainability, J. Chromatogr. A, 2023, 1707, 464291, DOI:10.1016/j.chroma.2023.464291.
- A. Ofrydopoulou, C. Nannou, E. Evgenidou and D. Lambropoulou, Sample preparation optimization by central composite design for multi class determination of 172 emerging contaminants in wastewaters and tap water using liquid chromatography high-resolution mass spectrometry, J. Chromatogr. A, 2021, 1652, 462369, DOI:10.1016/j.chroma.2021.462369.
- L. A. Koronaiou, C. Nannou, N. Xanthopoulou, G. Seretoudi, D. Bikiaris and D. A. Lambropoulou, High-resolution mass spectrometry-based strategies for the target analysis and suspect screening of per- and polyfluoroalkyl substances in aqueous matrices, Microchem. J., 2022, 179, 107457, DOI:10.1016/j.microc.2022.107457.
- R. Dan,
et al., The synthesis of molecular imprinted chitosan-gels copolymerized with multiform functional monomers at three different temperatures and the recognition for the template ovalbumin, Analyst, 2013, 138(12), 3433–3443, 10.1039/c3an36930g.
- X. F. Zheng,
et al., Preparation and characterization of temperature-memory nanoparticles of MIP-CS-: G -PMMA, RSC Adv., 2016, 6(112), 110722–110732, 10.1039/c6ra22730a.
- M. Filippousi,
et al., Modified chitosan coated mesoporous strontium hydroxyapatite
nanorods as drug carriers, J. Mater. Chem. B, 2015, 3(29), 5991–6000, 10.1039/c5tb00827a.
- R. L. Plackett and J. P. Burman, The Design of Optimum Multifactorial Experiments, Biometrika, 1946, 33(4), 305–325, DOI:10.1093/biomet/33.4.305.
-
R Core Team, R: A Language and Environment for Statistical Computing, Vienna, Austria, 2020, available: https://www.R-project.org/, accessed: Feb. 17, 2022 Search PubMed.
- R. V. Lenth, Response-surface methods in R, using RSM, J. Stat. Software, 2009, 32(7), 1–17, DOI:10.18637/jss.v032.i07.
- M. Lazaridou,
et al., Super absorbent chitosan-based hydrogel sponges as carriers for caspofungin antifungal drug, Int. J. Pharm., 2021, 606, 120925, DOI:10.1016/j.ijpharm.2021.120925.
- C. Branca,
et al., Role of the OH and NH vibrational groups in polysaccharide-nanocomposite interactions: A FTIR-ATR study on chitosan and chitosan/clay films, Polymer, 2016, 99, 614–622, DOI:10.1016/j.polymer.2016.07.086.
- M. Thommes,
et al., Physisorption of gases, with special reference to the evaluation of surface area and pore size distribution (IUPAC Technical Report), Pure Appl. Chem., 2015, 87(9–10), 1051–1069, DOI:10.1515/pac-2014-1117.
-
M. de la Guardia and F. A. Esteve-Turrillas, Handbook of Smart Materials in Analytical Chemistry, 2019 Search PubMed.
- Y. Sun,
et al., Economical amidoxime-functionalized non-porous β-cyclodextrin polymer for selective detection and extraction of uranium, Chem. Eng. J., 2023, 459, 141687, DOI:10.1016/j.cej.2023.141687.
- A. R. Bagheri, N. Aramesh and H. K. Lee, Chitosan- and/or cellulose-based materials in analytical extraction processes: A review, TrAC, Trends Anal. Chem., 2022, 157, 116770, DOI:10.1016/j.trac.2022.116770.
- E. A. Dil, A. H. Doustimotlagh, H. Javadian, A. Asfaram and M. Ghaedi, Nano-sized Fe3O4@SiO2-molecular imprinted polymer as a sorbent for dispersive solid-phase microextraction of melatonin in the methanolic extract of Portulaca oleracea, biological, and water samples, Talanta, 2021, 221, 121620, DOI:10.1016/j.talanta.2020.121620.
- G. J. Maranata, N. O. Surya and A. N. Hasanah, Optimising factors affecting solid phase extraction performances of molecular imprinted polymer as recent sample preparation technique, Heliyon, 2021, 7(1), e05934, DOI:10.1016/j.heliyon.2021.e05934.
- F. W. M. Hassan,
et al., A rapid and efficient dispersive trehalose biosurfactant enhanced magnetic solid phase extraction for the sensitive determination of organophosphorus pesticides in cabbage (Brassica olearaceae var. capitate) samples by GC-FID, J. Food Compos. Anal., 2021, 102, 104057, DOI:10.1016/j.jfca.2021.104057.
- G. E. P. Box and D. W. Behnken, Some New Three Level Designs for the Study of Quantitative Variables, Technometrics, 1960, 2(4), 455–475, DOI:10.1080/00401706.1960.10489912.
-
T. Pihlström, A. R. Fernández-Alba, C. Ferrer Amate, M. E. Poulsen, B. Hardebusch and M. Anastassiades, Guidance SANTE 11312/2021 – analytical quality control and method validation procedures for pesticide residues analysis in food and feed, 2021 Search PubMed.
- H. Lee, Y. Cho, G. Jung, H. Kim and W. Jeong, Comparison of recovery efficiency and matrix effect reduction in pesticide residue analysis: QuEChERS with d-SPE, SPE, and FaPEx in apples and Korean cabbage, Anal. Methods, 2023, 15(30), 3709–3716, 10.1039/d3ay00612c.
- C. Igualada, J. Giraldo, G. Font and V. Yusà, Validation of a multi-residue UHPLC-HRMS method for antibiotics screening in milk, fresh cheese, and whey, J. Food Compos. Anal., 2022, 106, 104265, DOI:10.1016/j.jfca.2021.104265.
- V. Gallo, S. Della Posta, A. Gentili, M. Gherardi, L. De Gara and C. Fanali, Back-extraction applied to green matrix solid-phase dispersion for fungicides determination in tomatoes, Sep. Sci. plus, 2023, 6(5), 2200140, DOI:10.1002/sscp.202200140.
- I. Quintanilla, C. Perelló, F. Merlo, A. Profumo, C. Fontàs and E. Anticó, Multiwalled Carbon Nanotubes Embedded in a Polymeric Matrix as a New Material for Thin Film Microextraction (TFME) in Organic Pollutant Monitoring, Polymers, 2023, 15(2), 314, DOI:10.3390/polym15020314.
- F. Di Ottavio,
et al., Determination of Pesticides in Wheat Flour
Using Microextraction on Packed Sorbent Coupled to Ultra-High Performance Liquid Chromatography and Tandem Mass Spectrometry, Food Anal. Methods, 2017, 10(6), 1699–1708, DOI:10.1007/s12161-016-0720-2.
- J. Palasak,
et al., Preconcentration of triazole fungicides using effervescent assisted switchable hydrophilicity solvent-based microextraction prior to high-performance liquid chromatographic analysis, Microchem. J., 2022, 182, 107882, DOI:10.1016/j.microc.2022.107882.
- L. P. Jing,
et al., Preparation and application of tebuconazole molecularly imprinted polymer for detection of pesticide residues in tobacco leaves, J. Polym. Res., 2022, 29(5) DOI:10.1007/s10965-022-03036-z.
- X. Jing,
et al., Cyclodextrin-based dispersive liquid–liquid microextraction for the determination of fungicides in water, juice, and vinegar samples via HPLC, Food Chem., 2022, 367, 130664, DOI:10.1016/j.foodchem.2021.130664.
- M. E. Hergueta-Castillo, E. López-Rodríguez, R. López-Ruiz, R. Romero-González and A. Garrido Frenich, Targeted and untargeted analysis of triazole fungicides and their metabolites in fruits and vegetables by UHPLC-orbitrap-MS2, Food Chem., 2022, 368, 130860, DOI:10.1016/j.foodchem.2021.130860.
|
This journal is © The Royal Society of Chemistry 2024 |
Click here to see how this site uses Cookies. View our privacy policy here.