DOI:
10.1039/D2TC05502C
(Paper)
J. Mater. Chem. C, 2023,
11, 3375-3385
Near-infrared Sr7NaGa(PO4)6:Cr3+,Ln3+ (Ln = Nd, Er, and Yb) phosphors with different energy transfer paths: photoluminescence enhancement and versatility†
Received
26th December 2022
, Accepted 7th February 2023
First published on 9th February 2023
Abstract
Near-infrared phosphors are of great interest due to their unique, versatile luminescence properties and exciting application prospects. Herein, a novel broadband emission NIR phosphor Sr7NaGa(PO4)6:Cr3+ was synthesized successfully, and photoluminescence properties of the phosphor were studied. When Ln3+ ions were co-doped, the photoluminescence was enhanced. Compared with Sr7NaGa(PO4)6:0.15Cr3+, the total emission intensity of Sr7NaGa(PO4)6:0.15Cr3+,0.15Yb3+ increased to 172%, the IQE of Sr7NaGa(PO4)6:0.15Cr3+,0.15Nd3+ increased from 22 to 72%, and the thermal quenching of Sr7NaGa(PO4)6:0.15Cr3+,0.50Er3+ suppressed effectively. Highly efficient energy transfer paths are formed in the Sr7NaGa(PO4)6:0.15Cr3+,Ln3+ phosphors because of the shorter distances between Cr3+ ions and Ln3+ ions in the Sr7NaGa(PO4)6 host. The energy transfer efficiencies of Cr3+ → Ln3+ are greater than 70%. Finally, the investigations on night vision, solar cells, and ratiometric luminescent thermometers demonstrate that the as-prepared NIR phosphors have the potential for multifunctional applications.
1. Introduction
Near-infrared (NIR) phosphors with emission in the range of 700–1700 nm have drawn widespread attention because of their unique, versatile luminescent properties and exciting application prospects.1–4 NIR phosphor-converted LEDs (NIR pc-LEDs) are expected to be the next generation NIR light source due to their small size, high conversion efficiency, long lifetime, and environmental friendliness.5,6 The NIR pc-LEDs with broadband NIR emission can be employed in some areas such as non-destructive analysis and night vision.7,8 And the NIR pc-LEDs in special ranges such as 720–740 nm and 650–950 nm (first biological window) can be used to facilitate plant growth, distinguish tumor tissues, and identify blood oxygen levels.9–11 In addition to NIR pc-LEDs, NIR phosphors are also used as luminescent thermometers or light-conversion materials. The luminescent thermometers prepared using NIR phosphors at first and second (1000–1700 nm) biological windows pave the way for photodynamic thermal therapy for cancer.12–15 Some NIR phosphors converting sunlight into NIR light at 900–1100 nm are added to c-Si solar cells to improve the solar energy conversion efficiency of c-Si solar cells.16–18 These application prospects provide a huge impetus to develop novel highly efficient multifunctional NIR phosphors.
Inorganic NIR phosphors are composed of host materials and active ions. The active ions for NIR emission comprise rare earth ions and transition metal ions, such as Nd3+, Yb3+, Er3+, and Cr3+.4,19–22 The Nd3+ and Yb3+ ions emit NIR light around 1000 nm corresponding to the 4F3/2–4I9/2 and 4F3/2–4I11/2 transitions of Nd3+ ions and the 2F5/2–2F7/2 transitions of Yb3+ ions.23,24 They show only a small amount of narrow-band absorption in visible light attributed to their spin-forbidden f–f transitions. In contrast, Cr3+ doped NIR phosphors show broadband absorption in visible light due to the spin-allowed d–d transition of the Cr3+ ion and exhibit good thermal stability and high quantum efficiency.25 However, the 3d electrons of Cr3+ ions tend to couple to host lattices leading to a non-radiative transition, which has a negative effect on the luminescence properties of Cr3+ doped NIR phosphors.26–28
Ln3+ and Cr3+ ion co-doped NIR phosphors can combine the advantages of both the ions to reduce non-radiative transition, improve the performance of NIR phosphors and increase their application potential. At present, some NIR phosphors have been reported such as Ca3Sc2Si3O12:Cr3+, LiGaP2O7:Cr3+, Ca2LuScAl2Si2O12:Cr3+, LaMgAl11O19:Cr3+,Nd3+/Yb3+, Ca3Ga2Ge3O12:Cr3+,Nd3+, Ba2LaGa11O20:Cr3+,/Nd3+/Yb3+, LaGaO3:Cr3+,Yb3+/Nd3+/Er3+, La3LuGa4O12:Cr3+,Nd3+, and LiScP2O7:Cr3+,Yb3+.12,16,29–35 The Sr9Ga(PO4)7:Cr3+ and Sr9Cr(PO4)7:Yb3+ phosphors have been reported by Liu's work;36,37 the work reported that the structure confinement of Sr9M(PO4)7 (M = Ga and Cr) can enhance the luminescence properties of the NIR phosphors. In the Sr9Cr(PO4)7:Yb3+ phosphors, the energy transfer between Cr3+ and Yb3+ has been studied in detail. However, the energy transfer between Cr3+ and other rare earth ions such as Nd3+ and Er3+ has not been studied in this system, and the effect of the structure on different energy transfer paths and the application of phosphors co-doped with different rare earth ions need to be further investigated.
In this work, we report a novel NIR phosphor Sr7NaGa(PO4)6:Cr3+ (SNGP:Cr3+) with a broadband emission (λmax = 840 nm, FWHM = 140 nm) that can be effectively excited by the light from the commercial blue chip. More importantly, the PL properties including total emission intensity, internal quantum efficiency (IQE), and thermal stability of the SNGP:Cr3+ phosphors can be enhanced by co-doping Ln3+ (Yb3+, Nd3+, and Er3+). The energy transfers from the Cr3+ to Ln3+ ions were studied in detail through analyzing the structure and radiation and non-radiation rates of Cr3+ ions, and decay rates of Ln3+ ions in the phosphors. We observed that some absorption energy of the Cr3+ ions is transferred to the Ln3+ ions, and the high energy transfer efficiencies of Cr3+ → Ln3+ (>70%) are attributed to the shorter distance between the Cr3+ ions and Ln3+ ions in the SNGP: Cr3+,Ln3+ phosphors. Furthermore, we explored the potential of the SNGP:Cr3+,Ln3+ phosphors for multifunctional applications.
2. Experimental section
2.1. Synthesis
Sr7NaGa(PO4)6:Cr3+,Yb3+/Nd3+/Er3+ NIR phosphors were synthesized using a traditional high temperature solid-state reaction. The raw materials, SrCO3 (AR), Na2CO3 (AR), Ga2O3 (99.99%), NH4H2PO4 (AR), Cr2O3 (AR), Yb2O3 (99.99%), Nd2O3 (99.99%), and Er2O3 (99.99%) were weighted according to stoichiometric amounts and grounded in an agate mortar for 20 min. Then, the mixtures were transferred to alumina crucibles and sintered at 1100 °C for 4 h in air. The final products were cooled to room temperature and reground to obtain homogeneous powders for analysis.
2.2. Characterization
X-ray diffraction (XRD) of the prepared samples was executed on a Bruker AXs D8 diffractometer with Cu Kα X-radiation at 40 kV, 40 mA and λ = 0.15405 nm. Energy-dispersive X-ray spectroscopy (EDS) elemental mappings were carried out using a Hitachi-S4800 system (Japan). The photoluminescence (PL) and photoluminescence excitation (PLE) spectra were recorded using an Edinburgh FLSP-920 fluorescence spectrophotometer equipped with a 450 W Xe lamp. The decay curves were collected using the same spectrophotometer with a μF900 flash lamp. A series of temperature dependent PL spectra from 298 to 423 K were recorded on a FLSP-920 system with a temperature controller.
2.3. NIR pc-LED fabrication and performance measurement
The as-prepared NIR phosphors were first mixed with epoxy resins and then coated on 460 nm chips. The mixtures were cured at 120 °C for 1 h to form the final LED devices. The electroluminescence performance was measured using a HAAS 2000 photoelectric measuring system from EVERFINE.
3. Results and discussion
3.1. Phase and structural analysis
Fig. 1a depicts the crystal structure of SNGP, and there are four different Sr sites, Sr1, Sr2, Sr3, and Sr4, in the structure. The Sr1, Sr2, Sr3, and Sr4 sites are surrounded by nine, eight, and seven oxygen atoms forming polyhedra, respectively. Ga connects six oxygen atoms forming [GaO6] octahedra. Due to the similar radii and charges of Cr3+ ions (r = 0.615 Å, CN = 6) and Ga3+ ions (r = 0.620 Å, CN = 6), Cr3+ ions prefer to substitute Ga3+ ions. While Ln3+ ions tend to replace Sr2+ ions because the radius of Ln3+ ions is much larger than that of Ga3+ ions but similar to that of Sr2+ ions. Notably, the distances between adjacent Ga3+ ions are large ranging from 8.984 to 17.968 Å, and the distances between Ga3+ ions and Sr2+ ions are short ranging from 3.687 to 5.314 Å. The XRD patterns of SNGP:xCr3+ (x = 0.10, 0.15, 0.30, 0.50, 0.70, and 0.90) were obtained to verify the phase purity. Fig. 1b shows that all diffraction peaks of samples match well with the standard card of Sr9Ga(PO4)7 (PDF # 53-0180). The result indicates that the pure phase samples have been prepared successfully. With an increase in Cr3+-concentration, the diffraction peaks of SNGP:xCr3+ shift to a higher angle region (Fig. 1b), which is caused by lattice expansion due to the large Ga3+ ions being replaced with smaller Cr3+ ions. The result means that smaller Cr3+ ions dissolve into the SNGP host and reduce the lattice space. Fig. S1 (ESI†) displays the XRD patterns of SNGP:Cr3+,Ln3+ phosphors, and no other peaks except pure phase peaks are observed.
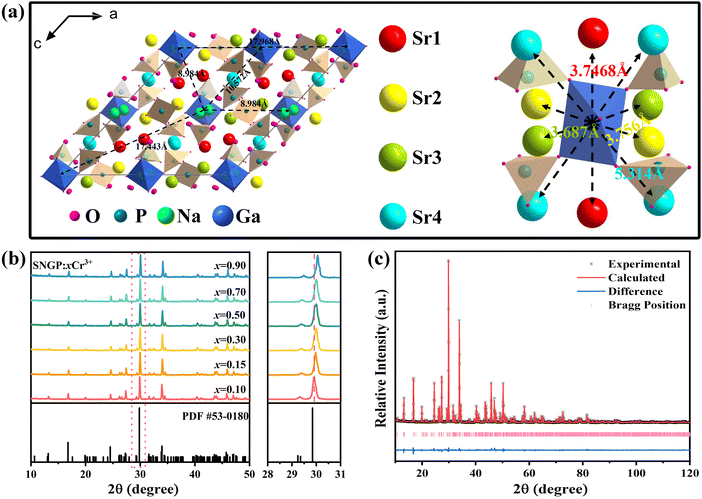 |
| Fig. 1 (a) Crystal structure of the SNGP host; distances between adjacent Ga3+ ions, and distances between Ga3+ ions and Sr2+ ions. (b) XRD patterns of SNGP:xCr3+ (x = 0.10, 0.15, 0.30, 0.50, 0.70, and 0.90) (c) Rietveld refinement of SNGP:0.15Cr3+ phosphors. | |
Fig. 1c illustrates the calculated and experimental diffractograms of SNGP:0.15Cr3+ phosphors obtained from XRD Rietveld refinement. Table 1 represents mainly the results of the refined crystal structure, where the lower R factor of the sample ensures the accuracy of the refinement. These results confirm that SNGP:Cr3+ possesses a monoclinic structure with space group I2/a1. Moreover, Fig. 2 shows the elemental mapping images of SNGP:0.30Cr3+, where the constituent elements Sr, Na, Ga, P, O, and Cr are well distributed within the hole particle, meaning that Cr3+ ions have been successfully introduced into the host.
Table 1 Refined crystal structure results of the SNGP:0.15Cr3+ phosphor
Compound |
SNGP:0.15Cr3+ |
Space group |
I2/a1 |
a/Å |
17.96 |
b/Å |
10.56 |
c/Å |
18.30 |
v/Å3 |
3102.8 |
α = β/deg |
90 |
γ/deg |
133 |
Z
|
4 |
2θ/deg |
10–120 |
R
wp, % |
0.0849 |
R
p, % |
0.0594 |
χ
2
|
6.7 |
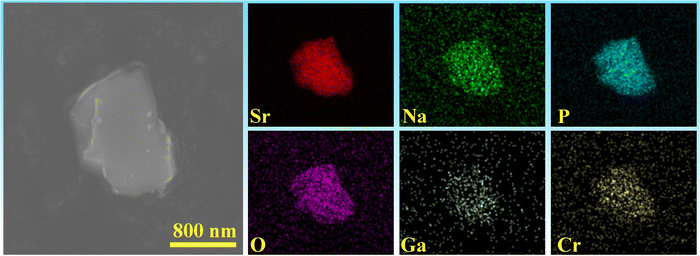 |
| Fig. 2 SEM image and elemental maps of the SNGP:0.30Cr3+ phosphor. | |
3.2. Luminescence properties of SNGP:Cr3+ phosphors
Fig. 3a illustrates the PL and PLE spectra of SNGP:0.15Cr3+. The PLE spectrum monitored at 840 nm exhibits two absorption bands centered at 487 and 704 nm, originating from the 4A2g → 4T1g and 4A2g → 4T2g transitions of Cr3+ ions. The effective excitation band is close to 460 nm, matching well with the emission of the commercial blue chip, and has application prospects in NIR pc-LED production. The PL spectrum shows a broadband emission peaking at 840 nm and a full width at half maximum (FWHM) of 140 nm (Fig. 3a). There is a strong relationship between crystal field strength of the host and Cr3+ emission. In general, when Dq/B is greater than 2.3, the host is considered to have a strong crystal field, and Cr3+ ions show a narrow-band emission around 700 nm; when Dq/B is less than 2.3, the host has a weak crystal field, and Cr3+ ions produce a broadband emission. The crystal field strength of SNGP:0.15Cr3+ was calculated using the following equations:11 | 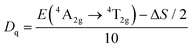 | (1) |
| 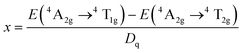 | (2) |
| 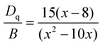 | (3) |
where E(4A2g–4T2g) and E(4A2g–4T1g) relate to the transition energy of 4A2g–4T2g and 4A2g–4T1g corresponding to the energy position of the excitation band, and ΔS is the Stokes shift. The Dq/B value of sample was determined to be 1.93, revealing that the Cr3+ ions are located in a weak crystal field environment. Fig. 3b depicts the Tanabe–Sugano diagram for the Cr3+ ions in an octahedral crystal field environment, where the 4A2g → 4T2g transition is the dominant transition as Dq/B is less than 2.3. It proves that the broadband emission of samples originates from the 4A2g → 4T2g transition in a weak crystal field environment. Fig. 3c plots the PL spectra of SNGP:xCr3+ (x = 0.10, 0.15, 0.30, 0.50, 0.70, and 0.90). As Cr3+-concentration increases, the emission intensity of samples first increases and then decreases, and the emission intensity is maximum at x = 0.15. The phenomenon is concentration quenching caused by energy transfer between adjacent Cr3+ ions. From the structural analysis above, the distances (8.984 to 17.968 Å) between adjacent Cr3+ ions exceed the exchange interaction distance (∼5 Å) because of Ga3+ ions being replaced with Cr3+. Thus, the energy transfer mechanism between adjacent Cr3+ ions in the host is electric multipolar interaction rather than exchange interaction. The concentration quenching was further studied through the relationship between concentration and intensity of SNGP:xCr3+ (Note S1, ESI†) and we found that the multipolar interaction belongs to dipole–dipole (d–d) interactions. As the Cr3+-concentration increases, the multipolar interaction strengthens, leading to a nonradiative transition enhancement and concentration quenching. Concentration-induced nonradiative transition can be demonstrated using decay curves.
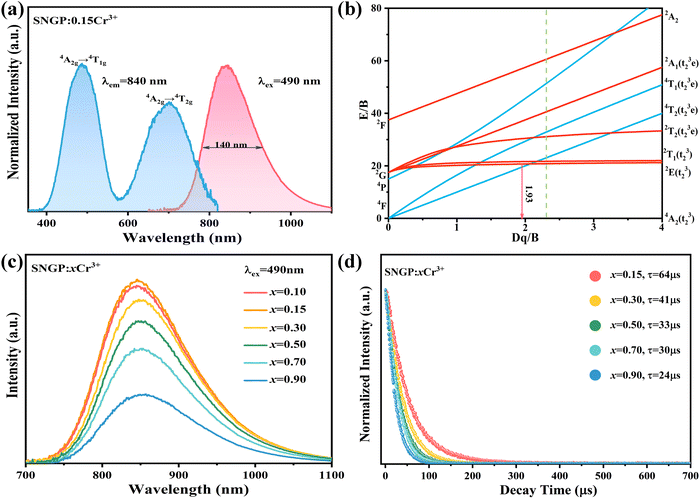 |
| Fig. 3 (a) PLE and PL spectra of SNGP:0.15Cr3+. (b) Tanabe–Sugano diagram for Cr3+ in an octahedral crystal field environment. (c) Relationship between concentration and emission intensity of the Cr3+ ions in SNGP:xCr3+ (x = 0.10, 0.15, 0.30, 0.50, 0.70, and 0.90). (d) Decay curves of SNGP:xCr3+ (x = 0.15, 0.30, 0.50, 0.70, and 0.90). | |
As shown in Fig. 3d, all decay curves are fitted with a single exponential function, meaning that there is only one site for Cr3+ to occupy in the host. The lifetimes of samples decrease with an increase in Cr3+-concentration, which can be explained using the formula given below:38,39
| 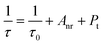 | (4) |
where
τ0 stands for the radiative lifetime,
Anr and
Pt represents the nonradiative rate depending on multiphonon relaxation and the energy transfer rate. On the basis of the equation, we know that the nonradiative rate increases with an increase in Cr
3+-concentration, leading to an attenuation of the lifetimes. The result demonstrates that concentration induces a nonradiative transition that not only has a negative effect on the luminescence intensity, but also reduces the thermal stability and quantum efficiency of phosphors. In addition to concentration variation, the nonradiative transition can be caused by other factors such as temperature and defects. The PL spectra of SNGP:0.15Cr
3+ (phosphor
1) at different temperatures (Fig. S2, ESI
†) and IQE of the phosphor were measured. The thermal stability of phosphor
1 is poor and the IQE is only about 22%. Therefore, it is necessary that the PL properties of SNGP:Cr
3+ are further improved by inhibiting the nonradiative transition of the phosphor.
3.3. PL enhancement through the energy transfer paths of SNGP:Cr3+,Ln3+
Fig. 4a, c and e displays the PL spectra of SNGP:0.15Cr3+,yYb3+ (y = 0, 0.10, 0.15, 0.20, and 0.25), SNGP:0.15Cr3+,mNd3+ (m = 0.01, 0.03, 0.05, 0.10, 0.15, 0.20, 0.25, and 0.30), and SNGP:0.15Cr3+,nEr3+ (n = 0, 0.05, 0.1, 0.3, 0.5, and 0.7), respectively. The spectra of SNGP:0.15Cr3+,yYb3+ (y = 0.10, 0.15, 0.20, and 0.25) ranging from 700 to 1100 nm show the characteristic emission of both Cr3+ ions and Yb3+ ions. With an increase in Yb3+-concentration, the emission intensity of the Cr3+ ions decreases, while that of Yb3+ ions increases and reaches the maximum when y = 0.15. The result indicates that the energy of some Cr3+ ions is transferred to Yb3+ ions in SNGP:0.15Cr3+,yYb3+. The total emission intensity of SNGP:0.15Cr3+,0.15Yb3+ (phosphor 2) increases to 172% (Fig. 4b), compared with that of phosphor 1. Similarly, the spectra of SNGP:0.15Cr3+,mNd3+ show the emission of Cr3+ ions and Nd3+ ions, and the emission intensity of the Nd3+ ions reaches the maximum with m = 0.10. In addition, the emission spectrum of the Nd3+ ions is composed of two peaks at 877 (P1) and 1060 nm (P2), corresponding to the 4F3/2 → 4I9/2 and 4F3/2 → 4I11/2 transitions of the Nd3+ ions, respectively, and a regular variation in the relative intensities of two peaks depends on Nd3+-concentration. As depicted in Fig. 4d, the emission intensity of P1 is higher than that of P2, when m is less than 0.05, while the emission intensity of P2 is higher than that of P1 when m exceeds 0.05. The interesting phenomenon can be explained by emission spectral overlap of the Cr3+ ions and the Nd3+ ions. Initially, the emission intensity of P1 is higher than that of P2 because the emission intensity of Cr3+ is high. However, with increasing Nd3+-concentration, the emission intensity of Cr3+ ions decreases because of the energy transfer from Cr3+ to Nd3+ ions. As a result, the intensity of P1 gradually decreases to less than the intensity of P2. As shown in Fig. S3 (ESI†), the total emission intensity of SNGP:0.15Cr3+,0.1Nd3+ (phosphor 3) increases to 113%. For SNGP:0.15Cr3+,nEr3+, the energy transfer from Cr3+ to Er3+ ions is observed in Fig. 4e, and the total emission intensity of SNGP:0.15Cr3+,0.5Er3+ (phosphor 4) increases to 156% in comparison with phosphor 1 (Fig. 4f). These results reveal that the enhancement of total emission intensity is caused by Cr3+ → Ln3+ energy transfer. The energy transfer mechanisms are revealed by the energy transfer scheme given in Fig. 5. The electrons of the Cr3+ ions are pumped from the ground state 4A2g to excited states 4T1g, and 4T2g after absorbing 490 and 704 nm light, respectively. Then the electrons relax to the 4T2g state and transfer some energy to the Yb3+ ions/ Nd3+ ions/ Er3+ ions, leading to the pumping of electrons of Yb3+ ions/the Nd3+ ions/the Er3+ ions from the ground state to the 2F5/2 state of Yb3+ ions/the 4F3/2 state of the Nd3+ ions/the 4I11/2 state of the Er3+ ions. The electrons in the excited state of the Ln3+ ions come to the lower excited state via nonradiative transition, and finally return to the ground state to produce NIR emission.
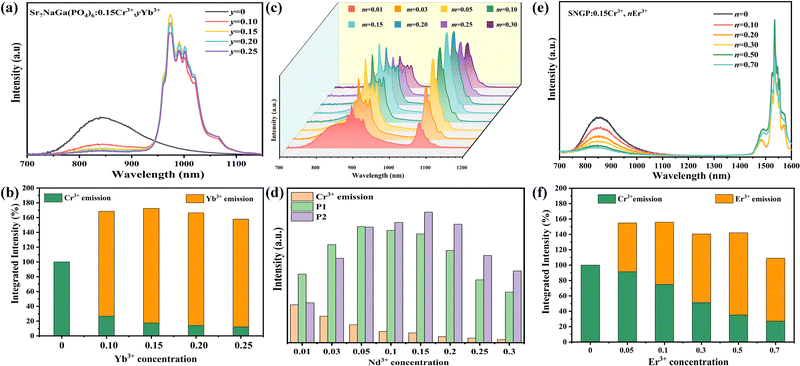 |
| Fig. 4 (a) PL spectra of SNGP:0.15Cr3+,yYb3+ (y = 0, 0.10, 0.15, 0.20, and 0.25) (b) integrated intensities of the total emission, Cr3+-emission, and Yb3+-emission in SNGP:0.15Cr3+,yYb3+ phosphors with various Yb3+-concentrations. The total emission is the sum of the Cr3+-emission and Yb3+-emission. (c) PL spectra of SNGP:0.15Cr3+,mNd3+ (m = 0.01, 0.03, 0.05, 0.10, 0.15, 0.20, 0.25, and 0.30). (d) Relative intensities of P1, P2 and Cr3+-emission in SNGP:0.15Cr3+,mNd3+ phosphors. (e) PL spectra of SNGP:0.15Cr3+,nEr3+ (n = 0, 0.05, 0.10, 0.30, 0.50, and 0.70). (f) Integrated intensities of the total emission, Cr3+-emission, and Er3+-emission in SNGP:0.15Cr3+,nEr3+ phosphors with various Er3+-concentrations. | |
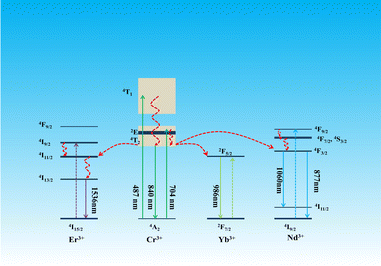 |
| Fig. 5 Energy transfer mechanisms of Cr3+ → Ln3+ in the SNGP:0.15Cr3+,Ln3+ phosphors. | |
The decay curves of the Cr3+ ions in SNGP:0.15Cr3+,Ln3+ (λex = 490 nm, λem = 840 nm) with different Ln3+-concentrations are plotted in Fig. 6a and Fig. S4a, b (ESI†). Due to Cr3+ → Ln3+ energy transfer, the lifetimes of Cr3+ in SNGP:Cr3+,Ln3+ decrease rapidly and the decay curves deviate from the single exponential rule. To better understand the energy transfer from the Cr3+ to Ln3+ ions, the average lifetime can be calculated using the equation as follows:21,40
|  | (5) |
The lifetimes of the Cr
3+ ions in SNGP:0.15Cr
3+,Ln
3+decrease continuously with increasing Ln
3+-concentration, confirming that the energy is transferred from Cr
3+ to Ln
3+ ions. The energy transfer efficiency (
η) was calculated using the following equation:
41 |  | (6) |
where
τ and
τ0 are the lifetimes of Cr
3+ ions in the presence and absence of Ln
3+ ions, respectively. As shown in
Fig. 6b and Fig. S4c, d (ESI
†), the
η value is determined to be 72%, 73% and 75% corresponding to SNGP:0.15Cr
3+,0.30Nd
3+, SNGP:0.15Cr
3+,0.70Er
3+ and SNGP:0.15Cr
3+,0.25Yb
3+, respectively. The high energy transfer efficiencies of Cr
3+ → Ln
3+ are attributed to the structure of SNGP:0.15Cr
3+,Ln
3+. On the basis of the structural analysis above, the distances between the Cr
3+ ions and Ln
3+ ions are much shorter than those between adjacent Cr
3+ ions because Ln
3+ ions replace Sr
2+ ions in the SNGP host. As a result, a compact Cr
3+–Ln
3+ pair is formed in the host and a highly efficient Cr
3+ → Ln
3+ energy transfer is realized. Nd
3+ usually connects eight or nine oxygen atoms, while Er
3+ and Yb
3+ can connect seven, eight or nine oxygen atoms. Thus, Nd
3+ can substitute Sr1 and Sr2 in SNGP:Cr
3+,Nd
3+, but Er
3+ and Yb
3+ can substitute Sr1, Sr2, Sr3 and Sr4 in SNGP:Cr
3+,Er
3+ and SNGP:Cr
3+,Yb
3+, respectively. According to the Dexter theory, the shorter the distance between the sensitizer and activator, the higher the energy transfer efficiency. The refined distances between Cr
3+ and Ln
3+ corresponding to SNGP:0.15Cr
3+,0.30Nd
3+, SNGP:0.15Cr
3+,0.70Er
3+ and SNGP:0.15Cr
3+,0.25Yb
3+ are listed in Table S1 (ESI
†). The average refined distance of Yb and Cr is shorter than that of the other two. The result means that the Cr
3+ → Yb
3+ energy transfer is the most efficient in SNGP:0.15Cr
3+,Ln
3+, which is consistent with the above experimental result.
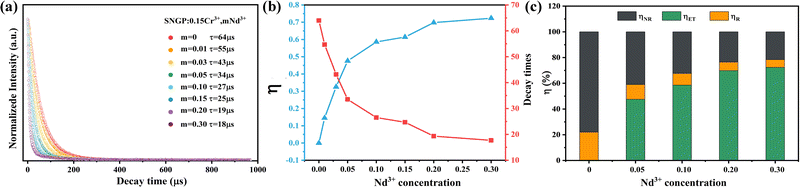 |
| Fig. 6 (a) Decay curves of Cr3+ ions in SNGP:0.15Cr3+,mNd3+ (m = 0, 0.01, 0.03, 0.05, 0.10, 0.15, 0.20, and 0.30). (b) Energy transfer efficiency (η) of Cr → Nd3+ and lifetimes of Cr3+ in the SNGP:0.15Cr3+,mNd3+ phosphors at different Nd3+ concentrations. (c) Values of ηET, ηR, and ηNR at various Nd3+ concentrations. | |
Moreover, the Cr3+ → Ln3+ energy transfer paths were analyzed in detail through the ET efficiency (η), radiative (WR) rates, and nonradiative (WNR) transition rates, which can be expressed using the following eqn (7)–(12):30
| 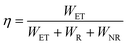 | (7) |
| 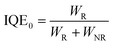 | (8) |
| 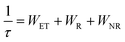 | (9) |
|  | (10) |
According to
eqn (6)–(10), the
WR and
WNR are defined as
| 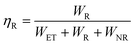 | (11) |
| 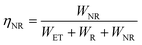 | (12) |
The
η,
ηR, and
ηNR values were calculated using
eqn (6)–(12). The values are plotted in
Fig. 6c and Fig. S4e, f (ESI
†), and the ratio of radiative and nonradiative transition rates reduces as Ln
3+-concentration increases. When Ln
3+ ions are co-doped, part of absorption energy for both radiative and nonradiative transitions of Cr
3+ ions is transferred to the Ln
3+ ions, which means that the nonradiative transition of the Cr
3+ ions is suppressed. As a result, the IQE and thermal stability of phosphor
1 are enhanced by co-doping Ln
3+ ions. The IQEs of phosphors
2–4 were measured to be 70.6, 72.8, and 49.3%, respectively (Note S2, ESI
†).
The PL spectra of phosphors 2–4 at different temperatures from 298 to 423 K are shown in Fig. S5a, b and Fig. 7a (ESI†), respectively. The relationships between the emission intensities of phosphors 2–4 and temperatures are plotted in Fig. 7b. Obviously, the thermal quenching is evidently reduced by co-doping Er3+ and Yb3+ ions. For instance, ∼56 and 75% of the original integral intensity can be retained at 100 °C for phosphors 2 and 3, respectively (∼16%@100 °C for SNGP:0.15Cr3+). However, there is basically no change in thermal stability of phosphor 3, compared with phosphors 1. According to a previous report, Yb3+ and Er3+ have the ability to replace a small amount of Ga3+, while the larger Nd3+ ions can only occupy Sr2+ sites.42 Thus, SNGP:Cr3+,Nd3+ phosphors have far more charge defects than SNGP:Cr3+,Yb3+ and SNGP:Cr3+,Er3+ phosphors. Although the IQE of phosphor 3 is the highest, the phosphor shows more non-radiative transitions due to the charge defects. Therefore, there is no significant improvement in thermal stability of phosphor 3. The non-radiative transitions of phosphors 2–4 were studied using temperature-dependent decay curves (Fig. S5c, d and Fig. 7c, ESI†). The lifetimes of the Ln3+ ions decrease as the temperature increases, and their decay rates (1/τ) are plotted in Fig. 7d as a function of temperature. All the decay rates of Ln3+ ions increase with increasing temperature because the rate of thermally activated nonradiative transition increases, and the decay rate of Nd3+ is highest and that of Er3+ is the lowest. Compared with phosphor 3, the nonradiative transitions of phosphors 2 and 4 are better suppressed to enhance the thermal stability of phosphor 1, promoting its application potential that will be explored in detail in the next section.
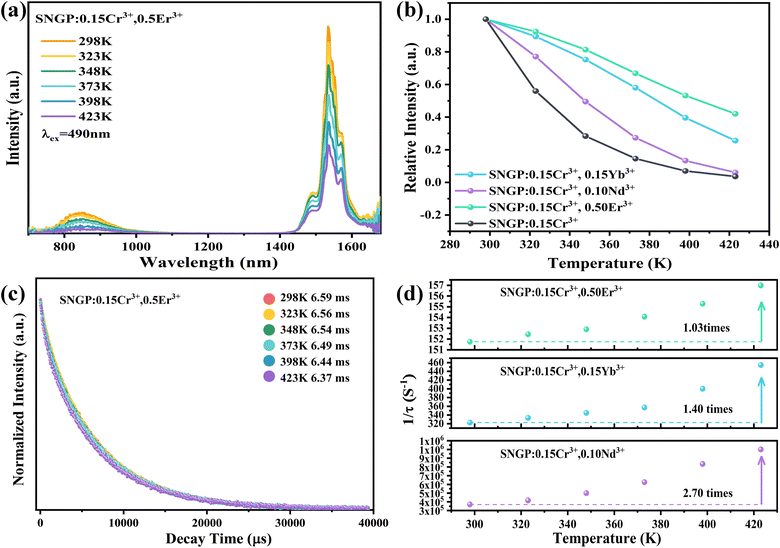 |
| Fig. 7 (a) PL spectra of phosphor 4 at different temperatures. (b) Dependencies of PL intensities for phosphors 1–4 on temperature. (c) Decay curves of phosphor 4 at different temperatures. (d) Decay rates (1/τ) of the Nd3+, Yb3+ and Er3+ luminescence at different temperatures corresponding to phosphors 2–4. | |
4. Multifunctional application of SNGP:Cr3+,Ln3+ phosphors
4.1. Applications in NIR pc-LEDs
The NIR pc-LEDs 1–3 were prepared by coating phosphors 1–3 on blue chips (λem = 450 nm), respectively, and the NIR pc-LEDs 1–3 are effectively excited using blue chips and exhibit NIR emissions (Fig. 8a). Fig. S6a–c (ESI†) shows their electroluminescence spectra at different driving currents. As detailed in Fig. 8b, the output powers of the NIR pc-LEDs due to the saturated effect first increase and then fall with increasing current. The application of NIR pc-LEDs for night vision was designed and tested. Fig. 9 shows the fruit images obtained under natural light and NIR pc-LED 2. The fruit image is clear and shows the conventional color under natural light, and nothing can be captured when the natural light is tuned off. When NIR pc-LED 3 is turned on, the fruit image is captured clearly using a 760 nm filter. Compared with NIR pc-LED 1, the fruit images under NIR pc-LEDs 2 and 3 are clearer due to their higher output powers (Fig. S7, ESI†). These results illustrate that phosphors 1–3 have a promising application in night vision. Then the penetrating abilities of NIR pc-LEDs 1–3 were tested. As shown in Fig. S7 (ESI†), the veins in the finger can be clearly observed under NIR pc-LEDs 1–3, indicating that phosphors 1–3 possess good penetrating abilities for application in tissue penetration.
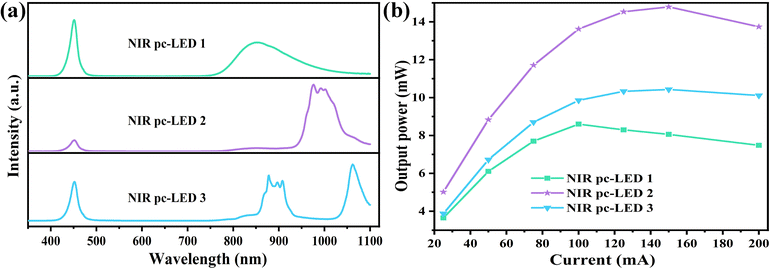 |
| Fig. 8 (a) Electroluminescence spectra of NIR pc-LED 1–3 at 20 mA drive current. (b) Output powers of NIR pc-LED 1–3 at different drive currents. | |
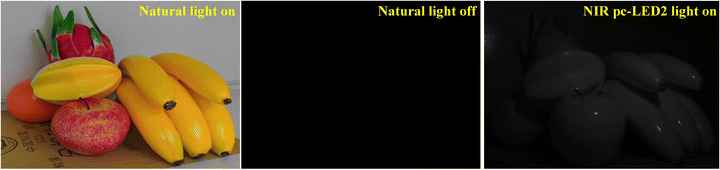 |
| Fig. 9 Images of fruits illuminated with natural light and the NIR pc-LED 2 light. | |
4.2. Applications of c-Si based solar cells
Fig. 10 represents the AM 1.5 solar spectrum, the spectral response of c-Si solar cells, and the PLE and PL spectra of phosphors 2 and 3. The PLE spectrum of phosphor 2 is consistent with the maximum photon flux region of the solar spectrum, and the PL spectrum matches well with the spectral response of c-Si solar cells. For phosphor 2, the PLE spectrum comprises more absorption bands distributed in the UV-visible region of the solar spectrum. Its PL spectrum consisting of two emission bands matches well with the spectral response of c-Si solar cells, especially, the P2 is coherent with the region of maximum response. Therefore, phosphors 2 and 3 possess a substantial application potential in c-Si solar cells.
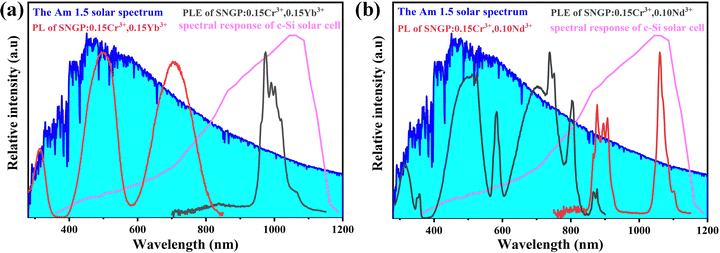 |
| Fig. 10 AM 1.5 solar spectrum, the spectral response of c-Si solar cells, and the PLE and PL spectra of phosphors 2 and 3. | |
4.3. Application in ratiometric luminescent thermometers
The SNGP:0.15Cr3+,0.03Nd3+,0.15Er3+ phosphor was designed as a ratiometric luminescent thermometer for investigation. Fig. S8 (ESI†) shows the PL spectra of SNGP:0.15Cr3+,0.03Nd3+,0.15Er3+ from 173 to 423 K, and the emission intensities of both Nd3+ and Er3+ ions decrease at different rates when the temperature increases. As shown in Fig. 11a, the emission intensity of Nd3+ ions attenuates faster than that of Er3+ ions. The thermometric property of the sample was further evaluated from the luminescence intensity ratio (LIR) and relative sensitivity (SR), which are two important parameters for thermometers. The LIR 1 (between P1 and Er3+-emission) and LIR 2 (between P2 and Er3+-emission) were calculated using the following equation:43–46 | 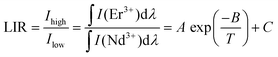 | (13) |
where Ihigh and Ilow present the integral intensities of the high-energy emission band and low-energy emission band, respectively. A, B and C are constants. Fig. 11b and c shows the LIR 1 and LIR 2 at various 1/T values. Both LIR 1 and LIR 2 curves exhibit a slow decline from 1/T = 0.00562 K−1 (T = 178 K) to 1/T = 0.00366 K−1 (T = 273 K) and a fast decline from 1/T = 0.00366 K−1 (273 K) to 1/T = 0.00236 K−1 (423 K). The values of LIR 1 and LIR 2 at 423 K are 0.43 and 0.60, respectively. Then the SR1 of P1 and Er3+-emission, and SR2 of P2 and Er3+-emission were calculated using the following equations:43–46 | 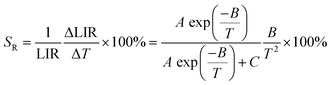 | (14) |
where A, B and C are constants, and ΔLIR represents its change for ΔT change in temperature. The values of A, B, and C for SR1 are 553, −2624.7, and 0.46, respectively; the values of A, B, and C for SR2 are 10
362, −3456.1, and 0.632, respectively. These values are obtained by fitting the experimental LIR 1 and LIR 2 data points in Fig. 11b and c. Fig. 11d plots the SR1 and SR2 at different temperatures. It is observed that both SR1 and SR2 increase and then decrease with increasing temperature. When T > 309 K, SR2 is significantly higher than SR1, and the value of SR2 is greater than 1% in the range from 340 to 588 K. The result means that the relative emission intensity of P2 and Er3+ emission is more suitable for ratiometric luminescent thermometers and indicates that SNGP:0.15Cr3+,0.03Nd3+,0.15Er3+ is a good candidate for ratiometric luminescent thermometers.
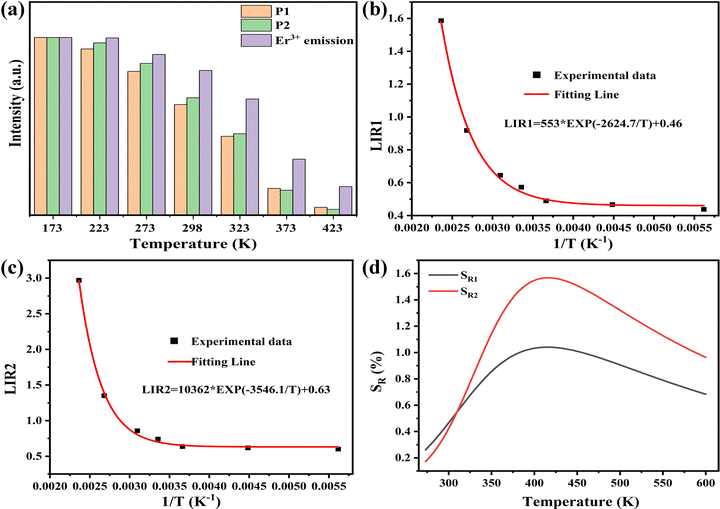 |
| Fig. 11 (a) Histogram of the temperature-dependent emission intensity for P1, P2 and Er3+-emission. (b) LIR 1 versus 1/T (c) LIR 2 versus 1/T. (d) Dependencies of SR1 and SR2 on temperature. | |
5. Conclusion
In this work, a novel NIR phosphor SNGP:Cr3+ was synthesized using a solid state reaction method, and the phosphor exhibits a broadband emission peak at 849 nm with a FWHM of 140 nm. When Ln3+ ions were co-doped into SNGP:0.15Cr3+ phosphors, different Cr3+ → Ln3+ energy transfer paths were formed which enhanced the PL properties of the phosphors. The total emission intensity of SNGP:0.15Cr3+,0.15Yb3+ increased to 172%, the IQE of SNGP:0.15Cr3+,0.15Yb3+/0.10Nd3+ is greater than 70%, and the thermal quenching of SNGP:Cr3+ is obviously inhibited by co-doping Er3+ ions. More importantly, the Cr3+ → Ln3+ energy transfer paths were further investigated. We found that the high energy transfer efficiency is attributed to the shorter distances between Cr3+ ions and Ln3+ ions; both radiative and nonradiative absorption energies of Cr3+ ions are transferred to Ln3+ ions. The energy transfer efficiency of Cr3+ → Yb3+ is the highest, reaching 75%, and co-doping with Yb3+ ions or Er3+ ions better suppress the temperature induced nonradiative transition of the SNGP:Cr3+ phosphor than with Nd3+ ions. The different energy transfer paths of Cr3+ → Ln3+ lead to different luminescence properties of the samples, which increases the potential of the phosphors for multifunctional applications. The NIR pc-LEDs prepared from phosphors 1–3 possess a good night vision and penetrating ability. Phosphors 2 and 3 have good application potential in c-Si solar cells. Furthermore, the SNGP:0.15Cr3+,0.03Nd3+,0.15Er3+ phosphor as a ratiometric luminescent thermometer exhibits a good relative sensitivity (SR > 1%) in the region from 340 to 588 K. The results suggest that the SNGP:Cr3+,Ln3+ NIR phosphors show satisfactory versatility.
Author contributions
This manuscript was written through contributions of all authors. All authors have given approval to the final version of the manuscript.
Conflicts of interest
The authors declare no competing financial interest.
Acknowledgements
This study was financially supported by the National Natural Science Foundation of China (grant no. 52072363), the special fund of Jiangsu Province for the transformation of scientific and technological achievements (BA2020007), the Key Research Program of the Chinese Academy of Sciences (grant no. ZDRW-CN-2021-3-3), and the Natural Science Foundation of Jilin province (20200201106JC).
References
- R. Jiang, J. Yang, Y. Meng, D. Yan, C. Liu, C. Xu and Y. Liu, Dalton Trans., 2020, 49, 6074–6083 RSC.
- Y. Zhang, S. Miao, Y. Liang, C. Liang, D. Chen, X. Shan, K. Sun and X. J. Wang, Light: Sci. Appl., 2022, 11, 136 CrossRef CAS PubMed.
- Z.-H. Zheng, B.-M. Liu, Z. Zhou, C.-G. Ma and J. Wang, J. Mater. Chem. C, 2022, 10, 8797–8805 RSC.
- J. Qin, J. Xiang, H. Suo, Y. Chen, Z. Zhang, X. Zhao, Y. Wu and C. Guo, J. Mater. Chem. C, 2019, 7, 11903–11910 RSC.
- S. Miao, Y. Liang, D. Chen, R. Shi, X. Shan, Y. Zhang, F. Xie and X. J. Wang, ACS Appl. Mater. Interfaces, 2022, 14, 53101–53110 CrossRef CAS PubMed.
- X. H. Chen, E. H. Song, Y. Y. Zhou, F. Q. He, J. Q. Yang and Q. Y. Zhang, J. Mater. Chem. C, 2021, 9, 13640–13646 RSC.
- Q. Lin, Q. Wang, M. Liao, M. Xiong, X. Feng, X. Zhang, H. Dong, D. Zhu, F. Wu and Z. Mu, ACS Appl. Mater. Interfaces, 2021, 13, 18274–18282 CrossRef CAS PubMed.
- H. Xiao, J. Zhang, L. Zhang, H. Wu, H. Wu, G. Pan, F. Liu and J. Zhang, Adv. Opt. Mater., 2021, 9, 2101134 CrossRef CAS.
- S. Fang, T. Lang, M. Cai and T. Han, J. Alloys Compd., 2022, 902, 163825 CrossRef CAS.
- K. Maciejewska, A. Bednarkiewicz and L. Marciniak, Physica B, 2021, 620, 413247 CrossRef CAS.
- H. Zhang, J. Zhong, X. Zhang, H. Yang, Z. Mu and W. Zhao, J. Alloys Compd., 2022, 894, 162386 CrossRef CAS.
- K. Elzbieciak-Piecka, C. Matuszewska and L. Marciniak, New J. Chem., 2019, 43, 12614–12622 RSC.
- W. Piotrowski, L. Dalipi, R. Szukiewicz, B. Fond, M. Dramicanin and L. Marciniak, J. Mater. Chem. C, 2021, 9, 12671–12680 RSC.
- L. Marciniak, A. Bednarkiewicz, J. Drabik, K. Trejgis and W. Strek, Phys. Chem. Chem. Phys., 2017, 19, 7343–7351 RSC.
- W. M. Piotrowski, K. Maciejewska, L. Dalipi, B. Fond and L. Marciniak, J. Alloys Compd., 2022, 923, 166343 CrossRef CAS.
- L. Dong, J. Hou, B. Shao, S. Zhao and Y. Fang, Opt. Mater., 2022, 127, 112290 CrossRef CAS.
- P. Kumar, Kanika, S. Singh, R. Lahon, A. Gundimeda, G. Gupta and B. K. Gupta, J. Lumin., 2018, 196, 207–213 CrossRef CAS.
- P. K. Tawalare, V. B. Bhatkar, S. K. Omanwar and S. V. Moharil, J. Alloys Compd., 2019, 790, 1192–1200 CrossRef CAS.
- L. Dong, L. Zhang, Y. Jia, Y. Xu, S. Yin and H. You, Ceram. Int., 2021, 47, 3127–3135 CrossRef CAS.
- F. Fan, S. Yu, Y. Li, Y. Xu, Y. Song, Y. Yan, H. Wu, W. Wang and L. Zhao, Inorg. Chem., 2022, 61, 13618–13626 CrossRef CAS PubMed.
- J. Zhong, Y. Zhuo, F. Du, H. Zhang, W. Zhao and J. Brgoch, ACS Appl. Mater. Interfaces, 2021, 13, 31835–31842 CrossRef CAS PubMed.
- X. Xu, Q. Shao, L. Yao, Y. Dong and J. Jiang, Chem. Eng. J., 2020, 383, 123108 CrossRef CAS.
- P. Huang, W. Zheng, D. Tu, X. Shang, M. Zhang, R. Li, J. Xu, Y. Liu and X. Chen, Adv. Sci., 2019, 6, 1802282 CrossRef PubMed.
- D. Huang, Q. Ouyang, H. Xiao, B. Wang, H. Lian, Q. Zeng and J. Lin, Dalton Trans., 2021, 50, 908–916 RSC.
- F. Zhao, Z. Song and Q. Liu, Laser Photonics Rev., 2022, 16, 2200380 CrossRef CAS.
- G. Liu, M. S. Molokeev and Z. Xia, Chem. Mater., 2022, 34, 1376–1384 CrossRef CAS.
- G. Wei, P. Li, R. Li, J. Li, Y. Shi, Y. Wang, S. He, Y. Yang, H. Suo and Z. Wang, Inorg. Chem., 2022, 61, 5665–5671 CrossRef CAS PubMed.
- X. Zou, H. Zhang, W. Li, M. Zheng, M. S. Molokeev, Z. Xia, Y. Zheng, Q. Li, Y. Liu, X. Zhang and B. Lei, Adv. Opt. Mater., 2022, 10, 2200882 CrossRef CAS.
- X. Luo, X. Yang and S. Xiao, Mater. Res. Bull., 2018, 101, 73–82 CrossRef CAS.
- L. Yao, Q. Shao, S. Han, C. Liang, J. He and J. Jiang, Chem. Mater., 2020, 32, 2430–2439 CrossRef CAS.
- L. Zhang, L. Dong, B. Shao, S. Zhao and H. You, Dalton Trans., 2019, 48, 11460–11468 RSC.
- J. Zhu, Z. Xia and Q. Liu, Mater. Res. Bull., 2016, 74, 9–14 CrossRef CAS.
- Y. Jin, Z. Zhou, R. Ran, S. Tan, Y. Liu, J. Zheng, G. Xiang, L. Ma and X. J. Wang, Adv. Opt. Mater., 2022, 10, 2202049 CrossRef CAS.
- C. Yuan, R. Li, Y. Liu, L. Zhang, J. Zhang, G. Leniec, P. Sun, Z. Liu, Z. Luo, R. Dong and J. Jiang, Laser Photonics Rev., 2021, 15, 2100227 CrossRef CAS.
- Z. Jia, C. Yuan, Y. Liu, X. J. Wang, P. Sun, L. Wang, H. Jiang and J. Jiang, Light: Sci. Appl., 2020, 9, 86 CrossRef CAS PubMed.
- F. Zhao, H. Cai, Z. Song and Q. Liu, Chem. Mater., 2021, 33, 3621–3630 CrossRef CAS.
- F. Zhao, H. Cai, Z. Song and Q. Liu, Chem. Mater., 2021, 33, 8360–8366 CrossRef CAS.
- Q. Zhang, X. Wang and Y. Wang, J. Alloys Compd., 2021, 886, 161217 CrossRef CAS.
- C. Zhong, L. Zhang, Y. Xu, X. Wu, S. Yin, X. Zhang and H. You, Mater. Today Chem., 2022, 26, 101233 CrossRef CAS.
- C. Zhong, L. Zhang, Y. Xu, X. Wu, S. Yin, X. Zhang and H. You, J. Alloys Compd., 2022, 903, 163945 CrossRef CAS.
- L. Kong, Y. Liu, L. Dong, L. Zhang, L. Qiao, W. Wang and H. You, Dalton Trans., 2020, 49, 8791–8798 RSC.
- F. Du, Y. Nakai, T. Tsuboi, Y. Huang and H. J. Seo, J. Mater. Chem., 2011, 21, 4669 RSC.
- L. Dong, L. Zhang, Y. Jia, Y. Xu, S. Yin and H. You, Inorg. Chem., 2020, 59, 15969–15976 CrossRef CAS PubMed.
- Y. Zhong, S. Gai, M. Xia, S. Gu, Y. Zhang, X. Wu, J. Wang, N. Zhou and Z. Zhou, Chem. Eng. J., 2019, 374, 381–391 CrossRef.
- S. Liang, G. Li, P. Dang, Y. Wei, H. Lian and J. Lin, Adv. Opt. Mater., 2019, 7, 1900093 CrossRef.
- Y. Chen, J. He, X. Zhang, M. Rong, Z. Xia, J. Wang and Z. Q. Liu, Inorg. Chem., 2020, 59, 1383–1392 CrossRef CAS PubMed.
|
This journal is © The Royal Society of Chemistry 2023 |