DOI:
10.1039/D3RA08680A
(Paper)
RSC Adv., 2024,
14, 5012-5021
Environmentally sustainable synthesis of whey-based carbon dots for ferric ion detection in human serum and water samples: evaluating the greenness of the method
Received
19th December 2023
, Accepted 29th January 2024
First published on 8th February 2024
Abstract
Carbon dots (CDs) are valued for their biocompatibility, easy fabrication, and distinct optical characteristics. The current study examines using whey to fabricate CDs using the hydrothermal method. When stimulated at 350 nm, the synthetic CDs emitted blue light at 423 nm and revealed a selective response to ferric ion (Fe3+) in actual samples with great sensitivity, making them a suitable probe for assessing Fe3+ ions. The produced carbon dots demonstrated great photostability, high sensitivity, and outstanding biocompatibility. The findings showed that Fe3+ ions could be quickly, sensitively, and extremely selectively detected in an aqueous solution of carbon dots, with a revealing limit of 0.409 μM in the linear range of 0–180 μM. Interestingly, this recognition boundary is far inferior to the WHO-recommended threshold of 0.77 μM. Two metric tools which were AGREE and the ComplexGAPI were also used to evaluate the method's greenness. The evaluation confirmed its superior environmental friendliness.
1 Introduction
CDs, or luminescent nanoparticles, have garnered widespread attention in recent years. They demonstrate fluorescence when exposed to ultraviolet or visible light.1–4 Moreover, they possess little toxicity,5 outstanding photostability,6 and high water solubility.7 There are two main synthetic approaches for CDs: the top-down and bottom-up methods, which are categorized based on the relationship between sources and products.8 The top-down method involves the utilization of carbon-rich materials or molecules as carbon sources, cutting larger carbon structures like graphite, carbon nanotubes, carbon soot, activated carbon, and graphite oxide into smaller CDs through techniques such as arc discharge,9 laser ablation10 and electrochemical methods.11 On the other hand, bottom-up methods use organic molecules, such as glucose12 and fructose13 to react and form larger CDs by applying external energy like ultrasonication,14 microwave pyrolysis,15 heating,16 hydrothermal,17 and microwave irradiation.18 Due to their unique properties, CDs have found applications in various fields, replacing toxic semiconductor quantum dots19 made of heavy metals like cadmium20 and lead.21 CDs are used in sensing,22 electronics,23 photocatalysis,24 and biomedical applications.25,26 They can be synthesized from diverse precursors, including wastes, edibles, and chemical substances,27 such as watermelon peel,28 mango,29 garlic,30 and ethanol.31 CDs have been applied in imaging mycobacterium and fungal cells, using carbon dots derived from apple juice,32 and in drug delivery and gene transfer.33 Additionally, nitrogen-doped carbon dots demonstrated by Liang served as an “on–off–on” fluorescent sensor for Fe3+ and glutathione detection.34
In this study, we developed a method for synthesizing CDs from yogurt, a widely popular fermented food enjoyed globally. Renowned for its delightful taste, yogurt frequently features on lists of healthy foods due to its rich content of vitamins, minerals, and proteins, a known source of nitrogen atoms that enhance the quantum efficiency of CDs.35 Moreover, yogurt is affordable and widely accessible in most supermarkets and convenience stores. This marks the pioneering synthesis of CDs from fermented milk water using the hydrothermal method. The characterization of the CDs was conducted with great care using UV-vis, fluorescence, FTIR, XRD spectroscopy, DLS, and TEM.
These CDs found application in various sensing scenarios, particularly in the detection of the metal ion Fe3+ in real samples. Our developed fluorescence sensor demonstrated excellent accuracy and stability in determining Fe3+ levels in drinking water and blood samples. Furthermore, CDs exhibited a selective response to Fe3+ even in complex biological environments.
Metal ions play a crucial role in regulating the health of the human body, influencing various biological processes, and serving as essential elements for enzymatic reactions.36,37 Among these, iron stands out as a vital metal ion, contributing to normal biological functions alongside other essential ions like sodium, calcium, zinc, magnesium, and cobalt. Notably, iron functions as a central component in proteins such as hemoglobin, the blood protein.38 Due to its involvement in electron transfer and essential biological processes like cellular respiration, energy production, and oxygen transport,39–41 iron plays a pivotal role in enzymatic reactions. However, an imbalance either excess or deficiency, can lead to molecular diseases and blood disorders, including hemochromatosis, anemia, decreased immunity, and low blood pressure.42–45 Maintaining the proper concentration of iron is crucial for sustaining the body's functions.
Water, a fundamental resource for human and environmental well-being, faces significant threats from pollution. Major contributors to water pollution include the discharge of domestic sewage, industrial wastes (from sectors like textile, leather, and dyeing), radioactive wastes, as well as fertilizers and pesticides from agricultural fields. The accumulation of industrial wastes and heavy metals in water bodies poses serious threats to both human and animal life.46 Therefore, there's a need for simple, effective, and eco-friendly sensor designs for metal ion detection.
Detecting metal ions at low concentrations poses a considerable challenge. Traditional methods like Atomic Absorption Spectroscopy (AAS),47,48 Inductively Coupled Plasma-Mass Spectrometry (ICP-MS),49 and electrochemical assays like voltammetry,50 are not only time-consuming but also demand expensive equipment and meticulous sample preparation. Consequently, straightforward fluorescence probes have surfaced as encouraging sensors. Their attributes, such as high quantum yield, ease of modification, excellent biocompatibility, heightened sensitivity, and the ability to provide real-time sensing.51,52 These advantages make them valuable tools in addressing the challenges associated with metal ion detection.53–55
In this study, we used whey extracted from yogurt as the main material for synthesizing carbon quantum dots (CDs) with a distinct fluorescent emission peak at 423 nm. The fluorescence at 423 nm, displayed by the CDs, can be quenched by Fe3+ ions. Leveraging the strong binding affinity between CDs and Fe3+ ions, we can explore the application of these CDs for detecting Fe3+ ions in both serum and tap water.
2 Experimental details
2.1 Materials
The yogurt used in this study was purchased from a local supermarket in Chamchamal, Iraq. All the chemicals available in the laboratory were utilized. The metal ions had the following purities: Fe(NO3)3·9H2O, ≥99%, Al(NO3)3·9H2O ≥ 99%, Co(NO3)2·6H2O ≥ 98%, Cu(NO3)2·3H2O ≥ 99%, Hg(NO3)2·H2O ≥ 98%, Mn(NO3)2·4H2O ≥ 97%, Mg(NO3)2·6H2O ≥ 98%, Ni(NO3)2·6H2O ≥ 98.5%, Zn(NO3)2·6H2O ≥ 98%, Pb(NO3)2 ≥ 99%, Cd(NO3)2·4H2O 98%. The molecules had the following purities: citric acid (CA, ≥99.5%), arginine (Arg, ≥98%), alanine (Ala, ≥98.5%), L-aspartic acid (L-AA, ≥98%), glycine (Gly, ≥98.5%), ascorbic acid (AA, 99%), histidine (His, ≥99%), phenylalanine (Phy, ≥98%), and cysteine (Cys, ≥97%). Deionized (DI) water was employed in all experiments. Deionized (DI) water with a resistivity of 10 MΩ cm and a conductivity of 0.1 μS was used in all experiments.
2.2 Apparatus
A Cary 60 spectrophotometer from Agilent Technologies, USA, was used to obtain the UV-vis absorbance spectrum (200–600 nm), while a Cary Eclipse Fluorescence spectrophotometer, also from Agilent Technologies, USA, was employed for spectrofluorometric scanning. The FEI Tecnai G2 F30 was used for High-Resolution Transmission Electron Microscopy (HRTEM), and a Nicolet FTIR spectrometer from Thermo Scientific, USA, was utilized. X-ray diffraction (XRD) analysis was conducted using an Empyrean XRD with a Cu Kα radiation source.
2.3 Synthesis of carbon dots
Yogurt was obtained after purchasing at a local supermarket in Chamchamal, Iraq. The whey was then collected from the yogurt and utilized for the fabrication of C-dots through a one-step hydrothermal process. The solution was centrifuged at 4500 rpm for 15 minutes to remove impurities, and the resulting supernatant was poured into a Teflon-lined stainless-steel chamber. It was then heated at 180 °C for 8 hours in an oven. The resultant solution underwent another round of centrifugation (12
000 rpm for 10 minutes) and was filtered through a sterile PTFE syringe filter (0.22 μm). To eliminate any residual unreacted species molecules, an extraction process was conducted twice, using chloroform as a solvent. The product was collected and refrigerated for further experiments. The process of preparing the luminescent CDs from whey is outlined below and illustrated in Scheme 1.
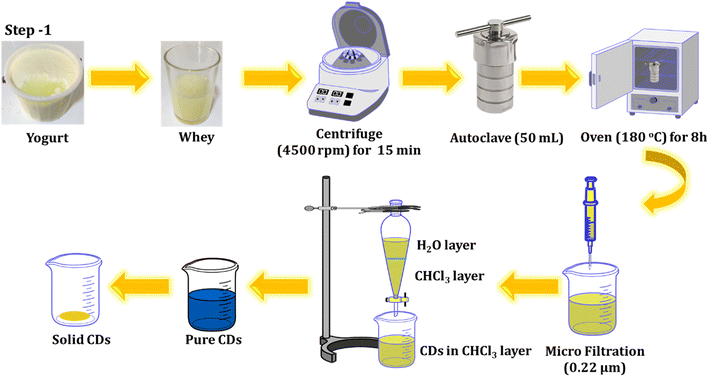 |
| Scheme 1 Illustration of the process for synthesizing the carbon material. | |
2.4 Analytical application
For the detection of Fe3+ ions, 0.5 mL of purified CD solution was mixed with varying amounts of ferric nitrate solution. The mixture was then diluted with deionized water to a total volume of 4 mL. Subsequently, the fluorescence spectra of the solution were recorded and utilized for quantitative analysis.
2.5 Detection of Fe3+ in serum and tap water
The initial step involved treating human serum to liberate Fe3+ from proteins. Essentially, equal amounts of human serum and pure ethanol were combined and heated at 95 °C for 15 minutes. Following cooling to room temperature, the serum solution underwent sonication for 2 minutes. Subsequently, the centrifugation process was employed to eliminate the protein precipitate, and the resulting supernatant was gathered for subsequent use.56 All experiments involving human blood serum samples were performed in compliance with the relevant laws and guidelines of Iraq. The experiments followed the institutional guidelines of Chamchamal Sub DOH Central Public Health Laboratory and were approved by its Ethical Committee (Approval No. CPHC100). Informed consent was obtained from all human subjects who participated in the study. In assessing the practicality of the carbon dots-based sensor for detecting Fe3+ in authentic samples, tap water samples sourced from our laboratory were subjected to analysis using the described method. All the samples of the water were spiked with Fe3+ at varying concentrations without undergoing any pre-treatment.
3 Results and discussion
3.1 Characterization
The structure and dimensions of the synthesized CDs were examined using HRTEM, as depicted in Fig. 1. Fig. 1A and B illustrate that the CDs exhibit uniform spherical shapes and are evenly dispersed. The size distribution, presented in the histogram (Fig. 1C), indicates a range from 1 to 5 nm, with a mean particle diameter of roughly 3.12 nm. Furthermore, the lattice fringes observed in the HRTEM image (Fig. 1C) have a d-spacing of approximately 0.34 nm, consistent with the lattice of graphitic carbon's (100) plane.57,58 Interestingly, the CDs do not exhibit a distinct crystal lattice, implying an amorphous structural nature.
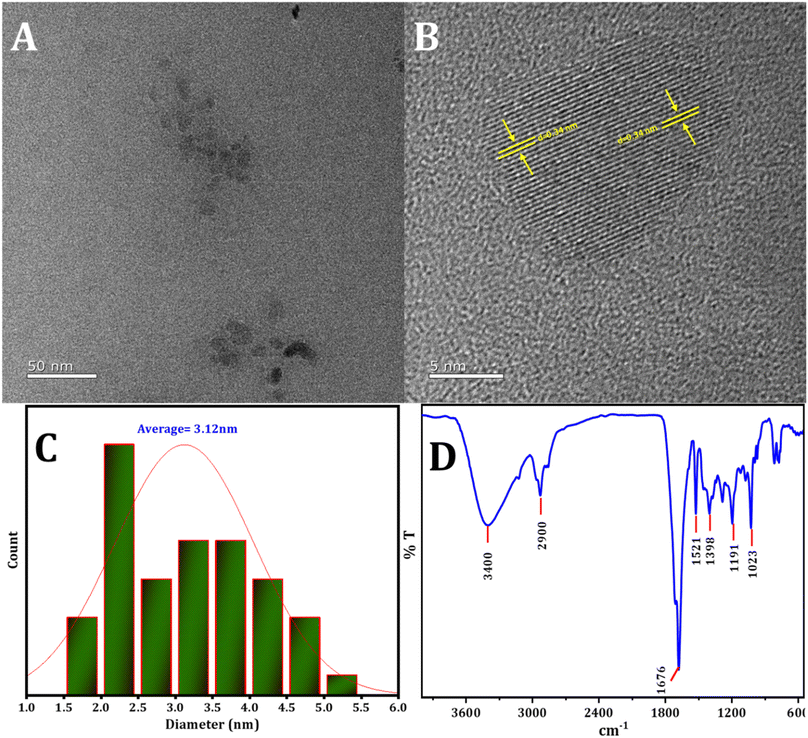 |
| Fig. 1 (A) and (B) The HRTEM image of CDs, (C) the statistical distribution of particle sizes of CDs, (D) the pattern of FTIR spectra of CDs. | |
FTIR examination was conducted to investigate the active groups present on the surface of the CDs. In Fig. 1B, the following observations were made: stretching vibrations of O–H and N–H in the region 2900 cm−1 to 3400 cm−1,59 an absorption peak at 1676 cm−1 corresponding to the stretching vibration of C
O,60 the absorption peak at 1521 cm−1 attributed to the stretching vibration of C
C, and the peak at 1398 cm−1 identified as C–N, N–H. Additionally, the peak at 1191 cm−1 was ascribed to the C–O, C–N, and C–S bonds.61 The peak at 1023 cm−1 is assigned to the stretching vibration of S
O.62
The XRD pattern of the CDs displays a range of broad peaks, indicating the presence of highly disordered carbon atoms in the as-prepared CDs (refer to Fig. 2).63,64 The CDs exhibited broad 2θ patterns around 22.5 and 41°, attributed to disordered carbon atoms which confirms the dominance of C(002) and C(100) planes associated with hexagonal graphite structure of CDs particles.65 The broad XRD pattern is evidence for the crystalline particles with small dimensions. The particle size distribution (Fig. 1C) supports the XRD approach. The Debye–Scherrer equation (D = Kλ/β
cos
θ) establishes the fact that the produced CDs are of small size ascribing to the fact the β-factor in the denominator of the equation is too high for broad peak around 2θ = 15–30° and consequently the crystallite size will be very small.
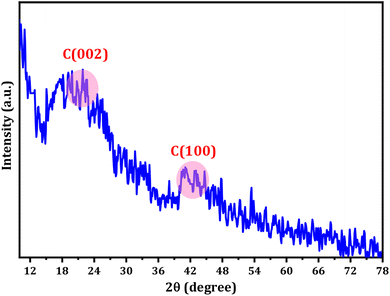 |
| Fig. 2 The XRD spectra of the prepared CDs. | |
3.2 The FL properties of CDs
The optical characteristics of the carbon dots were assessed using UV-vis and fluorescence spectroscopy. The UV-vis spectrum, illustrated in Fig. 3, revealed a wide absorption band spanning from 250 to 420 nm. Notably, the absorption bands peaked at 283 and 347 nm, matching the π–π* transition and the n–π* transition ascribed to aromatic sp2 hybridization and C
O bonds respectively. These peaks are attributed to the existence of carbonyl-based groups on the surface.35 The excitation and emission spectra were analyzed, and the excitation spectra were obtained by monitoring the emission at 423 nm, revealing a strong peak at 350 nm. The dots exhibited blue emission at 423 nm under 350 nm excitation. The visual confirmation of C-dot synthesis was established. As depicted in Fig. 3B, hydrothermal synthesis produces a colorless solution (in daylight) that transforms into a blue color under UV excitation (360 nm) of the aromatic sp2 domains.
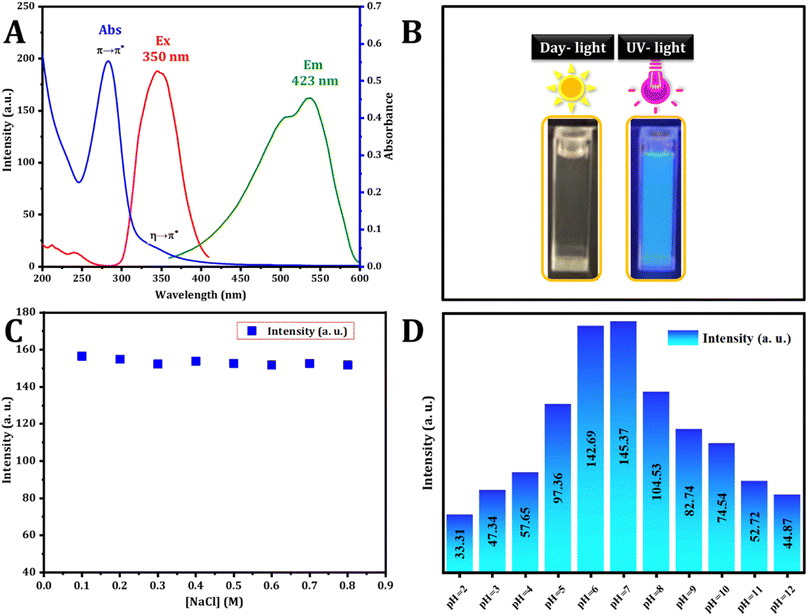 |
| Fig. 3 (A) Absorption (blue line), excitation (red line), and emission (green line) spectra of CDs. (B) Photographs depicting these carbon dots under daylight (left) and 365 nm UV light (right) illumination. (C) Examination of ionic strength at various concentrations of NaCl (0.1 to 0.8 M). (D) pH study of CDs (pH = 2.0–12.0). | |
3.3 The stability experiment
The durability of photoluminescence exhibited by CDs in aqueous settings is a subject of significant attention for realistic claims. Various factors, such as pH and ionic strength, can influence their recital. To explore this, the study initially examined the fluorescence emission intensity of CDs in solutions containing salt and in different aqueous environments at varying pH levels. The strength of the emission experienced minimal impact across various concentrations of NaCl solutions (Fig. 3C), aligning with findings from a prior study. Fig. 3D illustrates how the fluorescence intensity of CDs is influenced by pH. The CDs synthesized exhibit stability within the pH range of 2.0 to 12.0, rendering them suitable for a variety of applications.
The stability analysis of CDs reveals no significant change in fluorescence intensity during the three month storage period, as depicted in Fig. 4A. This result suggests that the CDs remained stable even after three months, with minimal variation.
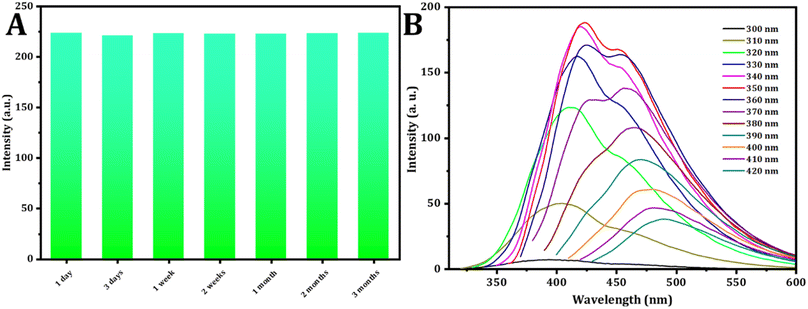 |
| Fig. 4 (A) Effect of time on the intensity of the CDs. (B) Study of excitation spectra (from 300 to 420 nm) of CDs. | |
The fluorescence spectra of the C-dots solution, prepared under normal temperature conditions, were recorded. When excited at varying wavelengths spanning from 300 nm to 420 nm with a 10 nm increment, as shown in Fig. 4B, the FL intensity of emission peaks initially increased under excitation from 300 to 350 nm. Subsequently, after excitation at 350 nm, it gradually decreased. These findings indicate that the optimal emission was observed at 423 nm when excited at 350 nm.
3.4 Selectivity studies
Various metal ions and molecules may exist in the real solution. The influence of 20 kinds of metal ions and molecules on the fluorescence intensity is compared. The procedure involved creating stock solutions of metals and molecules (0.01 M) and adding 500 μL of the probe to a total volume of 4 mL, resulting in a final concentration of interferences (1 mM). The fluorescence intensity of the mixtures was then recorded at an excitation wavelength of 350 nm. It was found that only Fe3+ ions can selectively quench the fluorescence of synthetic materials, as shown in Fig. 5. Metal ions, including Fe3+, Al3+, Co2+, Cu2+, Hg2+, Mn2+, Mg2+, Ni2+, Zn2+, Pb2+, and Cd2+, along with molecules such as citric acid (CA), arginine (Arg), alanine (Ala), L-aspartic acid (L-AA), glycine (Gly), ascorbic acid (AA), histidine (His), phenylalanine (Phy), and cysteine (Cys), were chosen to investigate their influence on C-dots.
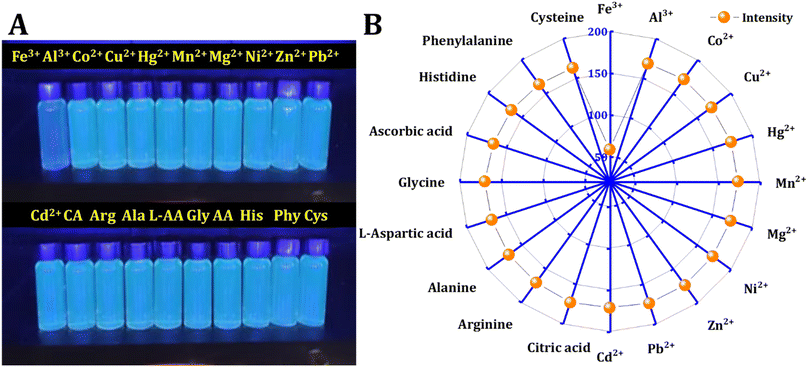 |
| Fig. 5 Interferences: (A) naked-eye detection of metal ions and molecules. (B) Fluorescence intensity of CD in the presence of 500 μM of various metal ions and molecules. | |
3.5 Analytical application
To conduct a quantitative investigation and set up calibration curves, a series of standard Fe3+ solutions were examined. Following optimized circumstances; the calibration plot was generated by drawing fluorescence intensity against concentrations of standard Fe3+, which is determined by the different degrees of the inter-filter effect, as depicted in Fig. 7.66 As seen in Fig. 6A, the fluorescence intensity exhibited a decrease as the Fe3+ concentration increased from 10 μM to 180 μM. simultaneously, the fluorescence emission peak at 423 nm lowered. Fig. 6B describes the relationship between Fe3+ ion concentration and FL intensity in the range from 10 to 180 μM, in accordance with the following equation:
F423 = 132.0203 − 0.5348[Fe3+] |
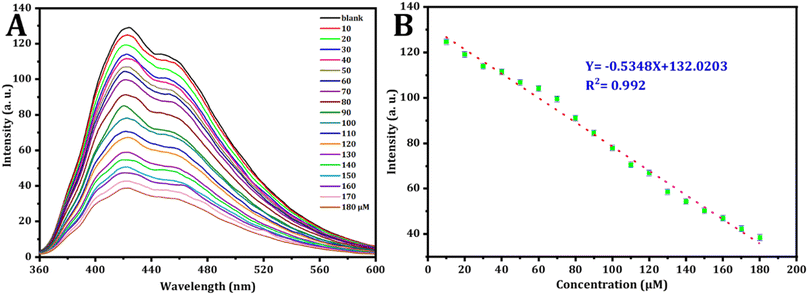 |
| Fig. 6 (A) The fluorescence behavior of CDs in the existence of varying concentrations of Fe3+ was examined. (B) Corresponding relationship between FL intensity and the concentrations of Fe3+ in μM. | |
The correlation coefficient is 0.992, the calculated detection limit for Fe3+ ions is 0.409 μM (S/N = 3) and the limit of quantification is 1.365 μM.
CDs have high sensitivity compared with different probes that have been used to quantification of Fe3+ in drinking water as shown in Table 1.
Table 1 Comparison of other CDs as probes for fluorescence Fe3+ detection in real samples
Probe |
Real sample |
Linear range (μM) |
LOD |
Ref. |
N,S–GQDs |
Drinking water |
0.1–30 |
55.49 nM |
67 |
CDs |
Drinking water |
8–80 |
3.8 μM |
68 |
CDs |
Tap water |
0.3–7 |
95 nM |
69 |
CDs |
Lake water |
0.0–250 |
1.68 μM |
70 |
N–CQDs/PTCA |
Tape water |
20–60 |
0.04 μM |
71 |
CDs |
Milk |
0.0–200 |
35 nM |
72 |
Phe–CDs |
Tap water |
5.0–500 |
0.720 μM |
73 |
CDs |
Underground water |
30–600 |
9.55 μM |
74 |
N–CQDs |
Tap water |
0.5–1000 |
0.079 μM |
75 |
CDs |
Serum and tap water |
0–180 |
409 nM |
This work |
3.6 Detection of Fe3+ in real samples
To assess the applicability of this developed technique, standard recovery experiments were carried out using samples of human serum and tap water (refer to Table 2). The results in Table 2 indicate that the recoveries for all samples ranged from 95.60% to 102.46% for human blood serum and from 83.75% to 90.26% for tap water. The associated relative standard deviations (RSDs) were within the ranges of 0.96% to 2.66%. These findings provide robust evidence supporting the potential of the proposed approach for the practical detection of Fe3+ in actual samples.
Table 2 Recovery results and spiking recoveries of Fe3+ in human blood serum, and water (n = 3)
Sample |
Added (μM) |
Found (μM) |
Recovery% |
RSD% |
Serum |
80 |
77.31 |
95.60 |
1.87 |
90 |
95.74 |
102.46 |
2.08 |
Tap water |
40 |
36.22 |
83.75 |
0.96 |
50 |
47.85 |
90.26 |
2.66 |
3.7 Greenness profile of the method
Recently, energy, waste, and hazard management have been recognized as crucial to improving green analysis. In this work, three different green metrics were utilized to evaluate the greenness of the proposed approach, comprising the analytical eco-scale (AES),76 the complimentary green analytical procedure index (ComplexGAPI),77 and the Analytical Greenness approach (AGREE).78 AES is a simple and semi-quantitative tool that is based on penalty points (PP) for different experimental steps subtracted from a total score of 100. The PPs are assigned for each step of analytical parameters such as the nature and quantity of solvents and reagents employed, energy consumption, hazards, and waste generated. From the results listed in Table 3, one can observe that the proposed method was given a score of 82 for tap water and 78 for blood serum samples, which confirms the greenness of the approach.
Table 3 Greenness evaluation of the proposed method according to the AESa
Category |
PPs (tap water) |
Category |
PPs (blood serum) |
PPs: penalty points. The assessment was conducted on a per-sample basis. Scores exceeding 75 are classified as excellent green analysis, scores within the range of 50–75 are considered acceptable green analysis, and scores below 50 are deemed inadequate green analysis. |
Reagents |
Water |
0 |
Water |
0 |
Ferric nitrate |
6 |
Ferric nitrate |
6 |
Chloroform |
6 |
Chloroform |
6 |
|
|
Ethanol |
4 |
![[thin space (1/6-em)]](https://www.rsc.org/images/entities/char_2009.gif) |
Instruments |
Spectrofluorometer |
0 |
Spectrofluorometer |
0 |
Occupational hazard |
0 |
Occupational hazard |
0 |
Waste |
6 |
Waste |
6 |
Total PPs: 18 score: 82 |
Total PPs: 22 score: 78 |
The ComplexGAPI metrics software is an innovative tool built upon GAPI, featuring an additional hexagonal area at its base. ComplexGAPI is essential for encompassing the entire experimental process, including pre-analysis procedures spanning from sample preparation to the final analysis. It covers various aspects such as yield and environmental factors, association with the green economy, workup, instrumentation, reagents and solvents, and purification. As in GAPI, the color scale of the pictogram changes from green to yellow to red with respect to the low, medium, and high environmental effects. However, the overall results of ComplexGAPI, as shown in Fig. 7, indicate the greenness of the method. In the suggested procedure, micro-scale extraction was applied as a pre-treatment step for protein precipitation in the blood serum samples to improve the sensitivity of the analysis. Due to the minimal quantity of reagent and solvent waste generated, the procedure seems to be eco-friendly. Whey was utilized as an environmentally friendly carbon source in CD production. Furthermore, the spectrofluorimeter consumes little energy per analysis.
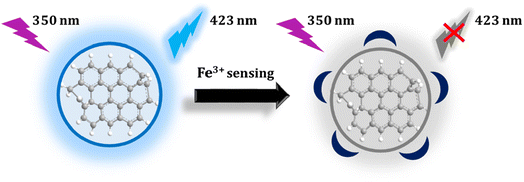 |
| Fig. 7 Schematic of the CDs as an on–off' fluorescent sensor for the detection of Fe3+. | |
AGREE Metrics is a software application that depends on a set of 12 criteria to evaluate the environmental and occupational risks involved in an analytical procedure. These criteria are arranged in a circular pattern resembling the face of a traditional clock, with scores ranging from 0 to 1. The average score, situated at the center, serves as an indicator of the environmental sustainability of the approach. As illustrated in Fig. 8, the high score obtained with the AGREE software reflects the adoption of the excellent green methodology.
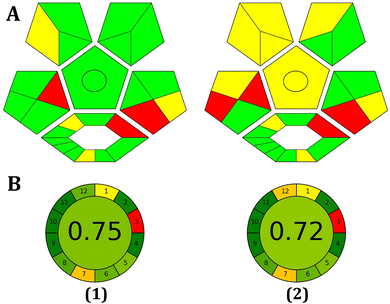 |
| Fig. 8 Evaluation of the environmental friendliness of the proposed method using ComplexGAPI (A) and AGREE (B) scales in tap water (1) and blood serum samples (2). | |
4 Conclusion
Using yogurt whey as a precursor, carbon dots were produced in a one-step hydrothermal method. When stimulated with the maximal excitation wavelength of 350 nm, the produced CDs emit blue fluorescent colors at 423 nm. The analytical figure of merit of CDs was investigated, and it demonstrates great selectivity and sensitivity with a low limit of detection for detecting Fe3+ ions in various matrixes. The addition of ferric ions may nearly completely suppress the fluorescence of carbon dots via a fluorescence “on/off” process. Furthermore, by analyzing multiple criteria for the method's greenness, we propose that these carbon dots, due to their greater environmental friendliness, might be used to monitor Fe3+ ions in a range of biological settings and for environmental monitoring.
Ethical statement
This article does not contain any studies with human or animal subjects.
Consent to participate
All authors provided their consent for the inclusion of their work in the manuscript.
Data availability
All data generated and analyzed during the current study are included in the manuscript.
Conflicts of interest
The authors declare no competing interests.
Acknowledgements
Special thanks to the University of Sulaimani and the University of Garmian for all of their cooperation.
References
- J. Zhou, C. Zou, D. Huo, C. Chu, S. Liu, M. Yang, S. Zhang, X. Wang and C. Hou, Anal. Methods, 2023, 15, 5181–5189 RSC.
- Z. Li, Y. Cao, T. Feng, T. Wei, C. Xue, Z. Li and J. Xu, Anal. Methods, 2023, 15, 1923–1931 RSC.
- U. Latief, S. ul Islam, Z. M. S. H. Khan and M. S. Khan, Spectrochim. Acta, Part A, 2021, 262, 120132 CrossRef CAS PubMed.
- Y. Sun, M. Zhang, B. Bhandari and C. Yang, Food Rev. Int., 2022, 38, 1513–1532 CrossRef CAS.
- J. Bi, Y. Li, H. Wang, Y. Song, S. Cong, D. Li, D. Zhou, B. W. Zhu and M. Tan, New J. Chem., 2017, 41, 8490–8496 RSC.
- S. Dua, P. Kumar, B. Pani, A. Kaur, M. Khanna and G. Bhatt, RSC Adv., 2023, 13, 13845–13861 RSC.
- J. Wei, J. Shen, X. Zhang, S. Guo, J. Pan, X. Hou, H. Zhang, L. Wang and B. Feng, RSC Adv., 2013, 3, 13119–13122 RSC.
- H. H. Jing, F. Bardakci, S. Akgöl, K. Kusat, M. Adnan, M. J. Alam, R. Gupta, S. Sahreen, Y. Chen, S. C. B. Gopinath and S. Sasidharan, J. Funct. Biomater., 2023, 14, 1–27 CrossRef PubMed.
- F. J. Chao-Mujica, L. Garcia-Hernández, S. Camacho-López, M. Camacho-López, M. A. Camacho-López, D. R. Contreras, A. Pérez-Rodríguez, J. P. Peña-Caravaca, A. Páez-Rodríguez, J. G. Darias-Gonzalez, L. Hernandez-Tabares, O. A. de Fuentes, E. Prokhorov, N. Torres-Figueredo, E. Reguera and L. F. Desdin-García, J. Appl. Phys., 2021, 129, 163301 CrossRef CAS.
- A. Kaczmarek, J. Hoffman, J. Morgiel, T. Mościcki, L. Stobiński, Z. Szymański and A. Małolepszy, Materials, 2021, 14, 1–13 Search PubMed.
- D. Rocco, V. G. Moldoveanu, M. Feroci, M. Bortolami and F. Vetica, ChemElectroChem, 2023, 10, 202201104 CrossRef PubMed , (1–15)..
- M. Javed, A. N. S. Saqib, A. U. Rehman, B. Ali, M. Faizan, D. A. Anang, Z. Iqbal and S. M. Abbas, Electrochim. Acta, 2019, 297, 250–257 CrossRef CAS.
- A. Sharma and J. Das, J. Nanobiotechnol., 2019, 17, 1–24 CrossRef CAS PubMed.
- Z. Ma, H. Ming, H. Huang, Y. Liu and Z. Kang, New J. Chem., 2012, 36, 861–864 RSC.
- W. L. Ang, Q. A. Alqasem and A. W. Mohammad, IOP Conf. Ser.: Mater. Sci. Eng., 2021, 1195, 012008 CAS.
- D. Stefanakis, A. Philippidis, L. Sygellou, G. Filippidis, D. Ghanotakis and D. Anglos, J. Nanoparticle Res., 2014, 16, 2646 CrossRef.
- O. B. A. Shatery and K. M. Omer, ACS Omega, 2022, 7, 16576–16583 CrossRef CAS PubMed.
- M. P. Romero, F. Alves, M. D. Stringasci, H. H. Buzzá, H. Ciol, N. M. Inada and V. S. Bagnato, Front. Microbiol., 2021, 12, 1–13 Search PubMed.
- W. Zhou and J. J. Coleman, Curr. Opin. Solid State Mater. Sci., 2016, 20, 352–360 CrossRef CAS.
- S. V. Rempel', A. A. Razvodov, M. S. Nebogatikov, E. V. Shishkina, V. Y. Shur and A. A. Rempel', Phys. Solid State, 2013, 55, 624–628 CrossRef.
- T. M. Abeywickrama, A. Hassan and P. T. Snee, Front. Chem., 2018, 6, 1–9 CrossRef PubMed.
- J. Fan, L. Kang, X. Cheng, D. Liu and S. Zhang, Nanomaterials, 2022, 2, 172–192 Search PubMed.
- E. A. Stepanidenko, E. V. Ushakova, A. V. Fedorov and A. L. Rogach, Nanomaterials, 2021, 11, 1–20 CrossRef PubMed.
- R. M. S. Sendão, J. C. G. E. da Silva and L. P. da Silva, Catalysts, 2023, 13, 179 CrossRef.
- N. Azam, M. N. Ali and T. J. Khan, Front. Mater., 2021, 8, 1–21 Search PubMed.
- P. Koutsogiannis, E. Thomou, H. Stamatis, D. Gournis and P. Rudolf, Adv. Phys.: X, 2020, 5, 1758592 CAS.
- I. A. Baragau, N. P. Power, D. J. Morgan, R. A. Lobo, C. S. Roberts, M. M. Titirici, V. Middelkoop, A. Diaz, S. Dunn and S. Kellici, ACS Sustain. Chem. Eng., 2021, 9, 2559–2569 CrossRef CAS.
- J. Zhou, Z. Sheng, H. Han, M. Zou and C. Li, Mater. Lett., 2012, 66, 222–224 CrossRef CAS.
- X. Y. Jiao, L. s. Li, S. Qin, Y. Zhang, K. Huang and L. Xu, Colloids Surf., A, 2019, 577, 306–314 CrossRef CAS.
- C. Yang, R. Ogaki, L. Hansen, J. Kjems and B. M. Teo, RSC Adv., 2015, 5, 97836–97840 RSC.
- B. Wang, H. Song, Z. Tang, B. Yang and S. Lu, Nano Res., 2022, 15, 942–949 CrossRef CAS.
- V. N. Mehta, S. Jha, H. Basu, R. K. Singhal and S. K. Kailasa, Sens. Actuators, B, 2015, 213, 434–443 CrossRef CAS.
- J. Mohammed, P. K. Desu, J. R. Namratha and G. K. Rao, Adv. Pharmacol. Pharm., 2023, 11, 36–45 CAS.
- Y. Liang, L. Xu, K. Tang, Y. Guan, T. Wang, H. Wang and W. W. Yu, Dyes Pigm., 2020, 178, 108358 CrossRef CAS.
- S. Moonrinta, B. Kwon, I. In, S. Kladsomboon, W. Sajomsang and P. Paoprasert, Opt. Mater., 2018, 81, 93–101 CrossRef CAS.
- S. P. Gupta, MOJ Bioorg. Org. Chem., 2018, 2, 221–224 Search PubMed.
- S. J. Oppenheimer, J. Nutr., 2001, 131, 616–635 CrossRef PubMed.
- S. Sharma, S. Chandra, A. Kumar, P. Bindraban, A. K. Saxena, V. Pande and R. Pandey, Indian J. Microbiol., 2019, 59, 344–350 CrossRef CAS PubMed.
- M. Sánchez, L. Sabio, N. Gálvez, M. Capdevila and J. M. Dominguez-Vera, IUBMB Life, 2017, 69, 382–388 CrossRef PubMed.
- D. C. P. Gupta, IOSR J. Appl. Chem., 2014, 7, 38–46 CrossRef.
- N. Abbaspour, R. Hurrell and R. Kelishadi, J. Res. Med. Sci., 2014, 19, 3–11 Search PubMed.
- P. Brissot, A. Pietrangelo, P. C. Adams, B. De Graaff, C. E. McLaren and O. Loreál, Nat. Rev. Dis. Prim., 2018, 4, 18016 CrossRef PubMed.
- B. J. Cherayil, Arch. Immunol. Ther. Exp., 2010, 58, 407–415 CrossRef CAS PubMed.
- S. Ni, Y. Yuan, Y. Kuang and X. Li, Front. Immunol., 2022, 13, 1–11 Search PubMed.
- M. K. Kim, K. H. Baek, K. H. Song, M. I. Kang, J. H. Choi, J. C. Bae, C. Y. Park, W. Y. Lee and K. W. Oh, Am. J. Hypertens., 2012, 25, 492–497 CrossRef CAS PubMed.
- R. K. Koshal, Water, Air, Soil Pollut., 1976, 5, 289–297 CrossRef.
- K. F. Kayani, N. N. Mohammad, D. A. Kader and S. J. Mohammed, ChemistrySelect, 2023, 8, 202303472 CrossRef.
- S. Tautkus, L. Steponeniene and R. Kazlauskas, J. Serb. Chem. Soc., 2004, 69, 393–402 CrossRef CAS.
- H. Wang, P. Zhang, J. Chen, Y. Li, M. Yu, Y. Long and P. Yi, Sens. Actuators, B, 2017, 242, 818–824 CrossRef CAS.
- M. Lu and R. G. Compton, Electroanalysis, 2013, 25, 1123–1129 CrossRef CAS.
- A. Lochab, R. Sharma, S. Kumar and R. Saxena, Mater. Today: Proc., 2020, 45, 3741–3753 Search PubMed.
- X. Xu, S. Yang, Y. Wang and K. Qian, Green Anal. Chem., 2022, 2, 100020 CrossRef.
- N. De Acha, C. Elosúa, J. M. Corres and F. J. Arregui, Sensors, 2019, 19, 599 CrossRef PubMed.
- P. Li and S. F. Y. Li, Nanophotonics, 2021, 10, 877–908 CrossRef CAS.
- K. F. Kayani and K. M. Omer, New J. Chem., 2022, 46, 8152–8161 RSC.
- J. Shangguan, J. Huang, D. He, X. He, K. Wang, R. Ye, X. Yang, T. Qing and J. Tang, Anal. Chem., 2017, 89, 7477–7484 CrossRef CAS PubMed.
- S. J. Mohammed, K. M. Omer and F. E. Hawaiz, RSC Adv., 2023, 13, 14340–14349 RSC.
- M. Li and S. X. A. Zhang, Chem. Mater., 2014, 26, 6084 CrossRef CAS.
- Q. Wu, X. J. Duan, H. T. Lv and L. T. Wang, Food Control, 2023, 149, 1–7 Search PubMed.
- G. Li, C. Liu, X. Zhang, P. Luo, G. Lin and W. Jiang, Food Chem., 2021, 355, 129443 CrossRef CAS PubMed.
- X. Cui, Y. Wang, J. Liu, Q. Yang, B. Zhang, Y. Gao, Y. Wang and G. Lu, Sens. Actuators, B, 2017, 242, 1272–1280 CrossRef CAS.
- T. Yoshinaga, M. Akiu, Y. Iso and T. Isobe, J. Lumin., 2020, 224, 117260 CrossRef CAS.
- J. Feng, Y. Chen, Y. Han, J. Liu, C. Ren and X. Chen, Anal. Chim. Acta, 2016, 926, 107–117 CrossRef CAS PubMed.
- Y. Wang, S. H. Kim and L. Feng, Anal. Chim. Acta, 2015, 890, 134–142 CrossRef CAS PubMed.
- T. N. J. I. Edison, R. Atchudan, M. G. Sethuraman, J. J. Shim and Y. R. Lee, J. Photochem. Photobiol., B, 2016, 154–161 CrossRef CAS PubMed.
- L. Shi, T. Wang, H. Zhang, K. Chang, X. Meng, H. Liu and J. Ye, Adv. Sci., 2015, 2, 1–8 CAS.
- Y. Yang, T. Zou, Z. Wang, X. Xing, S. Peng, R. Zhao, X. Zhang and Y. Wang, Nanomaterials, 2019, 9, 738 CrossRef CAS PubMed.
- Y. Chen, X. Sun, W. Pan, G. Yu and J. Wang, Front. Chem., 2020, 7, 1–9 RSC.
- J. Deng, J. Hu, J. Zhao, N. An, K. Liang, Q. Wang, Z. Zhang, R. Wu and F. Zhang, Arab. J. Chem., 2021, 14, 103195 CrossRef CAS.
- Z. Xiang, Y. Jiang, C. Cui, Y. Luo and Z. Peng, Molecules, 2022, 27, 6749 CrossRef CAS PubMed.
- K. Wang, J. Chen, H. Li, M. Zhang, Q. Liao, L. Wang, Y. Zhang and X. Niu, Ionics, 2021, 27, 4907–4916 CrossRef CAS.
- A. Iqbal, Y. Tian, X. Wang, D. Gong, Y. Guo, K. Iqbal, Z. Wang, W. Liu and W. Qin, Sens. Actuators, B, 2016, 237, 408–415 CrossRef CAS.
- Z. F. Pu, Q. L. Wen, Y. J. Yang, X. M. Cui, J. Ling, P. Liu and Q. E. Cao, Spectrochim. Acta, Part A, 2020, 229, 117944 CrossRef CAS PubMed.
- M. Zulfajri, G. Gedda, C. J. Chang, Y. P. Chang and G. G. Huang, ACS Omega, 2019, 4, 15382–15392 CrossRef CAS PubMed.
- P. Lv, C. Wang, C. Wang, D. Sun, Y. Yao, H. Zhou, J. Zhang and Z. Pang, Nanotechnology, 2017, 28, 165502 CrossRef PubMed.
- M. Sajid and J. Płotka-Wasylka, Talanta, 2022, 238, 123046 CrossRef CAS PubMed.
- J. Płotka-Wasylka and W. Wojnowski, Green Chem., 2021, 23, 8657–8665 RSC.
- F. Pena-Pereira, W. Wojnowski and M. Tobiszewski, Anal. Chem., 2020, 92, 10076–10082 CrossRef CAS PubMed.
|
This journal is © The Royal Society of Chemistry 2024 |
Click here to see how this site uses Cookies. View our privacy policy here.