DOI:
10.1039/D3YA00208J
(Paper)
Energy Adv., 2023,
2, 1357-1365
Beyond energy density: flow battery design driven by safety and location†
Received
13th May 2023
, Accepted 5th July 2023
First published on 6th July 2023
Abstract
As renewable energy penetration increases, energy storage is becoming urgently needed for several purposes, including frequency control, peak shifting, and relieving grid congestion. While battery research often focuses on cell level energy density, other aspects of large-scale battery energy storage systems, such as footprint, safety, and storage-duration are frequently overlooked. Here, we investigate forty-four MWh-scale battery energy storage systems via satellite imagery and show that the building footprint of lithium-ion battery systems is often comparable to much less energy-dense technologies such as aqueous flow batteries. We show that due to their intrinsic safety, aqueous chemistries can be built more vertically, enabling smaller footprints, and unlocking new use cases. Transmission constraints for example have a growing impact on energy prices and grid stability, and due to regulatory and practical challenges limiting the addition of more transmission capacity, storage deployed in strategic locations can play a critical role in mitigating grid congestion. Such grid-nodes are often found near energy consumers, where land cost and safety concerns are significant. Deployments in urban areas to locally mitigate congestion and installations in buildings or underground thus provide compelling value propositions for aqueous battery chemistries beyond direct competition with lithium-ion batteries near renewable generation sites.
Main
The low cost of renewables and recent geopolitical events have catalyzed a mass-scale adoption of renewable energy sources that will further gain momentum in coming years.1 This shift towards intermittent sources, however, poses unprecedented challenges for power grids due to mismatches between supply and demand both in time and, crucially, location. For instance, solar power generation drops off in the evening just before peak demand, creating the well-known “duck curve” that represents the difference between energy demand and the amount of available solar energy throughout the day.2 Moreover, transmission limitations result in variations of locational marginal energy prices (LMP, see Supplementary Discussion, ESI†) of hundreds of dollars per MWh between locations that are separated by only a few kilometers, as shown in Fig. 1. The cost-breakdown and overwhelming contribution of grid congestion to real-time electricity prices at several network nodes in the California power grid is shown in Fig. S1–S3 (ESI†).3,4 Note the maximum difference of more than one thousand dollars per MWh at 8:50 a.m. on November 2, 2022, between two network nodes that are separated by only 1.5 km (Fig. S3e and f, ESI†). In Fig. S4 (ESI†) we further show the contribution of congestion to LMP at one node in San Diego, California, throughout 2022. The extreme variability of congestion costs indicates that with more distributed energy production, a similarly scaled distributed energy storage system will be necessary to mitigate the substantial expansion of transmission and distribution infrastructure. Here, large-scale battery energy storage systems (BESS) can be attractive to grid operators by buffering loads at strategic network nodes to alleviate congestion in what has been called storage-as-transmission, or “Grid Booster”.5–7
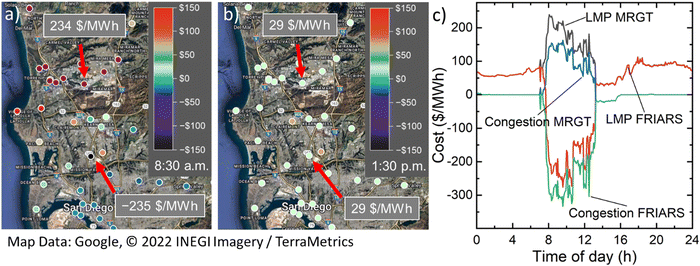 |
| Fig. 1 Real-time electricity prices in the renewable-rich power grid operated by the California Independent System Operator. (a and b) Locational Marginal Prices in dollar per MWh in the San Diego, California region for various network nodes on November 2, 2022, at (a) 8:30 a.m. and (b) 1:30 p.m., the bottom of the duck curve. The two marked nodes, FRIARS_1_N009 and MRGT_6_NODE1, are separated by only 10 km. (c) Real-time Locational Marginal Prices (LMP) and contribution of congestion to cost of electricity at those nodes in five-minute increments on November 2, 2022. Additional examples are shown in Fig. S1–S3 and the supplementary discussion (ESI†). All data obtained from ref. 3, 8. | |
A nexus of political and climatic factors further underscores the urgency of deploying grid-scale storage. Historic heatwaves, droughts, and other extreme weather events ever more regularly threaten power grids with both demand and supply shocks that are exacerbated by transmission limitations. The importance of resilient power grids and the economic impact of grid failures was tragically demonstrated by the February 2021 Texas Power Crisis that resulted in 246 deaths9 and economic losses of at least $195 billion.10,11
In a seminal push towards green grid resiliency, the California Independent System Operator (CAISO) has invested heavily in MWh-scale BESS and has seen great success. During a recent heatwave BESS provided 3.3 GW, or 6.8% of total supply, when demand peaked on the evening of September 5, 2022, providing grid stability and preventing blackouts.12,13 These BESS mainly use lithium-ion batteries (LIB) and are commonly deployed in rural areas where land cost is a small component of the installations’ overall budget, and safety risks can easily be mitigated by minimizing exposure to surrounding structures. However, to achieve deep decarbonization of the power grid and to relieve congestion without large-scale construction of expensive power lines, considerable energy storage will be necessary in densely populated areas as well. This makes safety and footprint of a BESS installation important design considerations that are often overlooked in the existing literature.
With a plethora of available BESS technologies, including lithium-ion, sodium–sulfur and flow batteries, much attention has been dedicated to energy density as a key metric for economic and practical viability.14–22 In fact, low energy density is frequently highlighted as the key limitation of flow batteries, with the energy density on cell level typically being an order of magnitude lower than for LIBs.14–21,23,24 As such, significant research efforts are being dedicated to finding new active materials, ideally with high solubility and multi-electron redox properties, translating into higher energy storage capacity per volume of electrolyte.17,25–29 This trend extends to other aqueous battery chemistries that are envisioned for stationary applications, where tremendous efforts are devoted to electrolyte development and overcoming cell voltage limitations imposed by the narrow electrochemical stability window of water.30–36 This stands in contrast to the fact that large BESS are most often found in locations with plentiful space and it is unclear how cell level energy density translates to MWh-scale installation footprint.
Here, we use satellite imagery to compare the building footprint of deployed MWh-scale BESS and to determine the areal energy density of installations with 1–900 MWh energy capacity, expressed in kWhm−2. We show that even demonstrator-scale flow batteries can achieve areal energy densities comparable to many LIB systems. Moreover, we demonstrate that aqueous batteries offer a key untapped advantage: their intrinsic non-flammability allows for vertical scalability. Sufficiently tall electrolyte tanks or container stacks enable unparalleled areal storage capacities, and they can safely be deployed underground or in buildings, unlocking added land value and use cases inaccessible to state-of-the-art LIBs. Therefore, in addition to deploying flow batteries at generation sites in wide-open spaces, directly competing with LIB-BESS, these batteries could be an ideal solution for installations at strategic locations near consumers in densely developed areas.
Deployed MWh-scale BESS
Utility-scale BESS are currently seeing tremendous deployment, with the global power capacity of BESS increasing from just 16 GW at the end of 2021 to a projected 345–585 GW by 2030.37,38 Interestingly, a concomitant change in the hydropower narrative is observed as installations such as the recently commissioned Nant de Drance plant in Switzerland are ever more often described as “water batteries”.39
Using satellite imagery, we show the areal energy density (the installation's energy rating divided by its footprint, Fig. M1, ESI†), of MWh-scale BESS using lithium-ion, sodium–sulfur, and aqueous flow batteries. The combined capacity of the studied systems considered for footprint calculations amounts to a total of 2752 MW/7879 MWh, corresponding to 15% of the globally installed BESS capacity at the end of 2022.40Fig. 2 shows satellite images for the largest lithium-ion and flow batteries deployed to date, and an extensive list of all examined BESS, including an additional 2505 MW/6535 MWh for which no exact footprint could be determined, can be found in the Supplementary Information (Fig. S7–S75, ESI†).
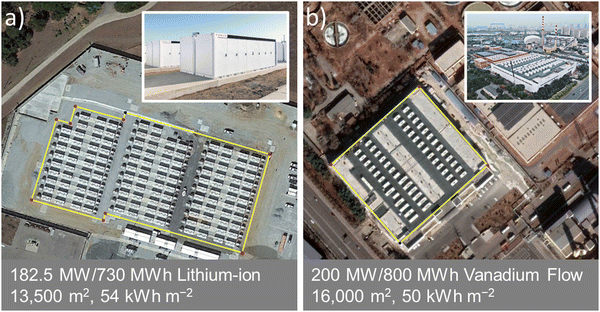 |
| Fig. 2 Satellite images and photos of some of the largest battery energy storage systems deployed to date. (a) Lithium-ion batteries in Moss Landing, California: Elkhorn Battery, operated by Pacific Gas & Electric Company; 182.5 MW/730 MWh, 54 kWh m−2. Map Data: Google, © 2022 AMBAG, Maxar Technologies, Photo adapted from ref. 42. (b) Vanadium flow battery in Dalian, China, by Dalian Rongke Power Co. Ltd; 100 MW/400 MWh, 25 kWh m−2. A second phase of equal capacity in the same building is under construction, bringing the areal energy density to 50 kWh m−2. Map Data: Google, © 2022 Maxar Technologies, Photo adapted from ref. 43. | |
LIB installations, often arranged in blocks of two units, enable areal energy densities of up to 62 kWh m−2, and over the 21 LIB-systems studied here we observe an average of 34 kWh m−2 (Table 1, Fig. 5a and Table S1, ESI†). It is noteworthy that only two installations could be identified where LIB containers are stacked, resulting in 26–33 kWh m−2. The average storage duration of the BESS examined here is 2.6 hours (Fig. S6a and Table S1, ESI†). Most deployed systems to date are based on cells using nickel manganese cobalt oxide cathode materials, but manufacturers are shifting towards less energy-dense lithium iron phosphate due to improved cost and safety aspects.38,41 We point out how much space is available around most large-scale LIB deployments, particularly near production facilities like solar farms (Fig. S18, S24 and S26, ESI†), and how the excellent cell level energy density of LIBs is largely rendered irrelevant in this application.
High-temperature sodium–sulfur batteries were a leading technology for BESS until 2010, when LIBs became generally favored for new installations, and several containerized MWh-scale systems have been in operation for more than a decade now.44 The primary developer, NGK Insulators Ltd, has roughly 5 GWh of installed energy capacity worldwide.45 Containers are commonly found to be stacked and deployed systems afford an average areal energy density of 30 kWh m−2, comparable to most LIB-BESS (Table 1 and Fig. S5b, ESI†). They are optimized for six hours of storage (Fig. S6b and Table S2, ESI†) and need to be cycled regularly for maintaining the high temperature required for operation.
Table 1 Summary of investigated MWh-scale BESS
Type of BESS |
Number of installations |
Average power rating (MW) |
Average energy capacity (MWh) |
Average energy density (kWh m−2) |
Average duration (hours) |
Lithium-ion |
21 |
118 ± 114 |
261 ± 312 |
34 ± 15 |
2.6 ± 1.6 |
Sodium–sulfur |
8 |
16 ± 16 |
102 ± 103 |
30 ± 20 |
6.3 ± 0.6 |
Aqueous flow |
15 |
10 ± 25 |
38 ± 98 |
15 ± 12 |
4.3 ± 1.9 |
Flow batteries made up only 1% of installed battery capacity in the United States by the end of 2018, globally only 350 MWh, and most installations were considered demonstrators for the technology.44 Emerging from the demonstrator-phase, deployment has more than doubled in 2019 alone, primarily via large installations in China.46 The largest vanadium flow battery in the world with a capacity of 100 MW/400 MWh was commissioned by Dalian Rongke Power Co. Ltd, with double this capacity planned in the same building.43 Deployed MWh-scale vanadium flow batteries achieve an average areal energy density of 15 kWh m−2 in containerized systems (Table 1, Fig. S5c and Table S3, ESI†) and are rated for approximately four hours of storage duration (Fig. S6c, ESI†). When deployed in the classically envisioned sense, using large tanks spatially separated from the cell stacks rather than containerized systems, flow batteries achieve up to 50 kWh m−2, in line with manufacturers’ advertisements (Fig. S53, S57–S59, S64 and S67, ESI†). Importantly, the technology can safely be implemented in multi-purpose buildings, as demonstrated by the 2 MW/20 MWh battery at the Fraunhofer Institute für Chemische Technologie in Germany (33 kWh m−2) or the 100 MW/400 MWh Rongke Power battery in Dalian, China (25 kWh m−2, 50 kWh m−2 upon completion); see Fig. S54 and S55 (ESI†). Alternative cell chemistries such as all-iron batteries have seen success with containerized installations achieving areal energy densities comparable to vanadium systems (Fig. S69 and S70, ESI†) and zinc–bromine flow batteries have been successful for smaller residential installations and one MWh-scale deployment (Fig. S73, ESI†).
Implications for state-of-the-art BESS
Lithium-ion batteries fulfill many of the requirements for short-term balancing of grid supply and demand, such as frequency control and peak shifting, thanks to excellent response times and high output power. Accordingly, LIBs currently dominate the BESS sector with a market share above 90%, largely thanks to technological maturity and the low costs brought about by the rapidly growing electric vehicle industry.37 With stretched supply chains and growing price pressures47 it is, however, important to discuss limitations of LIBs and to recognize where different technologies can complement each other.
The combination of high energy-density electrode materials and flammable electrolytes in LIBs presents a safety risk at scale. Burning MWh-scale LIBs cannot be put out like conventional fires and first responders are advised to allow a failed unit to burn out while protecting surrounding exposures.48 Hence, the National Fire Protection Association's NFPA855 code specifies limits for energy capacity and unit separation distances,49,50 and standards such as UL1973 and UL954051,52 or thermal runaway fire propagation testing methods such as UL9540A53 have been developed to help manufacturers prove their compliance with regulations and overall high industry standards make LIBs very safe to operate. Yet, BESS failures, a recent one in Moss Landing, California resulting in highway closures and shelter-in-place advisories, have attracted considerable media attention leading to reluctance in local communities to approve BESS in populated areas.54 Even with the highest safety standards, potentially catastrophic failures of LIBs cannot be completely excluded due to their intrinsic flammability, which is exacerbated in large-scale installations: Tesla Inc. alone has deployed more than 6 GWh of BESS in the form of its Megapacks, corresponding to about 2400 battery containers and some 350 million individual battery cells.55,56 While the cell failure rate is astoundingly low, at less than one in 100 million, the large number of cells per BESS unit compounds risks. There have been two Megapack fires and two overheating events in the past two years,57,58 corresponding to a BESS unit failure rate of about one per thousand. Globally, there have been almost 50 LIB-BESS failures and malfunctions since 2017, and more such events can be expected over the entire lifespan of these systems.59,60
An additional limitation of LIBs is that they are economical only for short durations and are currently not cost-competitive for durations longer than roughly six hours due to the fixed cost structure of additional modules, as evidenced in Fig. S6a (ESI†).41 The BESS are generally developed as containerized systems that fully integrate battery modules, inverters, and thermal, ventilation, and fire protection systems. Therefore, if twice the amount of energy has to be stored, doubling the storage duration at any given power rating, manufacturing costs double and average cost does not significantly decrease with storage duration. However, flexible resources at different duration levels are needed for a net-zero power system, and long-duration energy storage (LDES, typically >10 h) is increasingly recognized as an integral part of future power grids.41,61–65 For example, the average duration of continuous renewables curtailment in California, CAISO was forced to curtail more than 2.5 TWh of renewable energy in 2023,66 enough to power 235
000 average American households for a year,67 has increased from 2.5 to 9.5 hours since 2014, with some curtailment events even spanning multiple days.66,68 Also, more than three-quarters of New York state's gas-fired peaker plant fleet operates for periods longer than 15 hours when dispatched, and it is estimated that current BESS using LIBs rated at six hours can only cost-effectively replace 6% of these plants.69,70 This fraction is estimated to increase to 83% when LIBs are combined with LDES.69 Note, that most natural gas power plants, e.g., in California, are expected to continue operation in the next two decades, as their role is relegated to providing standby capacity, ensuring grid reliability when renewables fall short.71
Flow batteries as an alternative to lithium-ion BESS
Instead of storing energy in solid electrodes, flow batteries store energy in liquid electrolytes that are circulated through an electrochemical cell to provide power, decoupling power and energy elements. If the installation needs more power, additional cell stacks can be installed, and if the installation needs to store more energy, larger electrolyte tanks can be deployed. Such easy scalability and high safety, due to the intrinsic non-flammability of aqueous electrolytes, make flow batteries particularly promising for LDES, a market that is estimated to reach 1.5 TW/85 TWh to 2.5 TW/140 TWh of capacity, corresponding to up to three trillion USD, by 2040.63
As discussed above, the most often-cited drawback of flow batteries is low electrolyte energy density. However, our analysis of real-world MWh-scale BESS shows that this metric is relatively unimportant to many such installations. Additionally, even if highly soluble materials with suitable properties are identified, realizing energy-dense flow batteries even at lab-scale has proven very challenging. Concentrated electrolytes typically have high viscosity, leading to decreased power performance and low energy efficiency.72,73 Furthermore, high ionic strength can result in osmotic imbalance across the membrane in the cell,74 and high concentrations may negatively affect the longevity of active materials.75,76 This is not to say that energy density is entirely irrelevant, e.g. for indoor systems, but our analysis shows that for the grid-scale use case, for which flow batteries are generally considered, incremental increases of energy density are not going to dramatically improve economic viability. Instead, more brainpower, funding and policy support should be dedicated to improving cost, lifetime, scalability, automated manufacturability, supply chain security, or grid modelling to identify grid nodes where storage-as-transmission could have the highest impact.
Flow batteries for space-restricted energy storage
Counterintuitively, flow batteries may be excellent contenders for commercial and industrial applications where BESS need to be vertically scaled due to limited land availability. For instance, backup power supplies with excellent safety and reliability metrics without transmission risk are required in hospitals, government and corporate buildings, or data centers, where BESS must be deployed in confined spaces. In the following we discuss the impact of vertical scaling on the footprint of BESS.
The achievable areal energy density depends on the volumetric energy density of the electrolytes, the percentage of the site's area devoted to electrolyte storage, hereafter termed space utilization, and the tank height. For volumetric energy density, commercialized vanadium systems range roughly from 25–35 Wh L−1, while alternative chemistries have achieved up to 95 Wh L−1 in laboratory settings.29,77 The stated values correspond to energy density per total volume of electrolyte and systems that employ solid electrodes or capacity boosters can reach even higher values. The space utilization of sites with large non-containerized storage tanks was calculated using satellite images (Fig. M2, ESI†). Three of the four such sites studied here range from 10–15% (Fig. S48, S52 and S72, ESI†), which can be improved with a smaller number of larger tanks: the 120 MWh Regenesys installation achieved 23% space utilization (Fig. S75, ESI†).
Even with no effort to optimize space utilization or electrolyte energy density, modestly tall tanks already allow flow batteries to match or surpass LIB areal energy densities: Fig. 3a shows that with 30 Wh L−1 electrolytes and a space utilization of 15%, a tank height of 8 meters is sufficient to achieve areal energy densities on par with the average of all LIBs considered in this study, 34 kWh m−2. A 40 Wh L−1 electrolyte and 25% space utilization would more than double that. This tank height is chosen as an example because the Enervault demonstrator (Fig. S72, ESI†) had an estimated tank height of 8 m, and the Regenesys system (Fig. S75, ESI†) had 8.3 m tall tanks.
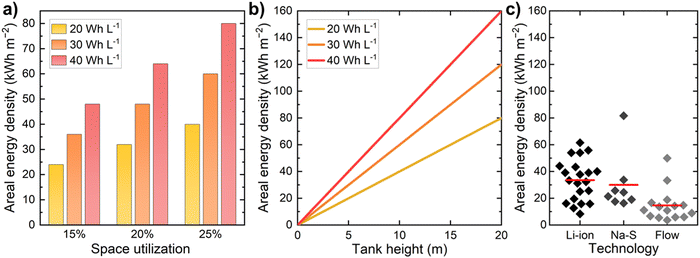 |
| Fig. 3 The potential of vertically scaled flow batteries. (a) Areal energy density in kWh m−2 of hypothetical flow battery installations assuming 8 m tall tanks and a range of electrolyte energy densities and site space utilizations. (b) Areal energy density as a function of tank height for different volumetric energy densities, assuming 20% space utilization. (c) Calculated areal energy densities of deployed lithium-ion, sodium–sulfur, and flow batteries. The red line represents the mean of each technology. | |
Taller tanks can cost-effectively be manufactured: some oil storage tanks hold around 100 million liters, with a typical height of 20 m and a diameter of roughly 80 m.78 Another example is a thermal storage project in Berlin, Germany, where a 45 m tall tank with a diameter of 41 m is under construction.79 Assuming 20% space utilization and 30 Wh L−1, such a tank would translate into 270 kWh m−2, eight times the LIB-BESS average. Fig. 3b shows the areal energy densities that can be achieved for various tank heights with 20–40 Wh L−1 chemistries under an assumption of 20% space utilization, compared to the values calculated in this study for lithium-ion, sodium–sulfur, and Flow BESS (Fig. 3c). We point out that vertical scaling has been exploited for pumped storage hydropower, which currently provides more than 90% of global electricity storage capacity,37 for decades: the volumetric energy density of such systems is exceptionally low, typically below 1 Wh L−1, but the areal energy density, particularly of installations using pre-existing waterbodies as lower reservoirs, even surpasses that of many LIB-BESS (Table S4, ESI†). These calculations only illustrate the issue and there will be a tradeoff between tank height and cost/feasibility. However, they show how vertical scaling can resolve an issue that the research community has often conceived as critical, although in the real-world it has only been of rather low importance.
The volumetric energy densities of current flow battery electrolytes are entirely sufficient to enable compact and energy dense storage solutions where needed, if vertically scaled. Such systems could safely be deployed close to consumers, for example as energy hubs in city centers or black start generators, or in behind-the-meter applications near critical structures and integrated in multi-use buildings. Recent legislation prohibiting the installation of LIB-BESS in residential or school zones on Staten Island, New York, highlights the need for safer alternatives in an urban environment.80Fig. 4 compares contrasting uses of LIB-BESS and flow batteries, and shows how vertical scaling can be exploited for highly efficient land use in densely populated industrial and urban areas, where distributed energy storage will be essential to alleviate grid congestion, among other applications.
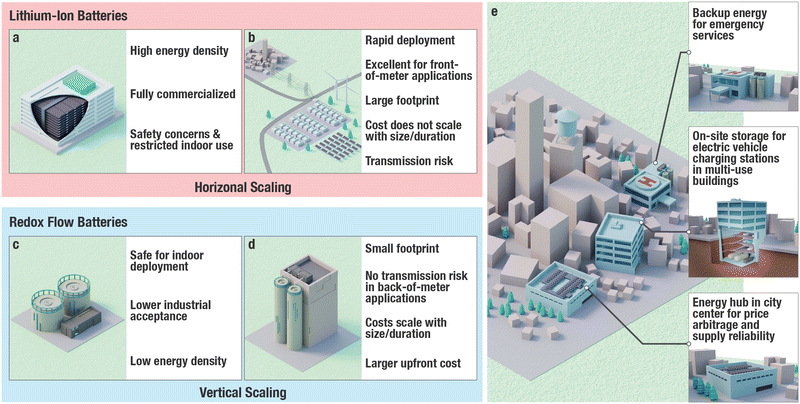 |
| Fig. 4 Schematic comparison of BESS using lithium-ion or flow batteries. (a) LIB-BESS on cell and unit level have high energy density, but due to safety restrictions must be scaled horizontally and cannot be employed indoors. (b) Horizontally scaled LIB-BESS have much shorter construction times than alternative power plants but cannot be deployed at scale in urban areas. Resulting transmission risks make such systems unsuitable for e.g., emergency backup power services. (c) Flow batteries have lower energy density on unit level but can be installed in buildings due to inherent non-flammability. (d) Vertical scaling of flow batteries translates into highly effective land-use but requires large upfront capital expenditures. (e) Vertically scaled flow batteries allow, for example, the deployment of small footprint energy hubs in city centers for price arbitrage, storage-as-transmission, and supply security, emergency backup power for critical infrastructure like hospitals, or on-site storage for electric vehicle charging stations that are integrated in e.g., parking infrastructure. | |
Summary
It is commonly regarded in industry that for large-scale battery energy storage systems, cell level energy density is not a key metric. Nonetheless, energy density is widely discussed as the major limitation of aqueous battery technologies such as flow batteries, and substantial research efforts are devoted to incrementally improving the energy density of systems inherently developed for stationary applications. Here, we systematically study and quantify the land area occupied by forty-four MWh-scale battery energy storage systems and show that the areal energy density of such installations is in many cases comparable between lithium-ion, sodium–sulfur, and flow batteries. Even though energy densities on cell level vary by an order of magnitude, installation footprint is largely unlinked from cell level energy density. We argue that safety and locality need to be considered more to unlock standalone BESS use cases beyond placing storage next to generation sites in rural areas. The fact that inherently non-flammable aqueous systems can be deployed in multi-purpose buildings and in densely populated areas unlocks added value of land and opens doors that remain closed to state-of-the-art lithium-ion batteries. Storage solutions that are cost-effective, scalable, recyclable, highly manufacturable, and have long cycle life and secure supply chains are needed sooner rather than later, and a technology-agnostic approach that exploits electrochemical, thermal, and mechanical solutions, without discarding any one candidate based on the single metric of energy density, is critical to reach Net Zero.
Data availability
The authors declare that all data supporting the findings of this study are included within the paper and its ESI† and are available from the corresponding authors on request.
Author contributions
D. R. conceptualization, methodology, investigation, data curation, visualization, writing – original draft, writing – review & editing, supervision. S. R. J. investigation, writing – review & editing. M. P. M. conceptualization, resources, funding acquisition, writing – review & editing.
Conflicts of interest
Prof. Marshak is the founder of a startup company, Otoro Energy, which is commercializing flow battery electrolyte materials.
Acknowledgements
The information, data, or work presented herein was funded by the National Science Foundation under Grant No. 2155227, and the DOE Office of Electricity Energy Storage Program at Pacific Northwest National Laboratory through subcontract 608616. We thank Dr Daniel Morton for preparing Fig. 4. The views and opinions of authors expressed herein do not necessarily state or reflect those of the United States Government or any agency thereof.
References
- N. M. Haegel,
et al., Photovoltaics at multi-terawatt scale: Waiting is not an option, Science, 2023, 380, 39–42 CrossRef CAS PubMed
.
- U.S. Office of Energy Efficiency & Renewable Energy. Confronting the Duck Curve: How to Address Over-Generation of Solar Energy. https://www.energy.gov/eere/articles/confronting-duck-curve-how-address-over-generation-solar-energy (2017).
- California ISO. California power grid price map. https://www.caiso.com/todaysoutlook/Pages/prices.html (2022).
- J. Seel, D. Millstein, A. Mills, M. Bolinger and R. Wiser, Plentiful electricity turns wholesale prices negative, Adv. Appl. Energy, 2021, 4, 100073 CrossRef
.
-
T. A. Nguyen and R. H. Byrne Evaluation of Energy Storage Providing Virtual Transmission Capacity. in 2021 IEEE Power & Energy Society General Meeting (PESGM)1–5 (IEEE, 2021).
- Fluence Energy GmbH. World's Largest Storage-as-Transmission Project Announced by Fluence and TransnetBW to Strengthen Energy Security and Renewable Integration in Germany. https://ir.fluenceenergy.com/news-releases/news-release-details/worlds-largest-storage-transmission-project-announced-fluence (2022).
- Transnet BW. Netzbooster Kupferzell. https://www.transnetbw.de/de/netzentwicklung/projekte/netzbooster-kupferzell (2022).
- California ISO Open Access Same-time Information System (OASIS). http://oasis.caiso.com/mrioasis/logon.do.
-
H. John & Texas Department of State Health Services. February 2021 Winter Storm-Related Deaths – Texas. https://www.dshs.texas.gov/news/updates/SMOC_FebWinterStorm_MortalitySurvReport_12-30-21.pdf (2021).
- City of Austin and Travis County. 2021 Winter weather storm Uri after-action review findings report. https://www.austintexas.gov/winter-storm-uri-after-action-resources (2021).
- The American Society of Civil Engineers Texas Section. Reliability and Resilience in the Balance. https://www.texasce.org/tce-news/reliability-and-resilience-in-the-balance/(2022).
- California ISO. California power grid supply data. http://www.caiso.com/TodaysOutlook/Pages/supply.html (2022).
- California ISO. Analysis of September heat wave. http://www.caiso.com/Documents/california-iso-posts-analysis-of-september-heat-wave.pdf (2022).
- Z. Zhu,
et al., Rechargeable Batteries for Grid Scale Energy Storage, Chem. Rev., 2022, 122, 16610–16751 CrossRef CAS PubMed
.
- S. Hameer and J. L. van Niekerk, A review of large-scale electrical energy storage, Int. J. Energy Res., 2015, 39, 1179–1195 CrossRef
.
- G. L. Soloveichik, Battery technologies for large-scale stationary energy storage, Annu. Rev. Chem. Biomol. Eng., 2011, 2, 503–527 CrossRef CAS PubMed
.
- G. L. Soloveichik, Flow batteries: current status and trends, Chem. Rev., 2015, 115, 11533–11558 CrossRef CAS PubMed
.
- A. Poullikkas, A comparative overview of large-scale battery systems for electricity storage, Renewable Sustainable Energy Rev., 2013, 27, 778–788 CrossRef
.
- X. Fan,
et al., Battery technologies for grid-level large-scale electrical energy storage, Trans. Tianjin Univ., 2020, 26, 92–103 CrossRef
.
- R. K. Emmett and M. E. Roberts, Recent developments in alternative aqueous redox flow batteries for grid-scale energy storage, J. Power Sources, 2021, 506, 230087 CrossRef CAS
.
- G. F. Frate, L. Ferrari and U. Desideri, Energy storage for grid-scale applications: Technology review and economic feasibility analysis, Renew. Energy, 2021, 163, 1754–1772 CrossRef
.
- Z. Huang,
et al., Comprehensive Analysis of Critical Issues in All-Vanadium Redox Flow Battery, ACS Sustainable Chem. Eng., 2022, 10(24), 7786–7810 CrossRef CAS
.
- H. Li, Practical evaluation of Li-ion batteries, Joule, 2019, 3, 911–914 CrossRef CAS
.
- M. Killer, M. Farrokhseresht and N. G. Paterakis, Implementation of large-scale Li-ion battery energy storage systems within the EMEA region, Appl. Energy, 2020, 260, 114166 CrossRef
.
- L. Zhang, R. Feng, W. Wang and G. Yu, Emerging chemistries and molecular designs for flow batteries, Nat. Rev. Chem., 2022, 6, 524–543 CrossRef PubMed
.
- Q. Huang and Q. Wang, Next-generation, high-energy-density redox flow batteries, ChemPlusChem, 2015, 80, 312–322 CrossRef CAS
.
- M. Park, J. Ryu, W. Wang and J. Cho, Material design and engineering of next-generation flow-battery technologies, Nat. Rev. Mater., 2016, 2, 1–18 Search PubMed
.
- Z. Li and Y. Lu, Material design of aqueous redox flow batteries: fundamental challenges and mitigation strategies, Adv. Mater., 2020, 32, 2002132 CrossRef CAS PubMed
.
- E. Sánchez-Díez,
et al., Redox flow batteries: Status and perspective towards sustainable stationary energy storage, J. Power Sources, 2021, 481, 228804 CrossRef
.
- Y. Yamada, J. Wang, S. Ko, E. Watanabe and A. Yamada, Advances and issues in developing salt-concentrated battery electrolytes, Nat. Energy, 2019, 4, 269–280 CrossRef CAS
.
- A. von Wald Cresce and K. Xu, Aqueous lithium-ion batteries, Carbon Energy, 2021, 3, 721–751 CrossRef CAS
.
- D. Chao and S.-Z. Qiao, Toward high-voltage aqueous batteries: super-or low-concentrated electrolyte?, Joule, 2020, 4, 1846–1851 CrossRef
.
- D. Chao,
et al., Roadmap for advanced aqueous batteries: From design of materials to applications, Sci. Adv., 2020, 6, eaba4098 CrossRef CAS PubMed
.
- J. O. G. Posada,
et al., Aqueous batteries as grid scale energy storage solutions, Renewable Sustainable Energy Rev., 2017, 68, 1174–1182 CrossRef CAS
.
- D. Larcher and J.-M. Tarascon, Towards greener and more sustainable batteries for electrical energy storage, Nat. Chem., 2015, 7, 19–29 CrossRef CAS PubMed
.
- H. Kim,
et al., Aqueous rechargeable Li and Na ion batteries, Chem. Rev., 2014, 114, 11788–11827 CrossRef CAS PubMed
.
- International Energy Agency. Grid Scale Storage Report. https://www.iea.org/reports/grid-scale-storage (2022).
-
V. Henze and BloombergNEF. Global Energy Storage Market Set to Hit One Terawatt-Hour by 2030. https://about.bnef.com/blog/global-energy-storage-market-set-to-hit-one-terawatt-hour-by-2030/(2021).
-
J. Luigi and Swissinfo. Inside Switzerland's giant water battery. https://www.swissinfo.ch/eng/sci-tech/inside-switzerland-s-giant-water-battery-46915530 (2021).
- Rho Motion. ESS – Global Grid Deployment Infographic, 2022. https://rhomotion.com/ess-global-grid-deployment-infographic-december-2022.
- Lazard Ltd. Lazard's Levelized Cost of Storage Analysis V 7.0.https://www.lazard.com/media/451882/lazards-levelized-cost-of-storage-version-70-vf.pdf (2022).
- Pacific Gas and Electric Company. PG&E Commissions Its Moss Landing Elkhorn Battery. https://www.pgecurrents.com/articles/3431-creating-clean-energy-future-pg-e-commissions-moss-landing-elkhorn-battery (2022).
- Chinese Academy of Sciences. World's Largest Flow Battery Energy Storage Station Connected to Grid. https://english.cas.cn/newsroom/research_news/chem/202205/t20220531_306054.shtml (2022).
- U.S. Energy Information Administration. Battery Storage in the United States: An Update on Market Trends. https://www.eia.gov/analysis/studies/electricity/batterystorage/pdf/battery_storage.pdf (2020).
- NGK Insulators Ltd. NaS solutions. https://www.ngk-insulators.com/en/product/nas-solutions.html (2022).
- U.S. Department of Energy. Energy Storage Grand Challenge: Energy Storage Market Report. https://www.energy.gov/sites/prod/files/2020/12/f81/EnergyStorageMarketReport2020_0.pdf (2020).
- BloombergNEF. Lithium-ion Battery Pack Prices Rise for First Time. https://about.bnef.com/blog/lithium-ion-battery-pack-prices-rise-for-first-time-to-an-average-of-151-kwh/.
- Tesla Inc. Lithium-Ion Battery Emergency Response Guide. https://www.tesla.com/sites/default/files/downloads/Lithium-Ion_Battery_Emergency_Response_Guide_en.pdf (2022).
- P. A. Christensen,
et al., Risk management over the life cycle of lithium-ion batteries in electric vehicles, Renewable Sustainable Energy Rev., 2021, 148, 111240 CrossRef
.
- National Fire Protection Association. NFPA 855 Standard for the Installation of Stationary Energy Storage Systems. https://www.nfpa.org/codes-and-standards/all-codes-and-standards/list-of-codes-and-standards/detail?code=855 (2023).
- UL Solutions. UL 1973 Batteries for Use in Stationary and Motive Auxiliary Power Applications. https://www.shopulstandards.com/ProductDetail.aspx?UniqueKey=42361(2022).
- UL Solutions. UL 9540 Energy Storage System (ESS) Requirements. https://www.ul.com/news/ul-9540-energy-storage-system-ess-requirements-evolving-meet-industry-and-regulatory-needs (2020).
- UL Solutions. UL 9540A Test Method. https://www.ul.com/services/ul-9540a-test-method (2022).
- Monterey County Office of Emergency Services. 2022 Moss Landing Incident. https://www.co.monterey.ca.us/government/departments-a-h/administrative-office/office-of-emergency-services/incidents/power-storage-facility-incident?fbclid=IwAR0FEYNK5oVCNidbUpirRbHIxH4-HE8k34pcx-bNyF8xCU-pzlEtQRwvt4g#!/ (2022).
- Tesla Inc. Tesla Megapack. https://www.tesla.com/megapack (2022).
- Tesla Inc. Tesla Q3 2022 Financial Statement. https://ir.tesla.com/#quarterly-disclosure (2022).
- Vistra Corp. Findings and Corrective Actions - Moss Landing Phase I. https://vistra.app.box.com/s/1aezfypko93vz5jm9nn9tckphtjkgzvb (2021).
- Fisher Engineering Inc. Victorian Big Battery Fire. https://victorianbigbattery.com.au/wp-content/uploads/2022/01/VBB-Fire-Independent-Report-of-Technical-Findings.pdf (2022).
- Electric Power Research Institute. EPRI BESS Failure Event Database. https://storagewiki.epri.com/index.php/BESS_Failure_Event_Database?oldid=1260.
- G. Marlair, A. Lecocq, A. Bordes, P. Christensen and B. Truchot, Key Learnings From Recent Lithium-ion Battery Incidents that have impacted e-mobility and Energy Storage Fast Growing Markets, Chem. Eng. Trans., 2022, 90, 643–648 Search PubMed
.
- N. A. Sepulveda, J. D. Jenkins, A. Edington, D. S. Mallapragada and R. K. Lester, The design space for long-duration energy storage in decarbonized power systems, Nat. Energy, 2021, 6, 506–516 CrossRef
.
- J. A. Dowling,
et al., Role of long-duration energy storage in variable renewable electricity systems, Joule, 2020, 4, 1907–1928 CrossRef
.
- LDES Council & McKinsey & Company. Flagship 2021 Net-zero power report: Long duration energy storage for a renewable grid. http://www.ldescouncil.com/assets/pdf/LDES-brochure-F3-HighRes.pdf (2021).
- Office of Energy Efficiency & Renewable Energy. Long Duration Storage Shot. https://www.energy.gov/eere/long-duration-storage-shot (2021).
- P. Albertus, J. S. Manser and S. Litzelman, Long-duration electricity storage applications, economics, and technologies, Joule, 2020, 4, 21–32 CrossRef CAS
.
- California ISO. California power grid oversupply and curtailments. http://www.caiso.com/informed/Pages/ManagingOversupply.aspx (2022).
- U.S. Energy Information Administration. How much electricity does an American home use? https://www.eia.gov/tools/faqs/faq.php?id=97&t=3 (2022).
-
B. Scott, H. Jason, S. Andea and W. Rachel and Form Energy. Long duration and multi-day storage tech will allow California to put surplus renewable energy to good use. https://www.energy-storage.news/long-duration-and-multi-day-storage-tech-allow-california-to-put-surplus-renewable-energy-to-good-use/ (2022).
- Energy and Environmental Economics Inc. The Potential for Energy Storage to Repower or Replace Peaking Units in New York State. https://www.ethree.com/wp-content/uploads/2019/08/E3_The_Potential_for_Energy_Storage_to_Repower_or_Replace_Peaking_Units_in_New_York_State_July_2019.pdf (2019).
-
R. Isabella and B. Scott & Form Energy. Solving the Clean Energy and Climate Justice Puzzle. https://formenergy.com/wp-content/uploads/2020/08/Form_Energy_NYGasReplaceWhitePaper_V2.pdf (2020).
-
Liz Gill, Aleecia Gutierrez and Terra Weeks, C. E. C. Senate Bill 100 Joint Agency Report, Achieving 100 Percent Clean Electricity in California: An Initial Assessment. https://www.energy.ca.gov/publications/2021/2021-sb-100-joint-agency-report-achieving-100-percent-clean-electricity (2021).
- Q. Xu, T. S. Zhao and C. Zhang, Effects of SOC-dependent electrolyte viscosity on performance of vanadium redox flow batteries, Appl. Energy, 2014, 130, 139–147 CrossRef CAS
.
- D. Reber,
et al., Mediating anion-cation interactions to improve aqueous flow battery electrolytes, Appl. Mater. Today, 2022, 28, 101512 CrossRef
.
- D. Reber, J. R. Thurston, M. Becker and M. P. Marshak, Stability of highly soluble ferrocyanides at neutral pH for energy-dense flow batteries, Cell Rep. Phys. Sci., 2023, 4, 101215 CrossRef CAS
.
- E. S. Beh,
et al., A neutral pH aqueous organic–organometallic redox flow battery with extremely high capacity retention, ACS Energy Lett., 2017, 2, 639–644 CrossRef CAS
.
- J. Luo, B. Hu, C. Debruler and T. L. Liu, A π-conjugation extended viologen as a two-electron storage anolyte for total organic aqueous redox flow batteries, Angew. Chem., Int. Ed., 2018, 57, 231–235 CrossRef CAS PubMed
.
- W. Liu,
et al., A highly stable neutral viologen/bromine aqueous flow battery with high energy and power density, Chem. Commun., 2019, 55, 4801–4804 RSC
.
- Viva Energy Australia. Viva Energy opens Australia's largest crude oil tank at the Geelong Refinery. https://www.vivaenergy.com.au/media/news/2017/viva-energy-opens-australias-largest-crude-oil-tank-at-the-geelong-refinery (2017).
-
J. Christian and A. B. Vatenfall Germany's largest heat storage in the starting blocks. https://group.vattenfall.com/press-and-media/newsroom/2022/germanys-largest-heat-storage-in-the-starting-blocks (2022).
- StatenIslander.org. Legislation to prohibit the installation of lithium battery energy storage systems (BESS) in residential neighborhoods and school zones. https://statenislander.org/2023/02/17/asm-pirozzolo-sen-lanza-introduce-legislation-to-prohibit-installation-of-lithium-battery-energy-storage-systems-bess-in-residential-neighborhoods-schools-zones/?utm_source=rss&utm_medium=rss&utm_campaign=asm-pirozzol (2023).
Footnote |
† Electronic supplementary information (ESI) available: The Methods, figures M1–M3, supplementary discussions, descriptions of battery energy storage systems, Fig. S1–S75 and Tables S1–S4. A Google Earth Pro file with pinned locations for all battery energy storage systems and pumped storage hydropower sites discussed here is included. See DOI: https://doi.org/10.1039/d3ya00208j |
|
This journal is © The Royal Society of Chemistry 2023 |