DOI:
10.1039/D2TC05454J
(Paper)
J. Mater. Chem. C, 2023,
11, 2484-2493
Orthogonal electric and ionic conductivities in the thin film of a thiophene–thiophene block copolymer†
Received
22nd December 2022
, Accepted 22nd January 2023
First published on 23rd January 2023
Abstract
A thiophene–thiophene block copolymer composed of hydrophilic and hydrophobic side chain functionalities was designed and synthesized. The deprotonative metalation nickel-catalyzed polymerization protocol successfully afforded the block copolymer, in which the side chains are derived from alkyl and benzenesulfonic acid ester groups. The benzene sulfonate moiety of the block copolymer in the film state was shown to be transformed into hydrophilic sulfonic acid upon thermal treatment at ca. 200 °C without the addition of an external additive. Thus, the formed block copolymer thin film exhibited cylindrical microphase separation and the hydrophilic domain was revealed to penetrate the film perpendicular to the substrate. The measurement of electric conductivity suggested that the block copolymer thin film was conductive when placed parallel to the substrate, while the film was insulative when placed perpendicular to the substrate. Electrochemical analyses revealed that lithium ions transfer through the cylindrical domain composed of a benzene sulfonic acid side chain, which is perpendicular to the substrate. These results represent dual and orthogonal conductivities of electrons and ions in the block copolymer thin film.
1. Introduction
Polythiophenes are currently the subject of extensive research as electronic materials for conductive and semiconductive devices.1–3 In particular, regioregular poly(3-substituted thiophene)s, in which the regularity is controlled in a head-to-tail (HT) manner, exhibit particularly suitable electric properties for this purpose. Accordingly, the development of facile synthetic protocols to afford HT-type polythiophenes is a major step in synthetic chemistry.4–6
We have previously reported the deprotonative synthesis of polythiophene that involves the reaction of a 2-halo-3-substituted thiophene with magnesium amide to afford the corresponding organometallic monomer,7–11 which may then be subjected to cross-coupling polymerization followed by the addition of a nickel catalyst to afford a regioregular HT-type polythiophene with a well-controlled molecular weight as shown in Fig. 1(a).
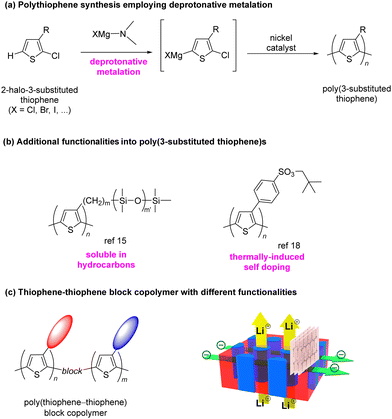 |
| Fig. 1 Synthesis of head-to-tail (HT) type polythiophene and side chain functionality of polythiophenes. | |
Furthermore, the rational design and introduction of side-chain moieties can be employed to confer additional functionality to polythiophenes.12–14 For instance, we have previously reported that (i) the introduction of an oligosiloxane side-chain moiety improves the solubility of polythiophene in organic solvents, particularly hydrocarbons such as hexanes,15 and (ii) polythiophenes bearing benzenesulfonic acid ester groups undergo thermally induced doping of the polymer main chain upon thermal decomposition of neopentyl benzene sulfonate to the corresponding sulfonic acid. This transformation at the side chain allows doping of the polythiophene main chain while simultaneously conferring water-solubility to the polymer (Fig. 1(b)).
Our extensive research on the structural design of polythiophenes has focused on the introduction of composite functionality, which can be achieved by the synthesis of polythiophene copolymers comprising monomers with different functional groups on their side chains.16,17 For instance, we have recently reported that introducing a small amount of a thiophene comonomer with thermally induced-doping functionality to poly(3-alkylthiophene) imparts self-doping ability to the resultant random copolymer.18
Our further concerns are related to the synthesis of copolymers composed of different polythiophene moieties, which represents a breakthrough in terms of functionality design in polythiophene synthesis.19 Because the formation of a block copolymer induces microphase separation in its corresponding polymer film, such films have application potential in areas such as selective gas permeation, ion transfer, etc.20 It has been shown that a block copolymer with a certain ratio of each copolymer exhibits a microdomain structure in the form of a cylinder or a lamella. Therefore, such a microphase separation would lead to the formation of a penetrating substructure in the thin film state on a substrate.21–23 Specifically, a copolymer containing some polythiophene units with benzenesulfonic acid side-chain functionality could potentially form micro-sized domains capable of ion conduction24,25 along with the electron-conductive characteristics of the polythiophene itself. The ion conductivity imparted by discrete sulfonate microdomains would reflect in the thin film formed on the substrate.26,27 However, such characteristics are rarely found in other electronic materials.
Herein, we report the block copolymerization of different types of thiophene monomers with either alkyl or benzenesulfonate side chains. A thin film formed from this copolymer was demonstrated to exhibit cylindrical microphase separation that penetrates throughout the film providing ion conductivity as well as the electrically conductive characteristics of regioregular poly(3-substituted thiophene). Both conductivities of the film are demonstrated in orthogonal directions perpendicular and parallel to the substrate, respectively (Fig. 1(c)).
2. Results and discussion
2.1 Synthesis and characterization
Synthesis of a thiophene block copolymer derived from alkyl-substituted 1 and benzenesulfonate 2 was performed as shown in Scheme 1. Initial polymerization of 2-chloro-3-hexylthiophene (1) was carried out via the reaction with the bulky magnesium amide 328 followed by the addition of the nickel catalyst NiCl2(IPr)PPh3 (4) (2 mol% relative to 1).7 The second organometallic monomer, which was prepared via the reaction of 2 and magnesium amide 3, was then added to the polymerization solution.29 Further stirring of the mixture for 24 h at room temperature afforded block copolymer 5a in 74% yield. 1H NMR analysis indicated a block ratio of 1.0
:
0.9 based on the integral values of the methylene signal for the hexylthiophene moiety derived from 1 (2H)n and the neopentyl methylene signal from 2 (2H)m.
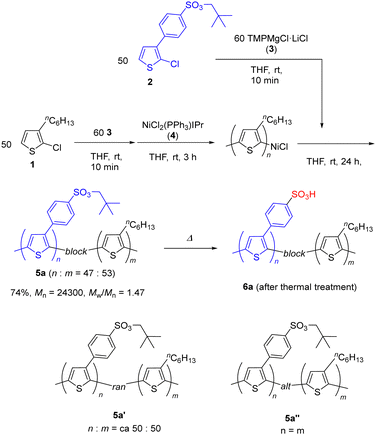 |
| Scheme 1 Synthesis of a head-to-tail (HT) type polythiophene and side chain functionality of polythiophenes. | |
Block copolymerization of monomer precursors 1 and 2 at different ratios was also performed. Table 1 shows the details of these products. The reactions provided the corresponding products in good to excellent yields with reasonable average molecular weights (Mn) and relatively narrow molecular weight distributions (Mw/Mn). The ratios of the components in block copolymers 5b–g were estimated from their 1H NMR spectra and are presented as n
:
m. This ratio was found to correspond reasonably well with the feed ratio of the monomer precursors 1 and 2. These results show that polymerization of metalated 2 proceeds at the living end of the polymerized alkylthiophene 1 smoothly and revealed that incorporation of both metalated monomers of 1 and 2 took place at room temperature within 24 h.
Table 1 Characterization of block copolymers 5 with different feed ratiosa
5
|
1/2b |
Yield (%) |
M
n
(Mw/Mn) |
m : nd |
The first polymerization was carried out by the reaction of chlorothiophene 1 (0.3 mmol), TMPMgCl·LiCl (3, 0.33 mmol) and nickel catalyst 4 in THF. Polymerization was carried out at room temperature for 3 h. The monomer derived from 2 (0.3 mmol) was added to the reaction mixture for the first polymerization to initiate the second propagation and the resulting thiophene–thiophene block copolymer 5 was obtained as a dark purple solid.
The feed ratio of 1 and 2 (mol mol−1). In parenthesis, the mass ratio of the benzenesulfonate moiety is shown.
M
n and Mw/Mn were determined by SEC analysis based on polystyrene standards.
The ratio of m : n was determined by 1H NMR analysis.
|
5a
|
0.5 : 0.5 |
74 |
24 300 (1.47) |
0.53 : 0.47 (62%) |
5b
|
0.8 : 0.2 |
71 |
16 000 (1.73) |
0.83 : 0.16 (28%) |
5c
|
0.7 : 0.3 |
87 |
13 000 (1.75) |
0.76 : 0.24 (37%) |
5d
|
0.6 : 0.4 |
79 |
17 700 (1.62) |
0.67 : 0.33(48%) |
5e
|
0.4 : 0.6 |
83 |
24 000 (1.36) |
0.43 : 0.57(71%) |
5f
|
0.3 : 0.7 |
71 |
21 800 (1.32) |
0.29 : 0.71 (82%) |
5g
|
0.2 : 0.80 |
48 |
14 000 (1.32) |
0.21 : 0.79 (87%) |
The obtained copolymer 5a was found to be soluble in organic solvents such as chloroform and THF. However, it was found to be insoluble in both chloroform and water after being heated at ca. 200 °C for 10 min. This result indicates the formation of an amphiphilic block copolymer 6a bearing hydrophobic alkyl groups and hydrophilic sulfonic acid (–SO3H) pendant groups. It is very different from the result for the corresponding benzenesulfonate-bearing homopolymer, which is soluble in water after heating. We also prepared and characterized two related copolymers, both with a similar n
:
m ratio to that of 5a. These were the random 5a′ prepared by the copolymerization of 1 and 218 and the alternating copolymer 5a′′, prepared by the polymerization of a bithiophene dimer bearing both the substituents present on 1 and 2.16
The TG-DTA trace for 5a (m
:
n = ca. 0.5
:
0.5) shows a weight loss of ca. 15% at 200 °C. (Fig. 2) This result was found to agree reasonably with the calculated 1H NMR result (m
:
n = 0.53
:
0.47) and the molecular formulas of 2 –(C10H14S)m–(C15H16S2O3)n–, indicating a 14% loss with the elimination of C5H10 from the side-chain neopentyl sulfonate. Based on the results from TG-DTA, spectroscopic analyses of block copolymer 5a in the film state were performed on a quartz substrate. Measurements after thermal treatment were performed by heating the substrate at 200 °C for 20 min. As shown in Fig. 3(a), the UV-vis absorption spectrum of 5a in the film state shows a λmax value of ca. 560 nm, while an emergent absorption at >700 nm along with a decrease in absorbance at λmax is observed in 6a upon thermal treatment, suggesting that thermally induced self-doping of the polythiophene main chain via the formation of SO3H on the side chains occurs.18 We next performed XRD with the thin film of block copolymer 6a after thermal treatment. The measurement of the out-of-plane profile revealed a characteristic peak at 2θ = 5.4°, which was assigned as the (100) reflection suggesting that the layer distance derived from the polythiophene side chain (16.4 Å). In contrast, a peak at 2θ = 15–20° that was characterized as the layer distance between conjugated polymers with π–π stacking (5.7 Å) was broad and relatively smaller toward the (100) reflection (Fig. 3(b)). These results in the out-of-plane measurement strongly suggest edge-on preference of the polythiophene film on the substrate, whereas attempted in-plane measurement was unsuccessful.
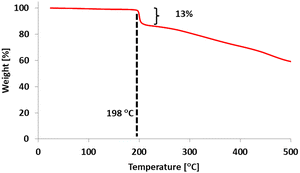 |
| Fig. 2 TG-DTA profile of block copolymer 5a. | |
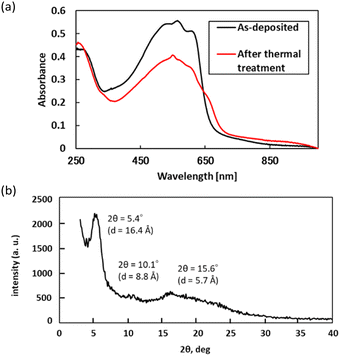 |
| Fig. 3 (a) UV-vis absorption spectra of block copolymer 5a before and after thermal treatment. (b) X-ray diffraction pattern (out-of-plane) of 5a after thermal treatment on a glass substrate. | |
2.2 Microscopic analyses of block copolymer thin films
We next investigated the properties of 5a as a thin film. Copolymer films were fabricated by spin coating a chloroform solution of 5a (ca. 5 mg mL−1) on a glass substrate. The spin-coated thin film was formed at a spin rate of 1000 rpm for 1 min. The obtained thin films were heated at 200 °C for 20 min, and AFM observation was performed before and after thermal treatment.24 Block copolymer 6a exhibits a clear dotted pattern in the tapping mode after heating, indicating microphase separation. The dark images suggest the polythiophene domain bearing a benzene sulfonic acid moiety at the side chain, while the bright ones as the alkylthiophene domain. Such a phase separation is only observed in the thermally treated film, whereas significant phase separation is not observed clearly in the as-cast film. In addition, this microphase separation is not observed despite heating the random copolymer 6a′ or the alternating copolymer 6a′′ (Fig. 4(a)–(d)).
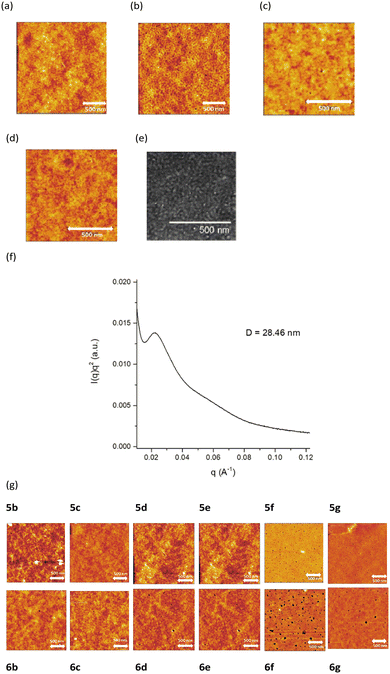 |
| Fig. 4 AFM and TEM images of copolymers 5 derived from 1 and 2 moieties. (a) AFM image of block copolymer 5a before thermal treatment. (b) Block copolymer 5a after thermal treatment. (c) Random copolymer 5a′ after thermal treatment. (d) Alternating copolymer 5a′′ after thermal treatment. (e) TEM image of block copolymer 5a at 200 kV after thermal treatment. (f) SAXS profile of block copolymer 5a after thermal treatment. (g) AFM images of block copolymers with different ratios of alkyl and benzenesulfonate substituents before (5b–g) and after the thermal treatment (6b–g). | |
These results suggest that microphase separation appears in 6a after thermal treatment at 200 °C for 20 min owing to the presence of the two different side chains in a specific arrangement. Although the dotted pattern is not observed for 5a before heating, this does not necessarily show that phase separation proceeds upon heating of the as-deposited film, because it becomes much more apparent in AFM observation after the decrease in volume caused by the elimination of the neopentyl group (as C5H10). Considering that the related dotted pattern was not observed in the related random (6a′) or alternating (6a′′) copolymer (Fig. 4(c) and (d), respectively), these results also support that the observed dots are the result of microphase separation and the formation of a gas bubble by the evolving C5H10 may also be ruled out (see also Fig. S1 and S2, ESI†).
Similar to the results for AFM, the TEM image of 6a (Fig. 4(e)) was measured at 200 kV and shows a dotted pattern, albeit weak contrast because of the structural similarity of the main chain in each domain, as that observed by AFM,23,30–32 confirming that the thin film of 5a features cylindrical phase separation that penetrates the film perpendicular to the substrate. Measurement of small-angle X-ray scattering (SAXS) of 6a also supported the formation of microphase separation. As shown in Fig. 4(f), the SAXS curve showed a primary peak along with a hump. The interdomain distance D determined from the peak position was 28.46 nm, which agreed with that estimated by AFM and TEM analyses. It should be noted that such a peak was only observed in the thermally treated block copolymer, where no peak was found in 5a before thermal treatment as well as the corresponding random copolymer 5a′ (see also the ESI†).
We also subjected 5b–5g to AMF analysis, as shown in Fig. 4(g). Similar dotted patterns are observed for 6b, 6c, 6d, and 6e but not for 6f and 6g. This suggests that phase separation occurs after heating when the alkylthiophene moiety content is high, while a higher benzenesulfonate content appears to disrupt the phase separation.
2.3 Electric properties of thin films
Having confirmed the potential occurrence of cylindrical microphase separation that penetrates the thin film, we next studied the electric properties of the films of block copolymers 5, which were fabricated on ITO electrodes or glass substrates, where gold electrodes were deposited as shown in Fig. 5. The ITO electrode was deposited on a quartz substrate with a gap length of ca. 200–800 μm and a chloroform solution of the block copolymer was cast on the electrode, and the thickness of the film was found to be ca. 200 nm, as depicted in Fig. 5(a).33 Conductivity measurements toward the parallel direction on the substrate were performed in the applied voltage range of 0–100 V.
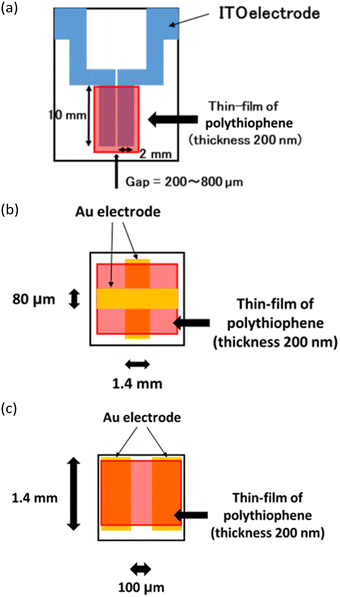 |
| Fig. 5 Measurement of electric conductivity of the block copolymer thin film of 5. (a) Measurement of the conductivity of the thin film of block copolymer 5 before/after thermal treatment at the applied voltage up to 100 V, (b) at low voltages (0–10 V) perpendicular to the film on the substrate, and (c) at the low voltage parallel to the film (0–10 V). | |
The conductivity in the perpendicular direction of the substrate was measured using a gold electrode at a lower voltage (0–10 V) as shown in Fig. 5(b), with a gap length of ca. 80 μm and a film thickness of ca. 200 nm, where the film was cast on the Au electrode followed by the additional deposition of the Au electrode on the polythiophene film. The related gold electrode for the measurement in the parallel direction under conditions similar to those shown in Fig. 5(b) (0–10 V) was also applied as shown in Fig. 5(c), where widths of the Au electrodes were set as 1.4 mm and 100 μm, respectively, and the film thickness was 200 nm.
The conductivities of the block copolymers at an applied voltage of 100 V before and after thermal treatment are summarized in Table 2. It was found that the conductivity of 5a is 8.92 × 10−5 S cm−1 before thermal treatment and that of 6a is 9.37 × 10−3 S cm−1 (100 V) after thermal treatment. This suggests that the conductivity is improved ca. 100-fold by thermal treatment. A smaller improvement in conductivity is observed for copolymer 6b because of its lower benzenesulfonate content (<20%). Improved conductivity after thermal treatment is observed for 6g owing to its higher sulfonate content, albeit with little microphase separation. The conductivity after thermal treatment is observed in the parallel direction to the substrate. The results suggest that the polythiophene block copolymer 5 in the film state on the substrate is cast as edge-on orientation as depicted in Fig. 1(c) (right).
Table 2 Summary of the results of the electric conductivity of polythiophene block copolymers 5aa
|
1/2b (mol mol−1) |
Applied voltate,c V |
Conductivity before thermal treatment, S cm−1 |
Conductivity after thermal treatment, S cm−1 |
Measurements were carried out using devices as depicted in Fig. 5(a)–(c).
The ratio derived from 1 and 2 in the block copolymer of 5. Based on the result of 1H NMR measurement.
In parenthesis, the measured device is shown in Fig. 5.
|
5a
|
0.53/0.47 |
100 (a) |
8.92 × 10−5 |
9.37 × 10−3 |
5b
|
0.83/0.16 |
100 (a) |
1.10 × 10−5 |
2.51 × 10−4 |
5g
|
0.21/0.79 |
100 (a) |
|
8.12 × 10−2 |
5a
|
0.53/0.47 |
10 (b) |
|
6.06 × 10−10 |
5a
|
0.53/0.47 |
10 (c) |
1.75 × 10−4 |
1.58 × 10−3 |
It should be pointed out that the conductivity using the thin film shown in Fig. 5(b) at the applied voltage of 0–10 V is remarkably low even after thermal treatment showing insulative characteristics in the perpendicular direction. On the other hand, the related Au electrode (Fig. 5(c)) showed a much superior conductivity of 6a (1.58 × 10−3 S cm−1 at 10 V) in the parallel direction of the film similar to the case of the ITO electrode. These results support the edge-on orientation of the thiophene–thiophene block copolymer 5a and that the thermally induced self-doping of the polythiophene moiety bearing benzenesulfonic acid improved the conductivity in the poly(alkylthiophene) moiety.34,35
2.4 Electrochemical properties of thin films
We then measured the ionic conductivities of 5a and 5a′ by electrochemical impedance spectroscopy (EIS) using a conventional three-electrode setup in an aqueous solution of 0.5 M Li2SO4, as shown in Fig. 6(a).36 The working electrode, composed of the polymer thin film spin-coated onto ITO glass, and the Pt reference electrode were configured such that the measurements reflect through-plane charge transport.
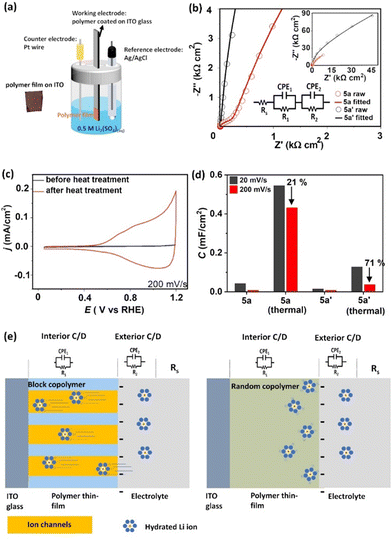 |
| Fig. 6 EIS and CV measurements. (a) EIS and CV measurements with three electrode electrochemical setups. (b) Nyquist plots of heat treated films of 5a and 5a′ (inset: at lower frequency and a EIS model). (c) CV measurements of a film of 5a before and after heat treatment. (d) Results of the measured capacitance of 5a and 5a′ before and after heat treatment. (e) Schematic illustration of the transfer of hydrated lithium ions through the copolymers 5a and 5a′. | |
The Nyquist plot acquired for the thermally treated film of 6a at 0.6 V vs. the reversible hydrogen electrode (RHE) features an additional semicircle at the high-frequency region, as shown in Fig. 6(b) (red plot), while that of 6a′ does not (black plot). The plots for 6a and 6a′ can be fitted with an equivalent circuit composed of one resistor (Rs) in series with two resistor–capacitor (R1-CPE1 and R2-CPE2) units (Fig. 6(b), solid lines). The impedances and capacitances of the systems fabricated with 6a and 6a′ were estimated as summarized in Table 3, demonstrating the remarkably low R1 and R2 values for 6a compared with those for 6a′. The obtained values for resistance for 6a, both R1 and R2, were 2.06 × 102 W cm2 and 8.06 × 104 W cm2, respectively. These values were significantly lower than those obtained for 6a′, which were 3.48 × 104 W cm2 and 2.66 × 105 W cm2, respectively (Table 3).
Table 3 Summary of EIS equivalent circuit fittinga
|
R
1
(Ω cm2) |
CPEb (F cm−2) |
R
2
(Ω cm2) |
CPE2-Tc (F cm−2) |
The measurement was performed as illustrated in Fig. 6(a).
Impedance and capacitance of copolymers before thermal treatment.
Impedance and capacitance of copolymers after thermal treatment.
|
5a
|
2.06 × 102 |
9.67 × 10−5 |
8.06 × 104 |
7.38 × 10−5 |
5a′
|
3.48 × 104 |
9.04 × 10−5 |
2.66 × 105 |
2.03 × 1015 |
Cyclic voltammetry (CV) analysis of 5a and 6a (Fig. 6(c)) supports the EIS results. A cyclic voltammogram is only clearly observed for the thermally treated film of 6a, and the capacitance was calculated to be 0.431 mF cm−2 at a scan rate of 200 mV s−1, as compared with 0.08 mF cm−2 before thermal treatment. In addition, the excellent retention of capacitance compared with that at the slower scan rate of 20 mV s−1 (21% loss) reflects the superior ionic conductivity of thermally treated 6a. The cyclic voltammograms for 5a′ and 6a′ also show differences before and after thermal treatment, but the capacitance of 5a′ is much lower (Fig. 6(d)). Furthermore, even after thermal treatment, 6a′ loses 71% of its capacitance when the scan rate is increased from 20 to 200 mV s−1.
These results show that lithium-ion conductivity is observed for the thermally treated copolymers 6a and 6a′, while the as-cast films are much less ion conductive. Because the thermal treatment of the polythiophene film converts benzenesulfonate (–SO2OR) into the corresponding sulfonic acid (–SO3H), lithium ions can interact with the SO3− moiety, resulting in ion conductivity.
Compared with the corresponding values for 6a′, the lower resistance of 6a by EIS measurements as well as its higher capacitance and a smaller decrease upon increasing the CV scan rate (200 vs. 20 mV s−1) indicate that 6a does indeed feature channel domains that transport hydrated lithium ions, as depicted in Fig. 6(e). These results also correspond with the AFM and TEM results shown in Fig. 4, which support the formation of cylinder-like microphase separation regions penetrating the film perpendicular to the substrate.
Combined with the electric conductivity measurements shown in Fig. 5, the thin film of 6a exhibits dual orthogonal conductivities for electrons (parallel to the substrate) and lithium ions (perpendicular to the substrate). To the best of our knowledge, these unique characteristics have not been observed in any previous polythiophene materials.
3. Conclusions
We demonstrated the novel properties of thiophene–thiophene block copolymers containing hydrophobic and hydrophilic side-chain structures. By performing block copolymerization of alkylthiophene 1 and benzenesulfonate pendant thiophene 2 followed by thermal treatment of the sulfonate ester leading to the corresponding sulfonic acid, a new class of amphiphilic block copolymers bearing hydrophilic and hydrophobic moieties composed of a single polythiophene main chain were produced. Such block copolymers undergo microphase separation, as confirmed by AFM and TEM analyses. Electric conductivity measurements showed that after thermal treatment, electrons transfer parallel to the thin-film substrate and that the film is insulative in the perpendicular direction based on the edge-on orientation of polythiophene. Conversely, lithium-ion channels through the domain of polythiophene bearing sulfonic acid at the side chain are formed perpendicular to the substrate. Thus, orthogonal dual functionalities in terms of electron and ion transportation have been achieved in polythiophene–polythiophene block copolymer thin films.
4. Experimental section
4.1 General
All the polymerization procedures were performed in a glove box under an argon atmosphere or using a standard Schlenk technique under a nitrogen atmosphere. 1H NMR (400 MHz) and 13C{1H} NMR (100 MHz) spectra were recorded on a JEOL ECZ400 in a CDCl3 solution. The chemical shifts were expressed in ppm with CHCl3 (7.26 ppm for 1H) or CDCl3 (77.00 ppm for 13C) as internal standards. SEC (size exclusion chromatography) analyses were performed using a standard HPLC system equipped with a UV detector at 40 °C using chloroform as an eluent with a Shodex GPC KH-404HQ or the related column. Molecular weights and molecular weight distributions were estimated on the basis of the calibration curve obtained by 6 standard polystyrenes (Mn = 2630–355
000). High resolution mass spectra (HRMS) were recorded using a JEOL JMS-T100LP AccuTOF LC-Plus (ESI) with a JEOL MS-5414DART attachment. Elemental analyses were carried out at the Department of Instrumental Analysis & Cryogenics Division of Instrumental Analysis, Okayama University on a PerkinElmer 2400II Elemental analyzer supported by the Inter-University Network for Common Utilization of Research Equipments. IR spectra were recorded on a Bruker Alpha with an ATR attachment (Ge). UV-vis spectra (as a thin film) were recorded using a Shimadzu UV-3101PC. Thermal analyses were carried out with a RIGAKU Thermo plus EVO2 TG-DTA 8121. XRD analysis was carried out with a Rigaku SmartLab with the power of 40 kV, 30 mA, and the wavelength of 1.5418 Å (CuKα). The incidence angle α of the X-ray beam was 0.20°. The XRD measurements were performed with the out-of-plane geometry. Atomic Force Microscopy (AFM) measurements of the thin film were performed in a tapping mode using a Shimadzu UV-3101PC. TEM measurements were performed using a HITACHI-SU8010 (200 kV). The formation of polymer thin films was performed using spin coater SWINCO and the thickness of the film was measured with stylus profiler ULVAC DEKTAK 8. SAXS measurements (transmission mode) were conducted at beamline TPS13A of the National Synchrotron Radiation Research Center (NSRRC), Hsinchu, Taiwan. The energy of the employed monochromatic radiation was 15 keV, affording the X-ray wavelength λ of 0.8266 Å. The 2D scattering patterns were collected using an In-vacuum Eiger X 9M (SAXS) detector. The conductivity of polymer films was measured using a digital electrometer ADCMT8340A and an Agilent B1500 semiconductor parameter analyzer. Cyclic voltammetry measurement and electrochemical impedance spectroscopy (EIS) analysis were carried out using a Biologic SP-200 potentiostat. For thin layer chromatography (TLC) analyses throughout the work, Merck-precoated TLC plates (silica gel 60 F254) were used. Purification by HPLC with a preparative SEC column (JAI-GEL-1H, JAI-GEL-2H) was performed using a JAI LC-9201.
4.2 Materials
Thiophene derivatives bearing a substituent at the 3-position were prepared in a manner shown in the literature. Chlorination of 3-hexylthiophene (1) was carried out in a similar manner as reported previously.7 Chlorothiophene bearing a benzenesulfonate group at the 3-position 2-chloro-3-(4-(2,2-dimethylpropylsulfonylbenzne)-1-yl)thiophene (2) was synthesized in a manner as reported.16 Magnesium amide (TMPMgCl·LiCl 2,2,6,6-tetramethylpiperidine-1-yl chloromagnesium lithium chloride salt (3): Knochel–Hauser base37) was purchased from Sigma–Aldrich Co. Ltd as a 1 M THF solution. Nickel catalyst NiCl2(IPr)PPh3 (4, IPr: 1,3-bis(2,6-diisopropylphenyl)imidazolin-1-yl)38 was purchased from TCI Co. Ltd and employed as a catalyst as received for polymerization. Random copolymer poly[(3-hexylthiophen-2,5-diyl)m-ran-(3-(4-(2,2-dimethylpropylsulfonylbenzne)-1-yl)thiophen-2,5-diyl)n] (5a′, m
:
n = ca. 1
:
1) was prepared in a manner as we reported previously.18 THF (anhydrous grade) was purchased from Kanto Chemical Co. Ltd and passed through alumina and copper columns (Nikko Hansen & Co. Ltd) or distilled from the sodium dispersion in a mineral oil/benzophenone ketyl prior to use.39
4.3 Synthesis of diblock copolymers
Poly[(3-hexylthiophen-2,5-diyl)m-b-(3-(4-(2,2-dimethylpropylsulfonylbenzn)-1-yl)thiophen-2,5-diyl)n] (5a): to a screw-capped test tube equipped with a magnetic stirring bar were added 2-chloro-3-hexylthiophene (1, 0.66 mL, 0.3 mmol) and THF (3 mL) in a glove box. A 1 M THF solution of TMPMgCl·LiCl (3, 0.36 mL, 0.36 mmol) was added to the resulting mixture and stirring was continued for 10 min to form the corresponding organometallic monomer. Nickel catalyst NiCl2(IPr)PPh3 (4, 4.7 mg, 6 × 10−6 mol) was added to the THF solution of the monomer and the mixture was stirred for 3 h to initiate polymerization. Chlorothiophene bearing a benzenesulfonate group 2 was dissolved in 3 mL of THF in a screw-capped test tube equipped with a magnetic stirring bar. Magnesium amide 3 was added to the solution and stirring was continued for 10 min at room temperature. The resulting solution was added to the solution of the living polymer derived from 1 and the mixture was removed from the glove box and stirring was further continued for 24 h at room temperature. The reaction was terminated by the addition of 1 M hydrochloric acid to form a dark purple precipitate, which was filtered off and washed repeatedly with methanol and diethyl ether to afford the block copolymer 5a (106 mg, 74%). The average molecular weight and the PDI value were estimated by SEC analysis to reveal Mn = 24300; PDI = 1.47. The ratio of the each block was calculated by 1H NMR measurement (m
:
n = 1.0
:
0.9). 1H NMR (400 MHz, CDCl3): δ 7.89 (d, 2H, J = 8.2 Hz), 7.55 (d, 2H, J = 8.2 Hz), 6.98 (s, 1H), 6.93 (s, 1H), 3.71 (s, 2H), 2.80 (t, 2H, J = 7.8 Hz), 1.74–1.67 (br, 2H), 1.44–1.42 (br, 2H), 1.37–1.34 (br, 4H), 0.93–0.84(br, 12H). 13C{1H} NMR (100 MHz, CDCl3) δ 140.0, 137.9, 135.6, 134.1, 133.8, 130.1, 128.7, 128.4, 80.0, 31.8, 30.6, 29.5, 29.4, 26.1, 22.7 14.3. Several signals were found to be not observed because of their broadening. IR (ATR) 3760, 3483, 3409, 2971, 2958, 1739, 1366, 1228, 1217, 1188 cm−1.
The related block copolymers 5b–5g composed of different ratios of m
:
n were prepared in a similar manner shown above and the results are summarized in Table 1.
4.4 Synthesis of 2-chloro-5-(3-hexylthiophen-2-yl)-3-(4-(2,2-dimethylpropylsulfonylbenzen)-1-yl)thiophene
Preparation of such bithiophene was carried out in a manner as reported previously.16 (52% yield) 1H NMR (400 MHz, CDCl3): δ 7.88 (d, 2H, J = 8.2 Hz), 7.54 (d, 2H, J = 8.2 Hz), 7.33 (d, 1H, J = 5.0 Hz), 7.09 (d, 1H, J = 5.0 Hz), 6.70 (s, 1H), 3.71 (s, 2H), 2.49 (t, 2H, J = 7.8 Hz), 1.47–1.57 (m, 2H), 1.26–1.36 (m, 6H), 0.91 (s, 9H); 13C {1H} NMR (100 MHz, CDCl3): δ 141.7, 140.0, 137.0, 134.8, 132.8, 131.3, 130.1, 130.0, 128.2, 127.9, 125.44, 125.35, 80.0, 31.8, 31.7, 29.6, 29.0, 28.0, 26.2, 22.7, 14.3. IR (ATR) 2960, 2924, 2855, 1597, 1462, 1400, 1362, 1210, 1191, 1178, 974, 937, 836, 756 cm−1. HRMS (DART–ESI+) m/z: calcd for C25H3135Cl23NaO3S3, 533.1021: found, 533.1043.
4.5 Formal synthesis of alternating copolymer 5a′′
5a′′ was synthesized by the polymerization of bithiophene prepared in a manner as shown previously. (83% yield) 1H NMR (400 MHz, CDCl3): δ 7.90 (d, 2H, J = 8.2 Hz), 7.61 (d, 1H, J = 8.2 Hz), 7.01 (s, 1H), 6.85 (s, 1H), 3.71 (s, 2H), 2.74 (brt, 2H, J = 7.4 Hz), 1.58–1.68 (m, 2H), 1.25–1.42 (m, 4H), 0.88 (m, 12H); 13C{1H} NMR (100 MHz, CDCl3): δ 141.4, 140.9, 137.2, 135.1, 135.0, 133.0, 132.6, 130.9, 130.3, 130.1, 128.6, 128.3, 79.9, 31.8, 31.7, 30.6, 29.5, 29.3, 26.2, 22.8, 14.3. IR (ATR) 3744, 3649, 3525, 2918, 1357, 1178, 965, 857, 825, 673, 660, cm−1.
4.6 Observation of AFM and TEM images
Polythiophene block copolymer 5, the related random copolymer 5a′, and alternating copolymer 5a′′ (10 mg), respectively, were dissolved in 0.5 mL of chloroform and cast on a glass substrate and subjected to the measurement. AFM images of the polythiophene block copolymer after thermal treatment were observed, thus the prepared glass substrate was treated at 200 °C for 20 min. For the observation of the TEM images of block copolymer 5a, 3 mg of the polymer was dissolved in 450 μL of chloroform and filtered using a 0.45 μm PTFE filter. A 50 μL solution was spin-coated on a glass substrate at 100 rpm for 1 min. After annealing at 200 °C for 20 min in air, the film of 6a was transferred to a TEM grid in a HF aqueous solution and dried in a vacuum.
4.7 SAXS measurement of block copolymer 5a
Polymer solid 5a (4 mg) was dissolved in 0.4 mL of chloroform and the solution was casted on a glass substrate. After the removal of the solvent, annealing was performed at 200 °C for 20 min. The polymer film was scratched from the glass substrate by a stainless steel scraper. The measured magnitude of the scattering vector q ranged from 0.07 to 4 nm−1, where q = 4π/λ sin(θ/2) with θ being the scattering angle. All the scattering intensity profiles were corrected for the scatterings from air and an empty cell.
4.8 Measurements of electric conductivity
The ITO-deposited glass substrate patterned as shown in Fig. 5(a) was employed for the measurement. The length of the electrode was 10 mm and the gap between electrodes was 100 μm. A solution of the polymer (10 mg) dissolved in 1 mL of chloroform was cast on the patterned substrate. The film thickness was determined by a stylus profiler as 200 nm. Measurements of conductivity at a higher voltage up to 100 V were performed in a similar manner as described in our previous report on the polythiophene homopolymer bearing a benzenesulfonate moiety at the side chain of thiophene.18 The measurement perpendicular to the substrate at a lower voltage (up to 10 V) was performed on a glass substrate as shown in Fig. 5(b), where 60 nm-thick gold electrodes with 5 nm thick chromium adhesion were deposited under a pressure of 10−4 to 10−3 Pa using a thermal evaporator with the width of 1.4 mm and the gap length between electrodes patterned as 80 μm with the thickness of 200 nm. A chloroform solution of polythiophene was cast on the substrate with the thickness of 200 nm in the glove box. The conductivity parallel to the glass substrate at a lower voltage was measured using the gold chromium electrode deposited on a glass substrate with the width of 1.4 nm. A polymer solution in chloroform (10 mg/1 mL) was cast with the thickness of 200 nm and a 45 nm-thick top gold electrode was deposited under a pressure of 10−4 to 10−3 Pa in the glove box using a thermal evaporator shown in Fig. 5(c). The current–voltage characteristics for samples shown in Fig. 5(b) and (c) were measured using a semiconductor parameter analyzer in a dry-nitrogen-filled glove box at room temperature.
4.9 Electrochemical impedance spectroscopy (EIS) measurement
The thin film electrode was prepared by spin coating. Specifically, 50 μL of copolymer solution (1 mg of copolymer in 150 μL of chloroform) was first drop cast on ITO glass. The solution-casted ITO glass was then mounted on a spin coater where the as-prepared polymer thin-film electrode was formed under a rotation speed of 1000 rpm. The thermally treated thin film electrodes were prepared by placing the as-prepared samples into a 200 °C preheated oven for 20 minutes. To minimize contact resistance between the clamps of the potentiostat cables and the as-prepared electrode, the copper tape was applied on the none-coated regions of the ITO glass to enhance the contact of the aforementioned components. Finally, to prevent the interference of copper during the electrochemical measurements, a layer of epoxy resin was applied to insulate regions of the copper tape that were immersed into the electrolyte. A conventional three-electrode system was utilized to carry out subsequent electrochemical testing in 0.5 M Li2SO4(aq) electrolyte with a Ag/AgCl reference electrode, a Pt wire counter electrode, and polymer thin-film-coated ITO as the working electrode. All measurements were conducted under Ar purged conditions to prevent the interference of dissolved oxygen. Electrochemical impedance spectroscopy (EIS) was performed at 0.6 V in a frequency range from 0.1 Hz to 300 kHz. Cyclic voltammetry was performed at 20 mV s−1 and 200 mV s−1 from 0.05 V to 1.2 V vs. RHE. To avoid the interference of the faradaic currents, the capacitance was calculated in the range of 0.5 V to 0.95 V vs. RHE. The above electrochemical techniques were employed using a Biologic SP-200 potentiostat.
Author contributions
A. M. conceived the ideas and designed the project. S. Y. performed all of the experimental works. R. Y., C. K., and K. O. contributed to the synthesis and characterization of polymers. M. K. and M. F. performed the measurement of electric conductivities. S.-C. Y., Y.-T. P., and M. H. performed TEM and electrochemical measurements. T. S., H. M., and H. M. supported thin-film formation and AMF measurements. All authors discussed the results and contributed to the interpretation of data. A. M. wrote the manuscript with contributions from all authors.
Conflicts of interest
There are no conflicts to declare.
Acknowledgements
This work was partially supported by JSPS Kakenhi B by the MEXT (JP19182273) and Kobe University Strategic International Collaborative Research Grant (Type B Fostering Joint Research). We thank Edanz (https://jp.edanz.com/ac) for editing the draft of this manuscript. We appreciate Professor Hsin-Lung Chen and Meng-Zhe Chen of National Tsing Hua University for valuable discussion on the morphology of polythiophene thin films and measurements of SAXS by synchrotron radiation and Professor Takashi Nishino of Kobe University for discussions on XRD measurements and thermal analyses.
References
- H. Sirringhaus, N. Tessler and R. H. Friend, Integrated Optoelectronic Devices Based on Conjugated Polymers, Science, 1998, 280, 1741–1744 CrossRef CAS PubMed
.
- A. C. Grimsdale, K. Leok Chan, R. E. Martin, P. G. Jokisz and A. B. Holmes, Synthesis of Light-Emitting Conjugated Polymers for Applications in Electroluminescent Devices, Chem. Rev., 2009, 109, 897–1091 CrossRef CAS PubMed
.
- P. M. Beaujuge and J. M. J. Fréchet, Molecular Design and Ordering Effects in π-Functional Materials for Transistor and Solar Cell Applications, J. Am. Chem. Soc., 2011, 133, 20009–20029 CrossRef CAS PubMed
.
- I. Osaka and R. D. McCullough, Advances in Molecular Design and Synthesis of Regioregular Polythiophenes, Acc. Chem. Res., 2008, 41, 1202–1214 CrossRef CAS PubMed
.
- T. Yokozawa and Y. Ohta, Transformation of Step-Growth Polymerization into Living Chain-Growth Polymerization, Chem. Rev., 2016, 116, 1950–1968 CrossRef CAS PubMed
.
- T. Yokozawa and A. Yokoyama, Chain-Growth Condensation Polymerization for the Synthesis of Well-Defined Condensation Polymers and π-Conjugated Polymers, Chem. Rev., 2009, 109, 5595–5619 CrossRef CAS PubMed
.
- S. Tamba, K. Shono, A. Sugie and A. Mori, C–H Functionalization Polycondensation of Chlorothiophenes in the Presence of Nickel Catalyst with Stoichiometric or Catalytically Generated Magnesium Amide, J. Am. Chem. Soc., 2011, 133, 9700–9703 CrossRef CAS PubMed
.
- S. Tamba, R. Fujii, A. Mori, K. Hara and N. Koumura, Synthesis and Properties of Seleno-analog MK-organic Dye for Photovoltaic Cells Prepared by C-H Functionalization Reactions of Selenophene Derivatives, Chem. Lett., 2011, 40, 922–924 CrossRef CAS
.
- Y. Shibuya and A. Mori, Dehalogenative or Deprotonative? The Preparation Pathway to the Organometallic Monomer for Transition-Metal-Catalyzed Catalyst-Transfer-Type Polymerization of Thiophene Derivatives, Chem. – Eur. J., 2020, 26, 6976–6987 CrossRef CAS PubMed
.
- A. Mori, Transition Metal-catalyzed Bond-forming Reactions at the C-H Bond of Heteroaromatic Compounds, J. Synth. Org. Chem., Jpn., 2011, 69, 1202–1211 CrossRef CAS
.
- A. Mori, Structure and functionality-based molecular design of azoles and thiophenes, Bull. Chem. Soc. Jpn., 2020, 93, 1200–1212 CrossRef CAS
.
- M. Chayer, K. Faïd and M. Leclerc, Highly Conducting Water-Soluble Polythiophene Derivatives, Chem. Mater., 1997, 9, 2902–2905 CrossRef CAS
.
- H. Goto and E. Yashima, Electron-Induced Switching of the Supramolecular Chirality of Optically Active Polythiophene Aggregates, J. Am. Chem. Soc., 2002, 124, 7943–7949 CrossRef CAS PubMed
.
- X. M. Hong, J. C. Tyson and D. M. Collard, Controlling the Macromolecular Architecture of Poly(3-alkylthiophene)s by Alternating Alkyl and Fluoroalkyl Substituents, Macromolecules, 2000, 33, 3502–3504 CrossRef CAS
.
- K. Fujita, Y. Sumino, K. Ide, S. Tamba, K. Shono, J. Shen, T. Nishino, A. Mori and T. Yasuda, Synthesis of Poly(3-substituted thiophene)s of Remarkably High Solubility in Hydrocarbon via Nickel-Catalyzed Deprotonative Cross-Coupling Polycondensation, Macromolecules, 2016, 49, 1259–1269 CrossRef CAS
.
- A. Mori, K. Fujita, C. Kubota, T. Suzuki, K. Okano, T. Matsumoto, T. Nishino and M. Horie, Formal preparation of regioregular and alternating thiophene–thiophene copolymers bearing different substituents, Beilstein J. Org. Chem., 2020, 16, 317–324 CrossRef CAS PubMed
.
- S. Berson, S. Cecioni, M. Billon, Y. Kervella, R. de Bettignies, S. Bailly and S. Guillerez, Effect of carbonitrile and hexyloxy substituents on alternated copolymer of polythiophene-Performances in photovoltaic cells, Sol. Energy Mater. Sol. Cells, 2010, 94, 699–708 CrossRef CAS
.
- A. Mori, C. Kubota, K. Fujita, M. Hayashi, T. Ogura, T. Suzuki, K. Okano, M. Funahashi and M. Horie, Thermally Induced Self-Doping of π-Conjugated Polymers Bearing a Pendant Neopentyl Sulfonate Group, Macromolecules, 2020, 53, 1171–1179 CrossRef CAS
.
- V. Hirschberg, L. Faust, D. Rodrigue and M. Wilhelm, Effect of Topology and Molecular Properties on the Rheology and Fatigue Behavior of Solid Polystyrene/Polyisoprene Di- And Triblock Copolymers, Macromolecules, 2020, 53, 5572–5587 CrossRef CAS
.
- X. Yu, K. Xiao, J. Chen, N. V. Lavrik, K. Hong, B. G. Sumpter and D. B. Geohegan, High-performance field-effect transistors based on polystyrene-b-poly(3- hexylthiophene) diblock copolymers, ACS Nano, 2011, 5, 3559–3567 CrossRef CAS PubMed
.
- Y. Tian, K. Watanabe, X. Kong, J. Abe and T. Iyoda, Synthesis, Nanostructures, and Functionality of Amphiphilic Liquid Crystalline Block Copolymers with Azobenzene Moieties, Macromolecules, 2002, 35, 3739–3747 CrossRef CAS
.
- M. Komura and T. Iyoda, AFM Cross-Sectional Imaging of Perpendicularly Oriented Nanocylinder Structures of Microphase-Separated Block Copolymer Films by Crystal-like Cleavage, Macromolecules, 2007, 40, 4106–4108 CrossRef CAS
.
- A. Mori, J. Shikuma, M. Kinoshita, T. Ikeda, M. Misaki, Y. Ueda, M. Komura, S. Asaoka and T. Iyoda, Controlled Homeotropic and Homogeneous Orientations for Nanoscale Phase-separated Domain of Light-emitting Amphiphilic Block Copolymer Bearing a 2,5-Diarylthiazole Moiety, Chem. Lett., 2008, 37, 272–273 CrossRef CAS
.
- Y. Takeoka, Y. Iguchi, M. Rikukawa and K. Sanui, Self-assembled multilayer films based on functionalized poly(thiophene)s, Synth. Met., 2005, 154, 109–112 CrossRef CAS
.
- K. Umezawa, T. Oshima, M. Yoshizawa-Fujita, Y. Takeoka and M. Rikukawa, Synthesis of Hydrophilic–Hydrophobic Block Copolymer Ionomers Based on Polyphenylenes, ACS Macro Lett., 2012, 1, 969–972 CrossRef CAS PubMed
.
- H.-R. Tseng, H. Phan, C. Luo, M. Wang, L. A. Perez, S. N. Patel, L. Ying, E. J. Kramer, T.-Q. Nguyen, G. C. Bazan and A. J. Heeger, High-Mobility Field-Effect Transistors Fabricated with Macroscopic Aligned Semiconducting Polymers, Adv. Mater., 2014, 26, 2993–2998 CrossRef CAS PubMed
.
- Y. Nagao and J. Matsui, Anisotropic Proton Conductivity of Poly(aspartic acid) Thin Films, Mater. Today Proc., 2019, 17, 953–958 CrossRef CAS
.
- X. M. Hong and D. M. Collard, Liquid Crystalline Regioregular Semifluoroalkyl-Substituted Polythiophenes, Macromolecules, 2000, 33, 6916–6917 CrossRef CAS
.
- A. Mori, C. Kubota, D. Morita, K. Fujita, S. Yamamoto, T. Suzuki, K. Okano, M. Funahashi and M. Horie, Thermally-Induced Doping of the Regioregular Polythiophene Bearing Alkylene Spacered Benzene sulfonate Group at the Side Chain, Heterocycles, 2021, 103, 249–257 CrossRef PubMed
.
- Y.-H. Lee, Y.-L. Yang, W.-C. Yen, W.-F. Su and C.-A. Dai, Solution self-assembly and phase transformations of form II crystals in nanoconfined poly(3-hexyl thiophene) based rod-coil block copolymers, Nanoscale, 2014, 6, 2194 RSC
.
- C.-A. Dai, W.-C. Yen, Y.-H. Lee, C.-C. Ho and W.-F. Su, Facile Synthesis of Well-Defined Block Copolymers Containing Regioregular Poly(3-hexyl thiophene) via Anionic Macroinitiation Method and Their Self-Assembly Behavior, J. Am. Chem. Soc., 2007, 129, 11036–11038 CrossRef CAS PubMed
.
- Y.-H. Lee, W.-C. Yen, W.-F. Su and C.-A. Dai, Self-assembly and phase transformations of π-conjugated block copolymers that bend and twist: from rigid-rod nanowires to highly curvaceous gyroids, Soft Matter, 2011, 7, 10429 RSC
.
- J. Bartuš, Electrically Conducting Thiophene Polymers, J. Macromol. Sci., Part A: Pure Appl. Chem., 1991, 28, 917–924 CrossRef
.
- T. Ghosh, S. Nagasawa, N. Raveendran, V. Darshan, A. Saeki and V. C. Nair, Preferential Face-on and Edge-on Orientation of Thiophene Oligomers by Rational Molecular Design, Chem. – Asian J., 2019, 14, 963–967 CrossRef CAS PubMed
.
- S. Y. Son, T. Park and W. You, Understanding of Face-On Crystallites Transitioning to Edge-On Crystallites in Thiophene-Based Conjugated Polymers, Chem. Mater., 2021, 33, 4541–4550 CrossRef CAS
.
- S. N. Patel, A. E. Javier and N. P. Balsara, Electrochemically Oxidized Electronic and Ionic Conducting Nanostructured Block Copolymers for Lithium Battery Electrodes, ACS Nano, 2013, 7, 6056–6068 CrossRef CAS PubMed
.
- A. H. Stoll and P. Knochel, Preparation of Fully Substituted Anilines for the Synthesis of Functionalized Indoles, Org. Lett., 2008, 10, 113–116 CrossRef CAS PubMed
.
- W. A. Herrmann and C. Köcher, N-Heterocyclic Carbenes, Angew. Chem., Int. Ed. Engl., 1997, 36, 2162–2187 CrossRef CAS
.
- R. Inoue, M. Yamaguchi, Y. Murakami, K. Okano and A. Mori, Revisiting of Benzophenone Ketyl Still: Use of a Sodium Dispersion for the Preparation of Anhydrous Solvents, ACS Omega, 2018, 3, 12703–12706 CrossRef CAS PubMed
.
Footnote |
† Electronic supplementary information (ESI) available: Experimental details, further details on microscopic analyses, SAXS analyses, electric conductivity measurements, and electrochemical analyses. See DOI: https://doi.org/10.1039/d2tc05454j |
|
This journal is © The Royal Society of Chemistry 2023 |
Click here to see how this site uses Cookies. View our privacy policy here.