DOI:
10.1039/D2TC02639B
(Review Article)
J. Mater. Chem. C, 2023,
11, 48-96
Recent progress in Ce3+/Eu2+-activated LEDs and persistent phosphors: focusing on the local structure and the electronic structure
Received
23rd June 2022
, Accepted 26th October 2022
First published on 2nd November 2022
Abstract
Ce3+/Eu2+ activated luminescent materials offer a versatile platform for precise emission light manipulation through structure control on the basis of the composition–structure–property correlations. To date, Ce3+/Eu2+ activated phosphors have been well developed as an indispensable component in the lighting industry and display systems due to their superior performance. Meanwhile, many persistent phosphors contain Ce3+/Eu2+ together with other lanthanide or transition-metal co-dopants. It is therefore of great importance to focus on their similarities and gain insight into the interplay effect of the local structure and electronic structure on emission peak modulation and persistent duration elongation. Here, we review the theoretical and experimental progress in the discovery and optimization of Ce3+/Eu2+ activated LEDs and persistent phosphors. The Dorenbos model on f–d transitions and the latest developments in the correlation of the local structure and luminescence characteristics are elaborated to give an overall vision on the composition–structure–property correlations in Ce3+/Eu2+-activated phosphors. Particular attention is devoted to highlighting the critical role of the electronic structure in tuning the properties of phosphors. The development and optimization routines of some typical phosphors are expounded, with an emphasis on phosphor design principles, aiming at providing inspirations for tailoring and optimizing the properties of Ce3+/Eu2+-activated phosphors toward specific applications. Finally, we propose an outlook toward potential theory developments and future material discovery.
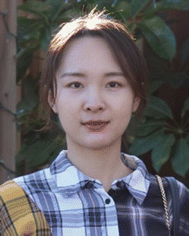
Shuxin Wang
| Shuxin Wang (S. X. Wang) received his PhD degree in Materials Science and Engineering from the University of Science and Technology Beijing (USTB) in 2021. From Dec. 2019 to Jan. 2021, she studied in Prof. Seshadri Ram's group at the University of California, Santa Barbara, as a visiting scholar. Now, she is a postdoc in Prof. Wai-yeung Wong's group in the Department of Applied Biology and Chemical Technology at The Hong Kong Polytechnic University. Her current research interests are focused on luminescent materials. |
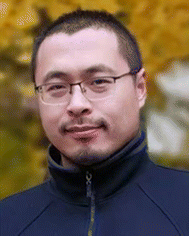
Zhen Song
| Zhen Song (Z. Song) received his PhD degree in Materials Science and Engineering from the University of Science and Technology Beijing (USTB) in 2014. He continued to work as a postdoc in Prof. Q. L. Liu's group until Dec. 2016. Now, he is an associate professor in USTB. From Dec. 2017 to Aug. 2018, he stayed in Prof Luis Seijo's group at Universidad Autónoma de Madrid (Spain) as a visiting scholar. His current research interests are focused on theoretical aspects of luminescent materials. |
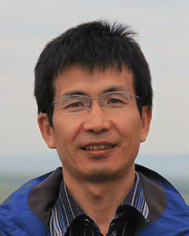
Quanlin Liu
| Quanlin Liu (Q. L. Liu) completed his PhD degree in Condensed Matter Physics in 1998 at the Institute of Physics, Chinese Academy of Science (IOP CAS). From 1998–2005, he worked as an assistant and associate professor at IOP CAS, including working as a JSPS fellow at the National Institute for Materials Science, Japan (2001–2003). Since then, he has worked as a full professor in Materials Science at the University of Science and Technology Beijing (USTB) (2005–now). His current research interests mainly concern luminescent materials. |
Introduction
Phosphor-converted light emitting diodes (pc-LEDs) have made their mark in the lighting industry and display systems as an indispensable solid-state light source driven by their unique properties including high luminescence efficiency, small volume, long lifetime, fast switching, and excellent durability.1–6 The concept of pc-LEDs, assembling blue/violet LED chips with phosphors to partially down-convert some of the blue/violet emission to longer wavelengths corresponding to colors such as green, yellow, and red, can be traced back to Bando's work in 1996,7 shortly after candela-class blue LEDs were first reported.8 The first commercially available pc-LED device was invented by Nichia Corporation, utilizing yellow emitting YAG:Ce (a cerium-doped yttrium aluminum garnet; Y3Al5O12:Ce3+) overcoated onto a blue InGaN LED chip.9 Since then, the pc-LED field really took off, and various fabrication strategies for creating white light were proposed.10 Meanwhile, tremendous attention has been focused on exploring high performance phosphors for white LED (w-LED) and LED backlights. Phosphors typically use optically active lanthanide or transition-metal ions as emission centers embedded in an inorganic matrix. The general requirements for appropriate LED phosphors include the following: (i) a broad excitation spectrum matching well with the emission spectrum of a LED chip; (ii) a suitable emission spectrum; (iii) high quantum efficiency; (iv) small thermal luminescence quenching; and (v) high chemical stability.11–13 Many of these requirements have been met by the discovery and development of new phosphors over the past decades including a variety of Ce3+/Eu2+-activated silicate,14,15 aluminate,16,17 nitride etc.,18,19 leading to commercial LEDs that cover a full range of white CCTs (correlated color temperatures) (Fig. 1a). The parity-allowed electric dipole f–d transitions of Ce3+ and Eu2+ are of particular interest due to their unique luminescence properties including a broad emission band and high efficiency.20–22 Besides its high performance, the strong interaction of the 5d-electron with the neighboring anion ligands in the host lattice not only grants access to tune the luminescence properties by structural modulation but also provides feasibility to design new phosphors based on composition–structure–property correlations, which forecasts great potential for more “smart” pc-LED devices.
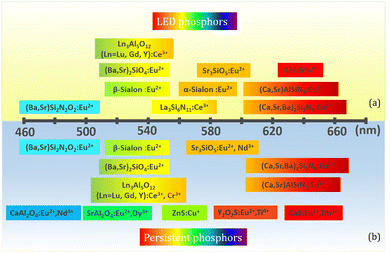 |
| Fig. 1 (a) Commercial LED phosphors for w-LEDs using blue chips. (b) Important persistent phosphors with promising or potential PersL properties. | |
On the basis of LED phosphors, by further introducing appropriate trap centers that can capture excited electrons and then release them by thermal assistance, mysterious persistent luminescence (PersL) can be achieved.23 Compared with LED phosphors, persistent phosphors have distinctive properties which can give continuous luminescence for seconds, minutes, hours, or even days after ceasing the stimulations.24 Owing to their unique optical performance, persistent phosphors are emerging as promising materials for safety signage, in vivo bioimaging, and energy storage applications in the past decades.25–28 The PersL materials have a rich and longstanding history that can be traced back to as early as 1000 years ago, when they were first used as special “night-vision” inks for ancient Chinese paintings.29 The landmark breakthrough occurred in 1996, when Murayama et al. reported the green persistent phosphor SrAl2O4:Eu2+,Dy3+ with extremely bright and long duration PersL in the dark (over 30 h before the emission intensity drops to 0.32 mcd m−2, 100 times higher than the sensitivity of the dark-adapted human eyes).30 Since then, research on persistent phosphors has become increasingly popular. Extensive studies have been devoted to discover new PersL materials, tune their properties, and explore the fundamental mechanism, aiming at broadening the application scope and realizing the ultimate “dream” of night lighting. To date, a variety of persistent phosphors have been discovered, and some important ones with promising or potential PersL properties are presented in Fig. 1b. It is worth noting that the majority of the persistent phosphors are originally from conventional phosphors. By controlling defect concentration and intentionally introducing aliovalent or isovalent co-dopants as trap centers, their intensities and afterglow durations can be effectively tuned, such as Y3Al2Ga3O5:Ce3+,Yb3+ and M2Si5N8:Eu2+,Tm3+ (M = Ca, Sr).31–33 The potential PersL possibilities of LED phosphors make them particularly interesting for the discovery of new promising PersL materials.
Generally, luminescence is determined by the average and local crystal structures of phosphors. Unlike band-to-band optical transitions, f–d transitions from isolated luminescent centers such as Ce3+/Eu2+ are strongly influenced by the coordinated crystalline environment.20 Within the local structure, energy levels of Ce3+/Eu2+ may be split and shifted, resulting in peak shifts observed in both photoluminescence (PL) and photoluminescence excitation (PLE) spectra.34,35 So, it is necessary to deeply understand the relationship between the local structure and luminescence. Recently, Xia's group developed several Eu2+-doped oxide phosphors, such as NaLi3SiO4:Eu2+, KSrScSi2O7:Eu2+, and Sr2Li(Al,Ga)O4:Eu2+. Through gaining insight into the local structure, the luminescence mechanism was well explained, and the luminescence properties were successfully engineered at will via local structure modification.36–46 On the other hand, the luminescent properties of materials are strongly dependent on their electronic structures; while the electronic structure is mainly determined by the crystal structure. To fully understand the luminescence, especially the thermal luminescence quenching behavior, it is necessary to investigate the electronic structure of rare-earth-doped luminescent materials. Recently, Dorenbos has proposed a method to construct the host referred binding energy (HRBE) and vacuum referred binding energy (VRBE) schemes, showing all the information regarding lanthanide energy levels and host bands.21,47,48 The electronic structure based on VRBE schemes is of vital importance to deeply understand Ce3+/Eu2+-activated LEDs and persistent phosphors.
The main object of this review is to provide a detailed and deep understanding of well-known Ce3+/Eu2+-activated LEDs and persistent phosphors, from the view point of the local structure and the electronic structure, to highlight the important design considerations for new high-performance phosphors and PersL materials. As we focus on the composition–structure–property correlations, we first introduce basic theory on f–d transitions to specify how the chemistry and structure affect the luminescence properties. Then the PersL mechanism for persistent phosphors is illustrated, which is followed by demonstrating the electronic structure diagram as a powerful design tool towards promising properties. We continue the discussion on the widely used LED phosphors. Based on the crystal structure, we analyze their luminescence properties, ascribe physical quantities to structural features (crystal and electronic structure) and extract the composition–structure–property correlations. A parallel focus is placed on the progress of converting LED phosphors into persistent phosphors by structure modification by virtue of electronic structure engineering. We conclude with a brief discussion on the prerequisites for LED and persistent phosphors and provide an outlook toward promising research directions and suggestions for future developments.
Basic theory on Ce3+/Eu2+-activated phosphors and persistent luminescence
Structural features are critical to physical properties. Understanding and developing the composition–structure–property correlations are the core of materials science, which lead mankind to materials revolution from time to time. In the past decade, scientific advances in experiments have enabled abundant theoretical insights into the rational design of high performance Ce3+/Eu2+-activated inorganic luminescent materials. Experimental techniques providing information about the local coordination environment have been developed, such as high-resolution transmission microscopy (FRTEM),49 extended X-ray absorption fine structure (EXAFS),50 solid-state nuclear magnetic resonance51 and Mössbauer spectroscopy.52 It has been widely acknowledged that luminescence properties are determined by the average and local structures of phosphors. Unlike band-to-band optical transitions, f–d transitions from the isolated luminescent center Ce3+/Eu2+ are strongly influenced by the coordinated crystalline environment, under which energy levels of Ce3+/Eu2+ may be split and shifted, resulting in their highly tunable luminescence properties. On the other hand, the electronic structures, deriving from the crystal structure, with information regarding dopant energy levels and host bands have superiority in gaining insight into the thermal luminescence stability and PersL properties. We discuss here the intrinsic properties of Ce3+/Eu2+-activated LED and persistent phosphors, and the effects of the host crystal and the dopant ions on phosphor optical properties.
Dorenbos model on f–d transition
In general, the luminescence process of Ce3+/Eu2+-doped phosphor can be divided into energy absorption, non-radiative transition and photon emission.53,54 Upon excitation, absorption occurs due to the transitions from the 4f ground state to the 5d excited states. Then, due to the coupling of the 5d electron with lattice phonons, partial energy is lost by non-radiative transition. Finally, emission occurs by the transition from the lowest 5d excited state to the 4f ground state. Therefore, the relative energy difference between the 4f and 5d levels dictates the excitation energy. Different from the invariable energy levels of 4f orbitals shielded by the filled 5s25p6 sub-shells, the energy levels of unshielded 5d electron can be tuned by tens of thousands of wave numbers from one compound to another benefiting from their subtle response to the host lattice, resulting in their crucial effect on excitation properties.20 The effect of the host lattice on 5d energy levels is typically characterized by two parameters, i.e., the centroid shift εc and the crystal field splitting εcfs, as illustrated in Fig. 2. Please note that Fig. 2 is a simplified schematic plot. Actually, for Eu2+ with seven 4f electrons, the situation is more complicated due to its more excited states. The details could be found in ref. 55. In the host, the 5d levels shift toward lower energy due to a decrease in the interelectron repulsion. Moreover, the degenerate 5d levels split into at most five different 5d states depending on the site symmetry for the activator ion. The downshift of the average energy of the five 5d levels is the centroid shift εc and the energy difference between the lowest 5d1 and the highest 5d5 energy levels defines the crystal field splitting εcfs.56,57 The overall effect of centroid shift εc and crystal field splitting εcfs leads to a decrease in energy difference between the 5d and 4f levels, directly determining the excitation energy. Based on the excitation energy, the emission energy is further determined by the Stokes shift ΔS, defined as the energy difference between the absorption and emission maxima of the same electronic transition, resulting from the surrounding lattice relaxation due to the coupling of the 5d electron with the lattice phonons upon the excitation of the activator from the 4f to the 5d configuration.58,59 In the past few decades, a series of sound theories have been established to gain insight into the composition–structure–property correlations and account for the physical nature behind them. With the rapid development of first principles calculation, some theoretical models have been established to finely understand the luminescence properties. In addition, as an increasing number of phosphors were developed, the effects of host crystal on the luminescence properties have been further qualitatively or quantitatively demonstrated by analyzing the f–d luminescence properties in more than 300 Ce3+/Eu2+-activated phosphors by virtue of the phenomenological approach proposed by Dorenbos.20 Detailed analysis and results are summarized below.
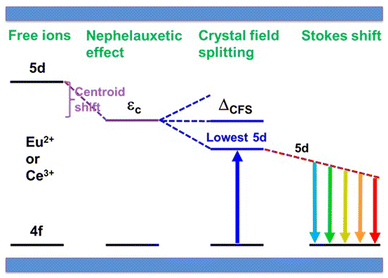 |
| Fig. 2 A schematic energy level diagram for Ce3+/Eu2+ shows the effects of the host crystal, including the centroid shift, crystal field splitting and Stokes shift. Such effects lead to an overall decrease in the energy difference between the 5d and 4f levels. Reproduced with permission from ref. 60, copyright 2017 American Chemical Society. | |
Centroid shift.
Once Ce3+/Eu2+ ions are introduced into a crystalline host, the average position of the 5d levels of Ce3+/Eu2+, i.e., the centroid, is lowered relative to that of the free ion. The downward shift of the centroid position of the five 5d-levels relative to that of the free ion is defined as the centroid shift εc. The centroid shift is commonly associated with the expansion of the charge cloud, i.e., the nephelauxetic effect, which is often attributed to the covalency between the 5d orbital and the p-orbitals of the anions. The covalency effect results in a larger average distance and hence a reduced Coulomb repulsion between the electrons of the lanthanide cation, giving rise to the centroid shift phenomenon.61 In 1980 Morrison first suggested a physical origin for the centroid shift involving ligand polarization, addressing the vital contribution of the correlated motion of 5d electron and anion ligand electrons to the centroid shift.62 The instantaneous position of the metal electron polarizes the surrounding ligands, which reacts back on the metal electron itself. Essentially a self-induced potential is generated that reduces the interelectron Coulomb repulsion between the metal electrons. It leads, like the covalency effect, to a lowering of the centroid energy. Models on covalency predict in the first order that a centroid shift is proportional to the S ≡ 〈ϕM|ψL〉 square of the overlap integral between the metal (M) and ligand (L) orbital as shown in the following formula:61,63 | 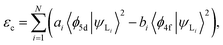 | (1) |
where ai and bi are appropriate constants. From the perspective of ligand polarization model, based on the model suggested by Morrison62 and Aull and Jenssen,61 centroid shift εc can in first approximation be written as | 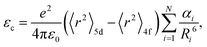 | (2) |
where r represents the radial position of the electron in either 5d or 4f orbital, and 〈r2〉 is the expectation value of r2, αi is the polarizability of the ligand i located at a distance Ri from the metal ion, e is the elementary charge, and ε0 is the permittivity of vacuum. The summation is over all the N nearest coordinating anion ligands. As shown in Fig. 3a, it is experimentally observed that the centroid shift tends to increase following the nephelauxetic sequence of the anion and to decrease following the nephelauxetic sequence of the most electronegative cation (the neighboring small cation of the lanthanide ion) in the compounds as shown below, proposed by Dorenbos64 |  | (3) |
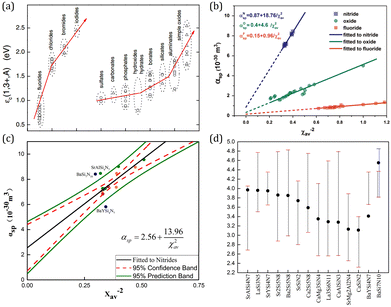 |
| Fig. 3 (a) The centroid shift for Ce3+ 5d configuration in halides and oxide compounds. Reproduced with permission from ref. 64, copyright 2012 The Electrochemical Society. (b) Spectroscopic polarizability against the inverse square of the average cation electronegativity in Ce3+ doped nitrides, oxides, and fluorides. Reproduced with permission from ref. 65, copyright 2015 Elsevier B.V. (c) The revised correlation between the spectroscopic polarizability and the average cation electronegativity in Ce3+ doped nitrides. (d) The relative energy of the 5d level centroid and the lowest and highest 5d levels in Ce3+ doped nitrides. Panels c and d reproduced with permission from ref. 66, copyright 2018 Elsevier B.V. | |
It can be understood from the viewpoint of charge cloud attraction, which can be comprehended as a form of bonding that increases the binding energy of the anion electrons. A weaker nephelauxetic effect of the anion ligand leads to the stronger binding with the lanthanide ion, which implies a larger oscillation force constant and therewith smaller anion polarizability, resulting in a smaller centroid shift according to eqn (2). A stronger nephelauxetic effect of the neighboring cation (the second nearest atom) leads to a stronger binding with the anion ligand, which also reduces the covalency between the ligand charge cloud and the 5d orbital of lanthanide ions behaving as a more ionic surrounding. In this case eqn (1) predicts a smaller centroid shift. Overall, strong binding lowers the electron donating power of the anion ligand toward the lanthanide ion, which is the original interpretation for not only the centroid shift but also the nephelauxetic effect.
The centroid shift has been investigated by Dorenbos using a phenomenologically parameterized model with the inclusion of spectroscopic polarizability, αsp, which merges together the effect of (1) ligand polarization, (2) covalency, and (3) possible charge cloud expansion.35 On the basis of the assumption that (1) the total centroid shift is the result of the added contribution of each coordinating anion individually; (2) only the nearest-neighbor anions give a significant contribution to the centroid shift; and (3) polarizability of all the ligands is identical, eqn (2) can be re-written as67
| 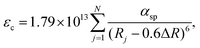 | (4) |
where
Rj (pm) is the distance from Ce
3+ to the anion
j in the unrelaxed lattice. The summation is overall N coordination anions of Ce
3+. Δ
R ≡
RM −
RLn, where
RM is the ionic radius of the cation which is replaced by Ce
3+ with the ionic radius
RLn. 0.6Δ
R is an estimation of the bond length relaxation. The most appealing aspect of this model is that it is a zero-parameter model, and it provides a direct quantitative and qualitative physical interpretation of the centroid shift. Pearson's work reported a proportional relationship between the force constant
K of the metal–hydride bond in diatomic MH molecules and the value for the electronegativity
χ of the metal atom.
68 Since in atomic physics the polarizability
α of an atom is related to the binding force constants
Ki of its electrons as

, the correlation between spectroscopic polarizability
αsp and the average cation electronegativity
χav was constructed as follows:
35 | 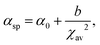 | (5) |
where
α0 is the limiting polarizability in the case of very large
χ, and
b represents the susceptibility of the anion to change its polarizability due to the bonding with the metal ion. The average cation electronegativity
χav is defined as follows:
|  | (6) |
where
ni is the number of the cation
i with charge
zi and electronegativity
χi in the chemical formula.
On the basis of the theory above, employing the phenomenological approach, Dorenbos obtained the quantitative correlations between αsp and χav as shown in Fig. 3b by analyzing the spectral and structural data in Ce3+-activated oxide and fluoride compounds.57,64,69 Over the past few decades, Ce3+/Eu2+-activated nitride and oxonitride phosphors have attracted tremendous attention due to their unique luminescence properties and potential application in pc-LEDs.70–73 The bonding in nitrides is significantly more covalent than that in oxides, because of the higher formation energy of N3− from atomic N (+2300 kJ mol−1) than that of O2− from atomic O (+700 kJ mol−1).74 Compared with oxide hosts, nitride and oxonitride hosts enable a more downward shift of 5d energy levels, giving rise to a large centroid shift εc. In this way Ce3+/Eu2+-activated nitride phosphors can absorb blue LED radiation and then emit visible light effectively, which are promising for pc-LED applications. In addition, nitridosilicates and their derivatives, as an important class of nitrides, typically consist of SiN4 tetrahedra, in which a partial nitrogen/silicon can be substituted by oxygen/aluminum to form Si/Al[O/N]4 tetrahedra.75 These tetrahedron units stack together by corner sharing to form the condensed framework, generally contributing to the chemical and thermal luminescence stability of nitridosilicates.76 Furthermore, these tetrahedron units take various stacking topologies providing Ce3+/Eu2+ with abundant coordination environments, allowing the Ce3+/Eu2+-activated nitridosilicate phosphors to be highly tunable. With the aim of approaching a guideline for further development of Ce3+/Eu2+-activated nitridosilicate phosphors, our group works on exploring the αsp and χav correlations in Ce3+-activated nitrides based on Dorenbos’ work.65 As expected from the nephelauxetic effect, the tendency of bF < bO < bN can be observed as demonstrated in Fig. 3b, which means that αsp is increasingly sensitive to χav in the sequence of fluorides, oxides and nitrides. This sequence provides a guideline for phosphor design that the larger εc and thereby the redshift of the spectrum can be realized by the substitution of the anion with a stronger nephelauxetic effect. The structural coordination data, as the critical structure parameter, play a vital role in studying the composition–structure–property correlations, such as the εc analysis. However, the determination of coordination number for Wyckoff sites in the crystal structure lacks explicit criteria, which results in that the coordination number of the cations in some compounds is ambiguous and sometimes there are conflicts for coordination number in different reports regarding certain compound.18,19,77–80 In order to clear it up, our group further proposed a uniform standard to determine the coordination number of cations in nitrides according to the bond valence theory and the requirement for stability of the coordination polyhedron.66 In general, the nitrogen anions which contribute more than 4% to the bond valence of the central cation are considered as the coordination atom. The threshold value can be adjusted on a small scale in order to obtain a higher stability of the coordination polyhedron. Based on the revised structural data, supplementing data in the latest nitride phosphors, our group re-investigated εc of the 5d configuration of Ce3+ in the nitride compounds. The updated correlation between αsp and χav is displayed in Fig. 3c, the credibility of which is ensured by the crystal field splitting εcfs data as shown in Fig. 3d. With the above results, one can predict the 5d centroid shift of Ce3+ in phosphors based on the composition and structure of the compounds.
Nevertheless, we would like to remind the readers of the overestimation of the centroid shift in garnet series, as pointed out by Seijo and Barandiarán.81 They performed spin–orbit coupling, relativistic, embedded cluster, wave function-based ab initio calculations on the (CeO8)13− cluster under the effects of the embedding potentials of garnets and obtained significantly smaller centroid-shift values with regard to those estimated using the Dorenbos model. Since the ab initio results are more advocated by the garnet family, the completeness of the Dorenbos’ model needs further improvement by elucidating some coefficients.
It is worth noting that the research on εc of the 5d configuration of Eu2+ is missing, which is caused by the difficulty in the identification of the transition energy from the 4f7 ground state to the five 4f65d excited states. In the f–d excitation spectra of Eu2+ always about 0.8 eV-wide bands appear because the 6 electrons remaining in the 4f-shell may occupy one of the seven 7FJ states that spread about 0.6 eV in energy.82,83 They overlap with the transitions to the 4f65d2 and higher 4f65di states, smearing out into a featureless 1 eV broad band, which makes it difficult to identify the energy of the five f–d transitions for developing εc theory in Eu2+-activated phosphors. In a recent paper, Joos et al. applied state-of-the-art multiconfigurational ab initio embedded-cluster methods to investigate the staircase structure in more detail.55 Other than the decoupled scheme, which attributes the fine structure to the J = 0–6 levels of the 4f6 (7FJ) subshell, it is the interplay between the spin–orbit coupling within the 4f6 (7FJ) subshell and the 4f–5d exchange splitting that presents the fine structure.
Crystal field splitting.
Ce3+/Eu2+-activated phosphors have the advantage of parity-allowed electric dipole f–d transition, giving rise to their broad and highly efficient emission. The crystal field splitting εcfs of 5d level further broadens the excitation spectra, which allows that Ce3+/Eu2+-activated phosphors generally could be excited by lower energy irradiation such as blue light. Crystal field splitting is caused by Coulomb interactions between the 5d electron and anion ligands. By analyzing the luminescence and structural data of more than 60 Ce3+/Eu2+-activated phosphors including halide, oxide, sulfide, and selenide compounds, Dorenbos revealed that the crystal field splitting εcfs is related to the polyhedral shape and size, while irrelevant to the types of the anions, as shown in Fig. 4a and b.34 For the compounds with the same shape of coordination polyhedron around Ce3+/Eu2+, the crystal field splitting appears to behave as follows:where βQpoly is a constant determined by the type of the coordination polyhedron. Rav is defined as | 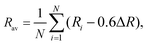 | (8) |
where Ri is the individual bond length of the N coordinating anions in the unrelaxed lattice. ΔR ≡ RM − RLn, where RM is the ionic radius of the cation which is replaced by the lanthanide Ln with an ionic radius RLn. 0.6ΔR is an estimation of the bond length relaxation. By fitting the data in Ce3+/Eu2+-activated phosphors with 6-fold octahedral (octa), 8-fold cubal (cubal), and 12-fold cuboctahedral (cubo) coordination through eqn (7), βpoly was obtained. As an example, βocta is 1.36 × 105 eV pm2 for Eu2+, and βocta
:
βcubal
:
βcubo equals 1
:
0.89
:
0.44 for both Ce3+ and Eu2+. It is revealed that higher coordination number tends to reduce the crystal field splitting, while the type of the anion, whether it is F, Cl, Br, I, O, S or Se, does not matter at all.34 Furthermore, comparing the Eu2+εcfs data with the Ce3+ data shows that84 | βpoly2+(Eu2+) = 0.81βpoly3+(Ce3+). | (9) |
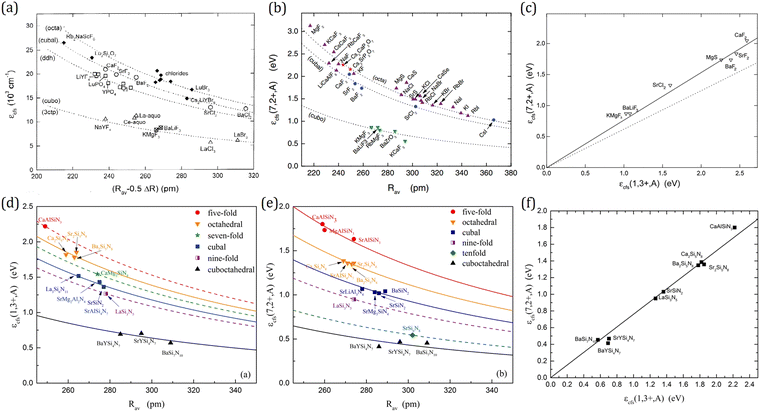 |
| Fig. 4 The crystal field splitting of the 5d configuration of (a) Ce3+ and (b) Eu2+ and (c) their correlation in phosphors including halide, oxide, sulfide, and selenide compounds. Panels a–c reproduced with permission from ref. 34, 64, 84, copyright 2002 Elsevier, 2012 The Electrochemical Society, and 2003 Institute of Physics Publishing. The crystal field splitting of the 5d configuration of (d) Ce3+ and (e) Eu2+, and (f) their correlation in nitride phosphors. Panels d–f reproduced with permission from ref. 85, copyright 2017 Elsevier B.V. | |
Taking the 12 pm larger size of Eu2+ into account, the correlation between Ce3+ and Eu2+ crystal field splitting εcfs was further achieved (Fig. 4c)84
| εcfs(Eu2+) = 0.77εcfs(Ce3+). | (10) |
As the emerging of nitride phosphors, our group then branched out to explore the structure-crystal field splitting εcfs correlations in Ce3+/Eu2+-activated nitrides. By analyzing the luminescence and structural data of tens of Ce3+/Eu2+-doped nitrides, the relationship between the crystal field splitting εcfs and the shape and size of the coordination polyhedron in nitride compounds is obtained as shown in Fig. 4d and e, which is consistent with the results of halide, oxide, sulfide, and selenide compounds, further confirming that εcfs is irrelevant of anion types.85 The crystal field splitting εcfs behaves as shown in eqn (7), and decreases with the coordination number increasing. A linear relationship as shown in eqn (10) also exists in nitride phosphors with a similar multiplication factor of 0.76 (Fig. 4f). In summary, with these quantitative correlations, the crystal field splitting εcfs in Ce3+/Eu2+-activated inorganic phosphors can be theoretically predicted based on the crystal structure.
Electronic structure.
Both the luminescence and persistent properties of lanthanide-doped phosphors are mainly determined by the electronic structure, specifically the energy of the lanthanide excited and ground states with respect to the band states of the host.86–88 With the aim of illustrating the electronic structure, Dorenbos proposed the concept of host referred binding energy (HRBE) and vacuum referred binding energy (VRBE) schemes, which provide energy information of all the lanthanide levels and host bands with sufficient accuracy.21,47,48 With the assumption that the valence band (VB) maximum energy is 0 eV, HRBE schemes provide the relative energy of 4f and 5d levels of divalent and trivalent lanthanides with respect to the band states of the host. On the basis of HRBE schemes, Dorenbos further proposed the chemical shift model, which relates the host referred binding energy with the vacuum referred binding energy, giving rise to the VRBE schemes representing the absolute energy of 4f and 5d levels of divalent and trivalent lanthanides as well as the band states of the host.89,90 Detailed construction instructions of HRBE and VRBE schemes have been elaborated in ref. 60, 86, 91. As an example, Fig. 5 shows the HRBE and VRBE schemes for Y3Al5O12 (YAG), in which the red and blue zigzag curves represent the energy for the 4f ground states of divalent and trivalent lanthanide ions, respectively, and the other red and blue curves represent the energy for the lowest 5d states of divalent and trivalent lanthanides, respectively. The HRBE and VRBE schemes enable us to interpret, predict, and engineer the properties of phosphors including but not limited to the thermal luminescence stability, trap depth introduced by lanthanides, the preferred valence of the lanthanides, and the presence or absence of emission.86,92,93 On the basis of the relative energy between the 5d states of the Ce3+/Eu2+ emission center and the CB edge of the host, one can rationally select a suitable host lattice with appropriate ionization energy for LED or persistent phosphors. In addition, the energy of the 5d states of divalent lanthanides gives information on the depth of the traps introduced by trivalent lanthanides, which provides a feasibility to rationally choose the trap center for persistent phosphors.94–96 As a summary, HRBE and VRBE schemes make it possible to systematically manipulate the LED and persistent phosphor properties by tuning the energy of the CB edge via cation substitution in the host and co-doping trivalent lanthanides as trap centers, which are the so-called electronic structure engineering. The examples for the excellent application of electronic structure engineering will be presented in detail in Section 3.
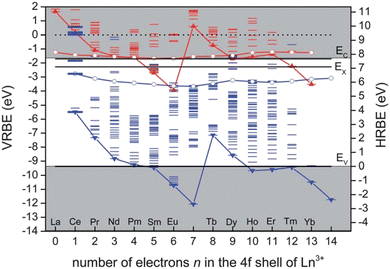 |
| Fig. 5 The HRBE and VRBE schemes of Y3Al5O12. Red and blue ‘zigzag’ curves connect the energy for electrons in the 4f ground state of divalent and trivalent lanthanides, respectively; The other red and blue curves connect the energy for the lowest 5d states of divalent and trivalent lanthanides, respectively. Reproduced with permission from ref. 86, copyright 2012 Royal Society of Chemistry. | |
It is worth noting that even though the Dorenbos model is of vital importance for illustrating, predicting, and tuning phosphor properties, it has limitations. It rather parameterizes the trends than explaining the mechanism, and it fails to explain individual phosphors in detail. Therefore, further exploration of the correlation between the Dorenbos model and the luminescence theory is required to optimize the Dorenbos model and gain insight into its physical origins. Nevertheless, the empirical/phenomenological approaches bear the advantages of the simple form and easy-to-use style. Both beginners and veterans could gain useful insights from those simple models involving bond lengths, bond angles and constitutional components. Yet it can be only applied in a limited region, and fails to respond to the structure–property relationships across different material systems. On the contrary, the advanced first principles/ab initio approaches could provide more information on the dopant energy levels and electronic structure of the host. Besides, the orbital analysis and defect level calculation are unique and give more fundamental insights into the luminescence behavior. Although those advanced calculations are prevailing in recent publications, the huge consumption of computational resources still remains a barrier for most researchers. Meanwhile, some user-friendly automatic DFT software may undermine the expertise needed for the calculation process.
Effects of the local structure on d–f luminescence
Crystal field.
The emission and absorption of light by a phosphor originate from the electronic transitions between the discrete energy levels of the luminescent center embedded in the host. The energy level distribution is determined by the coulomb interactions between nuclei/electron and electron/electron, together with the Stark effect of the valence electron exerted by the surrounding ligands. Rare-earth-doped phosphors employing f–d electronic transitions exhibit intensified oscillator strength from luminescent center, such as Ce3+ and Eu2+, compared to those of f–f or d–d transitions. For rare-earth ions, 4f electrons are screened by the outer-shell 5s25p6 electrons. Therefore, the energy levels are insensitive to the subtle change in the ligand environment. Unlike 4f electrons, the 5d electron is directly exposed to the crystalline environment and its energy levels could be tuned by the modifications of the surrounding ligands. As a result, the versatile host structures have a significant impact on 5d energy levels, and the emission spectrum of f–d transitions could reproduce the whole spectral region from ultraviolet to far red. The effect of crystalline ligand environment on luminescence tuning constitutes the main topic of crystal-field theory.
Crystal field theory has successful explanations in high-symmetry ligand polyhedra, such as ideal octahedron (6-coordination) and cube (8-coordination), both of which share the point symmetry Oh.97,98 The detailed analytical description is derived in the framework of perturbation theory. First, the crystal-field potentials of one d electron exerted by the surrounding ligands of octahedron and cube are99
|  | (11) |
|  | (12) |
respectively, with the substitution

. In the above formula, (
x,
y,
z) stands for the spatial coordinates of the d electron,
r for the distance between electron and the nuclei, and
a for the center–ligand distance. In order to calculate the perturbation matrix elements
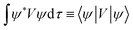
, the basis function could be selected as one d electron wavefunction, with an angular part |2,
ml〉, where
ml stands for the magnetic quantum number (±2, ±1 and 0 in the case of
l = 2). With further substitution of
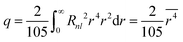
, in which
Rnl stands for the radial part of the d electron wavefunction, the perturbation matrix could be constructed in the form of
D and
q product, and the diagonalization result gives the splitting of 5-fold degenerate energy levels by the Stark effect due to the electrostatic interaction with ligand ions. It is interesting to note that the energy level splitting schemes are −4
Dq (triple) and 6
Dq (double) for octahedron and −16/3
Dq(double) and 32/9
Dq(triple) for the cube. Octahedron has the largest crystal-field splitting of 90/9
Dq, whereas cube has a smaller one of 80/9
Dq. In addition, in the case of ideal cube two orbitals are positioned in the lower energy scale in comparison to the three degenerate orbitals in the octahedron. Since both are proportional to
D, then a reciprocal 5th-power relationship could be obtained between the total crystal-field splitting and the center–ligand distance. This simple rule paves the way for explaining the energy level shift from the viewpoint of the ligand environment and serves as a bridge between the crystal structure and the luminescence properties.
The 5th-power rule seems to provide an ultimate answer to the structure–property problem in luminescence. Under this simple and clear guidance, only the 1D bond length magnitude instead of the 3D complexity of crystal structure needs to be considered. This rule, however, meets astonishing contradictory cases in a ligand polyhedron with a local symmetry lower than that of Oh. The structure–property relationship itself proves to be not only a mere bond-length comparison game, as will be demonstrated in the garnet-type phosphors.
Garnet-type phosphors constitute a large family of commercial phosphors. It has a remarkable capability to accommodate various luminescent dopants including both rare-earth and transition-metal elements, such as Ce3+, Eu3+, Tb3+, Cr3+, etc. Among them, Ce3+ doped garnet phosphors are most widely used in the field of wLEDs because of the relatively cheap cost, chemical stability, and convenience for mass production. The luminescence of Ce3+ in the garnet host originates from the parity-allowed d–f transitions, which was first reported by Blasse et al. in 1960s.100 In the garnet structure, the 8-ligand coordinated dodecahedral (A) site with point symmetry D2 accommodates Ce3+. In this low-symmetry crystal-field potential, the 5-fold degenerate 5d energy levels will be totally split. The energy level scheme could be understood as first split by a Td crystal-field potential, with 2E (2-fold, low-lying) and 2T2 (3-fold, higher) levels, and then further split by a D2 crystal-field potential.101–103 By composition substitution, the coordination environment of the dodecahedral site could be modulated and the luminescence of the Ce3+ doped garnet could be tuned. However, inspection of excitation spectra of Ce3+ in the garnet series of Y3Al5O12(YAG), Y3Ga5O12(YGG), Gd3Al5O12(GdAG), Gd3Ga5O12(GdGG), Lu3Al5O12 (LuAG), Lu3Ga5O12(LuGG) and their solid solutions presents a contradictory relationship between the crystal structure and crystal-field splitting.104–107
Composition substitution takes effect due to the difference in the ionic radius. For the above-mentioned garnet series, it has been confirmed that the cell parameters, volumes of dodecahedron (A) and mean bond-lengths have a consistent trend with the ionic radius of the substituted elements.108 However, the crystal-field splitting demonstrates contradictory trends with the ionic radius. Here, for convenience the crystal-field splitting is represented by the energy difference between the two lowest 5d levels, which could be easily read out as two excitation peaks. When the cell is expanding from YAG to YGG due to the larger Ga, the crystal-field splitting is decreasing,109 in accordance with the 5th-power rule. Yet from YAG to LuAG the crystal-field splitting is reduced with cell shrinkage.110,111 The deviation from the 5th-power rule in garnet phosphors is named the reverse garnet effect.5,112 It is surprising that the normal and reverse phenomena co-exist in the same material family.
Many efforts have been devoted to resolve this self-contradictory phenomenon. For example, the self-adjustment of the local structure on doping is considered as one of the reasons. DFT investigations on the structural relaxation of Ce3+ in the lutetium aluminum garnet shows longer Ce–O bonds, which could explain the smaller crystal-field splitting according to the 5th-power rule.113 Yet, the dilemma lies in that the 5th-power rule itself is derived without the consideration of local structure relaxation. In fact, the simple 5th-power rule only constitutes a small part of the crystal-field theory. It is derived under the highest-symmetry point group, i.e., Oh, which will eliminate all the 2nd rank crystal-field parameters due to the symmetry limitation in the crystal-field potential.114,115 This special constraint leaves only one 4th rank crystal-field parameter, i.e. B40, in the expression of the Oh crystal-field potential for d electron. Under the framework of the point-charge electrostatic model (PCEM), B40 has the form of a 5th power exponential of bond length in the denominator. But further symmetry degradation will set the 2nd crystal-field parameters nonzero, which will introduce 3rd power exponential of bond length together with that of the 5th power. In the real D2 site symmetry, the crystal-field potential could be expressed as114
|  | (13) |
The final analytical expression of the split energy levels will inevitably include the 2nd rank crystal-field parameters, and thus break the monotonous relationship between crystal-field splitting and center–vertex distance. With the D2 crystal-field potential, the crystal-field analysis has been performed assuming the crystal-field strength in the sequence of cubic field ≫ the spin–orbit interaction ≫D2 crystal-field,103 to calculate the energy level splitting of Ce3+ doped garnets.108,109 The results provide convincing explanation for the above-mentioned self-contradictory phenomena. For the aluminum and gallium series, the crystal-field splitting increases as Lu < Y < Gd. For LnAG/LnGG paris, i.e. LuAG/LuGG, YAG/YGG and GdAG/GdGG, it drops as Ga substitutes for Al. It can be concluded that the contradiction vanishes because both the normal and reverse phenomena originate from the crystal-field effect.
Although there have been similar responses of cell and polyhedral volumes on larger ion substitution at different cationic sites, subtle differences exist in how the oxygen ligands respond to different site substitutions. The ligand movement has two distinct patterns with regards to site substitution, as illustrated by the example of evolvements from LuAG to YAG and LuAG to LuGG in Fig. 6a. Further investigation shows that these two patterns are closely related to the squeeze or stretch of the octahedron (16a site), and the interplanar distance of the octahedron is selected to characterize the multi-site substitution. Meanwhile, the distance has a simple expression of
, in which L is the cell parameter and (x, y, z) stands for the oxygen coordinates resembling the form of (−0.0306, 0.0506, 0.1493).116 This proves to be an effective indicator of the crystal-field splitting of Ce3+ in the garnet structure, as shown in Fig. 6b.
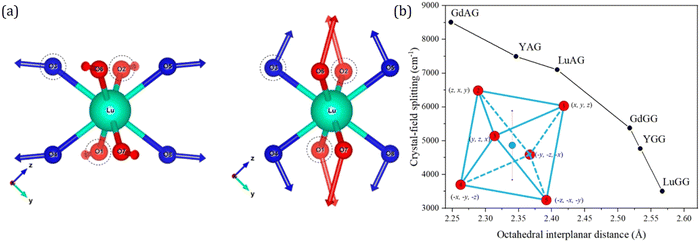 |
| Fig. 6 (a) Left: ligand movement pattern for LuAG to YAG; right: ligand movement pattern for LuAG to LuGG. The arrows indicate the moving direction of ligands when the garnet compound changes. The magnitude of the arrow has been magnified 30 times for better visualization. Reproduced with permission from ref. 108, copyright 2018 American Chemical Society. (b) Crystal-field splitting as a function of octahedral interplanar distance for different garnet series. The inserted graph shows octahedron and its ligands numbered from 1 to 6, with the fractional coordinates aside. The centers of the two regular triangles are denoted as small purple dots. A dotted purple line connecting the two centers represents the interplanar distance. Reproduced with permission from ref. 116, copyright 2019 American Chemical Society. | |
The validity of the octahedral interplanar distance as the structural indicator stems from its relation to the tetragonal distortion of the dodecahedron. The tetragonal distortion was first systematically studied by Seijo and Barandiarán, who performed quantum chemistry calculations of Ce3+ doped garnets based on AIMPs (ab initio model potentials).118 They establish the 8-coordinated distorted cube by transforming a reference perfect cube through a series of SBT (stretching, bending and twisting) operations. They find that only the symmetric stretching (S1) and symmetric bending (S3) modes have important impacts on the lowest 4f–5d transition. In addition, the symmetric bending (S3) also leads to tetragonal distortion of a perfect cube, which induces elongation or compression of the cube, resulting in a cuboid. Early in 2007, Wu et al.112 have found the dodecahedral edge-ratio as an indicator of tetragonal distortion. It also presents a monotonous relationship of excitation properties of Ce3+ in garnets. A simplified cuboid model is set up to provide a deeper understanding of the effect of tetragonal distortion on crystal-field splitting. The cuboid degrades the point symmetry from Oh of a perfect cube to D4h, and the energy level splitting scheme also differs as depicted in Fig. 7. Numerical simulation of the crystal-field analysis shows that in certain geometrical configurations, the tetragonal distortion induces strengthening of the crystal-field splitting even with a larger cell size and a longer center–vertex distance.117
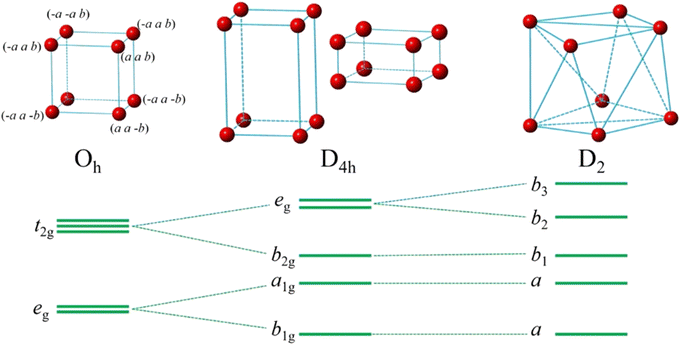 |
| Fig. 7 Cuboid model with Cartesian coordinates of vertex and energy level splitting scheme. Reproduced with permission from ref. 117, copyright 2019 American Chemical Society. | |
Since tetragonal distortion has such a profound impact on the complex crystal-field splitting variation of Ce3+ in garnets, it is necessary to find their crystallographic origin. The above-mentioned numerical simulation shows that on isotropic expansion or shrinkage of the ligand polyhedron, the 5th-power rule will be obeyed. But the tetragonal distortion is anisotropic, caused by the neighboring polyhedron competition that has the effect of compromising the volumetric change of all the polyhedra. In the real garnet structure, polyhedra are linked by shared edges, and polyhedron deformations induced by compositional substitution behave in different manners with or without the neighboring polyhedra. The dodecahedral deformation is far beyond isotropic because of the competitions between dodecahedron and neighboring polyhedra, i.e., suppression by the octahedron/tetrahedron grid. Therefore, the complexity in the structure–property relationship is principally portrayed by the subtle local crystal structure changes.
Inductive effect from second nearest atoms
The structure and composition are decisive for the physical and chemical properties. Typically, 5d energy level of Eu2+/Ce3+, acting as a crucial factor for luminescence characteristics, is mainly determined by the structure and composition of the host, as illustrated in Fig. 8a. The effect of average compositions and structure on the energy of 5d level is represented as the nephelauxetic effect, which determines the centroid shift of the 5d level. Besides, the local structure also plays a critical role in 5d level determination. The effect of the nearest coordination anion on the energy of the 5d level is expressed by crystal field splitting, as a well-known parameter widely used for predicting and understanding the luminescence properties. Likewise, the second nearest cation also has a significantly influence on the energy of 5d level, which can be conveyed by the inductive effect.
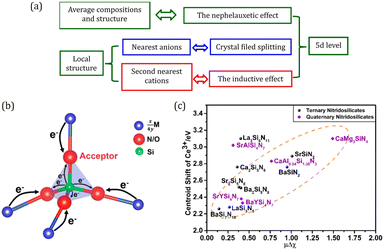 |
| Fig. 8 (a) Schematic diagram of structures on 5d levels. (b) Schematic diagram of the inductive effect of M. The thickness of arrow represents the degree of ability of donating electrons. (c) Centroid shift of the 5d levels of Ce3+versus the inductive factor in (qua)ternary nitridosilicates. Panels (b and c) reproduced with permission from ref. 119, copyright 2018 American Chemical Society. | |
Since the inductive effect was first proposed to explain the subtle changes of Si–O bond lengths in silicates by Noll in 1963,120 it has been widely used in understanding the physical and chemical properties of materials including inorganic and organic compounds. As illustrated in Fig. 8b, for a ternary compounds TxMyXz, where X is an anion and T and M are cations, if T is less electronegative than M, it will tend to act as an electron donor. Then the anion X prefers to get the electron density from T instead of M, causing the changes of the M–X bond. This is called the “inductive effect”.121 In 2017, it was introduced into luminescent materials and accounts for the tunable luminescence properties in K2(0.995−x)-Na2xAl2B2O7:0.01Eu2+ (KAB:2xNa).122 The excitation spectra analysis shows that the centroid shift decreases with x increasing, and then the inductive effect is employed to understand this phenomenon. Given that Eu occupies the K site and its neighboring cation K is increasingly replaced by Na as x increases, the covalency of the Eu–O bonds would be reduced since Na is more electronegative than K, which leads to a smaller centroid shift of the 5d levels. After this, our group further provided insight into the influence of the inductive effect on structural chemistry and luminescence properties in nitridosilicates and oxysilicates.119 A new parameter, the inductive factor, was proposed to relate the difference of electronegativity between metal elements and silicon. Through collecting and analyzing the structural and luminescence data of more than one hundred compounds, a linear relationship between the average length of the Si–N/Si–O bonds and the inductive factor is revealed. And centroid shift εc shows obvious positive correlation with the inductive factor as shown in Fig. 8c. In addition, the inductive effect also accounts well for the charge transfer (CT) process. CT transition is another kind of electric dipole allowed transition of the electron from ligands to lanthanide ions, which may give out intense luminescence, such as the CT luminescence of Yb3+ in Yb3+-containing compounds, and the CT state sensitized luminescence in red phosphor Y2O3:Eu3+.123 By introducing electronegativity factor and ionic radius factor, semiquantitative models are proposed that CT energy has a strong positive correlation with the electronegativity factor and a strong negative correlation with the ionic radius. These works highlight the key role of the neighboring cations in structural chemistry and luminescence properties, which provide us an interesting perspective for phosphor design.
Stokes shift.
The Stokes shift ΔS is defined as the energy difference between the excitation and emission maxima of the same electronic transition. As the excitation properties can be predicted based on the crystal field splitting εcfs and the centroid shift εc, combining with the Stokes shift ΔS, the emission properties can be further forecasted based on the composition and structure of the compounds. It is fair to say that gaining the knowledge of the effect of the composition and structure on the Stokes shift ΔS is of guiding importance for the development of Ce3+/Eu2+-activated inorganic phosphors.
For Ce3+/Eu2+-activated inorganic compounds, the degenerate 5d-level splits into at most five 5d states due to the interaction of the 5d-electron with the neighboring anion ligands. Excitation occurs by the transitions from the 4f ground state to all the 5d states. At room temperature, the decay from the higher 5d states to the lowest 5d state is dominantly non-radiative and emission occurs by the transition from the lowest 5d state to the ground state, as shown in Fig. 9a. Therefore, more specifically, the Stokes shift is the energy difference between excitation and emission maxima of transition between the lowest 5d and the 4f ground states.20,58,59 Based on the assumptions (1) Franck–Condon approximation, implicating that an optical transition on the dopant ion occurs so quickly that the lattice arrangement does not change during the transition, and (2) considering only one mode of lattice vibration, i.e., the breathing mode, which states that the surrounding lattice pulsates in and out about the dopant ion. The electronic and lattice states can be represented in a single energy level diagram called the configurational coordinate diagram.125 In Fig. 9a, the processes of the excitation and emission transitions are shown in the configurational coordinate diagram represented by the ground and excited state potential energy curves with vibrational levels depicted as horizontal lines, and the Stokes shift also being marked in the picture. For simplicity, in the analysis, the harmonic approximation and the assumption that the vibrational frequencies are the same in both ground and excited states are generally adopted. The Stokes shift ΔS has the relation of125
where
ħ is the reduced Planck constant,
ω is the angular frequency of vibration, and
ħω is the energy difference between the consecutive vibrational states (phonon energy), as shown in
Fig. 9a. Dimensionless Huang–Rhys factor
S gives information about the coupling of electronic and vibrational states (
i.e., electron–phonon coupling). The relation Δ
S = (2
S − 1)
ħω has been used in many reports, while the alternative relation Δ
S = 2
Sħω has also been used in some studies.
126–132 Jong
et al. resolved this ambiguity and pointed out that both the expressions are only approximately correct and the accurate Stokes shift is always between them.
58 Blasse classified the cases of weak (
S < 1), intermediate (1 <
S < 5) and strong (
S > 5) electron–phonon energy.
133 Huang–Rhys factor
S is given by
|  | (15) |
where
α = Mω/ħ and
M is the reduced mass of the vibrating system,
125 and Δ
Qe is the equilibrium position offset, as shown in
Fig. 9a. The existence of such a displacement corresponds to different equilibrium geometries in the electronic ground and excited states, which is caused by the change in the bonding between ions or atoms in the ground state and excited states as a result of the change in the electronic configuration.
58 It is affected by the cation substituted by lanthanide, coordination anions and local structure in the compounds. Blasse
et al. reported that the larger size of the cation substituted by lanthanide ion leads to a larger Stokes shift in three orthoborates.
134 In addition, the phonon energy
ħω is linearly related to the Stokes shift, as shown in
eqn (14). It was reported that phonon energy decreases in going through the halogenide series from fluoride (∼350 cm
−1)
via chloride (∼260 cm
−1) to bromide (∼200 cm
−1) compounds.
135 It also has been affirmed that oxides (∼600 cm
−1) have a larger phonon energy than sulfides (∼270 cm
−1), and the selenides (150 cm
−1) are the smallest among them.
136 This means that vibrational frequency
ω decreases from oxides
via sulfides to selenides, which originates from the increasing atomic weight, resulting in the increasing atomic radius, decreasing electronegativity and the weaker bond strength. And so does the situation in halogenides.
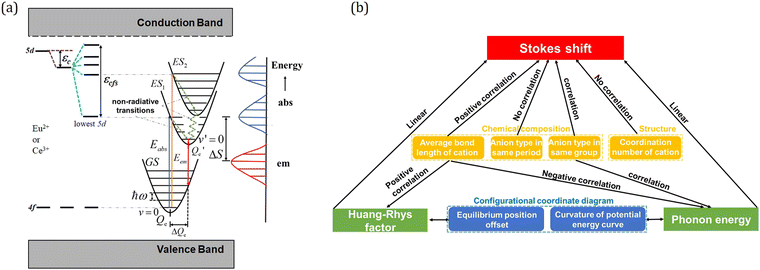 |
| Fig. 9 (a) Energy level diagram showing the crystal field splitting εcfs, centroid shift εc and the Stokes shift ΔS. Some vibrational states and phonon energy are indicated in the configurational coordinate diagram. Reproduced with permission from ref. 124, copyright 2019 Elsevier B.V. (b) A summary scheme representing the correlations between the Stokes shift, phonon energy, Huang–Rhys factor, chemical composition and structure of the compounds. | |
Through statistical analysis of the structural and luminescence data of more than 60 Ce3+/Eu2+-activated inorganic phosphors, our group delved into the effect of the chemical composition and structure on the Stokes shift, phonon energy, and Huang–Rhys factor.124 It has been demonstrated that both the cation substituted by Ce3+/Eu2+ and the coordination anion have crucial effects on the Stokes shift, phonon energy and Huang–Rhys factor. The Stokes shift ΔS has a positive correlation to the effective average coordination bond length Rav. It decreases in going through the halogenide series from fluorides to bromides, and the chalcogenides from oxides to selenides, respectively, while it has little difference in the compounds with the anions of the same period, which can be well digested using the effective charge concept. By replacing the formal charge Zfc of the anion (1 for fluorides, 2 for oxides and 3 for nitrides) with an effective charge Zec = Q/N (Q is 3 for Ce3+ and 2 for Eu2+, and N represents the number of coordination anions), the electric field around the Ce3+/Eu2+ is approximately independent of the anion type in the same period, which results in no obvious influence of the ligand charge on the bonding strength. It also reveals that the Stokes shift ΔS has no obvious correlation with the coordination number of cation. Besides, the phonon energy is negatively correlated to the average bond length R, while the Huang–Rhys factor S is positively related to R. These composition–structure–Stokes shift correlations provide a powerful tool to predict the emission spectrum of Ce3+/Eu2+-activated inorganic phosphors.
Quantum efficiency and luminescence quenching.
Luminescence of rare-earth elements originates from the electronic transition between separated energy levels. For a ground-state electron, it jumps to the high-energy level under light activation and stays at a meta-stable excitation state after the cross-relaxation process by emitting phonons. Next, those excited electrons will return to the low-energy ground state by emitting photons, which is called radiative transitions. However, this process occurs non-simultaneously for all the excited electrons. Those electrons could be thought as waiting in a sequence characterized by the decay lifetime. From the beginning to the lifetime, which has the magnitude of nanoseconds and microseconds for Ce3+ and Eu2+, respectively, about 63.2% of the excited electron ensemble will undergo the radiative transitions. Therefore, there is enough time for the excited electron to take part in other non-radiative transitions. When the energy-curve cross point between excited/ground states lies closer to the meta-stable excitation state, the excited electrons have the chance to return to the ground state with the help of thermal activation or by the tunneling process.137 In this case, the energy is dissipated within the original activator by emitting phonons. In other cases, the excited energy would be lost to “killer” centers such as defects, vacancies, etc., during the energy transfer between activators or through the conduction band. In order to characterize the luminescence efficiency, quantum efficiency (QE) is usually measured for the luminescent material.
Apart from suitable excitation and emission spectra, high (QE) and good thermal luminescence stability also plays key roles in the practical applications of LED phosphors. QE of phosphors is defined as the ratio between the number of emitted and absorbed photons.138 When an electron is excited from the ground state to the excited states, it will return to the ground state via two paths, either radiative transition or non-radiative transition. Radiative transition refers to the emission of lights, while in the non-radiative transition the energy is dissipated in some other way except for luminescence, which is generally called luminescence quenching.139 Thus, the QE of phosphors fundamentally depends on the radiative recombination efficiency of the electrons, thereby it is of vital importance to have the knowledge of the nature of the non-radiative channels that compete with radiative emission.
Till now much endeavors have been devoted to explore the luminescence quenching process, and three quenching mechanisms are widely recognized including configurational coordinate model, ionization model, and impurities and lattice defects model.140,141 For the configurational coordinate model, sufficient thermal energy could assist the excited electron to an upper vibrational level in the excited state. If the upper vibrational level is higher than the intersection of the excited and ground potential energy curves, the excited electron can return to the ground state by losing energy to the host lattice in the form of phonons (lattice vibration), which leads to luminescence quenching.142 The excited electron also has chance to return to the ground state by the tunneling process.137 In this model, the luminescence efficiency is mainly determined by the phonon energy ħω and the Huang–Rhys factor S of the LED phosphors. In the ionization model, the 5d1 excited electron can be photoionized or thermally ionized into CB. Subsequently, the ionized electron becomes delocalized and travels across the whole crystal, which increases the possibility to be trapped by a defect trap and lose excitation energy, resulting in luminescence quenching.143,144 In this case, the luminescence efficiency depends on the ionization energy of the 5d excited electron, which is the energy difference between the CB bottom edge of the host and the 5d1 excited state of the emission center. For the impurities and lattice defects model, energy can be transferred to defects or impurities, which have additional electronic levels to which excitation energy can be transferred. Such transitions are limited to the cases where the impurities or defects are sufficiently close to the activation ions, or the concentration of the activation ions is high enough so that the impurities or defects can be reached via energy migration over multiple activation ions, which is well known as concentration quenching. What is noteworthy is that these quenching processes related to impurities and defects are not intrinsic to the material and can be avoided by optimizing synthesis conditions and dopant concentrations. As for the first two quenching processes, while different, they are the intrinsic properties, related to the crossover point of the 4f and 5d potential wells and the relative energy position of the activation ions with respect to the host crystal bandgap, which can be adjusted by choosing a suitable host material or modifying the existed phosphors. Electronic structure schemes with the energy information on the activation ions and the host crystals are recently used as powerful tools for optimizing the luminescence efficiency and thermal luminescence stability as discussed above.
It is worth noting that luminescence quenching will be aggravated as the operating temperature increases since the quenching processes in configurational coordinate, ionization model and energy transfer to defects are thermally activated. While functioning, LED chips produce heat and transfer it to the coating phosphors. This heat activates more phonon modes of the phosphor, thereby decreasing the efficiency and luminous intensity.5,145 This phenomenon is typically onset between 100 and 200 °C, and is described as thermal luminescence quenching, which not only lowers the overall efficiency but also causes the undesirable change of the color rendering of the device. The preservation of phosphor emission intensity and quality with an increase in temperature is referred as the thermal luminescence stability. Therefore, taking thermal and concentration quenching into account, luminescent materials with optimized dopant concentration, extraordinary thermal stability, and fine structural rigidity are necessary to fulfil the requirements for LED applications.
Persistent luminescence mechanism.
Persistent phosphors generally consist of the host lattice and two kinds of activation centers including emission centers and trap centers. Emission centers are mostly Eu2+ or Ce3+ that give out luminescence. Trap centers are typically lattice/intrinsic defects (such as oxygen vacancies), or intentionally introduced aliovalent or isovalent co-dopants (such as Dy3+ or Cr3+), which can capture the excitation electron and then release it by thermal assistance, causing the luminescence delay or continuous luminescence as in PersL.24 Since the green persistent phosphor SrAl2O4:Eu2+,Dy3+ with excellent properties was discovered,30 tremendous research studies have been devoted to explore its underlying mechanisms. Although several models were proposed in order to give convincible explanations for this attractive phenomenon,146 we will limit ourselves to the most widely used electron trapping–detrapping mechanism for Ce3+/Eu2+ doped persistent phosphors.23 As illustrated in Fig. 10, the PersL process can be typically divided into four phases: (a) delocalization of electron. Upon effective optical charging by ultraviolent (UV) or visible (VIS) light irradiation, there are two pathways to get delocalized electrons: (1) the 4f electrons are excited to 5d excited states and thus thermally ionized to the conduction band (CB); and (2) 4f electrons are excited directly to CB. (b) Storage of electrons (charging): the delocalized electrons are captured by the traps. (c) Release of captured electrons: after ceasing the excitation, the captured electrons are thermally released from the traps via the CB to 5d excited states of Ce3+/Eu2+. (d) Recombination process: the released 5d excited electrons move back to the 4f ground state yielding delayed luminescence, which is called PersL. Consequently, the emitting properties of PersL including the emission wavelength and the bandwidth are determined by the emission center, while persistent properties including intensity and duration are determined by the characteristic of the trap center, such as trap depth and density. Trap depth is the energy gap (ΔE) between the bottom of CB and the trap level. Generally, electrons in shallow traps with a small ΔE will be released fast at the operating temperature, which contributes to a strong initial intensity but a short PersL duration. In contrast, electrons in deep traps with a large ΔE will be gradually or even hardly released at the operating temperature, which results in a long PersL duration but weak initial intensity. Thus, an ideal ΔE needs to match the operating temperature so that the captured electrons can be slowly released by the relevant assisted energy, giving rise to optimized PersL properties. In addition, an appropriate trap density generally contributes to a strong PersL intensity, which can be achieved by tuning the trap center concentration or introducing a co-dopant. What is more, emission center is also critical, since it not only determines the luminescence properties but also plays a vital role in achieving delocalized electrons (thermal ionization of 5d excited electrons). Therefore, two essentials for promising persistent phosphors can be summarized: (1) an appropriate host crystal with an emission center; and (2) trap center with a suitable trap density and depth for specific applications. Accordingly, the PersL performance can be optimized following two strategies: (1) manipulating the bandgap structure of the host especially the CB minimum energy by structure modulation; and (2) introducing rare earth or transition metal ions or deficiencies as trap centers. Both strategies can be approached by virtue of electronic structure schemes described above.
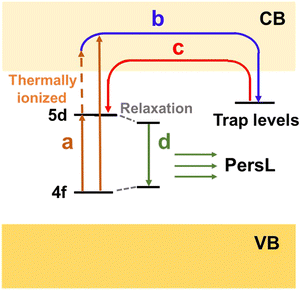 |
| Fig. 10 Illustration for the PersL mechanism. | |
Well known Ce3+/Eu2+-activated LED and persistent phosphors: focusing on the local structure and the electronic structure
The past several decades have witnessed the rapid development of LED phosphors and the increasing interest in strategies to improve their properties toward specific applications. In this section, we present several widely used promising phosphors, focusing on their crystal structure, local structure, electronic structure, and luminescence properties. Meanwhile, with the aim at illustrating the strategies to screen phosphors with potential PersL properties, the LED phosphors, in which decent PersL has been achieved successfully, get the priority here. With the emphasis on structure design principles and routines, here we discuss the composition–structure–property correlations and how the local structure and the electronic structure can alter the luminescence properties including emission characteristics, luminescence efficiency, thermal luminescence stability, and PersL properties. The electronic structure, luminescence and PersL properties of these materials are summarized in Tables 1–4 with the prospect of motivating inspirations of interested readers for further development of luminescent materials and other functional materials.
Table 1 Luminescence properties and band gap of garnet phosphors. λex,max and λem,max are the wavelengths corresponding to the peak of excitation and emission bands, respectively; QY is the quantum yield; I150
°C/IRT represents the relative emission intensity at 150 °C to that at room temperature
Chemical composition |
λ
ex,max (nm) |
λ
em,max (nm) |
QY (%) |
Band gap (eV) |
I
150 °C/IRT (%) |
Ref. |
Y3Al5O12:7%Ce3+ |
460 |
555 |
80 |
6.4 |
93 |
144, 145 and 154
|
(Y2.2Gd0.8)Al5O12:6%Ce3+ |
460 |
568 |
— |
— |
— |
153 and 162
|
Gd3Al5O12:1%Ce3+ |
470 |
600 |
— |
6.3 |
32 |
156 and 163
|
Gd3GaAl4O12:1%Ce3+ |
458 |
587 |
— |
6.3 |
37 |
163
|
Gd3Ga2Al3O12:1%Ce3+ |
448 |
574 |
— |
6.3 |
39 |
163
|
Gd3Ga3Al2O12:1%Ce3+ |
442 |
566 |
58–82 |
6.1 |
11 |
163 and 164
|
Gd3Ga4AlO12:1%Ce3+ |
437 |
561 |
— |
5.9 |
— |
163
|
Gd3Ga5O12:1%Ce3+ |
428 |
490 |
— |
5.4 |
— |
163
|
Lu3Al5O12:7%Ce3+ |
450 |
540 |
84 |
7 |
96 |
154
|
Ca3Sc2Si3O12:Ce3+ |
455 |
505 |
— |
7.35 |
90 |
161 and 165
|
Tb3Al5O12:1%Ce3+ |
470 |
552 |
— |
— |
56 |
156 and 166–168
|
Y3Sb2Al3O12:4%Ce3+ |
465 |
528 |
— |
— |
— |
169
|
Y3Al4.9Si0.1O11.9N0.1:3%Ce3+ |
470 |
595 |
68 |
— |
53 |
170 and 171
|
Y3Mg2AlSi2O12:1.5%Ce3+ |
475 |
600 |
45 |
— |
44 |
172 and 173
|
CaLu2Mg2Si3O12:Ce3+ |
470 |
605 |
80 |
— |
72 |
174
|
CaLu2Al4SiO12:1%Ce3+ |
450 |
520 |
77 |
5.77 |
85 |
175
|
Mg3Y2Ge3O12:3%Ce3+ |
460 |
535, 573 |
4.5 |
5.47 |
— |
176
|
MgY2Al4SiO12:6%Ce3+ |
452 |
568 |
— |
— |
— |
177
|
Ca2GdZr2(AlO4)3:2%Ce3+ |
417 |
500 |
40 |
— |
33 |
178
|
Ca2LuZr2(AlO4)3:2%Ce3+ |
411 |
480 |
50 |
— |
60 |
179
|
Ca2YZr2(AlO4)3:2%Ce3+ |
412 |
481 |
43 |
— |
51 |
178
|
CaGd2ZrSc(AlO4)3:2%Ce3+ |
448 |
545 |
13 |
— |
18 |
178
|
Ca2LaZr2Ga3O12:6%Ce3+ |
430 |
515 |
35 |
4.54 |
32 |
159
|
Ca3Hf2SiAl2O12:0.5%Ce3+ |
400 |
457, 482 |
75 |
— |
73 |
180
|
Tb2.2Lu0.8Al5O12:0.5%Ce3+ |
430 |
550 |
— |
— |
— |
181
|
Mg3Y2(Ge0.3Si0.7)3O12:Ce3+ |
460 |
604 |
54.3 |
5.82 |
— |
176
|
Y3AlGa4O12:Ce3+ |
422 |
504 |
17 |
— |
13 |
160 and 182
|
Y3Al2Ga3O12:Ce3+ |
430 |
512 |
47 |
5.9 |
21 |
144 and 182
|
Y3Al3Ga2O12:Ce3+ |
440 |
523 |
83 |
— |
85 |
182 and 183
|
Y3Al4GaO12:Ce3+ |
447 |
537 |
86 |
— |
94 |
182
|
Mg3YGdGe3O12:Ce3+ |
467 |
575 |
— |
— |
— |
160
|
Mg3Y0.25Gd0.75Ge3O12:Ce3+ |
465 |
590 |
— |
— |
— |
160
|
Mg3Gd2Ge2SiO12:Ce3+ |
467 |
606 |
— |
— |
— |
160
|
Mg3Gd2Ge3O12:2%Ce3+ |
464 |
600 |
— |
— |
— |
160
|
Lu3Al4.8Si0.2O11.8N0.2:3%Ce3+ |
470 |
587 |
— |
— |
71 |
170
|
Y3Al3MgSiO12:0.25%Ce3+ |
450 |
577 |
68 |
— |
56 |
184
|
Y2LuAl3MgSiO12:0.25%Ce3+ |
450 |
570 |
71 |
— |
62 |
185
|
YLu2Al3MgSiO12:0.25%Ce3+ |
450 |
564 |
78 |
— |
70 |
185
|
Lu3Al3MgSiO12:0.25%Ce3+ |
450 |
552 |
86 |
— |
82 |
185
|
Lu3(Al1.5Mg0.5)(Al2.5Si0.5)O12:3%Ce3+ |
447 |
551 |
85 |
— |
83 |
186
|
Lu3(AlMg)(Al2Si)O12:3%Ce3+ |
448 |
562 |
81 |
— |
— |
186
|
Lu3(Al0.5Mg1.5)(Al1.5Si1.5)O12:3%Ce3+ |
455 |
571 |
71 |
— |
— |
186
|
Lu3Mg2Si2O12:3%Ce3+ |
460 |
571 |
66 |
— |
— |
186
|
Y3Sc2Al3O12:5%Ce3+ |
460 |
520 |
74 |
— |
— |
141
|
Y3Sc2Al2GaO12:5%Ce3+ |
445 |
510 |
65 |
— |
— |
141
|
Y3Sc2AlGa2O12:5%Ce3+ |
430 |
500 |
63 |
— |
— |
141
|
Y3Sc2Ga3O12:5%Ce3+ |
420 |
496 |
37 |
— |
— |
141
|
(Lu2Mg)(Al4Si)O12:4%Ce3+ |
434 |
533 |
47 |
— |
76 |
187
|
(Lu2Ca)(Al4Si)O12:4%Ce3+ |
438 |
527 |
68 |
— |
85 |
187
|
(Lu2Sr)(Al4Si)O12:4%Ce3+ |
445 |
511 |
78 |
— |
92 |
187 and 188
|
(Lu2Ba)(Al4Si)O12:4%Ce3+ |
445 |
511 |
81 |
— |
93 |
187
|
(Y0.95La0.05)3Al4.2(Mg,Si)0.4O12:Ce3+ |
456 |
555 |
— |
— |
65 |
162
|
Sr3Y2Ge3O12:Ce3+ |
427 |
513 |
— |
6.37 |
0 |
165
|
Y2LuCaGaAl2SiO12:6%Ce3+ |
435 |
505 |
— |
— |
— |
189
|
Ca2YHf2Ga3O12:4%Ce3+ |
398 |
479 |
26 |
— |
— |
190
|
Ca3Sc2Ge3O12:8%Ce3+ |
450 |
530 |
13 |
5.45 |
90 |
191
|
Ca2LaHf2Al3O12:5%Ce3+ |
408 |
515 |
47 |
— |
— |
192
|
Lu2Mg2Al2Si2O12:8%Ce3+ |
436 |
575 |
73 |
4.83 |
75 |
193
|
Y2Mg2Al2Si2O12:6%Ce3+ |
460 |
599 |
57 |
— |
∼85 |
194
|
Table 2 The electronic structure and PersL properties of garnet persistent phosphors. λex is the excitation wavelength. λem,max is the wavelength corresponding to the peak of emission band
Chemical composition |
λ
ex (nm) |
λ
em,max (nm) |
PersL duration |
Trap depth (eV) |
Ref. |
Y3Al2Ga3O12:1%Ce3+ |
430 |
512 |
∼20 min |
0.73, 0.86 |
183
|
Y3Al2Ga3O12:1%Ce3+,1%Pr3+ |
430 |
512 |
∼1 h |
0.69, 0.71 |
183
|
Y3Al2Ga3O12:0.5%Ce3+,0.5%Cr3+ |
460 |
505, 700 |
265 min |
0.81 |
182, 196 and 204
|
Y3Al2Ga3O12:0.2%Ce3+,0.1%Yb3+ |
455 |
520 |
138.8 h |
1.01 |
31 and 197
|
Lu2CaMg2SiGe2O12:1%Ce3+ |
460 |
568 |
∼1 s |
0 |
202
|
Gd3Al4GaO12:0.2%Ce3+,0.1%Cr3+ |
450 |
537 |
22 min |
0.56 |
205
|
Gd3Al3Ga2O12:0.2%Ce3+,0.1%Cr3+ |
450 |
528 |
126 min |
0.47 |
205
|
Gd3Al2.5Ga2.5O12:0.2%Ce3+,0.1%Cr3+ |
450 |
515 |
405 min |
0.45 |
205
|
Gd3Al2Ga3O12:0.2%Ce3+,0.1%Cr3+ |
450 |
510 |
401 min |
0.37 |
205 and 206
|
Gd3AlGa4O12:0.2%Ce3+,0.1%Cr3+ |
450 |
511 |
8 min |
0.29 |
205
|
Gd3Sc2Ga3O12:Cr3+,Eu3+/Yb3+ |
254 |
784 |
— |
— |
198
|
Gd3Sc2Ga3O12:Cr3+,Yb3+ |
254 |
784 |
— |
— |
198
|
Y3Sc2Ga3O12:0.5%Ce3+ |
440 |
500 |
1 h |
— |
203
|
Y3Sc2Ga2.5Al0.5O12:4%Ce3+ |
365 |
503 |
∼500 s |
0.62, 0.79 |
201
|
Table 3 Luminescence properties and band gap of commercial LED phosphors reviewed in this work. λex,max and λem,max are the wavelength corresponding to the peak of excitation and emission band, respectively; QY is the quantum yield; I150
°C/IRT represents the relative emission intensity at 150 °C to that at room temperature
Chemical composition |
λ
ex,max (nm) |
λ
em,max (nm) |
QY (%) |
Bandgap (eV) |
I
150 °C/IRT (%) |
Ref. |
CaAlSiN3:Eu2+ |
455 |
650 |
87 |
5.5 |
90 |
18, 79, 225 and 228–230
|
Sr0.8Ca0.2AlSiN3:0.8%Eu2+ |
455 |
628 |
83 |
— |
79 |
209 and 231
|
SrAlSiN3:0.8%Eu2+ |
455 |
610 |
— |
— |
81 |
209
|
CaAlSi1+2xN3+2xOx:2%Eu2+ |
460 |
605 |
— |
— |
80 |
223
|
Ca2Si5N8:Eu2+ |
395 |
605 |
∼35 |
5 |
40 |
232 and 233
|
Sr2Si5N8:4%Eu2+ |
450 |
626 |
79 |
4.9 |
86 |
232–234
|
Ba2Si5N8:Eu2+ |
395 |
574 |
∼75 |
4.9 |
83 |
232 and 233
|
CaSrSi5N8:2%Eu2+ |
455 |
632 |
— |
4.41 |
82 |
235 and 236
|
Sr0.5(Ca0.55Ba0.45)1.5Si5N8:2%Eu2+ |
460 |
654 |
— |
— |
96 |
237
|
La3Si6N11:6%Ce3+ |
460 |
535 |
100 |
4.0 |
∼100 |
238 and 239
|
La2.9Al0.3Si5.7N10.9:1%Ce3+ |
530 |
535, 665 |
13 |
|
55 |
239
|
CaSi2O2N2:2%Eu2+ |
355 |
560 |
76 |
6.6c |
83 |
240
|
SrSi2O2N2:2%Eu2+ |
360 |
538 |
91 |
6.67 |
99 |
240 and 241
|
BaSi2O2N2:2%Eu2+ |
380 |
494 |
71 |
6.6c |
83 |
240
|
(Sr0.5Ba0.5Eu0.02)Si2O2N2:2%Eu2+ |
450 |
548 |
92 |
— |
94 |
240
|
β-SiAlON (z = 0.18):0.02%Eu2+ |
325 |
540 |
82 |
7.2 |
97 |
242 and 243
|
Ca2SiO4:Eu2+ |
365 |
502 |
— |
5.2 |
60 |
244–248
|
Sr2SiO4:Eu2+ |
350 |
574 |
— |
5.29 |
∼80 |
142 and 249–251
|
Ba2SiO4:Eu2+ |
370 |
505 |
— |
5.5 |
57 |
142, 252 and 253
|
Ca1.2Eu0.8SiO4 |
450 |
653 |
50 |
— |
43 |
14
|
Sr0.9Ba1.1SiO4:1%Eu2+ |
394 |
530 |
90 |
— |
∼75 |
51
|
(Ba0.68Ca0.32)2SiO4:Eu2+ |
340 |
484 |
— |
— |
79 |
244
|
CaSrSiO4:0.25%Eu2+ |
450 |
620 |
68 |
— |
— |
254
|
Sr3SiO5:5%Eu2+ |
460 |
581 |
68 |
6.05 |
85 |
255–257
|
Ba3SiO5:1%Eu2+ |
350 |
504, 566 |
43 |
5.42 |
77 |
257 and 258
|
Sr2.6Ba0.4SiO5:0.02Eu2+ |
417 |
598 |
76 |
— |
— |
259
|
Sr3SiO5: Ce3+,Li+ |
415 |
540 |
79 |
6.05 |
∼50 |
5 and 259–262
|
Table 4 The electronic structure and PersL properties of persistent phosphors reviewed in this work. λex is the excitation wavelength. λem,max is the wavelength corresponding to the peak of emission band
Chemical composition |
λ
ex (nm) |
λ
em,max (nm) |
PersL duration |
Trap depth (eV) |
Ref. |
Sr0.8Ca0.2AlSiN3:0.15%Eu2+ |
254 |
628 |
9600 s |
0.86 |
94
|
Ca2Si5N8:1%Eu2+ |
365 |
610 |
150 s |
0.87 |
263
|
Sr2Si5N8:1%Eu2+ |
365 |
620 |
80 s |
0.66 |
263 and 264
|
Ba2Si5N8:1%Eu2+ |
365 |
580 |
400 s |
0.71 |
263
|
Ca2Si5N8:1%Eu2+,Tm3+ |
365 |
610 |
2500 s |
0.91 |
263
|
Sr2Si5N8:Eu2+,Tm3+ |
254 |
610 |
600 s |
0.65 |
264 and 265
|
Sr2Si5N8:0.5%Eu2+,1%H3BO3 |
254 |
610 |
— |
0.69 |
264
|
SrCaSi5N8:2%Eu2+,2%Tm3+ |
365 |
647 |
∼30 min |
— |
266
|
SrSi2O2N2:Eu2+,Ho3+ |
254 |
540 |
360 min |
1.05 |
241
|
SrSi2O2N2:Eu2+,Dy3+ |
254 |
540 |
50 min |
1.18 |
241
|
β-Sialon (z = 0.5):1.5%Eu2+ |
254 |
537 |
400 s |
0.27–0.59 |
243
|
Sr2SiO4:Eu2+,Dy3+ |
313 |
550 |
∼5 min |
0.5–0.66 |
267 and 268
|
Sr2SiO4:1%Eu2+,0.5%Tm3+ |
365 |
540 |
— |
1.35 |
269
|
Ba2SiO4:0.5%Eu2+,1%Ho3+ |
254 |
504 |
24 h |
0.71–0.86 |
253
|
Ba2SiO4:0.2%Eu2+,2%Er3+ |
360 |
510 |
402 min |
0.59, 0.78 |
270
|
Sr3SiO5: Eu2+,Dy3+ |
370 |
570 |
6 h |
0.66, 1.05, 1.21 |
271
|
Sr3SiO5:0.1%Eu2+,0.5%Nd3+ |
254 |
580 |
14 h |
0.79–1.05 |
272
|
Sr3SiO5:0.01%Eu2+,0.5%Ge3+ |
365 |
580 |
7000 s |
0.73, 0.97 |
273
|
(Sr0.88Ba0.12)3SiO5:Eu2+,Nb5+ |
254 |
590 |
17 h |
0.48–0.71 |
274
|
Ce3+-activated garnets
The Ce3+-activated garnets, as a class of highly efficient luminescent materials, have been widely used in various applications ranging from lighting and displays to persistent illumination fields.140 The garnet structures crystallize in the cubic space group Ia
d, and the crystal chemical formula is (A)3{B}2[C]3O12 where (), {}, and [] represent the dodecahedral, octahedral and tetrahedral sites, respectively.147 As shown in Fig. 11a, tetrahedral C and octahedral B form a corner-connected 3D network, with the 8-coordinating A atoms filling the remaining space. Owing to the unique crystal structure, garnet based compounds have a large variety of compositional derivatives. Recently, Song et al. borrowed theory from the perovskite structure, introducing the tolerance factor into the garnet structure for interpretation and prediction of the phase stability with the garnet structures.148 A survey of the tolerance factor over 130 garnet compounds reveals the correlation between the tolerance factor and the garnet-phase stability, demonstrating the critical role of the tolerance factor not only in understanding the crystal chemistry of garnet compounds but also in predicting the stability of the garnet phase. The various cation sites and the rich structural diversity allow Ce3+-activated garnets to be remarkably flexible in tuning and optimizing luminescence properties for specific applications. The Ce3+-activated yttrium aluminum garnet (YAG:Ce) with its blue to yellow down-conversion luminescence was first reported by Blasse & Bril in 1967 as a new phosphor for flying-spot cathode-ray tubes for color television.149 After the bright blue InGaN LED chip became available, YAG:Ce was widely used as the down-converting phosphors fabricated with the blue chips to produce white light.9 Taking the advantage of the parity-allowed electric dipole f–d transition of Ce3+, the emission of YAG:Ce features with a broad emission band ranging from 500–650 nm, as shown in Fig. 11b.11,150 Owing to the strong crystal field splitting of the 5d energy level of Ce3+, the excitation from the 2F5/2 term to the lowest-energy 5d orbital of Ce3+ occurs in a broad range of 440–480 nm, which matches well with the commercial blue LED chip, leading to its successful applications in general lighting and backlights. The improved thermal stability was successfully realized by coating an ultrathin layer of Al2O3.151
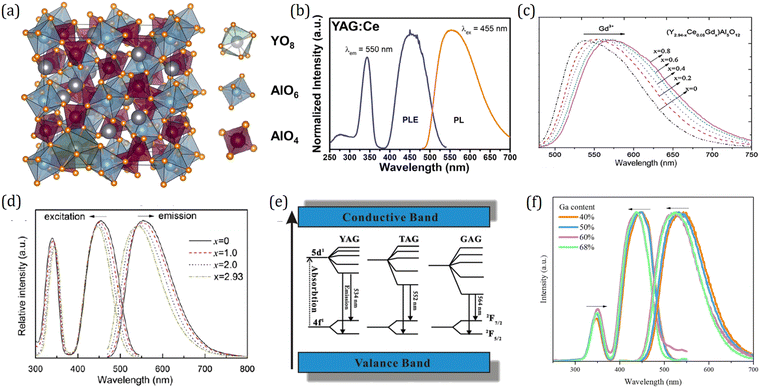 |
| Fig. 11 (a) The crystal structure of garnet phosphor Y3Al5O12:Ce3+ (YAG:Ce). Reproduced with permission from ref. 152, copyright 2013 American Chemical Society. (b) Typical excitation and emission spectra of YAG:2%Ce. Reproduced with permission from ref. 11, copyright 2020 Elsevier Ltd. (c) Emission spectra (λex = 460 nm) of (Y2.94−xCe0.06Gdx)Al5O12 phosphors for different Gd contents. Reproduced with permission from ref. 153, copyright 2011 Elsevier GmbH. (d) Excitation and emission spectra of Y2.93−xLuxAl5O12:Ce0.07 phosphors. Reproduced with permission from ref. 154, copyright 2011 Elsevier B.V. (e) Schematic energy diagram of Ce3+ in YAG, TAG, and GAG and the emission process of Ce3+. Note that in this schematic picture the variation of the bandgap with composition is not taken into account. Reproduced with permission from ref. 155, copyright 2007 The Electrochemical Society. (f) Excitation and emission spectra of Y3Al5−xGaxO12:0.5%Ce3+. Reproduced with permission from ref. 109, copyright 2017 Elsevier B.V. | |
However, pc-LEDs fabricated with sole YAG:Ce phosphor have drawbacks. One deficiency is that they are limited to cold white light due to the lack of a red spectral component. Many endeavors have been devoted to improving the emitting color of garnet phosphors by cation or anion substitution. Through replacing the dodecahedral Y3+ ions with larger ions such as Gd3+ and Tb3+,153,156 the red shifted emission can be achieved, while replacing Y3+ with smaller Lu3+ ions leads to blue shifted emission with respect to the emission of YAG:Ce.154 The emission spectra for a series of (Y2.94−xCe0.06Gdx)Al5O12 are presented in Fig. 11c as an example. As the Gd3+ concentration increases, the emission peak wavelength shifts from 532 to 568 nm, which enables (Y3−xGdx)Al5O12:Ce3+ to be applied for w-LEDs with a warmer white light. Of the various garnets, LuAG has a more condensed structure and a high effective atomic number, and thereby a high stopping power for ionizing radiation, which allows the successful application of LuAG:Ce as scintillator materials in medical imaging and security.157,158 As shown in Fig. 11d, the photoluminescence excitation (PLE) spectra of Y2.93−xLuxAl5O12:Ce3+ comprise two excitation bands including a low-energy band peaking at ∼455 nm and high-energy band peaking at ∼345 nm, which are assigned to the transitions from the 4f ground state to the 5d1 and 5d2 excited states, respectively. With increasing Lu content, the peak of the low-energy band shifts toward a shorter wavelength while that of a high-energy band shifts toward a longer wavelength, indicating that the 5d1–5d2 crystal field splitting decreases upon substitution of Y3+ by Lu3+. A schematic energy diagram for Ce3+ in LnAG (Ln = Y, Lu, Gd) is summarized in Fig. 11e. It is intriguing to find that the crystal field splitting increases as the size of the cations increases, which contradicts with the crystal field theory revealing that a larger polyhedron leads to a smaller crystal-field splitting. Extensive research has been devoted to gain insight into this so-called reverse garnet effect phenomenon, and it has been demonstrated that, besides the shape and size of the polyhedron, polyhedron distortion also has an effect on crystal field splitting and in this case it is dominant.108,116,117 In addition, the dodecahedral A site can also accommodate Ca, La, Mg, Sr and Ba atoms, giving rise to the abundant structural diversity and tunable luminescence properties of garnet phosphor.159–161 The electronic structure and luminescence properties are summarized in Table 1.
Cation substitution at the tetrahedral and octahedral Al sites in Ln3Al5O12:Ce3+ also helps in tuning their luminescence properties. Since Al3+ ions in garnets occupy two Wyckoff sites, the garnet structure has different cationic substitution modes. Previous research studies have demonstrated that the octahedral Al site can be replaced by Ga3+, Sc3+, Mg2+, Sb3+, Zr3+, and Hf3+ and the tetrahedral Al site can be replaced by Ga3+, Si4+, and Ge4+ while maintaining the garnet-type structure.140 For example, Ga can occupy both tetrahedral and octahedral sites. Replacing partial Al with Ga leads to a blue shift in both the lowest energy 4f–5d excitation and 5d–4f emission of Ce3+ (Fig. 11f), which can be understood from the view of the inductive effect.195 The emission of Y3Al5−xGaxO12:Ce3+ can be tuned gradually from yellow to green, which enables it to be fabricated together with narrow-band red-emitting phosphors to realize a wide gamut of liquid crystal display (LCD). It is noteworthy that although these substitutions improve the red component of the emission, they reduce the thermal luminescence stability (Table 1) since the structure rigidity and the bandgap structure can be changed by the cation or anion substitution. By means of temperature dependence of photoconductivity measurement, it is verified that the thermal ionization process takes the responsibility for luminescence quenching in Y3Al5−xGaxO12:Ce3+.144 Inspired by the empirical theory that the lower electronegativity of N compared with O generally leads to a larger 5d centroid shift and thereby lower energy emission, Setlur et al. successfully prepared garnet phosphors with a low energy emission by incorporating Si4+-N3− into Ce3+ doped RE3Al5O12:Ce3+ (RE = Lu, Y, and Tb).170
What is surprising is that garnet compounds also serve as superior PersL materials. Originally, Holloway and Kestigian first observed several seconds of PersL in Y3Al2Ga3O12:Ce3+ and Y3Al1.5Ga3.5O12:Ce3+ after removal of UV excitation.199 About 40 years later, Kanai et al. reported another garnet phosphor Gd3+d(Al, Ga)5−dO12:Ce3+ which exhibited PersL for hundreds of milliseconds using X-ray excitation.200 Since then, more and more Ce3+ singly doped garnets with PersL performance were reported successively such as Y3Al5−xGaxO12:Ce3+, Y3Sc2Al3−xGaxO12:Ce3+ and Lu2CaMg2(Si1−xGex)3O12:Ce3+, but the brightness and duration were still rather limited.183,201–203 A breakthrough was made by Ueda et al. in 2014.196 By introducing Cr3+ into Y3Al2Ga3O12:Ce3+ as a trap center, the green PersL of Ce3+ in Y3Al5−xGaxO12 was dramatically (∼3900 times) improved, and the duration can be reached over 5 hours after blue light excitation. They also took a quick look into the PersL of Lu3Al2Ga3O12:Ce3+,Cr3+ and Gd3Al2Ga3O12:Ce3+,Cr3+, which gave out PersL with tunable emission colors from greenish-blue to yellow, as shown in Fig. 12a. The electronic structure and PersL properties of garnet persistent phosphors are summarized in Table 2.
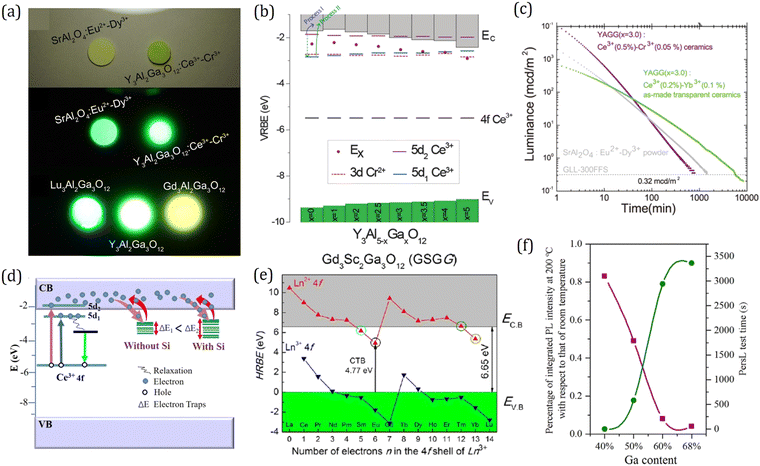 |
| Fig. 12 (a) Image of Ln3Al2Ga3O12:Ce3+ (Ln = Y, Lu, Gd) and SrAl2O4:Eu2+, Dy3+ at 5 min after ceasing the excitation. Reproduced with permission from ref. 196, copyright 2014 American Institute of Physics. (b) VRBE scheme of Y3Al5−xGaxO12:Ce3+, Cr3+. Reproduced with permission from ref. 182, copyright 2015 Royal Society of Chemistry. (c) PersL decay curves of Y3Al2Ga3O12:0.2%Ce3+, 0.1%Yb3+ transparent ceramics, Y3Al2Ga3O12:0.2%Ce3+, 0.1%Yb3+ normal ceramics, and SrAl2O4:Eu2+, Dy3+ powder after blue light charging. Reproduced with permission from ref. 31, copyright 2018 American Chemical Society. (d) VRBE schemes revealing the PersL mechanism for Y3Al2Ga3O12:Ce3+, Yb3+, B3+ phosphors with and without Si4+. Reproduced with permission from ref. 197, copyright 2020 Elsevier B.V. (e) HRBE scheme of Gd3Sc2Ga3O12. Reproduced with permission from ref. 198, copyright 2017 Wiley. (f) Correlation curves between thermal quenching performance and the longest tested duration of (Y1−xCex)3(Al1−xGax)5O12:Ce3+ samples. The time for samples with a 40% Ga content is set to zero. Reproduced with permission from ref. 195, copyright 2017 Elsevier B.V. | |
As the concept of electronic structure schemes matures, the HRBE and VRBE schemes of garnets are applied for interpreting and predicting PersL properties, and designing new potential persistent phosphors.92 Since PersL behavior is realized by the electron transfer between luminescent centers and trap centers via CB, the control of bandgap structure and trap depth are two effective strategies towards promising persistent phosphors, which are named bandgap engineering and trap depth engineering, respectively. By means of bandgap engineering, the bandgap and thereby trap depth have been systematically tuned by varying the Ga content in Y3Al5−xGaxO12:Ce3+,Cr3+.182 By constructing the VRBE scheme with energy level of the 3d electron of Cr2+ (Fig. 12b), it is revealed that, with increasing Ga content, the energy level of the CB bottom decreases while the energy level of the 3d electron of Cr2+ remains almost the same, giving rise to a smaller trap depth and thereby different PersL properties for various applications, such as phosphors with deeper traps Y3Al5−xGaxO12:Ce3+,Cr3+, x = 0–1 for energy storage and those with shallower traps Y3Al5−xGaxO12:Ce3+,Cr3+, x = 2.5–3.5 for emergency signage at room temperature. Since the ionization energy for luminescence quenching originating from thermal ionization directly affects the charging efficiency for PersL at room temperature, by comprehensively analyzing luminescence quenching and PersL properties, the optimum energy gap between the 5d1 level and the CB bottom for efficient charging is estimated to be around 0.6 eV, which can improve the PersL properties at room temperature without compromising the blue light charging efficiency and quantum efficiency. In order to extend the PersL duration of Y3Al2Ga3O12:Ce3+,Cr3+, the trap center with a deeper depth relative to Cr3+ is required. Employing trap depth engineering, Yb3+ with a lower energy level (−3.52 eV) with respect to Cr2+ (−1.99 eV) in VRBE schemes was introduced into Y3Al2Ga3O12:Ce3+ as a trap center.31 As expected the Y3Al2Ga3O12:Ce3+,Yb3+ as-made transparent ceramic phosphor shows super-long persistent luminescence for over 138.8 h after blue light charging, but its initial intensity is relatively lower compared to that of Y3Al2Ga3O12:Ce3+,Cr3+ (Fig. 12c), which is common in the case of deep traps. Recently, Zhou et al. proposed a multi-doping strategy towards outstanding PersL performance combining strong intensity and long duration through introducing wide trap distribution ranging from shallow traps for strong initial intensity to deep traps for long duration.197,207 By multi-doping B3+ and Si4+ into Y3Al2Ga3O12:Ce3+,Yb3+, the wide trap distribution from 0.47 to 1.11 eV is achieved (Fig. 12d), and the PersL performance including intensity and duration is improved substantially. As another example for trap depth engineering, Xu et al. prepared Gd3Sc2Ga3O12:Cr3+,Ln3+ garnet persistent phosphors with Cr3+ as the emission center and Ln3+ as the trap center. The lanthanide ions were selected to be Sm3+, Eu3+, Tm3+, and Yb3+ with 4f energy level of the relevant divalent ions located below the CB bottom according to the HRBE scheme as shown in Fig. 12e.198,208 Through analysis of the thermoluminescence glow curves, it was confirmed that Eu3+ and Yb3+ can introduce trap centers with different depths while Sm3+ and Tm3+ cannot, which suggests that a relatively larger energy gap between the 4f energy level of divalent lanthanide ions and the CB bottom is required for efficient electron storage. Considering that both luminescence quenching and PersL properties are related to the energy gap between the 5d1 level and the CB bottom, Song et al. investigated their correlations in the (Y1−xCex)3(Al1−xGax)5O12 system.195 As expected, a negative correlation between luminescence quenching and PersL properties is revealed (Fig. 12f), which provides us a clue that the luminescence quenching properties of LED phosphor can be regarded as a criterion to evaluate the possibility of LED phosphors possessing potential PersL performance. The development of Ce3+-activated garnets with promising properties has been established as an inspiring template for further design and modulation of novel outstanding LED and persistent phosphors.
Eu2+-activated MAlSiN3 (M = Ca, Sr)
As discussed above, conventional w-LEDs composed of blue InGaN LED chip and yellow-emitting YAG:Ce phosphor have a low color rendering index because they lack a red component. Therefore, stable red-emitting phosphors, which can be efficiently excited by blue light, are essential to compensate the red color deficiency and thereby obtain w-LEDs with warm white light. For developing red-emitting phosphors, three approaches have been recognized as promising: (1) Eu2+-activated nitrides and oxynitrides;209 (2) Eu3+-activated phosphors;210 and (3) Mn4+-activated phosphors.211 Since the line-shaped excitation band corresponding to the forbidden f–f transition of Eu3+ is not suitable for solid-state lighting for which a broad excitation band is generally required,212 and the valence state of Mn in host materials is hard to control,213 Eu2+-activated nitrides and oxynitrides are regarded as the most potential materials to be efficient red emitters.
CaAlSiN3:Eu2+ is one of the widely used red-emitting phosphors in w-LEDs due to its high quantum efficiency and outstanding thermal luminescence stability. The crystal structure of CaAlSiN3:Eu2+ was first reported by Ottinger in 2004.214 It has an orthorhombic structure with the space group of Cmc21. As shown in Fig. 13a, alternating [SiN4] and [AlN4] tetrahedra form conner-connected 3D network, with the 5-coordinated Ca2+ ions in the cavities. It has a rigid crystal structure consisting of a highly dense and corner-sharing MN4 (M = Al3+, Si4+) tetrahedral network. There are two sheets of six-membered MN4 tetrahedra rings as shown in Fig. 13b. The two sheets are 180° rotated and overlaid to form a rigid three-dimensional framework. The crystal chemical formula of CaAlSiN3 could be written as Ca(Al/Si)2NI2NII,215 in which Ca atoms occupy the 4a site, Al/Si atoms take up 8b site randomly, and NI, NII are accommodated in two different crystallographic sites of 8b and 4a, respectively. Many nitrides are crystalized in the CaAlSiN3-type structure with the general formula of AB2X2X′, such as LiSi2N3 and NaSi2N3 (with A = Li or Na, B = Si and X = X′ =N), Si2N2O (A = vacancy, B = Si, X = N, X′ = O), and SrAlSiN3 and MgAlSiN3 (A = Sr/Mg, B = Al/Si). Generally, A site could accommodate larger cations such as Li, Na, Mg, Ca and Sr ions, or vacancies. B site has various Al/Si ratios. In X′-sites O could substitute for N.
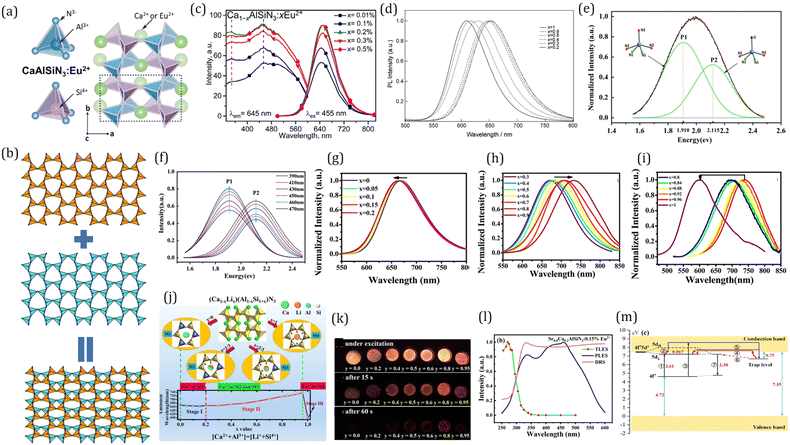 |
| Fig. 13 (a) Crystal structure of CaAlSiN3:Eu2+ with the AlN4 and SiN4 tetrahedra. Reproduced with permission from ref. 222, copyright 2018 American Institute of Physics. (b) Two 180° rotated sheets consisting of six-membered MN4 tetrahedra rings. They are overlaid to form a rigid three-dimensional framework. (c) PLE and PL spectra of Ca1−xAlSiN3:xEu2+ (x = 0.01, 0.1, 0.2, 0.3, and 0.5%). Reproduced with permission from ref. 216, copyright 2016 American Chemical Society. (d) Emission spectra of SrxCa1−xAlSiN3:Eu2+. The figures indicate Sr occupancy. Reproduced with permission from ref. 209, copyright 2008 Elsevier B.V. (e) Fitting curves by bi-Gaussian method of Ca0.625[Al0.75Si1.25]N2 (N0.85O0.15):0.02Eu. The inset figures reveal the EuNI2NII3 and EuNI2NII2O models corresponding to P1 (1.910 eV) and P2 (2.115 eV), respectively. (f) Fitting curves by the bi-Gaussian method of Ca(Al/Si)2N2(N0.8O0.2):0.02Eu. The figures indicate different excitation wavelengths. The arrows show that the relative intensity of fitting curves change with increasing excitation wavelength. With longer wavelength excitation, P1 intensities increase and P2 intensities decrease. Panels (e and f): reproduced with permission from ref. 215, copyright 2013 Elsevier B.V. The normalized emission spectra of (Ca1−xLix)0.98(Al1−xSi1+x)N3:0.02Eu2+ samples for different ranges: (g) x = 0–0.2, (h) x = 0.3–0.9, (i) x = 0.8–1. (j) Proposed model on the chemical unit co-substitution strategy represented by structural evolution of (Ca1−xLix)0.98(Al1−xSi1+x)N3:0.02Eu2+, from CaAlSiN3 (x = 0) to LiSi2N3 (x = 1) via the (LiSi)5+ substitution for (CaAl)5+ couple. It corresponds to three stages in the evolution of photoluminescence. Panels (g–j): reproduced with permission from ref. 219, copyright 2016 American Chemical Society. (k) Photographs of the persistent luminescence phenomenon in SryCa1−yAlSiN3:0.05%Eu2+ (y = 0–0.95) (up) under UV light excitation (254 nm), and (middle) 15 s and (down) 60 s after the removal of the excitation light. (l) PLE, TLE and DR spectra of Sr0.8Ca0.2AlSiN3:0.15% Eu2+. The monitoring emission wavelength is 628 nm for the PLE spectrum. The TLE spectral intensity is obtained by integration of the TL glow between 30 and 400 °C. The DR spectrum is for the Sr0.8Ca0.2AlSiN3 host. (m) Persistent luminescence mechanism for Sr0.8Ca0.2AlSiN3:xEu2+. Panels (k–m): reproduced with permission from ref. 94, copyright 2019 Elsevier B.V. | |
In 2006 Uheda et al. reported the luminescence properties of CaAlSiN3:Eu2+ synthesized under high temperature and pressure, and pointed out its great potential as a red-emitting phosphor for practical application in w-LEDs with a high color rendering index.18 As displayed in Fig. 13c, CaAlSiN3:Eu2+ exhibits a broad excitation band ranging from UV to blue light, and under 455 nm excitation it gives out a deep-red emission peaking at ∼650 nm.216 In order to save energy and reduce cost, self-propagating high-temperature and low-temperature alloy-nitridation synthesis methods were successively developed to prepare high-performance CaAlSiN3:Eu2+ phosphors.79,217,218 For lighting applications with the aim of both high color rendering and efficiency, deep-red emission is desired to be shifted to shorter wavelengths. For this purpose, Watanable et al. replaced partial Ca in CaAlSiN3:Eu2+ by larger Sr ions to weaken the crystal field strength around Eu2+ and thus tune the emission color.209 The solid solutions Eu2+-doped SrxCa1−xAlSiN3 (0 ≤ x ≤ 1) with an isotopic structure of CaAlSiN3 were synthesized under high pressure and temperature. As x increases, the Eu2+ emission gets a blue shift from 650 nm to 610 nm due to the decrease in the crystal field strength caused by unit-cell expansion (Fig. 13d). What is surprising is that the x = 0.8 sample Sr0.8Ca0.2AlSiN3 with the optimum Eu2+ concentration has high internal quantum efficiency (80–83%) and external quantum efficiency (70%), which are comparable to that of the x = 0 sample CaAlSiN3. In addition, by replacing partial N with O, the blue shift of the emission wavelength can also be achieved in Ca(Al/Si)2N2(N1−xOx):Eu2+.215 Fitting of the emission spectra into two sub-Gaussian peaks P1 and P2 (Fig. 13e) suggests two types of Eu2+ luminescent centers with different ligand environments: EuNI2NII3 and EuNI2NII2O tetrahedra.18,79,215 By increasing the O concentration, P1 is intensified while P2 weakens (Fig. 13f), indicating that oxygen atoms only substitute for one kind of N atoms, i.e., NII at the 4a crystallographic site.215 Co-substitution of the (LiSi)5+ pair for the (CaAl)5+ pair in the CaAlSiN3 host forms a completely miscible solid solution, i.e., (Ca1−xLix)0.98(Al1−xSi1+x)N3. The Eu2+-doped samples exhibit dark red emission with a broad band peaking at about 650–700 nm apart from the compound with x = 1, i.e., LiSi2N3:Eu2+, as shown in Fig. 13g–i. The 3-stage spectral evolution depends on chemical compositions, which could be explained by local structural evolution of the (Ca1−xLix)0.98(Al1−xSi1+x)N3:0.02Eu2+, from CaAlSiN3 (x = 0) to LiSi2N3 (x = 1) via the (LiSi)5+ substitution for (CaAl)5+ couple as shown in Fig. 13j.219
It is worth mentioning that there are some well-known Eu2+-doped aluminate phosphors, such as BaMgAl10O17:Eu2+ and BaAl2O4:Eu2+, showing potential for LED applications.220,221 Fine-tuning of the luminescence properties may be achieved by modification of the phosphors as a result of the incorporation of nitrogen.
Compared with the well commercialized green-emitting and blue-emitting persistent phosphors, such as SrAl2O4:Eu2+,Dy3+, CaAl2O4:Eu2+,Nd3+, Sr2MgSi2O7:Eu2+,Dy3+, and Sr4Al14O25:Eu2+,Dy3+, red-emitting persistent phosphors with efficient PersL properties are relatively deficient. Although red PersL phenomena have been observed in some oxides, sulfides, and oxysulfides, such as Y2O2S:Eu3+,Mg2+,Ti3+, CaS:Eu2+,Ti3+,Ce3+, Ca3Ti2O7:Pr3+, Sr2ZnSi2O7:Sm3+, and β-Zn3(PO4)2:Mn2+,Sm3+/Al3+/Ga3+, their applications are highly restricted due to either low chemical stability or poor PersL performance.223–227 Hence, developing efficient red-emitting PersL phosphors has attracted continuous attention. Note that a lot of persistent phosphors are original LED phosphors, and their PersL properties can be effectively improved by controlling defect concentration and introducing trap centers. Therefore, exploring the potential PersL possibilities of red-emitting LED phosphors has been focused on as an effective approach for the discovery of new promising red PersL materials. Initially, the red PersL phenomenon in CaAlSiN3:Eu2+ was accidently observed by Ueda et al. in 2018 when they studied its luminescence quenching mechanism.228 Shortly after, while Suda et al. studied the origin of the abnormal blue emission of the red phosphor CaAlSiN3:Eu2+ at low Eu2+ concentration, the red PersL phenomenon was observed again, and it is proposed that the nitrogen vacancies which can capture electrons take the responsibility for both the blue emission and the red PersL from Eu2+.222 In 2019, Liu et al. systemically investigated the PersL properties of SryCa1−yAlSiN3 (0 ≤ y ≤ 0.95) doped with various Eu2+ concentrations under UV light excitation, and found that the y = 0.8 sample Sr0.8Ca0.2AlSiN3 doped with 0.15% Eu2+ shows the best red PersL performance with a emission peak at ∼628 nm and a duration of 9600 s at the 0.32 mcd m−2 threshold value (Fig. 13k).94 By comparing the thermoluminescence excitation spectrum (TLES) to the photoluminescence excitation spectrum (PLES) (Fig. 13l), a new PersL mechanism different from that of SrAl2O4:Eu2+,Dy3+ was proposed. In the trapping process, the electrons captured by the traps are first excited from the 4f states to the 5d states of Eu2+ and then thermally stimulated to CB in SrAl2O4:Eu2+,Dy3+, while in Sr0.8Ca0.2AlSiN3:Eu2+ the electrons are directly excited from the 4f states or VB to CB and then captured by the traps (Fig. 13m). Looking forward, future work on exploring the appropriate rare-earth or transition metal co-dopant as the trap center would improve the PersL performance, providing opportunities for Sr0.8Ca0.2AlSiN3:Eu2+ toward commercial PersL applications.
Eu2+-activated M2Si5N8 (M = Ca, Sr, Ba)
Eu2+-activated M2Si5N8 (M = Ca, Sr, Ba), as a class of high-performance orange-red emitting phosphors, have been successfully commercialized in w-LED applications due to their high efficiency and high color purity under near-UV to blue light excitation and excellent thermal luminescence stability up to 200 °C. The structures of M2Si5N8 (M = Ca, Sr, Ba) were first reported by Schnick et al. in 1995.275,276 M2Si5N8 (M = Sr, Ba) phosphors share the same orthorhombic structure in the space group of Pmn21, while Ca2Si5N8 crystalizes in the monoclinic structure with a space group of Cc. The structures of all these three compounds are constructed by [SiN4] tetrahedra through corner sharing, as shown in Fig. 14a. What is different is that Sr2+ and Ba2+ ions are located in cavities formed by Si6N6 rings parallel along the [100] direction whereas Ca2+ ions are accommodated in the structure along the channels of the [010] direction. In 2000, van Krevel first reported the orange-red Eu2+ emission of M2Si5N8 (M = Ca, Sr, Ba) under blue light excitation.277 The character of blue light excitation can be attributed to the large covalency of nitrogen and thereby a larger centroid shift of the 5d energy levels of Eu2+. Later, Höppe et al. studied the luminescence properties of Ba2Si5N8 with various Eu2+ concentrations and ascribed the red shift of the emission maxima with increasing Eu2+ concentration to the reabsorption process of Eu2+.78 After that, dozens of research studies came up, systematically investigating the effect of M cation and Eu2+ concentration on the luminescence properties of M2Si5N8 (M = Ca, Sr, Ba):Eu2+ (Fig. 14b).232,235 The maximum solubility of Eu2+ in Ca2Si5N8 is about 7 mol%, whereas M cation in M2Si5N8 (M = Sr, Ba) can be completely substituted by Eu2+ due to the isostructural character of M2Si5N8 (M = Sr, Ba) and Eu2Si5N8. By varying the composition of different M cations and Eu2+ concentrations, the emission wavelength of M2Si5N8 (M = Ca, Sr, Ba):Eu2+ can be tuned within a wide range from 570 nm to 680 nm. It was proposed that the emission band shift caused by both different M cation compositions and Eu concentrations originates from the difference in the Stokes shift, which is generally affected by the cation size and the structural rigidity. Among them, Sr2Si5N8:Eu2+ exhibits the highest quantum yield of 79% at room temperature and the best luminescence thermal stability with only a few percent of quantum efficiency loss up to 150 °C (Table 3).234,278 In 2017, Lin et al. further studied the effect of the cation disorder on the luminescence properties in Sr1.98−x(Ca0.55Ba0.45)xSi5N8:2% Eu (x = 0–1.5) systems, demonstrating that replacing Sr by the (Ca0.55Ba0.45) mixture with the same average radius as Sr but increased cation size variance results in the increased quantum efficiency and thermal stability of Eu2+ emission.237 It was proposed that cation disorder can suppress nonradiative processes through disruption of lattice vibrations and create deep traps that release electrons to compensate for thermal quenching, which provides a fresh perspective for performance optimization of luminescent materials. Since high chemical stability and minimal thermal degradation are the prerequisites for practical applications, it is revealed that a higher degree of cross-linking between Si(O,N)4 tetrahedral enhances the chemical stability of M–Si–N compounds and the oxidation resistance of Ln–Si–O–N and Ce–Si–O compounds.279,280 It has been demonstrated that the degradation stability of Sr2Si5N8:Eu2+ can be enhanced by deposition of an ultra-thin Al2O3 coating using atomic layer deposition in a fluidized bed reactor.281
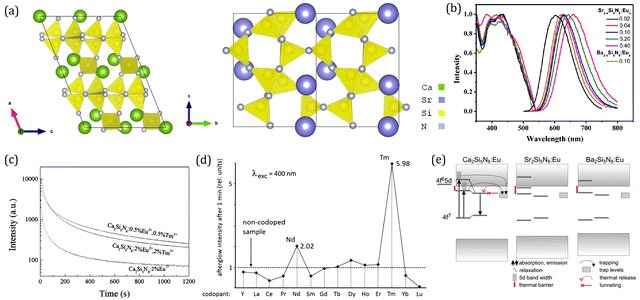 |
| Fig. 14 (a) Crystal structure of M2Si5N8 (M = Ca, Sr). (b) Emission spectra and excitation spectra of Sr2−xSi5N8:Eux with varied contents from x = 0.02–0.40 and Ba2−xSi5N8:Eux (x = 0.10). Reproduced with permission from ref. 282, copyright 2012 American Chemical Society. (c) Afterglow decay curves of the Ca2Si5N8:2% Eu2+, Ca2Si5N8:2% Eu2+, 2% Tm3+ and Ca2Si5N8:0.5% Eu2+, 0.5% Tm3+ phosphor λex = 400 nm and λem = 600 nm. Reproduced with permission from ref. 32, copyright 2010 The Electrochemical Society. (d) Afterglow intensity of Ca2Si5N8:Eu,R 1 min after excitation with 400 nm light, as a function of the co-dopant. Reproduced with permission from ref. 263, copyright 2011 Multidisciplinary Digital Publishing Institute. (e) Energy level scheme for M2Si5N8:Eu persistent phosphors. Reproduced with permission from ref. 96, copyright 2011 Elsevier B.V. | |
The PersL performance of M2Si5N8 (M = Ca, Sr, Ba):Eu2+ was first discovered in Ba2Si5N8:Eu2+, but its PersL duration is pretty short of about 15 min under the naked eye.78 Until 2009, Miyamoto et al.33 and Eeckhout et al.283 independently reported PersL in Ca2Si5N8:Eu2+, and found that its PersL intensity and duration can be enhanced by co-doping with Tm3+ as shown in Fig. 14c. Although the PersL duration of Ca2Si5N8 with a single Eu2+ dopant is only 150 s under 400 nm excitation taking 0.32 mcd m−2 as the threshold; it can be increased to 1 h upon co-doping with Tm3+, which is comparable with the conventional red-emitting persistent phosphor Y2O2S:Eu3+,Mg2+,Ti3+ but with better luminescence and chemical stability.32 After that, Eeckhout et al. further investigated the PersL behavior in the M2Si5N8 (M = Ca, Sr, Ba):Eu2+ family and thoroughly studied the effect of the rare-earth co-dopant, dopant concentration, and starting materials on their PersL properties.263 All of the samples show PersL after 300 nm irradiation, but it is weak for all the M = Sr samples. Ca2Si5N8:Eu2+,Tm3+ shows the brightest and longest PersL duration while Dy3+, Nd3+, Ho3+ or Sm3+ co-dopant scarcely improves the PersL performance of Ca2Si5N8:Eu2+ (Fig. 14d), and thermoluminescence measurements indicate that the addition of Tm3+ in Ca2Si5N8:Eu2+ deepens the relevant charge trap to a more suitable depth for improved PersL performance while others do not. It was verified by the HRBE scheme of Ca2Si5N8 constructed soon after.233 The energy gap between the 4f level of Tm3+ and the CB bottom is well consistent with the trap depth introduced by Tm3+ of about 0.91 eV extracted from thermoluminescence spectra, which is suitable for PersL at room temperature. Whereas, trap depth introduced by Dy3+, Nd3+, Ho3+ or Sm3+ inferred from the HRBE scheme is too shallow to contribute to PersL at room temperature. It was also suggested that the dopant and co-dopant in their fluoride form with concentrations not higher than 1 mol% work better for PersL performance. In addition, Teng et al. reported that the PersL properties of Sr2Si5N8:Eu2+ can also be enhanced by co-doping Tm3+.265 Inspired by the excellent PersL performance of SrAl2O4:Eu2+,Dy3+,B3+, our group further improved the PersL performance of Sr2Si5N8:Eu2+,Tm3+ by co-doping B3+ and Zhang et al. improved that of Ca2Si5N8:Eu2+,Tm3+ by co-doping Dy3+.264,284 Apart from emergency signage, Ca2Si5N8:Eu2+,Tm3+ also shows potential for application in in vivo imaging, by following the PersL of nano-sized particles which were excited just before injection.285
Combining thermoluminescence excitation spectroscopy and a study on the influence of temperature during excitation, Smet et al. studied the trap filling behavior in M2Si5N8:Eu2+ (Tm3+) in depth and addressed the crucial role of the thermal barrier between the 5d excited state of Eu2+ and the CB bottom in PersL performance.96 They showed that the traps relevant for PersL can be filled after electrons are excited into the 5d energy levels of Eu2+, and for those into lower 5d levels, a thermal barrier is present before trapping can occur. By virtue of HRBE schemes, it is demonstrated that Ca2Si5N8:Eu2+,Tm3+ being the phosphor with the best PersL performance upon excitation with visible light has the smallest thermal barrier (Fig. 14e), which makes it easy for electrons to be captured by the trap and then released to give out PersL. Note that this thermal barrier also takes responsibility for thermal luminescence stability. Hence, the large thermal barrier endows Sr2Si5N8:Eu2+ with the best thermal luminescence stability, enabling it to be developed as a high-performance LED phosphor, while Ca2Si5N8:Eu2+ with a small thermal barrier exhibits the best PersL performance. This work provides a clue that the quest for Eu2+-based persistent phosphors needs to focus on those compounds with a relatively low thermal quenching temperature, as this makes the trap filling process easier.
Ce3+-activated La3Si6N11
Yellow emitting La3Si6N11:Ce3+ is an important commercial phosphor for high-power w-LEDs due to its excellent thermal luminescence stability (almost no emission loss at 150 °C) and sufficient emission efficiency (nearly 100%).238,286 The crystal structure of La3Si6N11 was first established in 1983 in the compound Sm3Si6N11.287 It crystallizes in the tetragonal space group P4bm. The structure consists of La layers and SiN4 tetrahedra. As shown in Fig. 15a, the SiN4 groups are connected via corner sharing, forming a three-dimensional network, in which the La atoms occupy two different cavities both coordinated by eight nitrogen neighbors. As the problem of underestimation of the band gap for first principles density functional theory (DFT) calculations has been reduced by more recent first principles approaches, it is revealed that for M–Si–N compounds the top of the VB band is composed of N atoms with the lowest coordination number with Si, implying that the band gap of the M–Si–N compounds becomes higher for increasing degree of cross-linking between SiN4 tetrahedra.288–291 It is also demonstrated that a higher Si/N ratio generally leads to a higher Eu2+ 4f–5d absorption energy, a higher 5d–4f emission energy and a larger Stokes shift.292 Under 460 nm excitation, La3Si6N11:Ce3+ shows a broad-band emission peaking at 535 nm with an FWHM of 112 nm (Fig. 15b).293 With regard to the luminescence mechanism, several different viewpoints have been put forward. George et al. believed that Ce3+ on different La3+ sites exhibit similar emission properties for their identical La/Ce–N coordination numbers and similar bond lengths,238 whereas Jia et al. proposed that the yellow emission could be ascribed to CeLa(2), and no luminescence in CeLa(1) for its 5d excited states immersed into the conduction band (CB) based on DFT calculations.294 Coincidentally, in 2015, Park et al. prepared La3Si6N11:Ce3+ with a low Ce3+ concentration to study its decay behavior and observed an extra blue emission ranging from 400 to 475 nm under 355 nm excitation.295 Based on this phenomenon, combining with both theoretical and experimental methods, Zhong et al. ascribed the yellow emission to CeLa(2) and the blue emission to CeLa(1).296
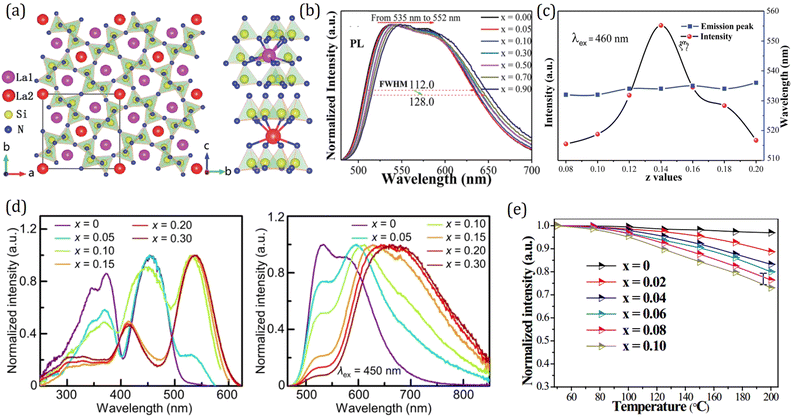 |
| Fig. 15 (a) Crystal structure and local structure around La sites of La3Si6N11. (b) Normalized emission spectra of La2.86−xYxSi6N11:0.14Ce3+ (x = 0–0.9) phosphors under 460 nm excitation. (c) Emission intensity and peak location of La3−zSi6N11:zCe3+ with varying Ce3+ concentrations (z = 0–0.2). Panels (a–c): reproduced with permission from ref. 293, copyright 2016 Royal Society of Chemistry. (d) Excitation spectra monitored at 580, 597, 612, 627, 643 and 664 nm, respectively, and emission spectra under 450 nm excitation of La2.9Si6−xAlxN11−x/3:0.1Ce3+ (x = 0–0.3). Reproduced with permission from ref. 239, copyright 2020 American Chemical Society. (e) Temperature-dependent normalized integrated PL intensities of La2.9Si6−xAlxN11−x/3:0.1Ce3+ (x = 0–0.3). Reproduced with permission from ref. 297, copyright 2017 Royal Society of Chemistry. | |
Compared with the YAG:Ce3+ phosphor, La3Si6N11:Ce3+ exhibits superior thermal luminescence stability and high quantum efficiency due to the high rigidity of the host.238,293 However, it suffers from the low color rendering and high color temperature of the fabricated devices resulting from its greenish yellow emission (peaking at 535 nm), and also the pure phase requires harsh synthesis conditions.293 Therefore, extensive research studies have been devoted to optimizing the synthesis process and tuning the luminescence properties. In 2017, Chen et al.298 and Suehiro et al.299 reported that incorporating M (M = Ca, Sr, Ba) at La sites in La3Si6N11:Ce3+ could not only improve the sample purity but also lead to the slight red shift of emission with an increased external quantum efficiency up to ∼42%. Du et al. also made a great effort to shift the emission of La3Si6N11:Ce3+ toward a longer wavelength, including varying Ce3+ concentrations, Y3+ substitution for La3+, and Al3+ substitution for Si4+, but unfortunately the effect was fairly insignificant either way.293,297 As shown in Fig. 15b and c, the emission spectra of La3−z−xYxSi6−yAlyN11−y/3:zCe3+ (z = 0.14, y = 0) show a very small red shift from 535 nm to 552 nm as the substituting Y3+ concentration x increases from 0 to 0.9, and no obvious change in the emission peak could be observed with either different Ce3+ concentrations (0 ≤ z ≤ 0.2) or Al substitution (0 ≤ y ≤ 0.1). Recently, almost at the same time, You et al.239 and Nitta et al.300 reported that an efficient red shift can be achieved by increasing the substituting Al3+ concentration (Fig. 15d). Under 530 nm excitation, La2.9Si6−xAlxN11−x/3:0.1Ce3+ (x = 0.3) shows red emission peaking at ∼650 nm, but the thermal luminescence stability decreases as Al3+ concentration increases (Fig. 15e). Through DFT calculation, You et al.239 believed that this unusual red emission band originates from the interstitial Ce3+ site occupying the [Si8N8] void in the c ∼ 1/2 layer, created as a charge compensator for the aliovalent Al3+ substitution for Si4+. Therefore, the interstitial site engineering was proposed, for the first time, as an efficient strategy for phosphor design, and the aliovalent ion co-doping was proved to be an effective way to create interstitial sites.
Another characteristic of the Ce3+ luminescence in La3Si6N11 is the neutralization of cationic substitution on the excitation peak shift. As reported by Du et al., both yttrium and cerium substitution for lanthanum almost have no effect on the peak shift of excitation spectra, as shown in Fig. 16a and b.286,293 In contrast, the lattice parameter and bond lengths demonstrate significant changes depending on cationic substitution contents. This anomaly has been investigated by crystal structure modelling and crystal-field calculation.301 The real coordination polyhedron is simplified to a truncated square pyramid with point symmetry C4v. The different sizes of the parallel top and down squares, together with the pyramid height serve as the varying coefficient in expressing the split crystal-field levels. From the simulation results, it is found that specific geometrical shapes have the effect of freezing the crystal-field levels on cationic substitution, regardless of the significant bond-length variance. Further investigation of the orbital shape in Fig. 16c reveals possible explanation of the crystal-field passivation, i.e., the diminished influence of the expanding square on z-oriented orbitals dz2, dxz and dyz.
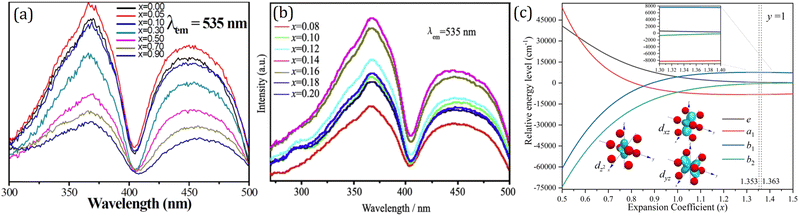 |
| Fig. 16 (a) PLE spectra of La2.86−xYxSi6N11:0.14Ce3+ with different Y contents. Reproduced with permission from ref. 293, copyright 2016 Royal Society of Chemistry. (b) PLE spectra of La3−xSi6N11:xCe3+ with different cerium contents. Reproduced with permission from ref. 286, copyright 2017 Elsevier B. V. (c) Schematic orbital shape and the passivation of crystal-field effect. Reproduced with permission from ref. 301, copyright 2020 Royal Society of Chemistry. | |
Eu2+-activated UCr4C4-type compounds
UCr4C4-type compounds have been investigated for narrow band emitting phosphors due to their highly condensed, rigid framework structure. The general formula of UCr4C4-type structures is written as M(A,B)4X4. [AX4] and [BX4] tetrahedra connect to each other by vertex- and edge-sharing to form the highly condensed three-dimensional network with the degree of condensation k = 1 (k equals to the atomic ratio (A,B):X). M cations (alkali/alkali-earth metal ions) fill in a highly symmetric cube-like site within vierer ring channels along the [001] direction.11 The narrow band emitting phosphors based on the UCr4C4 structure were first discovered by Schnik's group. They reported a series of Eu2+-doped nitridoaluminates which give out narrow band red emitting characteristics, such as M[LiAl3N4]:Eu2+ (M = Ca, Sr),1,302 M[Mg2Al2N4]:Eu2+ (M = Ca, Sr, Ba),303 and Sr[Mg3SiN4]:Eu2+.304 Among them, SrLiAl3N4:Eu2+ shows the most superior luminescence properties. As shown in Fig. 17a, the ordered edge- and corner-sharing AlN4 and LiN4 tetrahedra with channels of vierer rings along [011] form the highly condensed and rigid framework of Sr[LiAl3N4].1 There are two crystallographic Sr sites, each coordinated by eight N atoms in a highly symmetric cuboid-like environment. The Eu2+ dopant is expected to replace Sr ions.305 Sr[LiAl3N4]:Eu2+, which can be efficiently excited by GaN-based blue LEDs, shows highly efficient red emission peaking at ∼650 nm with a full-width at half-maximum of ∼50 nm and pretty low thermal quenching (>95% relative to the quantum efficiency at 200 °C) (Fig. 17b). The emission peak position of Sr[LiAl3N4]:Eu2+ is similar to that of commercial CaAlSiN3:Eu2+, but the significantly narrowed bandwidth can improve the color gamut and concentrate the emission within the visible spectral region. The color gamut of LED fabricated by β-SiAlON:Eu2+, Sr[LiAl3N4]:Eu2+ with a blue LED chip reaches up to 84.2% of NTSC in CIE 1931 after passing through the color filter.306 By DFT calculations, the electronic structure of Sr[LiAl3N4] was investigated and it was revealed that the DOS of the two non-equivalent Sr sites shows a high degree of similarity, contributing to the narrow band emission of Sr[LiAl3N4]:Eu2+ (Fig. 17c).60,307 The PersL properties of Sr[LiAl3N4]:Eu2+ were also explored. After 254 nm light pre-irradiation, Sr[LiAl3N4]:0.1%Eu2+ phosphor shows 395 s red PersL at a 0.32 mcd m−2 threshold value as shown in Fig. 17d.308
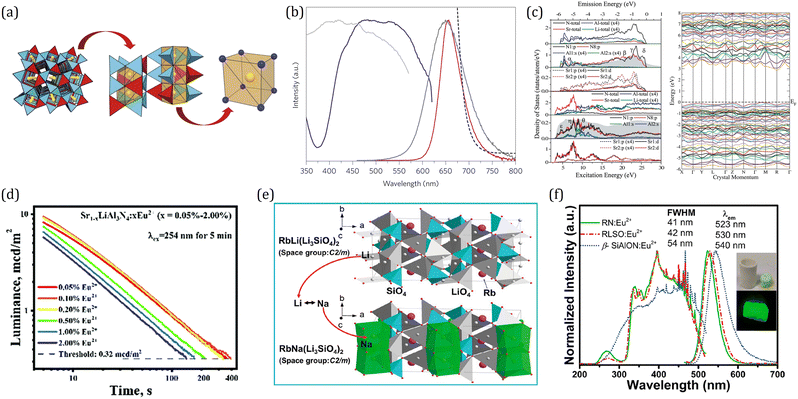 |
| Fig. 17 (a) Crystal structure of SrLiAl3N4. Yellow spheres are Sr; blue tetrahedra are AlN4; red polyhedral are LiN4; and blue spheres are N. (b) Excitation (Sr[LiAl3N4]:Eu2+, blue; CaAlSiN3:Eu2+, light grey) and emission spectra for λexc = 440 m of Sr[LiAl3N4]:Eu2+ (pink) and CaAlSiN3:Eu2+ (dark grey). The dotted curve indicates the upper limit of sensitivity of the human eye. (c) Calculated DOS in the VB (upper half of left panel) and CB (lower half of left panel) and the band structure (right panel) of Sr[LiAl3N4]. (d) The persistent decay curves (log–log plot) of Sr[LiAl3N4]:Eu2+ after 254 nm light irradiation for 5 min at RT. (e) Crystal structures of RbLi(Li3SiO4)2:Eu2+ and RbNa(Li3SiO4)2:Eu2+. (f) Excitation and emission spectra of RbLi(Li3SiO4)2:Eu2+ (RLSO:Eu2+), RbNa(Li3SiO4)2:Eu2+ (RN:Eu2+), and β-SiAlON:Eu2. Panels a and b: reproduced with permission from ref. 1, copyright 2014 Macmillan Publishers Limited. Panel c: reproduced from ref. 307, copyright 2015 Willy-VCH. Panel (d): reproduced from ref. 308, copyright The Royal Society of Chemistry 2020. Panels e and f: reproduced from ref. 312, copyright 2019 Willy-VCH. | |
Based on the insight into nitridoaluminates with the UCr4C4-type structure, Huppertz and co-workers together with OSRAM Opto Semidonductors issued the patent WO 2018/029304 A1 on the alkali lithosilicate phosphors in Germany, where several narrow band emitting representatives with the UCr4C4-type structure were described.309 They also reported that alkali metal silicates such as Na[Li3SiO4]:Eu2+ and K[Li3SiO4]:Eu2+ show efficient narrow band emission.310 Almost at the same time, Xia et al. independently reported a series of Eu2+-doped oxidesilicate phosphors with the UCr4C4-type structure which exhibit narrow band emitting characteristics, such as RbLi(Li3SiO4)2:Eu2+,311 RbNa(Li3SiO4)2:Eu2+,312 RbNa3(Li3SiO4)4:Eu2+,313 and Na0.5K0.5Li3SiO4:Eu2+.314 RbLi(Li3SiO4)2:Eu2+ exhibits a narrow band green emission peaking at 530 nm with the full width of highest maximum of 42 nm. Later, by cation substitution strategy, RbNa(Li3SiO4)2:Eu2+ was designed via partially substituting Li by Na in RbLi(Li3SiO4)2. It shows similar luminescence properties to RbLi(Li3SiO4)2:Eu2+ with emission peaking at 523 nm and the full width of highest maximum of 41 nm. Both phosphors share a similar crystal structure with the monoclinic system and a space group of C2/m (Fig. 17e). Compared with the commercial phosphor β-SiAlON:Eu2+, the PL spectra of RbLi(Li3SiO4)2:Eu2+ and RbNa(Li3SiO4)2:Eu2+ show narrower emission band (Fig. 17f), and thus the color gamut of RbLi(Li3SiO4)2:Eu2+ and RbNa(Li3SiO4)2:Eu2+ based LED is 107% and 113% of NTSC, respectively, which is larger than that of β-SiAlON:Eu2+ based LED. These inspiring breakthroughs in narrow band emitting phosphors provide more opportunities for wide color gamut LCD technology. However, these Eu2+-doped UCr4C4-type phosphors suffer from poor chemical stability. Even though endeavors have been devoted to improve the chemical stability by hydrophobic modification, its commercial application is still restricted.315,316 Further work for improving the chemical stability of Eu2+-doped UCr4C4-type phosphors is highly required to realize their practical applications.
Eu2+-activated MSi2O2N2 (M = Ca, Sr, Ba)
Oxynitridosilicates, also known as siones, represent a class of solid compounds, which can be formally derived from oxysilicates by partial substitution of oxygen by nitrogen.70 These materials combine attractive material properties like high mechanical hardness and strength, and great thermal and chemical stability. Meanwhile, higher condensed siones are known to be highly covalent and stable towards oxidation and hydrolysis. Among them, MSi2O2N2 (M = Ca, Sr, Ba) have been well developed as famous phosphors applied in LED applications by doping with Eu2+. This family of compounds is constructed with silicate layers separated by M2+ layers.317–319 As an example, Fig. 18a shows the triclinic structure of SrSi2O2N2 that crystallizes in space group P1. The CaSi2O2N2 crystallizes in the monoclinic space group P21, whereas BaSi2O2N2 crystallizes in the orthorhombic space group Cmcm. Each of the four M sites in MSi2O2N2 (M = Ca, Sr) is coordinated with six oxygen ions and one nitrogen ion. When doped with Eu2+, these compounds exhibit a broad excitation spectrum covering the UV-blue range (250–460 nm), and yield efficient emission in the blue-green to yellow region. As shown in Fig. 18b, CaSi2O2N2:Eu2+ shows yellow emission with a maximum at 560 nm, and green-yellow emission over 530–570 nm is observed in SrSi2O2N2:Eu2+ depending on the O/N ratio and Eu2+ concentration.240,320 Remarkably, BaSi2O2N2:Eu2+ shows blue-green emission with a narrow emission band at 494 nm, which indicates its potential application in LED backlights. The high quantum yield (90%) and high quenching temperature (>500 k) of SrSi2O2N2:Eu2+ were also verified, demonstrating that it is a promising material for pc-LED application.
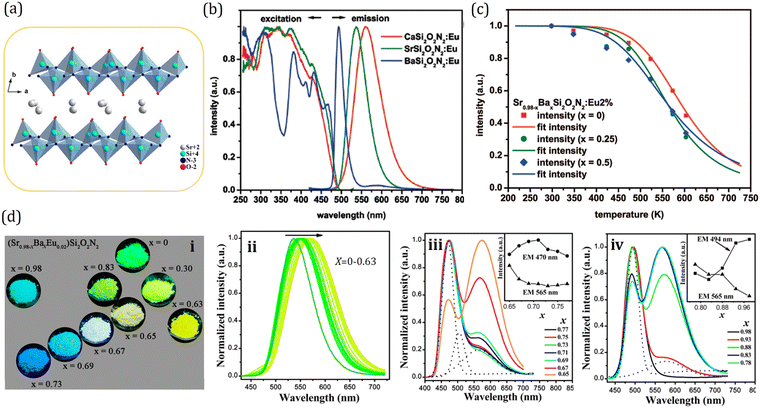 |
| Fig. 18 (a) Crystal structure of SrSi2O2N2. Reproduced with permission from ref. 241, copyright 2017 American Chemical Society. (b) PLE and PL spectra of 2% Eu2+ doped CaSi2O2N2, SrSi2O2N2, and BaSi2O2N2 phosphors, as recorded at 4 K; PL spectra were taken upon excitation at 450 nm; PLE spectra were obtained at the corresponding emission maxima. (c) Temperature dependence of the integrated emission intensity Sr0.98−xBaxSi2O2N2:Eu 2% (x = 0, 0.25, 0.5). Panels (b and c): reproduced with permission from ref. 240, copyright 2009 American Chemical Society. (d) Normalized PL emission spectra (λex = 405 nm): (i) digital phosphor images upon irradiation using a 365 nm UV lamp of the typical (Sr0.98−xBaxEu0.02)Si2O2N2 phosphors; (ii) x = 0–0.63; (iii) x = 0.65–0.77; and (iv) x = 0.78–0.98. Reproduced with permission from ref. 321, copyright 2014 American Chemical Society. | |
To improve the color rendering index, correlated color temperature, and thermal luminescence stability of MSi2O2N2 (M = Ca, Sr, Ba):Eu2+ phosphors, cation substitutions and Eu2+ concentration variation have been used to adjust their luminescent properties.240,321 As Eu2+ concentration increases upon 2%, the emission gets a red shift, which can be attributed to the energy migration and energy transfer to Eu2+ ions emitting at longer wavelengths. Unfortunately, the lowering of quantum efficiency and thermal quenching temperature due to concentration quenching occurs along with the red shift. On the other hand, partial substitution of Sr2+ by either Ca2+ or Ba2+ also results in a red shifted Eu2+ emission. Similar to the case of increasing Eu2+ concentration, the quenching temperature drops upon substitution of Sr by Ca. What is exciting is that the quantum efficiency and quenching temperature remain high for Ba substitution, as shown in Fig. 18c and Table 3. For Ca2+ substitution, the red shift is expected and can be understood by an increased crystal field splitting for Eu2+ on the smaller Ca2+ cation site. Whereas, the red shift from substitution of Sr2+ with Ba2+ cation is intriguing since a blue shift of the f–d emission of Eu2+ is generally observed in case of substitution of Sr2+ by the larger Ba2+ cation. In order to explore the underlying mechanism of this abnormal red shift, Li et al. conducted a detailed work on the phase structure and tunable luminescence of (Sr1−xBax)Si2O2N2:Eu2+ (0 ≤ x ≤ 1). The multicolor emission of (Sr0.98−xBaxEu0.02)Si2O2N2 (0 ≤ x ≤ 1) phosphors can be clearly observed upon irradiation using a 365 nm UV lamp as displayed in Fig. 18d–i. By virtue of X-ray diffraction measurements and first-principles calculations, it is revealed that the crystal structures of (Sr1−xBax)Si2O2N2:Eu2+ (0 ≤ x ≤ 1) can be nominally divided into three sections, namely, Phase 1 (0 ≤ x ≤ 0.65), Phase 2 (0.65 < x < 0.80), and Phase 3 (0.80 ≤ x ≤ 1). Through extended X-ray absorption fine structure analysis, it is proposed that the abnormal red shift in Phase 1 with gradual replacement of Sr2+ by Ba2+ (Fig. 18d-ii) is caused by the Sr(Eu)-O/N bond length shrinkage in the local structure. The controllable luminescence in Phase 2 from blue to white (Fig. 18d-iii) and Phase 3 from cyan to yellowish green (Fig. 18d-iv) is attributed to phase segregation based on the high-resolution transmission electron microscopy and selected area electron diffraction analysis. This work serves as a guide in developing oxynitride luminescent materials with controllable optical properties based on variations in local coordination environments through cation substitutions.
The green-yellow PersL in SrSi2O2N2:Eu2+ was first reported by Zhang et al. in 2013.322 It was in 2018 that Zhuang et al. investigated its potential for information storage applications.241 Different from room temperature applications, the information storage applications require PersL materials with a much larger trap depth, typically in the range of 0.8–1.6 eV, to retain the recorded information at room temperature for a sufficiently long duration. On the basis of HRBE and VRBE schemes, led by the strategy of trap depth engineering, Dy3+, Ho3+, and Er3+ with a 0.84–1.16 eV energy gap between their 4f energy levels and the CB bottom are introduced into SrSi2O2N2:Eu2+ as the trap center (Fig. 19a). After irradiated by a 254 nm lamp, SrSi2O2N2:Eu2+,Ho3+ shows the highest intensity and the longest duration (360 min) at room temperature, while SrSi2O2N2:Eu2+,Dy3+ shows the weakest intensity and shortest duration as shown in Fig. 19b and c. The capability of these materials for information storage application is demonstrated in Fig. 19d. After removing the UV light, the samples were kept at room temperature for 60 min, and finally heated to 100 °C. The Er3+ co-doped sample gives the most intense emission in the first several minutes, whereas the luminous pattern from the Dy co-doped samples is almost unrecognizable in the first 60 min. However, when the sample is heated to 100 °C, the emission is greatly enhanced in all samples, and the emission intensity increases in the sequence of Er, Ho, and Dy. This is exactly opposite to the intensity that is detected at RT, which can be explained by the increased trap depth in the sequence of Er, Ho, and Dy from 0.8 to 1.2 eV derived from thermal luminescence spectra shown in Fig. 19e–g and Table 4. The captured electrons in the shallow traps can be released by thermal assistance at room temperature, giving rise to the most intense initial PersL intensity of SrSi2O2N2:Eu2+,Er3+, while the sufficiently deep traps introduced by Dy3+ have the ability to effectively restrain the detrapping at room temperature and thus ensure intense emissions as optical signals for the information readout. This work not only validates the capability of deep-trap PersL materials like SrSi2O2N2:Eu2+,Dy3+ in the information storage applications, but also provides inspirations to trigger more explorations toward new materials for applications in anticounterfeiting and advanced displays.
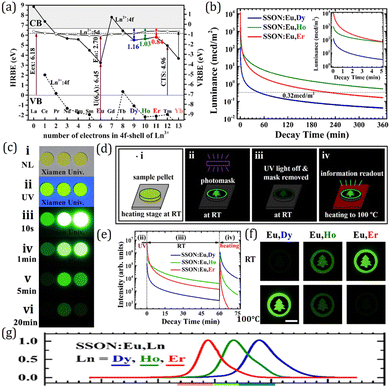 |
| Fig. 19 (a) HRBE (left ordinate) and VRBE (right ordinate) schemes of SrSi2O2N2 (SSON). The 4f ground states (Ln2+:4f) and the lowest 5d excited states (Ln2+:5d) of Ln2+ are labeled by black dots and hollow circles and connected by solid curves. The 4f ground states of Ln3+ (Ln3+:4f) are connected by a dashed curve. (b) PersL decay curves in SSON:Eu,Ln (Ln = Dy, Ho, and Er). The samples are irradiated by a Hg lamp dominantly at 254 nm for 20 s before recording. The insets show enlarged parts in the beginning stage. (c) Photographic images of the pellet samples under natural light (i); under UV excitation (ii); and 10 s (iii), 1 min (iv), 5 min (v), and 20 min (vi) after removal of the excitation source at RT. The samples for 1, 2, 3 are SSON:Eu,Ln, Ln = Dy, Ho, and Er, respectively. The UV excitation source is 254 nm. (d) Schematic illustration of the application in information storage using deep-trap persistent luminescent materials. (e) Decay curves of the luminous patterns on SSON:Eu,Ln. The samples were heated from RT to 100 °C at 60 min. (f) Photographic images of the pellets at RT and 100 °C. Exposure times of the digital camera are 0.5 s. The photos marked by RT and 100 °C were taken at approximately 1 and 61 min after removal of the UV light. The scale bar is 5 mm. (g) TL glow curves of SSON:Eu,Ln. Panels a-g: reproduced with permission from ref. 241, copyright 2017 American Chemical Society. | |
Eu2+-activated sialon
Sialons are solid solutions of the M–Si–Al–O–N system derived from silicon nitride Si3N4,323 which has two phases denoted as α and β. They could be described as different stackings of Si–N layers in ABCD… and ABAB… sequences, respectively. α-Si3N4 has two large closed interstitial sites per unit cell and β-Si3N4 has long continuous channels.324 As shown in Fig. 20, α-Si3N4 has two Si sites, and β-Si3N4 has only one Si site. The composition of α-sialon could be expressed by MxSi12−(m+n)Al(m+n)OnN16−n, where m (Al–N) pairs and n (Al–O) pairs replace m + n (Si–N) pairs in each unit cell. The molar fraction x is obtained by m divided by the valence of M cation, which includes Li, Mg, Ca, Y or heavier lanthanide ions. Since the two interstitial sites exist in every unit cell, the value of x representing the M content has a limit of 2, and m needs to be adjusted to meet the electroneutrality requirement.
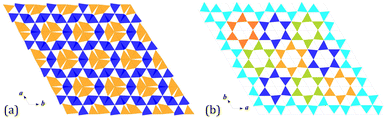 |
| Fig. 20 Crystal structures of Si3N4. (a) α-Si3N4 has two Si sites as represented by the blue and orange SiN4 tetrahedra. (b) β-Si3N4 only has one type of Si site. The different colors of the SiN4 tetrahedra reveal the regularly placed 6 unit ring channel. | |
In 2002, van Krevel et al. and Xie et al. reported the luminescence properties of Eu2+-doped Ca-α-sialon.277,325,326 It can be excited by UV to blue light (300–470 nm) and give yellow emission (570–600 nm). The emission could be tuned by composition modulation, such as changing the m and n values, substituting Ca for other metal ions such as Li, Mg and Y, adjusting the O/N content and varying the Eu concentration.327,328 Yang et al. synthesized Eu2+-doped pure-nitride α-sialons with nominal formula (Ca0.995Eu0.005)m/2Si12−mAlmN16 using the alloy-nitridation method.329 Samples with 2.4 ≤ m ≤ 4.0 have the strongest emission, and exhibit a red-shift with increasing m values as well as Eu concentrations.
The PersL of Eu2+-doped Ca-α-sialon has been reported in CaSi10−nAl2+nOnN16−n:xEu2+(m = 2,n = 0–1, x = 0.1–8%).330 It has a maximum persistent duration of about 60 min for the CaSi10A12N16:0.5% Eu2+ sample. DFT calculations prove that the charge carriers are electrons which are excited from the 4f ground state of Eu to the conduction band directly in the charging process, and the trap levels are introduced by oxygen/nitrogen defects. It has a potential application in anti-counterfeit and information storage.
As a currently commercial narrow-band green phosphor, β-sialon:Eu2+ is widely applied to fabricate wide color gamut backlights owing to its outstanding luminescence properties with a narrow emission band (FWHM ∼ 55 nm), superior thermal luminescence stability (∼10% emission loss at 150 °C), and high quantum efficiency.242,331 The solid solution β-sialon with formula Si6−zAlzOzN8−z, 0 < z ≤ 4.2, structurally derived from β-Si3N4via simultaneous equivalent substitution of Al–O for Si–N, was discovered by Oyama and Kamigaito in 1971.332 It crystallizes in a hexagonal structure with the space group of P63/m. As shown in Fig. 21a, the structure is built up by corner sharing (Si/Al)(N/O)4. About 30 years later, Hirosaki et al. reported for the first time the green photoluminescence of Eu2+-doped β-sialon with z = 0.17, which exhibits a narrow-band green emission centering at 535 nm with an FWHM of 55 nm under near UV or blue light excitation.333 Then, Xie et al. demonstrated that its emission wavelength can be tuned from 528 to 550 nm with an increasing z value.334 The typical PLE and PL spectra for β-sialon:Eu2+ with the nominal composition of Eu0.015Si5.5Al0.485O0.515N7.485 are displayed in Fig. 21b as an example. In 2008, Li. et al. gave the formula EuxSi6−zAlz−xOz+xN8−z−x for Eu2+-doped β-sialon, and proposed that Eu2+ ions are located in the large channels along the c-axis, which are formed by six (Si/Al)(O/N)4 tetrahedra.335 Later, it was confirmed by Kimoto et al. using scanning transmission electron microscopy (STEM).49 What is more, many endeavours have been devoted to investigating the origin of the narrow band emission, and it is revealed that the interstitial site in the highly symmetric hexagonal channel accommodating Eu2+ plays a crucial role in the narrow band emission.331 Since the unusual occupation of Eu2+ in the host lattice and some Eu3+ ions existing in the channel of β-sialon instead of Eu2+ generally result in decreased luminescence efficiency, Li et al. promote the reduction of Eu3+ to Eu2+ in β-sialon by post-annealing in an N2–H2 reducing atmosphere and thereby improve the luminescence efficiency of β-sialon:Eu2+.336 Recently by virtue of density functional theory (DFT) calculations, Wang et al. revealed that Eu2+ is first coordinated by nine nitrogen atoms, forming a highly symmetric EuN9 polyhedron in β-sialon:Eu2+.337 They also attributed its excellent thermal luminescence stability to the large energy gap between the 5d levels and the conduction band (CB) of the host (∼0.6 eV) calculated by DFT calculations.
β-sialon:Eu2+ also exhibits PersL behaviour. After 254 nm irradiation, green PersL is observed for 400 s with the threshold value of 0.32 mcd m−2 (Fig. 21c).243 By virtue of density functional theory (DFT) calculations, it is revealed that the trap levels responsible for PersL are impurity levels induced by Si–O bonds located below the bottom of the conduction band (CB) on random substitution of Al–O for Si–N in β-Si3N4, as shown in Fig. 21d. Through comparing thermoluminescence excitation (TLE) and photoluminescence excitation (PLE) spectra in Fig. 21e, it is confirmed that, in the charging process for PersL, electrons are directly excited from the 4f7 ground state of Eu2+ to the CB, which can be explained by the large barrier for thermal ionization of β-sialon:Eu2+ and is well consistent with its excellent thermal luminescence stability. Based on the TLE spectrum, a novel strategy is proposed to construct HRBE and VRBE schemes, i.e., using the onset energy of the TLE spectrum as the energy difference between the 4f ground state and the bottom of CB to pinpoint the 4f energy level location of Eu2+ (Fig. 21f). Hopefully, through co-dopant introduction and ion substitution led by electronic structure engineering, the PersL properties of β-sialon:Eu2+ could be further improved toward practical applications in emergency signage and optical information storage.
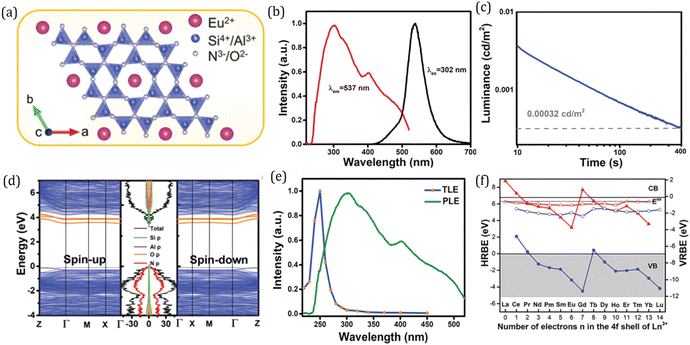 |
| Fig. 21 (a) Crystal structure of β-sialon:Eu2+. (b) PLE (λem = 537 nm) and PL (λex = 302 nm) spectra of Eu0.015Si5.5Al0.485O0.515N7.485 (ESAON). (c) PersL decay time of ESAON after 254 nm irradiation for 10 min at room temperature. (d) Computed electronic band dispersion, DOS, and PDOS of the perfect compound β-sialon (z = 0.5). The impurity levels of the Si–O bond are denoted by orange lines. (e) PLE and TLE spectra of ESAON. (f) The HRBE and VRBE schemes for ESAON. Red and blue ‘zigzag’ curves connect the energy for electrons in the 4f ground state of divalent and trivalent lanthanides, respectively. The other red and blue curves connect the energy for the lowest 5d states of divalent and trivalent lanthanides, respectively. Panels a-f: reproduced with permission from ref. 243, 2019 Royal Society of Chemistry. | |
Eu2+-activated M2SiO4 (M = Ca, Sr, Ba)
Although nitride and oxynitride phosphors, taking advantage of their excellent luminescent properties, low thermal luminescence quenching, and high chemical stability due to their high covalencies, are the most qualified candidates for LED applications, their synthesis with refractory Si3N4/AlN and unstable alkaline-earth nitrides as raw materials, generally requiring high temperature (>1500 °C) and high pressure (>0.1 Mpa), is rigorous, energy intensive and expensive. By contrast, the production of oxide phosphors is more convenient and energy-efficient as their raw materials are non-refractory and air-stable.338 Therefore, research studies on orthosilicate phosphors for LED applications have been the hotspot in luminescent materials science. Among them, M2SiO4 (M = Ca, Sr, Ba):Eu2+ are a classical class of phosphors widely used for LED applications like daily lighting and traffic lights. M2SiO4 (M = Ca, Sr, Ba) adopt slightly different structures, depending on the M cations, but all look similar to Ba2SiO4 shown in Fig. 22a. Ba compound only exists in an orthorhombic structure with a space group of Pmcn.339 Two types of cations exist in the crystal structure, and thus the crystal chemical formula could be written as MM′SiO4. The M and M′ sites differ in the coordination number and polyhedral volume. For Sr compound, at 85 °C the displacive phase transition from α′ to β occurs.340 β-Sr2SiO4 is monoclinic with space group P21/n. α′-Sr2SiO4 is isostructural with Ba2SiO4 and can be stabilized at room temperature by adding a small amount of Ba.340 The solid solution MM’SiO4 with M, M′ = Ba, Sr presents preferential site occupation, i.e., Sr prefers the site with a small volumetric size while Ba prefers the larger one.142 The stable Ca2SiO4 polymorph at room temperature is the γ type, which has an orthorhombic olivine structure with space group Pbnm. Besides, it also possesses a metastable β phase, which is an isotype with the β-Sr2SiO4 phase.341
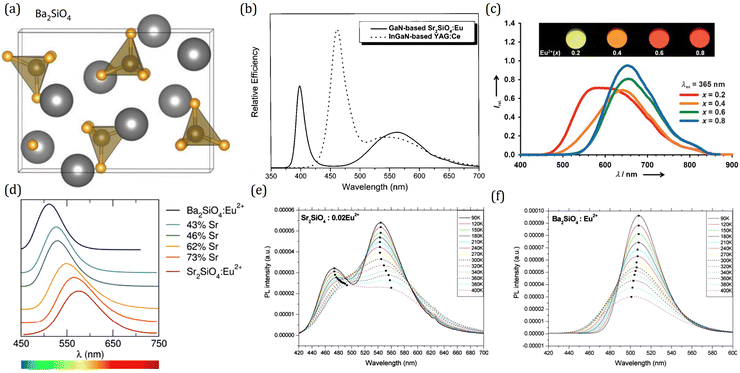 |
| Fig. 22 (a) Crystal structures of Ba2SiO4. Reproduced with permission from ref. 5, copyright 2013 Annual Reviews. (b) Relative emission spectra of a white-emitting InGaN-based YAG:Ce LED and GaN-based Sr2SiO4:Eu LED under a 20 mA drive current. Reproduced with permission from ref. 342, copyright 2003 American Institute of Physics. (c) Emission spectra (λex = 365 nm) of Ca2−xEuxSiO4 with the Eu2+ content (x) equal to 0.2, 0.4, 0.6, and 0.8. Inset: Photographs of these samples upon photo-excitation at 365 nm. Reproduced with permission from ref. 14, copyright 2014 Wiley. (d) Emission spectra collected at room temperature using the optimal excitation wavelength for each sample show broad emission across the green to yellow region of the visible spectrum. Reproduced with permission from ref. 51, copyright 2014 American Chemical Society. (e) Photoluminescence spectra excited at 370 nm of Sr2SiO4:Eu2+ phosphor at various temperatures. (f) Photoluminescence spectra excited at 370 nm of Ba2SiO4:Eu2+ phosphor at various temperatures. Panels e and f: reproduced with permission from ref. 343, copyright 2004 Elsevier Ltd. | |
The first report of phosphors within this system was provided as a brief description by Jenkins and McKeag in 1950,344 and a full description of their luminescence properties was made by Barry345 and Blasse346 in the 1960s. From an efficiency viewpoint, they found that phosphors based on solid solution between Ba2SiO4 and Sr2SiO4 with the orthorhombic phase have higher efficiencies versus that based upon monoclinic β-Ca2SiO4. Then it was in 1990s, since the blue LED chips were discovered successfully, the research on luminescence properties of M2SiO4 (M = Ca, Sr, Ba):Eu2+ came again under the spotlight. All of them can be efficiently excited with both blue and near-UV light and give out emissions within the visible region, making these materials ideal for LED applications. The yellow-emitting Sr2SiO4:Eu2+ phosphors fabricated with blue GaN chips (400 nm) exhibit high-quality white light with a better luminous efficiency than that of the InGaN chips (460 nm) coated with YAG:Ce phosphors as shown in Fig. 22b.342 The applications in traffic lights and automotive displays of green-emitting Ba2SiO4:Eu2+ phosphors combined with 395 nm emitting InGaN chips were also demonstrated by Zhang et al.252 What is intriguing is that the emission of Ca2SiO4:Eu2+ varies from green-yellow to deep red upon different Eu2+ concentrations (Fig. 22c), which was suggested to be resulting from the different Eu2+ substitutions on two types of Ca sites in αL′-Ca2−xEuxSiO4 (0.2 ≤ x ≤ 0.8) including 10-coordinated Ca1 sites and 8-coordianted Ca2 sites.14 Following this work, Sato et al. further studied the effect of Eu2+ concentrations on the photoluminescence properties of α′-Sr2SiO4 and α′-Ba2SiO4.347 In the case of Sr2−xEuxSiO4 the emission wavelength was shifted from 585 nm to 611 nm with increasing Eu2+ concentration (x) from 0.1 to o.8, and a similar trend occurs in Ba2−xEuxSiO4 in which the emission was shifted from 513–545 nm. Also the low energy edges of the excitation spectra for both samples were significantly shifted to a longer wavelength by 40–50 nm. The induction of such large redshifts in the emission and excitation spectra of both systems was attributed to the increasing Eu2+ substitution in Sr2 and Ba2 sites, the coordinated polyhedra of which are smaller and more distorted than that of Sr1 and Ba1 sites.
Besides, cation or anion substitution also plays an important role in the modification of the luminescence properties in the M2SiO4 (M = Ca, Sr, Ba):Eu2+ system. As an example, Denault et al. studied the luminescence properties of SrxBa2−xSiO4:Eu2+ and explored the structure–property correlations.51 With the increasing Sr content, the excitation spectra exhibit a slight red-shift while the emission spectra show a large red-shift of about 65 nm (Fig. 22d), which were originating from the decreased polyhedral volume and increased distortion as Sr content increases. Surprisingly, the intermediate composition with 46% Sr has the highest thermal luminescence stability, remaining stable up to 413 K, and maintaining an emission efficiency of 75%. Structural studies using high resolution X-ray diffraction and total scattering show that the intermediate compositions possess optimal bonding that creates a more rigid crystal structure compared to the end-member compositions. The increased structural rigidity of Sr0.9Ba1.1SiO4 is confirmed by its highest Debye temperature determined by the low temperature heat capacity. The high Debye temperature limits access to phonon modes which contribute to luminescence thermal quenching. This work provides an impetus for searching novel efficient phosphor hosts that possess highly connected structures and optimized bonding interactions. Furthermore, He et al. found that the composition dependence of quenching temperatures coincides with that of Sr/Ba atomic ordering degree in the host lattice.142 Therefore, the improved thermal luminescence quenching resistance of the intermediate compositions in (Ba1−xSrx)2SiO4:Eu2+ phosphors originates from the ordered cationic distribution, which could be represented by the degree of ordering η = OccSr2 − OccSr1 = OccBa1 − OccBa2. The volumetric difference of the two cationic sites in MM’SiO4 suggests that BaSrSiO4 could be thought as the end-member compound since the size-mismatch between Ba and Sr ions is annihilated. From this point of view, Ba2SiO4 and Sr2SiO4 have the intermediate compositions of which the size-mismatch will degrade structural rigidity and deteriorate the thermal luminescence quenching resistance. In addition, the temperature-dependent emission spectra of M2SiO4 (M = Ca, Sr, Ba):Eu2+ were investigated by Kim et al.343 It was demonstrated that two emission bands of Sr2SiO4:Eu2+ show normal redshift with increasing bandwidth and decreasing intensity as temperature increases (Fig. 22e), which can be explained by the interaction between the electron and thermally active phonon. Different from Sr2SiO4:Eu2+, the emission bands of M2SiO4 (M = Ca, Ba):Eu2+ show an anomalous blueshift (Fig. 22f), which can be described in terms of back tunneling from the excited states of low-energy emission band to that of high-energy emission band with the assistance of thermally active phonon.
The optimal bonding has also been reported in the T phase of the (Ba,Ca)2SiO4 system. Fukuda et al. found that the disordered crystal structure contains many split crystallographic sites.348 He et al. carefully evaluated the coordination environments of all the cation sites of the representative T-phase (Ba0.65Ca0.35)2SiO4, to understand the origin of the atom site splitting phenomenon, as shown in Fig. 23a and b.244 By analyzing the cation site coordination environments by split- and unsplit-atom-site models, it is found that the three cation sites in the split-atom-site structure are optimally bonded with the ligand O atoms. The coordination environments of the five cation sites together with the corresponding average bond lengths are shown in Fig. 23c. Due to the split of the O2 site, the unsplit M5 site has the average bond length and coordination number modified from 3.063–2.815 Å and 12–8, respectively. Meanwhile, the M1 and M2 sites are split for optimal bonding due to the co-occupation of Ba2+/Ca2+ ions, and two sets of coordination environments are formed for larger Ba2+ ions and smaller Ca2+ ions, leading to a more rigid structure and better thermal luminescence stability compared to end-member Ba2SiO4 and Ca2SiO4 crystals.
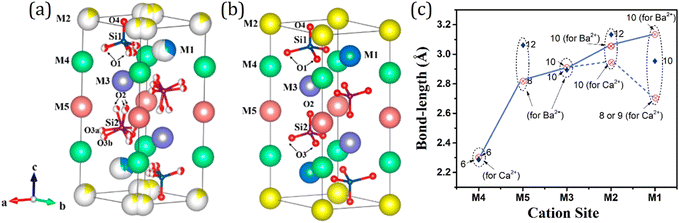 |
| Fig. 23 Crystal structures of the T-phase (Ba,Ca)2SiO4 for (a) the spilt-atom-site and (b) unsplit-atom-site models. (c) Average bond lengths and the coordination numbers of the different Ca2+/Ba2+ sites for the split-atom-site model (⊗) and unsplit-atom-site model (♦) for T-phase (Ba,Ca)SiO4, respectively. Panels (a–c): reproduced with permission from ref. 244, copyright 2018 Royal Society of Chemistry. | |
M2SiO4 (M = Ca, Sr, Ba):Eu2+ with Ln3+ co-dopant also exhibit PersL performance. In 2008, Lakshminarasimhan et al. first reported that Sr2SiO4:Eu2+,Dy3+ shows yellow emitting PersL for about 5 min after 313 nm irradiation as displayed in Fig. 24a.349 After that, Dutczak et al. further studied the effect of the excitation energy on the PersL properties of Sr2SiO4:Eu2+,Dy3+, and found that two times stronger PersL can be achieved after supra-bandgap vacuum ultra violet (VUV) excitation than after sub-bandgap near-UV or visible excitation, which indicates that the direct creation of free charge carriers under supra-bandgap excitation is followed by trapping and slow release of charge carriers resulting in a strong yellow PersL.267 Then in 2014, Zhang et al. doped Tm3+ into Sr2SiO4:Eu2+ to introduce deep traps for optical storage applications.269 As shown in Fig. 24b, Sr2SiO4:Eu2+,Tm3+ shows green photostimulated luminescence (PSL) upon infrared stimulation at 980 nm after being pre-irradiated by UV light. In 2018, the outstanding PersL performance of Ba2SiO4:Eu2+,Ho3+ was demonstrated by Wang et al.253 It shows supper-long green PersL which remains above 2.97 mcd m−2 even 24 h after the removal of UV irradiation as shown in Fig. 24c. What is more, green luminescence by 980 nm stimulation is still observable even 15 days after the UV irradiation (Fig. 24d). It is proposed that the outstanding PersL and PSL performance is mainly derived from the tailoring of trap distribution via Ho3+ co-doping (Fig. 24e). On the basis of HBRE and VRBE schemes of Ba2SiO4, Er3+ with a suitable energy gap between the CB bottom and the 4f ground state, 0.64 eV was selected as an efficient co-dopant, and 7 h PersL duration was achieved for Ba2SiO4:Eu2+,Er3+.270 All of these studies show the great potential of the M2SiO4 (M = Ca, Sr, Ba):Eu2+ family in PersL applications. With the advantage of bandgap engineering and trap depth engineering based on HRBE and VRBE schemes, further research studies toward improved PersL in solid solution or with multi-doping are expected to give a bright future of M2SiO4 (M = Ca, Sr, Ba):Eu2+ in the practical applications of emergency signage and optical information storage.
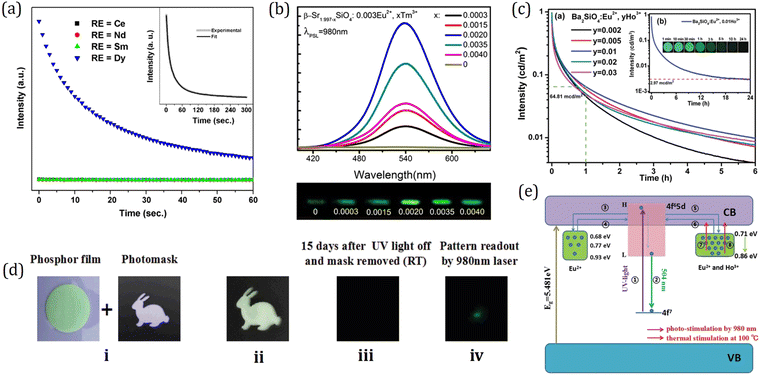 |
| Fig. 24 (a) Decay curves of Eu2+ emission in Sr1.98SiO4:0.01Eu2+,0.01RE3+ [RE = Ce, Nd, Sm and Dy]; λex = 313 nm. The inset shows the fitting of the decay curve of Sr1.98SiO4:0.01Eu2+,0.01Dy3+. Reproduced with permission from ref. 349, copyright 2007 Elsevier Ltd. (b) The PSL emission spectrum of β-Sr1.997−xSiO4:0.003Eu2+,xTm3+ (x = 0–0.004). The photos present the corresponding brightness green PSL of serial samples under 980 nm stimulation after preirradiation with UV excitation. Reproduced with permission from ref. 269, copyright 2014 American Ceramic Society. (c) PersL decay curves of Ba2SiO4:0.005Eu2+,yHo3+ (y = 0.002–0.03). (d) The write-in using UV (254 nm) irradiation and read-out (980 nm laser) of the rabbit pattern on the flexible phosphor film. (i) Phosphor film and photomask (rabbit pattern). (ii) Phosphor film is covered by photomask using UV 254 nm irradiation to write in the patterns. (iii) The phosphor film was kept at RT in the dark for 15 days, after removing the UV light and photomasks. (iv) The phosphor film was stimulated by a 980 nm laser to read out the stored patterns. (e) Schematic illustration of the PersL and PSL mechanisms of the Ba2SiO4:Eu2+,Ho3+ phosphor. Panels (c–e): reproduced with permission from ref. 253, copyright 2018 Royal Society of Chemistry. | |
Ce3+/Eu2+-activated M3SiO5 (M = Sr, Ba)
Within the MO–SiO2 (M = Ca, Sr, Ba) phase diagram, another compound with M3SiO5 stoichiometry, exhibiting efficient yellow-orange emission under near-UV to blue excitation when doped with either Eu2+ or Ce3+, has been successfully commercialized for applications in warm w-LEDs. The symmetry of these materials is different for each M cation, generally upgrading in the symmetry with the size of the alkaline earth cation. For the Ca, Sr, Ba variants, the crystal structures are monoclinic with a space group of Cm, tetragonal with a space group of P4/ncc, and tetragonal with a space group of I4/mcm, respectively.350,351 The structure of Sr3SiO5 is displayed in Fig. 25a as an example. It has two sites of strontium ions in the unit cell, i.e. 8f and 4c sites. The latter one has a larger polyhedral volume. Its crystal chemical formula could be thus written as (Sr)2[Sr]SiO5. Blasse et al. first reported these compounds as phosphors in 1968, doping them with Eu2+.346 These researchers showed that these phosphors can be excited efficiently by near-UV or blue light, and give out a broad emission peaking at approximately 500–600 nm (Fig. 25b), which can be systematically tuned toward the lower-energy part of the spectrum by varying the M cation from smaller to larger. In 2004, Park et al. demonstrated that InGaN (460 nm)-based Sr3SiO5:Eu2+ exhibits a better luminous efficiency than InGaN (460 nm)-based YAG:Ce, but a lower color rendering index (CRI, 64) due to the lack of red component.255 Then, in order to improve the quality of Sr3SiO5:Eu2+-based w-LEDs, the emission of Sr3SiO5:Eu2+ was shifted to a longer wavelength by adding the co-dopant Ba2+.352 Through integration of the InGaN (460 nm) with two phosphor blends (Sr2SiO4:Eu2+ yellow phosphors and a Ba2+ co-doped Sr3SiO5:Eu2+ yellow-orange phosphor) into a single package, CRI of the as-made w-LEDs was improved to over 85. It is worth noting that the red shift leading by the larger cation does not conform with the typical positive relationship between size of cation occupied by activator ions and emission energy. And this abnormal red shift may result from the different structures of Sr3SiO5 and Ba3SiO5, and the different occupancy modes of Eu2+ in two M2+ sites of M3SiO5 (M = Sr, Ba). The temperature-dependent PL intensity in Sr3−xEuxSiO5 (x = 0.05) is shown in Fig. 25c. Different with the normal case like in YAG:Ce phosphors that the emission intensity continuously drops as the temperature increases since the possibility of non-radiative process increases with thermal assistance, in Sr3−xEuxSiO5, the emission intensity slightly increases at 50 °C and then decreases with increasing temperature.256 This abnormal emission intensity increase under the elevated temperature originates from the traps in the host, which can capture the electrons and then release them at elevated temperatures. These traps also contribute to the PersL performance of Sr3SiO5:Eu2+, which will be discussed below. This phenomenon indicates that the traps with an appropriate trap depth not only contribute to the PersL, but also could give rise to improved thermal luminescence stability.
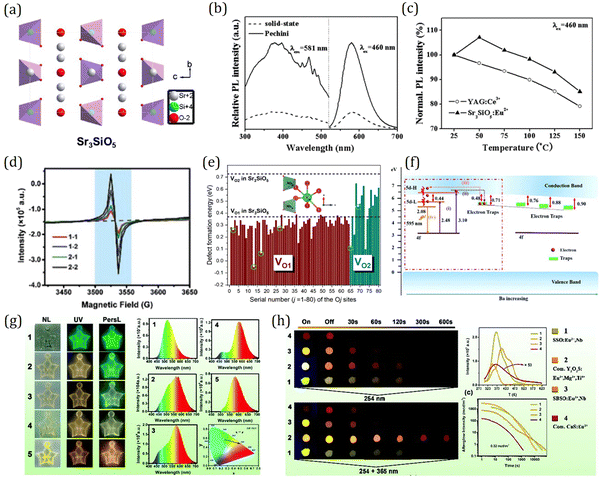 |
| Fig. 25 (a) Crystal structure of Sr3SiO5. Reproduced with permission from ref. 3, copyright 2016 Elsevier Ltd. (b) Excitation and emission spectra of Sr3−xEuxSiO5 (x = 0.05) phosphors annealed at 1600 °C. (c) Temperature dependence of the emission intensities of commercial YAG:Ce3+ phosphor and Sr3−xEuxSiO5 (x = 0.05) phosphor synthesized via the Pechini process. (Annealing temperature = 1600 °C, λex = 460 nm). Panels (b and c) reproduced with permission from ref. 256, copyright 2011 The electrochemical Society. (d) Room temperature EPR spectra of Sr3SiO5:Eu2+ (red and blue lines) and Sr3SiO5:Eu2+,Nb (green and black lines). Magnetic field from 3420 to 3650 Gauss before and after 100 s UV radiation. The sharp signal at about 3530 G corresponds to oxygen vacancies. (e) Calculated defect formation energies for oxygen vacancies in Sr3SiO5 with incorporation of a most probable 2NbSi–VSr configurations (as shown pictorially in the inset). (f) A schematic representation of the yellow-orange PersL mechanisms of (Sr1−xBax)3SiO5:Eu2+,Nb5+. (g) Photographic images of ‘‘Pisces’’ patterns for different commercialized SrAl2O4:Eu2+,Dy3+ persistent phosphor and Sr3SiO5:Eu2+,Nb phosphor ratios (denoted by numbers 1–5) under natural light (NL), 254 nm UV excitation, and 5 s after shutting down the UV light (PersL), persistent emission spectra of ‘‘Pisces’’ patterns, and CIE coordinates of ‘‘Pisces’’ patterns. (h) Warm-light PersL images of (Sr, Ba)3SiO5:Eu2+,Nb5+ obtained at different PersL decay times after 254 nm UV-lamp excitation for 5 min (upper image) and after 254 nm + 365 nm UV-lamp excitation for 5 min (lower image), and TL glow curves of Sr3SiO5:Eu2+,Nb5+, Y2O2S:Eu3+,Mg2+,Ti4+, (Sr0.88Ba0.12)3SiO5:Eu2+,Nb5+ and (Ca,Sr)S:Eu2+. Panels (d, e, and g): reproduced with permission from ref. 272, copyright 2020 Royal Society of Chemistry. Panels (f and h): reproduced with permission from ref. 274, copyright 2021 Royal Society of Chemistry. | |
Apart from Eu2+ activator, the green-yellow phosphors for LED applications can be created when the M3SiO5 host is doped with Ce3+. As an example, Sr3SiO5:Ce3+,Li+ phosphor exhibits a very broad emission peaking at ∼540 nm under near-UV or blue light excitation.260 Thereinto, Li+ was added for charge compensation since Sr2+ was replaced by aliovalent Ce3+. The concentration quenching happens at Ce3+ levels of ∼0.75%, which results from the energy migration over multiple activation ions and then impurities or defects in phosphor can be reached out. Besides single phosphor LEDs, Sr3SiO5:Ce3+,Li+ phosphors were also used in combination with red-emitting CdSe quantum dots to give white light with a high CRI. Unfortunately, Ce3+-activated M3SiO5 phosphors suffer from severe thermal luminescence quenching at T > 100 °C. At 170 °C, Sr3SiO5:Ce3+,Li+ loses about 50% of its room temperature intensity, which to a large extent limits its applications. Hopefully, by exerting bandgap engineering based on VRBE schemes, the thermal luminescence stability of M3SiO5:Ce3+ could be improved by manipulating the energy gap between the 5d energy level and the CB bottom by cation or anion substitution, giving them a bright prospect for LED applications.
The yellow-orange PersL and photostimulated luminescence (PSL) of Sr3SiO5:Eu2+ were first discovered by Sun et al. in 2009, and they found that the introduction of Dy3+ can enhance PSL and prolong the PersL duration to 6 h after UV irradiation.353 Subsequently, the trap depth distribution and retrapping process in Sr3SiO5:Eu2+,Dy3+ were further studied. By analyzing the result of time response in PSL, it is revealed that both shallow and deep traps can retrap electrons during the PersL and PSL processes. Then Dong et al. reported that yellow-orange PersL of Sr3SiO5:Eu2+,Dy3+ can be further improved by adding alkaline earth metal fluorides MF2 (M = Ca, Sr, Ba).354 In particular, the PersL of Sr2.95SiO5,0.05BaF2:Eu2+,Dy3+ can last for 12 h. In 2013, Li et al. systemically studied the effect of rare earth codopant on PersL of Sr3SiO5:Eu2+, and found that, after 365 nm irradiation, Sr3SiO5:Eu2+,Dy3+ has the longest PersL duration while Sr3SiO5:Eu2+,Nd3+ exhibits the highest initial PersL intensity.355 Recently, Wang et al. further investigated the mechanism of PersL enhancement by Nb-incorporation. By virtue of electron paramagnetic resonance (EPR) and density functional theory (DFT) (Fig. 25d and e), it is revealed that Nb-incorporation reduces the formation energy of oxygen vacancies and thereby increases the density of oxygen vacancies, which act as electron trapping centers with appropriate trap depths (Fig. 25f), giving rise to the enhanced PersL performance of Sr3SiO5:Eu2+,Nb3+. It is noteworthy that Sr3SiO5:Eu2+,Nb3+ can also be activated by sunlight (Fig. 25g), which indicates its potential in specific applications, such as emergency signage and night lighting. These researchers also reported that PersL of Sr3SiO5:Eu2+ can also be enhanced through Ge incorporation with a similar mechanism as Nd incorporation, by inducing increased oxygen vacancies.272 Furthermore, Wang et al. reported that the PersL color of Sr3SiO5:Eu2+,Nb3+ can be finely tuned by Ba substitution. It can be tuned from 580–600 nm as x varies from 0 to 0.75 in Sr3−xBaxSiO5:Eu2+,Nb3+ system, realizing warm-color PersL with x = 0.12. Surprisingly, the PersL duration is significantly prolonged to 22 h in Sr3−xBaxSiO5:Eu2+,Nb3+ (x = 0.15) (Fig. 25h).274 The high PersL brightness, superlong PersL duration, and warm color PersL emission make Sr3−xBaxSiO5:Eu2+,Nb3+ a potential candidate for applications in energy, environmental, and optical information storage fields.
Challenge and outlook
Although substantial progress has been achieved in LED and persistent phosphors, challenges remain with respect to fundamental questions within the science and technology of luminescent materials including establishing the coupling model of multiple structural effect, understanding the mechanism of quantum efficiency, and unveiling the mystery of trap centers. It is also tricky to be able to exert the progress in fundamental scientific understanding on realizing and optimizing the practical applications. Therefore, collaborative efforts from multidisciplinary researchers are required in order to understand the physical properties and enhance the performance in practical devices, so that Ce3+/Eu2+-activated phosphors progress to higher levels. For this to happen, we envision that the following pillars need to be strengthened in the future:
(1) Developing blue light excitable cyan and deep red-near infrared (NIR) emitting phosphors
To fulfill the requirements of practical applications in security monitoring, food safety, and solar-like LED health lighting, developing blue light excitable cyan and deep red-near infrared (NIR) emitting phosphors is currently under the spotlight. In order to accelerate the phosphor development process, significant progress will be required in understanding the coupling mechanism of multiple structural effects. Based on luminescence theory, by virtue of first principles calculations, establishing the multiple effects of crystal field splitting, centroid shift and Stokes shift is going to be helpful to effectively tune and even predict the luminescence properties, paving way to the aimed phosphors and practical applications.
(2) Improving thermal luminescence stability and quantum efficiency
Non-radiative process suppresses the luminescence efficiency of both LED phosphor and persistent phosphor. Host with rigid and ordered framework can efficiently restrain the possibility of luminescence quenching due to lattice-phonon coupling, contributing to higher luminescence efficiency, better thermal luminescence stability, and narrow band emission. Exploration of rigid structures will open the door for development of phosphors with advanced performance.
(3) Exploring sunlight excitable high performance persistent phosphors
Although several persistent phosphors have been successfully commercialized for practical applications, the number of persistent phosphors with sufficient duration and intensity is still limited, especially the sunlight excitable ones. To make the most use of solar energy and realize the ultimate “dream” of night lighting, exploring sunlight excitable high performance persistent phosphors is an emergent task. Since PersL properties including duration and intensity are mainly determined by the characteristic of the trap center, such as trap depth and density, exploration and functionalization of dopants and structural defects as efficient trap center and investigation on functional mechanism of multiple trap centers will be helpful to accelerate the development of sunlight excitable high performance persistent phosphors.
(4) Developing high performance red-emitting persistent phosphors
Relative to blue and green emitting persistent phosphors, efficient red-emitting persistent phosphors are still deficient. Based on electronic structure engineering, designing high performance red-emitting persistent phosphors is of vital importance for developing white PersL and broadening the application fields of persistent phosphors.
Summary
Over the past few decades, great progress has been achieved in the discovery and optimization of LED and persistent phosphors. Nowadays, Ce3+/Eu2+-activated phosphors have been developed as an indispensable component for various applications including daily lighting, LED backlights, emergency signage, and information storage. In this review, we summarize the state of art in Ce3+/Eu2+-activated LEDs and persistent phosphors from the perspectives of both theory and application, highlighting the design principles for new high-performance phosphors. We first introduced Dorenbos theory on f–d transitions in detail, and elaborated some of the latest developments on the effect of the local structure on f–d transitions, to give an overall vision on the composition–structure–property correlations in Ce3+/Eu2+-activated LED phosphors. We also demonstrated the electronic structure diagram as a powerful design tool towards promising properties. Then the luminescence mechanism for persistent phosphor is illustrated, addressing on the critical role of the band gap structure and trap characteristics on PersL properties. As templates, the development and optimization routines of some typical phosphors were expounded, with emphasis on design principles and how the chemical characteristic affects the luminescence properties, aiming at providing inspirations for tailoring and optimizing the properties of Ce3+/Eu2+-activated phosphors toward specific applications. Taking advantage of electronic structure engineering, employing structure manipulation of the host via doping control and cationic/anionic substitution, the luminescence properties of LED phosphors can be effectively tuned towards desired emission color, improved luminescence efficiency, and better thermal luminescence stability. As for persistent phosphors, led by band gap engineering and trap depth engineering, the intensity and PersL duration can be significantly enhanced by controlling defect concentration, intentionally introducing aliovalent or isovalent co-dopants as trap centers. Despite the prosperity of this well-established research filed, there are clear challenges ahead. We thus provide a look toward promising research directions, and offer suggestions for future development.
We anticipate that the luminescence theory elaborated and the strategy exemplified in this review will provide a huge boost in the advanced performance of Ce3+/Eu2+-activated phosphors for practical applications and, also, provide inspirations to drive the development of other functional materials.
Conflicts of interest
There are no conflicts to declare.
Acknowledgements
This work was supported by the National Key Research and Development Program of China (No. 2022YFB3503800) and the National Natural Science Foundation of China (No. 51832005).
References
- P. Pust, V. Weiler, C. Hecht, A. Tücks, A. S. Wochnik, A.-K. Henß, D. Wiechert, C. Scheu, P. J. Schmidt and W. Schnick, Narrow-Band Red-Emitting Sr[LiAl3N4]:Eu2+ as a next-Generation LED-Phosphor Material, Nat. Mater., 2014, 13(9), 891–896 CrossRef CAS PubMed.
- P. Pust, P. J. Schmidt and W. Schnick, A Revolution in Lighting, Nat. Mater., 2015, 14(5), 454–458 CrossRef CAS PubMed.
- Z. G. Xia and Q. L. Liu, Progress in Discovery and Structural Design of Color Conversion Phosphors for LEDs, Prog. Mater. Sci., 2016, 84, 59–117 CrossRef CAS.
- S. Ye, F. Xiao, Y. X. Pan, Y. Y. Ma and Q. Y. Zhang, Phosphors in Phosphor-Converted White Light-Emitting Diodes: Recent Advances in Materials, Techniques and Properties, Mater. Sci. Eng., R, 2010, 71(1), 1–34 CrossRef.
- N. C. George, K. A. Denault and R. Seshadri, Phosphors for Solid-State White Lighting, Annu. Rev. Mater. Res., 2013, 43(1), 481–501 CrossRef CAS.
- C. C. Lin and R.-S. Liu, Advances in Phosphors for Light-Emitting Diodes, J. Phys. Chem. Lett., 2011, 2(11), 1268–1277 CrossRef CAS PubMed.
- K. Bando, A. Donai and M. Yamada, Practical-Uses and Applications of Highbright Three-Primary Color LED Lamps, J. Inst. Image Electron. Eng. Jap., 1996, 25, 290–297 Search PubMed.
- S. Nakamura, T. Mukai and M. Senoh, Candela-class High-brightness InGaN/AlGaN Double-heterostructure Blue-light-emitting Diodes, Appl. Phys. Lett., 1994, 64(13), 1687–1689 CrossRef CAS.
- S. Nakamura, Present Performance of InGaN-Based Blue/Green/Yellow LEDs, Proceedings of SPIE - The International Society for Optical Engineering, 1997, 3002, 26–35 CAS.
- S. Pimputkar, J. S. Speck, S. P. DenBaars and S. Nakamura, Prospects for LED Lighting, Nat. Photonics, 2009, 3(4), 180–182 CrossRef CAS.
- M. Zhao, Q. Y. Zhang and Z. G. Xia, Narrow-Band Emitters in LED Backlights for Liquid-Crystal Displays, Mater. Today, 2020, 40, 246–265 CrossRef CAS.
-
R. J. Xie, Y. Q. Li and N. Hirosaki, Nitride Phosphors and Solid-State Lighting, Crc Press, 2019 Search PubMed.
- Y. H. Kim, P. Arunkumar, B. Y. Kim, S. Unithrattil, E. Kim, S.-H. Moon, J. Y. Hyun, K. H. Kim, D. Lee, J.-S. Lee and W. B. Im, A Zero-Thermal-Quenching Phosphor, Nat. Mater., 2017, 16(5), 543–550 CrossRef CAS.
- Y. Sato, H. Kato, M. Kobayashi, T. Masaki, D.-H. Yoon and M. Kakihana, Tailoring of Deep-Red Luminescence in Ca2SiO4:Eu2+, Angew. Chem., Int. Ed., 2014, 53(30), 7756–7759, DOI:10.1002/ange.201402520.
- P. L. Li, Z. P. Yang, Z. J. Wang, Q. L. Guo and X. Li, Preparation and Luminescence Characteristics of Sr3SiO5:Eu2+ Phosphor for White LED, Sci. Bull., 2008, 53(7), 974–977 CrossRef CAS.
- S. Fu, J. Tan, X. Bai, S. J. Yang, L. You and Z. K. Du, Effect of Al/Ga Substitution on the Structural and Luminescence Properties of Y3(Al1−xGax)5O12:Ce3+ Phosphors, Opt. Mater., 2018, 75, 619–625 CrossRef CAS.
- M. Kottaisamy, P. Thiyagarajan, J. Mishra and M. S. Ramachandra Rao, Color Tuning of Y3(Al1−xGax)5O12:Ce Phosphor and Their Blend for White LEDs, Mater. Res. Bull., 2008, 43(7), 1657–1663 CrossRef CAS.
- K. Uheda, N. Hirosaki, Y. Yamamoto, A. Naito, T. Nakajima and H. Yamamoto, Luminescence
Properties of a Red Phosphor, CaAlSiN3:Eu2+, for White Light-Emitting Diodes, Electrochem. Solid-State Lett., 2006, 9(4), H22 CrossRef CAS.
- Y. Q. Li, J. E. J. van Steen, J. W. H. van Krevel, G. Botty, A. C. A. Delsing, F. J. DiSalvo, G. de With and H. T. Hintzen, Luminescence Properties of Red-Emitting M2Si5N8:Eu2+ (M=Ca, Sr, Ba) LED Conversion Phosphors, J. Alloys Compd., 2006, 417(1), 273–279 CrossRef CAS.
- P. Dorenbos, The 5d Level Positions of the Trivalent Lanthanides in Inorganic Compounds, J. Lumin., 2000, 91(3–4), 155–176, DOI:10.1016/S0022-2313(00)00229-5.
- P. Dorenbos, f→d Transition Energies of Divalent Lanthanides in Inorganic Compounds, J. Phys.: Condens. Matter, 2003, 15(3), 575–594, DOI:10.1088/0953-8984/15/3/322.
- X. Qin, X. W. Liu, W. Huang, M. Bettinelli and X. G. Liu, Lanthanide-Activated Phosphors Based on 4f–5d Optical Transitions: Theoretical and Experimental Aspects, Chem. Rev., 2017, 117(5), 4488–4527, DOI:10.1021/acs.chemrev.6b00691.
- P. Dorenbos, Mechanism of Persistent Luminescence in Eu2+ and Dy3+ Codoped Aluminate and Silicate Compounds, J. Electrochem. Soc., 2005, 152(7), H107, DOI:10.1149/1.1926652.
- J. Xu and S. Tanabe, Persistent Luminescence Instead of Phosphorescence: History, Mechanism, and Perspective, J. Lumin., 2019, 205, 581–620, DOI:10.1016/j.jlumin.2018.09.047.
- T. Maldiney, A. Bessière, J. Seguin, E. Teston, S. K. Sharma, B. Viana, A. J. J. Bos, P. Dorenbos, M. Bessodes, D. Gourier, D. Scherman and C. Richard, The in Vivo Activation of Persistent Nanophosphors for Optical Imaging of Vascularization, Tumours and Grafted Cells, Nat. Mater., 2014, 13(4), 418–426, DOI:10.1038/nmat3908.
- Z. J. Li, Y. W. Zhang, X. Wu, L. Huang, D. S. Li, W. Fan and G. Han, Direct Aqueous-Phase Synthesis of Sub-10 nm “Luminous Pearls” with Enhanced in vivo Renewable Near-Infrared Persistent Luminescence, J. Am. Chem. Soc., 2015, 137(16), 5304–5307, DOI:10.1021/jacs.5b00872.
- Y. X. Zhuang, L. Wang, Y. Lv, T.-L. Zhou and R.-J. Xie, Optical Data Storage and Multicolor Emission Readout on Flexible Films Using Deep-Trap Persistent Luminescence Materials, Adv. Funct. Mater., 2018, 28(8), 1705769, DOI:10.1002/adfm.201705769.
- J. Wang, Q. Su and S. B. Wang, A Novel Red Long Lasting Phosphorescent (LLP) Material β-Zn3(PO4)2:Mn2+, Sm3+, Mater. Res. Bull., 2005, 40(4), 590–598, DOI:10.1016/j.materresbull.2005.01.011.
-
E. N. Harvey, A History of Luminescence from the Earliest Times until 1900, American Philosophical Society, Philadelphia, PA, USA, 1957 Search PubMed.
- T. Matsuzawa, Y. Aoki, N. Takeuchi and Y. Murayama, A New Long Phosphorescent Phosphor with High Brightness, SrAl2O4:Eu2+, Dy3+, J. Electrochem. Soc., 1996, 143(8), 2670–2673, DOI:10.1149/1.1837067.
- J. Ueda, S. Miyano and S. Tanabe, Formation of Deep Electron Traps by Yb3+ Codoping Leads to Super-Long Persistent Luminescence in Ce3+ -Doped Yttrium Aluminum Gallium Garnet Phosphors, ACS Appl. Mater. Interfaces, 2018, 10(24), 20652–20660, DOI:10.1021/acsami.8b02758.
- B. Lei, K. Machida, T. Horikawa, H. Hanzawa, N. Kijima, Y. Shimomura and H. Yamamoto, Reddish-Orange Long-Lasting Phosphorescence of Ca2Si5N8:Eu2+,Tm3+ Phosphor, J. Electrochem. Soc., 2010, 157(6), J196, DOI:10.1149/1.3364953.
- Y. Miyamoto, H. Kato, Y. Honna, H. Yamamoto and K. Ohmi, An Orange-Emitting, Long-Persistent Phosphor, Ca2Si5N8:Eu2+,Tm3+, J. Electrochem. Soc., 2009, 156(9), J235, DOI:10.1149/1.3153114.
- P. Dorenbos, Crystal Field Splitting of Lanthanide
4fn−15d-Levels in Inorganic Compounds, J. Alloys Compd., 2002, 341, 156–159 CrossRef CAS.
- P. Dorenbos, Relating the Energy of the [Xe]5d1 Configuration of Ce3+ in Inorganic Compounds with Anion Polarizability and Cation Electronegativity, Phys. Rev. B: Condens. Matter Mater. Phys., 2002, 65(23), 235110, DOI:10.1103/PhysRevB.65.235110.
- Z. Yang, G. Liu, Y. Zhao, Y. Zhou, J. Qiao, M. S. Molokeev, H. C. Swart and Z. Xia, Competitive Site Occupation toward Improved Quantum Efficiency of SrLaScO4:Eu Red Phosphors for Warm White LEDs, Adv. Opt. Mater., 2022, 10(6), 2102373, DOI:10.1002/adom.202102373.
- S. Lai, M. Zhao, Y. Zhao, M. S. Molokeev and Z. Xia, Eu2+ Doping Concentration-Induced Site-Selective Occupation and Photoluminescence Tuning in KSrScSi2O7:Eu2+ Phosphor, ACS Mater. Au, 2022, 2(3), 374–380, DOI:10.1021/acsmaterialsau.1c00081.
- T. Hu, Y. Gao, M. S. Molokeev, Z. Xia and Q. Zhang, Eu2+ Stabilized at Octahedrally Coordinated Ln3+ Site Enabling Red Emission in Sr3LnAl2O7.5 (Ln = Y or Lu) Phosphors, Adv. Opt. Mater., 2021, 9(9), 2100077, DOI:10.1002/adom.202100077.
- Z. Yang, Y. Zhao, Y. Zhou, J. Qiao, Y. Chuang, M. S. Molokeev and Z. Xia, Giant Red-Shifted Emission in (Sr,Ba)Y2O4:Eu2+ Phosphor Toward Broadband Near-Infrared Luminescence, Adv. Funct. Mater., 2022, 32(1), 2103927, DOI:10.1002/adfm.202103927.
- T. Hu, Z. Xia and Q. Zhang, INVITED)Broadband Emission of Lu2SrAl4SiO12:Eu2+ Phosphor for Full-Spectrum Lighting, Opt. Mater.: X, 2022, 13, 100138, DOI:10.1016/j.omx.2022.100138.
- J. Qiao, Y. Zhou, M. S. Molokeev, Q. Zhang and Z. Xia, Narrow Bandwidth Luminescence in Sr2Li(Al,Ga)O4:Eu2+ by Selective Site Occupancy Engineering for High Definition Displays, Laser Photonics Rev., 2021, 15(11), 2100392, DOI:10.1002/lpor.202100392.
- J. Qiao, S. Zhang, X. Zhou, W. Chen, R. Gautier and Z. Xia, Near-Infrared Light-Emitting Diodes Utilizing a Europium-Activated Calcium Oxide Phosphor with External Quantum Efficiency of up to 54.7%, Adv. Mater., 2022, 34(26), 2201887, DOI:10.1002/adma.202201887.
- Z. Yang, Y. Zhou, J. Qiao, M. S. Molokeev and Z. Xia, Rapid Synthesis of Red-Emitting Sr2Sc0.5Ga1.5O5:Eu2+ Phosphors and the Tunable Photoluminescence Via Sr/Ba Substitution, Adv. Opt. Mater., 2021, 9(16), 2100131, DOI:10.1002/adom.202100131.
- J. Qiao, L. Ning, M. S. Molokeev, Y. Chuang, Q. Zhang, K. R. Poeppelmeier and Z. Xia, Site-Selective Occupancy of Eu2+ Toward Blue-Light-Excited Red Emission in a Rb3YSi2O7:Eu Phosphor, Angew. Chem., 2019, ange.201905787, DOI:10.1002/ange.201905787.
- M. Zhao, Q. Zhang and Z. Xia, Structural Engineering of Eu2+-Doped Silicates Phosphors for LED Applications, Acc. Mater. Res., 2020, 1(2), 137–145, DOI:10.1021/accountsmr.0c00014.
- M. Zhao, Z. Yang, L. Ning and Z. Xia, Tailoring of White Luminescence in a NaLi3SiO4:Eu2+ Phosphor Containing Broad-Band Defect-Induced Charge-Transfer Emission, Adv. Mater., 2021, 33(29), 2101428, DOI:10.1002/adma.202101428.
- P. Dorenbos, Systematic Behaviour in Trivalent Lanthanide Charge Transfer Energies, J. Phys.: Condens. Matter, 2003, 15(49), 8417–8434, DOI:10.1088/0953-8984/15/49/018.
- P. Dorenbos, Anomalous Luminescence of Eu2+ and Yb2+ in Inorganic Compounds, J. Phys.: Condens. Matter, 2003, 15(17), 2645–2665, DOI:10.1088/0953-8984/15/17/318.
- K. Kimoto, R.-J. Xie, Y. Matsui, K. Ishizuka and N. Hirosaki, Direct Observation of Single Dopant Atom in Light-Emitting Phosphor of β-SiAlON:Eu2+, Appl. Phys. Lett., 2009, 94(4), 041908, DOI:10.1063/1.3076110.
- S.-S. Wang, W.-T. Chen, Y. Li, J. Wang, H.-S. Sheu and R.-S. Liu, Neighboring-Cation Substitution Tuning of Photoluminescence by Remote-Controlled Activator in Phosphor Lattice, J. Am. Chem. Soc., 2013, 135(34), 12504–12507, DOI:10.1021/ja404510v.
- K. A. Denault, J. Brgoch, M. W. Gaultois, A. Mikhailovsky, R. Petry, H. Winkler, S. P. DenBaars and R. Seshadri, Consequences of Optimal Bond Valence on Structural Rigidity and Improved Luminescence Properties in SrxBa2–xSiO4:Eu2+ Orthosilicate Phosphors, Chem. Mater., 2014, 26(7), 2275–2282, DOI:10.1021/cm500116u.
- H. T. Hintzen, C. J. M. Denisscn and H. M. van Noort,
151Eu Mössbauer Spectroscopic Study of the Phosphor SrAl12O19:Eu with the Magnetoplumbite Structure, Mater. Res. Bull., 1989, 24(2), 247–259, DOI:10.1016/0025-5408(89)90132-3.
-
G. Blasse and B. C. Grabmaier, Luminescent Materials, Springer Berlin Heidelberg, Berlin, Heidelberg, 1994 DOI:10.1007/978-3-642-79017-1_1.
- J. A. DeLuca, An Introduction to Luminescence in Inorganic Solids, J. Chem. Educ., 1980, 57(8), 541, DOI:10.1021/ed057p541.
- J. J. Joos, P. F. Smet, L. Seijo and Z. Barandiarán, Insights into the Complexity of the Excited States of Eu-Doped Luminescent Materials, Inorg. Chem. Front., 2020, 18 Search PubMed.
- P. Dorenbos, Energy of the First 4f7→4f65d Transition of Eu2+ in Inorganic Compounds, J. Lumin., 2003, 104(4), 239–260, DOI:10.1016/S0022-2313(03)00078-4.
- P. Dorenbos, 5d-Level Energies of Ce3+ and the Crystalline Environment. I. Fluoride Compounds, Phys. Rev. B: Condens. Matter Mater. Phys., 2000, 62(23), 15640–15649, DOI:10.1103/PhysRevB.62.15640.
- M. de Jong, L. Seijo, A. Meijerink and F. T. Rabouw, Resolving the Ambiguity in the Relation between Stokes Shift and Huang–Rhys Parameter, Phys. Chem. Chem. Phys., 2015, 17(26), 16959–16969, 10.1039/C5CP02093J.
- P. A. Tanner, Some Misconceptions Concerning the Electronic Spectra of Tri-Positive Europium and Cerium, Chem. Soc. Rev., 2013, 42(12), 5090, 10.1039/c3cs60033e.
- D. P. Cui, Z. Song, Z. G. Xia and Q. L. Liu, Luminescence Tuning, Thermal Quenching, and Electronic Structure of Narrow-Band Red-Emitting Nitride Phosphors, Inorg. Chem., 2017, 56(19), 11837–11844, DOI:10.1021/acs.inorgchem.7b01816.
- B. F. Aull and H. P. Jenssen, Impact of Ion-Host Interactions on the 5d-to-4f Spectra of Lanthanide Rare-Earth-Metal Ions. I. A Phenomenological Crystal-Field Model, Phys. Rev. B: Condens. Matter Mater. Phys., 1986, 34(10), 6640–6646, DOI:10.1103/PhysRevB.34.6640.
- C. A. Morrison, Host Dependence of the Rare-earth Ion Energy Separation 4fN–4fN−1nl, J. Chem. Phys., 1980, 72(2), 1001–1002, DOI:10.1063/1.439265.
- J. D. Axe and G. Burns, Influence of Covalency upon Rare-Earth Ligand Field Splittings, Phys. Rev., 1966, 152(1), 331–340, DOI:10.1103/PhysRev.152.331.
- P. Dorenbos, A Review on How Lanthanide Impurity Levels Change with Chemistry and Structure of Inorganic Compounds, ECS J. Solid State Sci. Technol., 2013, 2(2), R3001–R3011, DOI:10.1149/2.001302jss.
- T. Wang, Z. G. Xia, Q. C. Xiang, S. Q. Qin and Q. L. Liu, Relationship of 5d-Level Energies of Ce3+ with the Structure and Composition of Nitride Hosts, J. Lumin., 2015, 166, 106–110, DOI:10.1016/j.jlumin.2015.05.017.
- S. X. Wang, Z. Song, Y. W. Kong, Z. G. Xia and Q. L. Liu, 5d-Level Centroid Shift and Coordination Number of Ce3+ in Nitride Compounds, J. Lumin., 2018, 200, 35–42, DOI:10.1016/j.jlumin.2018.03.079.
- P. Dorenbos, Ce3+ 5d-Centroid Shift and Vacuum Referred 4f-Electron Binding Energies of All Lanthanide Impurities in 150 Different Compounds, J. Lumin., 2013, 135, 93–104, DOI:10.1016/j.jlumin.2012.09.034.
- R. G. Pearson, Bond Energies, Force Constants and Electronegativities, J. Mol. Struct., 1993, 300, 519–525, DOI:10.1016/0022-2860(93)87044-A.
- P. Dorenbos, 5d-Level Energies of Ce3+ and the Crystalline Environment. III. Oxides Containing Ionic Complexes, Phys. Rev. B: Condens. Matter Mater. Phys., 2001, 64(12), 125117, DOI:10.1103/PhysRevB.64.125117.
- R.-J. Xie and N. Hirosaki, Silicon-Based Oxynitride and Nitride Phosphors for White LEDs—A Review, Sci. Technol. Adv. Mater., 2007, 8(7–8), 588–600, DOI:10.1016/j.stam.2007.08.005.
- R. Mueller-Mach, G. Mueller, M. R. Krames, H. A. Höppe, F. Stadler, W. Schnick, T. Juestel and P. Schmidt, Highly Efficient All-Nitride Phosphor-Converted White Light Emitting Diode, phys. stat. sol. (a), 2005, 202(9), 1727–1732, DOI:10.1002/pssa.200520045.
- P. Strobel, S. Schmiechen, M. Siegert, A. Tücks, P. J. Schmidt and W. Schnick, Narrow-Band Green Emitting Nitridolithoalumosilicate Ba[Li2(Al2Si2)N6]:Eu2+ with Framework Topology Whj for LED/LCD-Backlighting Applications, Chem. Mater., 2015, 27(17), 6109–6115, DOI:10.1021/acs.chemmater.5b02702.
- Y. Xia, S. Li, Y. Zhang, T. Takeda, N. Hirosaki and R.-J. Xie, Discovery of a Ce3+ -Activated Red Nitride Phosphor for High-Brightness Solid-State Lighting, J. Mater. Chem. C, 2020, 8(41), 14402–14408, 10.1039/D0TC03964K.
- F. J. DiSalvo and S. J. Clarke, Ternary Nitrides: A Rapidly Growing Class of New Materials, Curr. Opin. Solid State Mater. Sci., 1996, 1(2), 241–249, DOI:10.1016/S1359-0286(96)80091-X.
- R. Niewa and F. J. DiSalvo, Recent Developments in Nitride Chemistry, Chem. Mater., 1998, 10(10), 2733–2752, DOI:10.1021/cm980137c.
-
P. Schmidt, A. Tuecks, J. Meyer, H. Bechtel, D. Wiechert, R. Mueller-Mach, G. Mueller and W. Schnick, in Materials Design and Properties of Nitride Phosphors for LEDs, ed. I. T. Ferguson, N. Narendran, T. Taguchi, I. E. Ashdown, San Diego, CA, 2007, p. 66690P DOI:10.1117/12.734227.
- S. R. Römer and P. Kroll, Schnick, W. High-Pressure Phases and Transitions of the Layered Alkaline Earth Nitridosilicates SrSiN2 and BaSiN2, J. Phys.: Condens. Matter, 2009, 21(27), 275408, DOI:10.1088/0953-8984/21/27/275408.
- H. A. Höppe, H. Lutz, P. Morys, W. Schnick and A. Seilmeier, Luminescence in Eu2+-Doped Ba2Si5N8: Fluorescence, Thermoluminescence, and Upconversion, J. Phys. Chem. Solids, 2000, 61(12), 2001–2006, DOI:10.1016/S0022-3697(00)00194-3.
- X. Q. Piao, K. Machida, T. Horikawa, H. Hanzawa, Y. Shimomura and N. Kijima, Preparation of CaAlSiN3:Eu2+ Phosphors by the Self-Propagating High-Temperature Synthesis and Their Luminescent Properties, Chem. Mater., 2007, 19(18), 4592–4599, DOI:10.1021/cm070623c.
- Z. A. Gál, P. M. Mallinson, H. J. Orchard and S. J. Clarke, Synthesis and Structure of Alkaline Earth Silicon Nitrides: BaSiN2, SrSiN2, and CaSiN2, Inorg. Chem., 2004, 43(13), 3998–4006, DOI:10.1021/ic049901p.
- L. Seijo and Z. Barandiarán, Host Effects on the Optically Active 4f and 5d Levels of Ce3+ in Garnets, Phys. Chem. Chem. Phys., 2013, 15(44), 19221, 10.1039/c3cp53465k.
- J. O. Rubio, H. S. Murrieta, J. A. Hernández and F. J. López, Addendum to “Optical Absorption and Luminescence Investigations of the Precipitated Phases of Eu2+ in NaCl and KCl Single Crystals.”, Phys. Rev. B: Condens. Matter Mater. Phys., 1981, 24(8), 4847–4851, DOI:10.1103/PhysRevB.24.4847.
- J. Sugar and N. Spector, Spectrum and Energy Levels of Doubly Ionized Europium (Eu III, J. Opt. Soc. Am., 1974, 64(11), 1484, DOI:10.1364/JOSA.64.001484.
- P. Dorenbos, Relation between Eu2+ and Ce3+ f↔d-Transition Energies in Inorganic Compounds, J. Phys.: Condens. Matter, 2003, 15(27), 4797–4807, DOI:10.1088/0953-8984/15/27/311.
- S. X. Wang, Z. Song, Y. W. Kong, Z. G. Xia and Q. L. Liu, Crystal Field Splitting of 4fn−15d-Levels of Ce3+ and Eu2+ in Nitride Compounds, J. Lumin., 2018, 194, 461–466, DOI:10.1016/j.jlumin.2017.10.073.
- P. Dorenbos, Electronic Structure Engineering of Lanthanide Activated Materials, J. Mater. Chem., 2012, 22(42), 22344, 10.1039/c2jm34252a.
- W. M. Yen, General Factors Governing the Efficiency of Luminescent Devices, Phys. Solid State, 2005, 47(8), 1393, DOI:10.1134/1.2014476.
- A. J. J. Bos, P. Dorenbos, A. Bessière, A. Lecointre, M. Bedu, M. Bettinelli and F. Piccinelli, Study of TL Glow Curves of YPO4 Double Doped with Lanthanide Ions, Radiat. Meas., 2011, 46(12), 1410–1416, DOI:10.1016/j.radmeas.2011.04.021.
- P. Dorenbos, Modeling the Chemical Shift of Lanthanide 4f Electron Binding Energies, Phys. Rev. B: Condens. Matter Mater. Phys., 2012, 85(16), 165107, DOI:10.1103/PhysRevB.85.165107.
- P. Dorenbos, Determining Binding Energies of Valence-Band Electrons in Insulators and Semiconductors via Lanthanide Spectroscopy, Phys. Rev. B: Condens. Matter Mater. Phys., 2013, 87(3), 035118, DOI:10.1103/PhysRevB.87.035118.
- P. Dorenbos, Charge Transfer Bands in Optical Materials and Related Defect Level Location, Opt. Mater., 2017, 69, 8–22, DOI:10.1016/j.optmat.2017.03.061.
- P. Dorenbos, Electronic Structure and Optical Properties of the Lanthanide Activated RE3(Al1−xGax)5O12 (RE=Gd, Y, Lu) Garnet Compounds, J. Lumin., 2013, 134, 310–318, DOI:10.1016/j.jlumin.2012.08.028.
- P. Dorenbos, Valence Stability of Lanthanide Ions in Inorganic Compounds, Chem. Mater., 2005, 17(25), 6452–6456, DOI:10.1021/cm051456o.
- X. L. Liu, Z. Song, S. X. Wang and Q. L. Liu, The Red Persistent Luminescence of (Sr,Ca)AlSiN3:Eu2+ and Mechanism Different to SrAl2O4:Eu2+,Dy3+, J. Lumin., 2019, 208, 313–321, DOI:10.1016/j.jlumin.2018.12.069.
- A. J. J. Bos, R. M. van Duijvenvoorde, E. van der Kolk, W. Drozdowski and P. Dorenbos, Thermoluminescence Excitation Spectroscopy: A Versatile Technique to Study Persistent Luminescence Phosphors, J. Lumin., 2011, 131(7), 1465–1471, DOI:10.1016/j.jlumin.2011.03.033.
- P. F. Smet, K. Van den Eeckhout, A. J. J. Bos, E. van der Kolk and P. Dorenbos, Temperature and Wavelength Dependent Trap Filling in M2Si5N8:Eu (M=Ca, Sr, Ba) Persistent Phosphors, J. Lumin., 2012, 132(3), 682–689, DOI:10.1016/j.jlumin.2011.10.022.
-
T. M. Dunn, D. S. McClure and R. G. Pearson, Some Aspects of Crystal Field Theory, Harper & Row, New York, 1965 Search PubMed.
-
S. Sugano, Y. Tanabe and H. Kamimura, Multiplets of Transition-Metal Ions in Crystals, Academic Press, 1970 Search PubMed.
- Zhen Song and Quan Lin Liu, Crystal-Field Splitting in Regular Polyhedron. Chin, J. Lumin., 2022, 43(9), 1428–1435 Search PubMed.
- G. Blasse and A. Bril, Investigation of Some Ce3+-Activated Phosphors, J. Chem. Phys., 1967, 47(12), 5139–5145, DOI:10.1063/1.1701771.
- Z. Song, X. L. Liu, L. Z. He and Q. L. Liu, Correlation between the Energy Level Structure of Cerium-Doped Yttrium Aluminum Garnet and Luminescent Behavior at Varying Temperatures, Mater. Res. Express, 2016, 3(5), 055501, DOI:10.1088/2053-1591/3/5/055501.
- J. G. Li and Y. Sakka, Recent Progress in Advanced Optical Materials Based on Gadolinium Aluminate Garnet (Gd3Al5O12, Sci. Technol. Adv. Mater., 2015, 16(1), 014902, DOI:10.1088/1468-6996/16/1/014902.
- T. Hoshina and S. Kuboniwa, 4f–5d Transition of Tb3+ and Ce3+ in MPO4 (M= Sc, Y and Lu), J. Phys. Soc. Jpn., 1971, 31(3), 828–840, DOI:10.1143/JPSJ.31.828.
- D. J. Robbins, The Effects of Crystal Field and Temperature on the Photoluminescence Excitation Efficiency
of Ce3+ in YAG, J. Electrochem. Soc., 1979, 126(9), 1550–1555, DOI:10.1149/1.2129328.
- T. Y. Tien, E. F. Gibbons, R. G. DeLosh, P. J. Zacmanidis, D. E. Smith and H. L. Stadler, Ce3+ Activated Y3Al5O12 and Some of Its Solid Solutions, J. Electrochem. Soc., 1973, 120(2), 278–281, DOI:10.1149/1.2403436.
- D. Robbins, B. Cockayne, J. Glasper and B. Lent, The Temperature Dependence of Rare-Earth Activated Garnet Phosphors: I. Intensity and Lifetime Measurements on Undoped and Ce-Doped, J. Electrochem. Soc., 1979, 126(7), 1213 CrossRef CAS.
- X. Liu, X. Wang and W. Shun, Luminescence Properties of the Ce3+ Ion in Yttrium Gallium Garnet, Phys. Status Solidi A, 1987, 101(2), K161–K165, DOI:10.1002/pssa.2211010256.
- Z. Song, Z. G. Xia and Q. L. Liu, Insight into the Relationship between Crystal Structure and Crystal-Field Splitting of Ce3+ Doped Garnet Compounds, J. Phys. Chem. C, 2018, 122(6), 3567–3574, DOI:10.1021/ACS.JPCC.7B12826.
- Z. Song, Z. Z. Wang, L. Z. He, R. J. Zhang, X. L. Liu, Z. G. Xia, W. T. Geng and Q. L. Liu, After-Glow, Luminescent Thermal Quenching, and Energy Band Structure of Ce-Doped Yttrium Aluminum-Gallium Garnets, J. Lumin., 2017, 192, 1278–1287, DOI:10.1016/J.JLUMIN.2017.09.008.
- W. Ahn and Y. J. Kim, Substitutional Solubility Limit for Ce3+ Ions in Lu3Al5O12: xCe3+ and Its Effect on Photoluminescence, Ceram. Int., 2017, 43(S1), S412–S416, DOI:10.1016/j.ceramint.2017.05.221.
- P. Dorenbos, Electronic Structure and Optical Properties of the Lanthanide Activated RE3(Al1−xGax)5O12 (RE=Gd, Y, Lu) Garnet Compounds, J. Lumin., 2013, 134, 310–318, DOI:10.1016/j.jlumin.2012.08.028.
- J. L. Wu, G. Gundiah and A. K. Cheetham, Structure–Property Correlations in Ce-Doped Garnet Phosphors for Use in Solid State Lighting, Chem. Phys. Lett., 2007, 441(4–6), 250–254, DOI:10.1016/j.cplett.2007.05.023.
- J. Zhong, W. Zhao, W. Zhuang, W. Xiao, Y. Zheng, F. Du and L. Wang, Origin of Spectral Blue Shift of Lu3+-Codoped YAG:Ce3+ Phosphor: First-Principles Study, ACS Omega, 2017, 2(9), 5935–5941, DOI:10.1021/acsomega.7b00304.
- C. Görller-Walrand and K. Binnemans, Rationalization of Crystal-Field Parametrization, Handbook on the physics and chemistry of rare earths, 1996, 23, 121–283, DOI:10.1016/s0168-1273(96)23006-5.
-
G. Liu and B. Jacquier, Spectroscopic Properties of Rare Earths in Optical Materials, Springer Science & Business Media, 2006, vol. 83 Search PubMed.
- Z. Song and Q. L. Liu, Structural Indicator to Characterize the Crystal-Field Splitting of Ce3+ in Garnets, J. Phys. Chem. C, 2020, 124(1), 870–873, DOI:10.1021/acs.jpcc.9b09322.
- Z. Song and Q. L. Liu, Effects of Neighboring Polyhedron Competition on the 5d Level of Ce3+ in Lanthanide Garnets, J. Phys. Chem. C, 2019, 123(14), 8656–8662, DOI:10.1021/acs.jpcc.9b00395.
- L. Seijo and Z. Barandiarán, 4f and 5d Levels of Ce3+ in D2 8-Fold Oxygen Coordination, Opt. Mater., 2013, 35(11), 1932–1940, DOI:10.1016/j.optmat.2012.12.007.
- Y. W. Kong, Z. Song, S. X. Wang, Z. G. Xia and Q. L. Liu, The Inductive Effect in Nitridosilicates and Oxysilicates and Its Effects on 5d Energy Levels of Ce3+, Inorg. Chem., 2018, 57(4), 2320–2331, DOI:10.1021/acs.inorgchem.7b03253.
- W. Noll, The Silicate Bond from the Standpoint of Electronic Theory, Angew. Chem., Int. Ed. Engl., 1963, 2(2), 73–80, DOI:10.1002/anie.196300731.
- J. Etourneau, J. Portier and F. Ménil, The Role of the Inductive Effect in Solid State Chemistry: How the Chemist Can Use It to Modify Both the Structural and the Physical Properties of the Materials, J. Alloys Compd., 1992, 188, 1–7, DOI:10.1016/0925-8388(92)90635-M.
- W. G. Xiao, D. Wu, L. L. Zhang, X. Zhang, Z. D. Hao, G.-H. Pan, L. G. Zhang, X. W. Ba and J. H. Zhang, The Inductive Effect of Neighboring Cations in Tuning Luminescence Properties of the Solid Solution Phosphors, Inorg. Chem., 2017, 56(16), 9938–9945, DOI:10.1021/acs.inorgchem.7b01457.
- Y. W. Kong, Z. Song, S. X. Wang, Z. G. Xia and Q. L. Liu, Charge Transfer, Local Structure, and the Inductive Effect in Rare-Earth-Doped Inorganic Solids, Inorg. Chem., 2018, 57(19), 12376–12383, DOI:10.1021/acs.inorgchem.8b02141.
- S. X. Wang, Z. Song, Y. W. Kong and Q. L. Liu, Relationship of Stokes Shift with Composition and Structure in Ce3+/Eu2+-Doped Inorganic Compounds, J. Lumin., 2019, 212, 250–263, DOI:10.1016/j.jlumin.2019.04.036.
-
B. Henderson and G. F. Imbusch, Optical Spectroscopy of Inorganic Solids, Clarendon Press, Oxford, 1989 Search PubMed.
- M. Nazarov, B. Tsukerblat and D. Y. Noh, Electron–Vibrational Interaction in 4f–5d Optical Transitions in Ba, Ca, Sr Thiogallates Doped with Eu2+ Ions, J. Lumin., 2008, 128(9), 1533–1540, DOI:10.1016/j.jlumin.2008.02.008.
- P. Bhoyar and S. J. Dhoble, Electron–Vibrational Interaction in 5d State of Eu2+ Ion in LiMgBF6, Li2NaBF6 and Li3BF6:Eu2+ Phosphors, J. Lumin., 2013, 139, 22–27, DOI:10.1016/j.jlumin.2013.01.038.
- M. Kottaisamy, R. Jagannathan, P. Jeyagopal, R. P. Rao and R. Narayanan, Eu2+ Luminescence in M5(PO4)3X Apatites, where M is Ca2+, Sr2+ and Ba 2+, and X is F−, Cl−, Br− and OH−, J. Phys. Appl. Phys., 1994, 27(10), 2210–2215, DOI:10.1088/0022-3727/27/10/034.
- M. G. Brik and C. N. Avram, Exchange Charge Model and Analysis of the Microscopic Crystal Field Effects in KAl(MoO4)2:Cr3+, J. Lumin., 2011, 131(12), 2642–2645, DOI:10.1016/j.jlumin.2011.06.034.
- S. J. Camardello, P. J. Toscano, M. G. Brik and A. M. Srivastava, Optical Spectroscopy, Thermal Quenching and Electron–Vibrational Interaction of the Octahedrally Coordinated Eu2+ Ion in SrAl2B2O7, J. Lumin., 2014, 151, 256–260, DOI:10.1016/j.jlumin.2014.02.029.
- A. Yelisseyev, Z. S. Lin, M. Starikova, L. Isaenko and S. Lobanov, Optical Transitions Due to Native Defects in Nonlinear Optical Crystals LiGaS2, J. Appl. Phys., 2012, 111(11), 113507, DOI:10.1063/1.4723645.
- L. Guerbous, M. Seraiche and O. Krachni, Photoluminescence and Electron-Vibrational Interaction in 4fn−15d States of Ce3+ or Pr3+ Ions Doped LnBO3 (Ln=Lu, Y, La) Orthoborates Materials, J. Lumin., 2013, 134, 165–173, DOI:10.1016/j.jlumin.2012.08.053.
-
G. Blasse, Interaction Between Optical Centers and Their Surroundings: An Inorganic Chemist's Approach, Advances in Inorganic Chemistry, Elsevier, 1990, vol. 35, pp. 319–402 DOI:10.1016/S0898-8838(08)60165-8.
- G. Blasse, J. P. M. van Vliet, J. W. M. Verwey, R. Hoogendam and M. Wiegel, Luminescence of Pr3+ in Scandium Borate (ScBO3) and the Host Lattice Dependence of the Stokes Shift, J. Phys. Chem. Solids, 1989, 50(6), 583–585, DOI:10.1016/0022-3697(89)90451-4.
- M. Y. Ding, T. J. Chen, C. H. Lu, Y. R. Ni and Z. Z. Xu, Preparation and Upconversion Luminescence Properties of Hexagonal NaYF4:Yb3+,Er3+ Microparticles, J. NanJing Tech University, 2015, 37, 11–22 CAS.
- N. Yamashita, O. Harada and K. Nakamura, Photoluminescence Spectra of Eu2+ Centers in Ca(S, Se):Eu and Sr(S, Se):Eu, Jpn. J. Appl. Phys., 1995, 34(Part 1, No. 10), 5539–5545, DOI:10.1143/JJAP.34.5539.
- C. W. Struck and W. H. Fonger, Unified model of the temperature quenching of narrow-line and broad-band emissions, J. Lumin., 1975, 10, 1–30 CrossRef CAS.
- L. S. Rohwer and J. E. Martin, Measuring the Absolute Quantum Efficiency of Luminescent Materials, J. Lumin., 2005, 115(3–4), 77–90, DOI:10.1016/j.jlumin.2005.01.013.
- P. F. Smet and J. J. Joos, Stabilizing Colour and Intensity, Nat. Mater., 2017, 16(5), 500–501, DOI:10.1038/nmat4901.
- Z. G. Xia and A. Meijerink, Ce3+ -Doped Garnet Phosphors: Composition Modification, Luminescence Properties and Applications, Chem. Soc. Rev., 2017, 46(1), 275–299, 10.1039/C6CS00551A.
- J. Ueda, K. Aishima and S. Tanabe, Temperature and Compositional Dependence of Optical and Optoelectronic Properties in Ce3+-Doped Y3Sc2Al3−xGaxO12 (X=0, 1, 2, 3, Opt. Mater., 2013, 35(11), 1952–1957, DOI:10.1016/j.optmat.2012.11.016.
- L. Z. He, Z. Song, Q. C. Xiang, Z. G. Xia and Q. L. Liu, Relationship between Thermal Quenching of Eu2+ Luminescence and Cation Ordering in (Ba1−xSrx)2SiO4:Eu Phosphors, J. Lumin., 2016, 180, 163–168, DOI:10.1016/j.jlumin.2016.08.031.
- P. Dorenbos, Thermal Quenching of Eu2+ 5d–4f Luminescence in Inorganic Compounds, J. Phys.: Condens. Matter, 2005, 17(50), 8103–8111, DOI:10.1088/0953-8984/17/50/027.
- J. Ueda, S. Tanabe and T. Nakanishi, Analysis of Ce3+ Luminescence Quenching in Solid Solutions between Y3Al5O12 and Y3Ga5O12 by Temperature Dependence of Photoconductivity Measurement, Journal of Applied Physics, 2011, 110(5), 053102, DOI:10.1063/1.3632069.
- V. Bachmann, C. Ronda and A. Meijerink, Temperature Quenching of Yellow Ce3+ Luminescence in YAG:Ce, Chem. Mater., 2009, 21(10), 2077–2084, DOI:10.1021/cm8030768.
- K. Van den Eeckhout, P. F. Smet and D. Poelman, Persistent Luminescence in Eu2+-Doped Compounds: A Review, Materials, 2010, 3(4), 2536–2566, DOI:10.3390/ma3042536.
- A. Nakatsuka, A. Yoshiasa and T. Yamanaka, Cation Distribution and Crystal Chemistry of Y3Al5−xGaxO12 (0 ≤ x ≤ 5) Garnet Solid Solutions, Acta Crystallogr., Sect. B: Struct. Sci., 1999, 55(3), 266–272, DOI:10.1107/S0108768198012567.
- Z. Song, D. D. Zhou and Q. L. Liu, Tolerance Factor and Phase Stability of the Garnet Structure, Acta Crystallogr., Sect. C: Struct. Chem., 2019, 75(10), 1353–1358, DOI:10.1107/S2053229619011975.
- G. Blasse and A. Bril, A New Phosphor for Flying-spot Cathode-ray Tubes for Color Television: Yellow-emitting Y3Al5O12–Ce3+, Appl. Phys. Lett., 1967, 11(2), 53–55, DOI:10.1063/1.1755025.
- Y. X. Pan, M. M. Wu and Q. Su, Tailored Photoluminescence of YAG:Ce Phosphor through Various Methods, J. Phys. Chem. Solids, 2004, 65(5), 845–850, DOI:10.1016/j.jpcs.2003.08.018.
- Z. Zhou, N. Zhou, X. Lu, M. T. Kate, D. Valdesueiro, J. Ruud van Ommen and H. T. (Bert) Hintzen, Performance Improvement by Alumina Coatings on Y3Al5O12:Ce3+ Phosphor Powder Deposited Using Atomic Layer Deposition in a Fluidized Bed Reactor, RSC Adv., 2016, 6(80), 76454–76462, 10.1039/C6RA12983H.
- N. C. George, A. J. Pell, G. Dantelle, K. Page, A. Llobet, M. Balasubramanian, G. Pintacuda, B. F. Chmelka and R. Seshadri, Local Environments of Dilute Activator Ions in the Solid-State Lighting Phosphor Y3–xCexAl5O12, Chem. Mater., 2013, 25(20), 3979–3995, DOI:10.1021/cm401598n.
- K. Li and C. Y. Shen, White LED Based on Nano-YAG:Ce3+/YAG:Ce3+,Gd3+ Hybrid Phosphors, Optik, 2012, 123(7), 621–623, DOI:10.1016/j.ijleo.2011.06.005.
- Q. Y. Shao, Y. Dong, J. Q. Jiang, C. Liang and J. H. He, Temperature-Dependent Photoluminescence Properties of (Y, Lu)3Al5O12:Ce3+ Phosphors for White LEDs Applications, J. Lumin., 2011, 131(5), 1013–1015, DOI:10.1016/j.jlumin.2011.01.013.
- C.-C. Chiang, M.-S. Tsai and M.-H. Hon, Preparation of Cerium-Activated GAG Phosphor Powders: Influence of Co-Doping on Crystallinity and Luminescent Properties, J. Electrochem. Soc., 2007, 154(10), J326, DOI:10.1149/1.2768900.
- C.-C. Chiang, M.-S. Tsai and M.-H. Hon, Luminescent Properties of Cerium-Activated Garnet Series Phosphor: Structure and Temperature Effects, J. Electrochem. Soc., 2008, 155(6), B517, DOI:10.1149/1.2898093.
- J. A. Mares, A. Beitlerova, M. Nikl, N. Solovieva, C. D’Ambrosio, K. Blazek, P. Maly, K. Nejezchleb and F. de Notaristefani, Scintillation Response of Ce-Doped or Intrinsic Scintillating Crystals in the Range up to 1 MeV, Radiat. Meas., 2004, 38(4–6), 353–357, DOI:10.1016/j.radmeas.2004.04.004.
- J. M. Ogiegło, A. Zych, K. V. Ivanovskikh, T. Jüstel, C. R. Ronda and A. Meijerink, Luminescence and Energy Transfer in Lu3Al5O12 Scintillators Co-Doped with Ce3+ and Tb3+, J. Phys. Chem. A, 2012, 116(33), 8464–8474, DOI:10.1021/jp301337f.
- J. Y. Zhong, W. D. Zhuang, X. R. Xing, R. H. Liu, Y. F. Li, Y. H. Liu and Y. S. Hu, Synthesis, Crystal Structures, and Photoluminescence Properties of Ce3+-Doped Ca2LaZr2Ga3O12: New Garnet Green-Emitting Phosphors for White LEDs, J. Phys. Chem. C, 2015, 119(10), 5562–5569, DOI:10.1021/jp508409r.
- J. L. Wu, G. Gundiah and A. K. Cheetham, Structure–Property Correlations in Ce-Doped Garnet Phosphors for Use in Solid State Lighting, Chem. Phys. Lett., 2007, 441(4–6), 250–254, DOI:10.1016/j.cplett.2007.05.023.
- Y. Shimomura, T. Honma, M. Shigeiwa, T. Akai, K. Okamoto and N. Kijima, Photoluminescence and Crystal Structure of Green-Emitting Ca3Sc2Si3O12:Ce3+ Phosphor for White Light Emitting Diodes, J. Electrochem. Soc., 2007, 154(1), J35, DOI:10.1149/1.2388856.
- M. C. Maniquiz, K. Y. Jung and S. M. Jeong, Luminescence Comparison and Enhancement of Ce-Doped Yttrium Aluminum Garnet Phosphor via Cation Substitution and Adding Flux, J. Electrochem. Soc., 2011, 158(7), H697, DOI:10.1149/1.3586043.
- J. M. Ogiegło, A. Katelnikovas, A. Zych, T. Jüstel, A. Meijerink and C. R. Ronda, Luminescence and Luminescence Quenching in Gd3(Ga,Al)5O12 Scintillators Doped with Ce3+, J. Phys. Chem. A, 2013, 117(12), 2479–2484, DOI:10.1021/jp309572p.
- Y.-F. Liu, P. Liu, L. Wang, C.-E. Cui, H.-C. Jiang and J. Jiang, A Two-Step Solid-State Reaction to Synthesize the Yellow Persistent Gd3Al2Ga3O12:Ce3+ Phosphor with an Enhanced Optical Performance for AC-LEDs, Chem. Commun., 2017, 53(77), 10636–10639, 10.1039/C7CC05041K.
- S. K. Sharma, Y.-C. Lin, I. Carrasco, T. Tingberg, M. Bettinelli and M. Karlsson, Weak Thermal Quenching of the Luminescence in the Ca3Sc2Si3O12:Ce3+ Garnet Phosphor, J. Mater. Chem. C, 2018, 6(33), 8923–8933, 10.1039/C8TC02907E.
- Y. B. Chen, M. L. Gong, G. Wang and Q. Su, High Efficient and Low Color-Temperature White Light-Emitting Diodes with Tb3Al5O12:Ce3+ Phosphor, Appl. Phys. Lett., 2007, 91(7), 071117, DOI:10.1063/1.2771538.
- C. C. Chiang, M. S. Tsai and M. H. Hon, Synthesis and Photoluminescent Properties of Ce3+ Doped Terbium Aluminum Garnet Phosphors, J. Alloys Compd., 2007, 431(1–2), 298–302, DOI:10.1016/j.jallcom.2006.05.068.
- Y. Chen, J. Wang, M. L. Gong and Q. Su, Comparative Study on the Synthesis, Photoluminescence and Application in InGaN-Based Light-Emitting Diodes of TAG:Ce3+ Phosphors, J. Solid State Chem., 2007, 180(4), 1165–1170, DOI:10.1016/j.jssc.2007.01.011.
- H. C. Jung, J. Y. Park, G. S. R. Raju, B. C. Choi, J. H. Jeong and B. K. Moon, Enhancement of Red Emission in Aluminum Garnet Yellow Phosphors by Sb3+ Substitution for the Octahedral Site: Enhancement of Red Emission in Aluminum Garnet Yellow Phosphors, J. Am. Ceram. Soc., 2011, 94(2), 551–555, DOI:10.1111/j.1551-2916.2010.04130.x.
- A. A. Setlur, W. J. Heward, M. E. Hannah and U. Happek, Incorporation of Si4+–N3− into Ce3+-Doped Garnets for Warm White LED Phosphors, Chem. Mater., 2008, 20(19), 6277–6283, DOI:10.1021/cm801732d.
- X. J. Wang, G. H. Zhou, H. L. Zhang, H. L. Li, Z. J. Zhang and Z. Sun, Luminescent Properties of Yellowish Orange Y3Al5−xSixO12−xNx:Ce Phosphors and Their Applications in Warm White Light-Emitting Diodes, J. Alloys Compd., 2012, 519, 149–155, DOI:10.1016/j.jallcom.2011.12.158.
- A. Katelnikovas, H. Bettentrup, D. Uhlich, S. Sakirzanovas, T. Jüstel and A. Kareiva, Synthesis and Optical Properties of Ce3+-Doped Y3Mg2AlSi2O12 Phosphors, J. Lumin., 2009, 129(11), 1356–1361, DOI:10.1016/j.jlumin.2009.07.006.
- A. Katelnikovas, J. Jurkevičius, K. Kazlauskas, P. Vitta, T. Jüstel, A. Kareiva, A. Žukauskas and G. Tamulaitis, Efficient Cerium-Based Sol–Gel Derived Phosphors in Different Garnet Matrices for Light-Emitting Diodes, J. Alloys Compd., 2011, 509(21), 6247–6251, DOI:10.1016/j.jallcom.2011.03.032.
- A. A. Setlur, W. J. Heward, Y. Gao, A. M. Srivastava, R. G. Chandran and M. V. Shankar, Crystal Chemistry and Luminescence of Ce3+-Doped Lu2CaMg2(Si,Ge)3O12 and Its Use in LED Based Lighting, Chem. Mater., 2006, 18(14), 3314–3322, DOI:10.1021/cm060898c.
- A. Katelnikovas, J. Plewa, D. Dutczak, S. Möller, D. Enseling, H. Winkler, A. Kareiva and T. Jüstel, Synthesis and Optical Properties of Green Emitting Garnet Phosphors for Phosphor-Converted Light Emitting Diodes, Opt. Mater., 2012, 34(7), 1195–1201, DOI:10.1016/j.optmat.2012.01.034.
- Z. Q. Jiang, Y. H. Wang and L. S. Wang, Enhanced Yellow-to-Orange Emission of Si-Doped Mg3Y2Ge3O12:Ce3+ Garnet Phosphors for Warm White Light-Emitting Diodes, J. Electrochem. Soc., 2010, 157(5), J155, DOI:10.1149/1.3328530.
- Z. F. Pan, W. Q. Li, Y. Xu, Q. S. Hu and Y. F. Zheng, Structure and Redshift of Ce3+ Emission in Anisotropically Expanded Garnet Phosphor MgY2Al4SiO12:Ce3+, RSC Adv., 2016, 6(25), 20458–20466, 10.1039/C6RA00356G.
- X. H. Gong, J. H. Huang, Y. J. Chen, Y. F. Lin, Z. D. Luo and Y. D. Huang, Novel Garnet-Structure Ca2GdZr2(AlO4)3:Ce3+ Phosphor and Its Structural Tuning of Optical Properties, Inorg. Chem., 2014, 53(13), 6607–6614, DOI:10.1021/ic500153u.
- L. L. Sun, B. Devakumar, J. Liang, S. Y. Wang, Q. Sun and X. Y. Huang, A Broadband Cyan-Emitting Ca2LuZr2(AlO4)3:Ce3+ Garnet Phosphor for near-Ultraviolet-Pumped Warm-White Light-Emitting Diodes with an Improved Color Rendering Index, J. Mater. Chem. C, 2020, 8(3), 1095–1103, 10.1039/C9TC04952E.
- X. Ding, G. Zhu, W. Y. Geng, Q. Wang and Y. H. Wang, Highly Efficient Cyan-Emitting Garnet Ca3Hf2SiAl2O12: XCe3+ Phosphor for Solid State White Lighting, CrystEngComm, 2015, 17(17), 3235–3242, 10.1039/C5CE00020C.
- Y. T. Wu and G. H. Ren, Crystal Growth, Structure, Optical and Scintillation Properties of Ce3+-Doped Tb2.2Lu0.8Al5O12 Single Crystals, CrystEngComm, 2013, 15(20), 4153, 10.1039/c3ce40324f.
- J. Ueda, P. Dorenbos, A. J. J. Bos, K. Kuroishi and S. Tanabe, Control of Electron Transfer between Ce3+ and Cr3+ in the Y3Al5−xGaxO12 Host via Conduction Band Engineering, J. Mater. Chem. C, 2015, 3(22), 5642–5651, 10.1039/C5TC00546A.
- B. Wang, H. Lin, Y. L. Yu, D. Q. Chen, R. Zhang, J. Xu and Y. S. Wang, Ce3+/Pr3+: YAGG: A Long Persistent Phosphor Activated by Blue-Light, J. Am. Ceram. Soc., 2014, 97(8), 2539–2545, DOI:10.1111/jace.12967.
- A. Katelnikovas, H. Winkler, A. Kareiva and T. Jüstel, Synthesis and Optical Properties of Green to Orange Tunable Garnet Phosphors for PcLEDs, Opt. Mater., 2011, 33(7), 992–995, DOI:10.1016/j.optmat.2010.11.023.
- H. Ji, L. Wang, M. S. Molokeev, N. Hirosaki, Z. H. Huang, Z. G. Xia, O. M. ten Kate, L. H. Liu and R. J. Xie, New Garnet Structure Phosphors, Lu3−xYxMgAl3SiO12:Ce3+ (x = 0–3), developed by Solid Solution Design, J. Mater. Chem. C, 2016, 4(12), 2359–2366, 10.1039/C6TC00089D.
- H. P. Ji, L. Wang, M. S. Molokeev, N. Hirosaki, R. Xie, Z. H. Huang, Z. G. Xia, O. M. ten Kate, L. H. Liu and V. V. Atuchin, Structure Evolution and Photoluminescence of Lu3(Al,Mg)2(Al,Si)3O12:Ce3+ Phosphors: New Yellow-Color Converters for Blue LED-Driven Solid State Lighting, J. Mater. Chem. C, 2016, 4(28), 6855–6863, 10.1039/C6TC00966B.
- Y. Zhou, W. D. Zhuang, Y. S. Hu, R. H. Liu, H. Xu, M. Y. Chen, Y. H. Liu, Y. F. Li, Y. L. Zheng and G. T. Chen, Cyan-Green Phosphor (Lu2M)(Al4Si)O12:Ce3+ for High-Quality LED Lamp: Tunable Photoluminescence Properties and Enhanced Thermal Stability, Inorg. Chem., 2019, 58(2), 1492–1500, DOI:10.1021/acs.inorgchem.8b03017.
- Y. Xiao, W. G. Xiao, L. L. Zhang, Z. D. Hao, G.-H. Pan, Y. Yang, X. Zhang and J. H. Zhang, A Highly Efficient and Thermally Stable Green Phosphor (Lu2SrAl4SiO12:Ce3+) for Full-Spectrum White LEDs, J. Mater. Chem. C, 2018, 6(45), 12159–12163, 10.1039/C8TC04101F.
- D. A. Hakeem, J. W. Pi, S. W. Kim and K. Park, New Y2LuCaAl2SiO12:Ln (Ln = Ce3+, Eu3+, and Tb3+) Phosphors for White LED Applications, Inorg. Chem. Front., 2018, 5(6), 1336–1345, 10.1039/C8QI00111A.
- Z. J. Zhang, J. Liang, L. L. Sun, S. Y. Wang, Q. Sun, B. Devakumar, S. Rtimi and X. Y. Huang, Synthesis and Photoluminescence Properties of Near-UV-Excitable Cyan-Emitting Ca2YHf2Ga3O12:Ce3+ Garnet Phosphors, J. Lumin., 2020, 227, 117544, DOI:10.1016/j.jlumin.2020.117544.
- B. C. Wang, R. Y. Mi, Y. Liu, M. Yu, Z. H. Huang and M. H. Fang, Identification of Dual Luminescence Centers from a Single Site in a Novel Blue-Pumped Ca3Sc2Ge3O12:Ce3+ Phosphor, Dalton Trans., 2019, 48(31), 11791–11802, 10.1039/C9DT01252D.
- J. Liang, L. L. Sun, S. Y. Wang, Q. Sun, B. Devakumar and X. Y. Huang, Filling the Cyan Gap toward Full-Visible-Spectrum LED Lighting with Ca2LaHf2Al3O12:Ce3+ Broadband Green Phosphor, J. Alloys Compd., 2020, 836, 155469, DOI:10.1016/j.jallcom.2020.155469.
- Y. Zhou, W. D. Zhuang, Y. S. Hu, R. H. Liu, Z. Jiang, Y. H. Liu, Y. F. Li, Y. L. Zheng, L. Chen and J. Y. Zhong, A Broad-Band Orange-Yellow-Emitting Lu2Mg2Al2Si2O12: Ce3+ Phosphor for Application in Warm White Light-Emitting Diodes, RSC Adv., 2017, 7(74), 46713–46720, 10.1039/C7RA08760H.
- Z. Wei, Y. F. Ji, M. H. Zhu, W. H. Qu, Z. Feng, W. N. Guo and J. J. Li, Luminescence Properties of Garnet Y2Mg2Al2Si2O12:Ce3+ Phosphors, Chem. Phys. Lett., 2021, 771, 138516, DOI:10.1016/j.cplett.2021.138516.
- Z. Song, Z. Z. Wang, L. Z. He, R. J. Zhang, X. L. Liu, Z. G. Xia, W. T. Geng and Q. L. Liu, After-Glow, Luminescent Thermal Quenching, and Energy Band Structure of Ce-Doped Yttrium Aluminum-Gallium Garnets, J. Lumin., 2017, 192, 1278–1287, DOI:10.1016/j.jlumin.2017.09.008.
- J. Ueda, K. Kuroishi and S. Tanabe, Bright Persistent Ceramic Phosphors of Ce3+-Cr3+-Codoped Garnet Able to Store by Blue Light, Appl. Phys. Lett., 2014, 104(10), 101904, DOI:10.1063/1.4868138.
- D. D. Zhou, Z. Song, H. Zhou and Q. L. Liu, Enhanced Persistent Luminescence via Si4+ Co-Doping in Y3Al2Ga3O12:Ce3+,Yb3+,B3+, J. Lumin., 2020, 222, 117190, DOI:10.1016/j.jlumin.2020.117190.
- J. Xu and J. Ueda, Tanabe, S. Toward Tunable and Bright Deep-Red Persistent Luminescence of Cr3+ in Garnets, J Am Ceram Soc, 2017, 100(9), 4033–4044, DOI:10.1111/jace.14942.
- W. W. Holloway and M. Kestigian, Optical Properties of Cerium-Activated Garnet Crystals, J. Opt. Soc. Am., 1969, 59(1), 60, DOI:10.1364/JOSA.59.000060.
- T. Kanai, M. Satoh and I. Miura, Characteristics of a Nonstoichiometric Gd3+δ(Al,Ga)5−δO12:Ce Garnet Scintillator, J. Am. Ceram. Soc., 2008, 91(2), 456–462, DOI:10.1111/j.1551-2916.2007.02123.x.
- Y. Luo and Z. G. Xia, Effect of Al/Ga Substitution on Photoluminescence and Phosphorescence Properties of Garnet-Type Y3Sc2Ga3–xAlxO12:Ce3+ Phosphor, J. Phys. Chem. C, 2014, 118(40), 23297–23305, DOI:10.1021/jp507695n.
- H. Lin, B. Wang, Q. Huang, F. Huang, J. Xu, H. Chen, Z. B. Lin, J. Wang, T. Hu and Y. S. Wang, Lu2CaMg2(Si1−xGex)3O12:Ce3+ Solid-Solution Phosphors: Bandgap Engineering for Blue-Light Activated Afterglow Applicable to AC-LED, J. Mater. Chem. C, 2016, 4(43), 10329–10338, 10.1039/C6TC03818B.
- J. Ueda, K. Aishima, S. Nishiura and S. Tanabe, Afterglow Luminescence in Ce3+-Doped Y3Sc2Ga3O12 Ceramics, Appl. Phys. Express, 2011, 4(4), 042602, DOI:10.1143/APEX.4.042602.
- J. Ueda, Analysis of Optoelectronic Properties and Development of New Persistent Phosphor in Ce3+-Doped Garnet Ceramics, J. Ceram. Soc. Jpn., 2015, 123(1444), 1059–1064, DOI:10.2109/jcersj2.123.1059.
- K. Asami, J. Ueda and S. Tanabe, Trap Depth and Color Variation of Ce3+-Cr3+ Co-Doped Gd3(Al,Ga)5O12 Garnet Persistent Phosphors, Opt. Mater., 2016, 62, 171–175, DOI:10.1016/j.optmat.2016.09.052.
- J. Ueda, K. Kuroishi and S. Tanabe, Yellow Persistent Luminescence in Ce3+–Cr3+-Codoped Gadolinium Aluminum Gallium Garnet Transparent Ceramics after Blue-Light Excitation, Appl. Phys. Express, 2014, 7(6), 062201, DOI:10.7567/APEX.7.062201.
- D. D. Zhou, Z. Z. Wang, Z. Song, F. X. Wang, S. Y. Zhang and Q. L. Liu, Enhanced Persistence Properties through Modifying the Trap Depth and Density in Y3Al2Ga3O12: Ce3+,Yb3+ Phosphor by Co-Doping B3+, Inorg. Chem., 2019, 58(2), 1684–1689, DOI:10.1021/acs.inorgchem.8b03270.
- J. Xu, J. Ueda and S. Tanabe, Novel Persistent Phosphors of Lanthanide–Chromium Co-Doped Yttrium Aluminum Gallium Garnet: Design Concept with Vacuum Referred Binding Energy Diagram, J. Mater. Chem. C, 2016, 4(20), 4380–4386, 10.1039/C6TC00802J.
- H. Watanabe and N. Kijima, Crystal Structure and Luminescence Properties of SrxCa1−xAlSiN3:Eu2+ Mixed Nitride Phosphors, J. Alloys Compd., 2009, 475(1–2), 434–439, DOI:10.1016/j.jallcom.2008.07.054.
- G. B. Nair, A. Kumar, H. C. Swart and S. J. Dhoble, Improved Steady-State Photoluminescence Derived from the Compensation of the Charge-Imbalance in Ca3Mg3(PO4)4:Eu3+ Phosphor, Ceram. Int., 2019, 45(17), 21709–21715, DOI:10.1016/j.ceramint.2019.07.171.
- T. Senden, E. J. van Harten and A. Meijerink, Synthesis and Narrow Red Luminescence of Cs2HfF6:Mn4+, a New Phosphor for Warm White LEDs, J. Lumin., 2018, 194, 131–138, DOI:10.1016/j.jlumin.2017.10.006.
- P. Dorenbos, The Eu3+ Charge Transfer Energy and the Relation with the Band Gap of Compounds, J. Lumin., 2005, 111(1–2), 89–104, DOI:10.1016/j.jlumin.2004.07.003.
- H.-D. Nguyen, C. C. Lin, M.-H. Fang and R.-S. Liu, Synthesis of Na2SiF6:Mn4+ Red Phosphors for White LED Applications by Co-Precipitation, J. Mater. Chem. C, 2014, 2(48), 10268–10272, 10.1039/C4TC02062F.
-
F. Ottinger, PhD thesis, Universitat Karlsruhe, Karlsruhe, 2004 Search PubMed.
- T. Wang, J. J. Yang, Y. D. Mo, L. Bian, Z. Song and Q. L. Liu, Synthesis, Structure and Tunable Red Emissions of Ca(Al/Si)2N2(N1−xOx): Eu2+ Prepared by Alloy-Nitridation Method, J. Lumin., 2013, 137, 173–179, DOI:10.1016/j.jlumin.2012.12.053.
- L. Chen, M. Fei, Z. Zhang, Y. Jiang, S. F. Chen, Y. Q. Dong, Z. H. Sun, Z. Zhao, Y. B. Fu, J. H. He, C. Li and Z. Jiang, Understanding the Local and Electronic Structures toward Enhanced Thermal Stable Luminescence of CaAlSiN3:Eu2+, Chem. Mater., 2016, 28(15), 5505–5515, DOI:10.1021/acs.chemmater.6b02121.
- J. Li, T. Watanabe, H. Wada, T. Setoyama and M. Yoshimura, Synthesis of Eu-Doped CaAlSiN3 from Ammonometallates: Effects of Sodium Content and Pressure, J. Am. Ceram. Soc., 2009, 92(2), 344–349, DOI:10.1111/j.1551-2916.2008.02883.x.
- J. W. Li, T. Watanabe, H. Wada, T. Setoyama and M. Yoshimura, Low-Temperature Crystallization of Eu-Doped Red-Emitting CaAlSiN3 from Alloy-Derived Ammonometallates, Chem. Mater., 2007, 19(15), 3592–3594, DOI:10.1021/cm070994t.
- T. Wang, Q. C. Xiang, Z. G. Xia, J. Chen and Q. L. Liu, Evolution of Structure and Photoluminescence by Cation Cosubstitution in Eu2+-Doped (Ca1−xLix)(Al1−xSi1+x)N3 Solid Solutions, Inorg. Chem., 2016, 55(6), 2929–2933, DOI:10.1021/acs.inorgchem.5b02845.
- S. R. Jansen, J. M. Migchels, H. T. Hintzen and R. Metselaar, Eu-Doped Barium Aluminum Oxynitride with the B-Alumina-Type Structure as New Blue-Emitting Phosphor, J. Electrochem. Soc., 1999, 146(2), 800–806, DOI:10.1149/1.1391684.
- Y. Q. Li, G. de With and H. T. Hintzen, Luminescence Properties of Eu2+-Doped MAl2−xSixO4−xNx (M=Ca, Sr, Ba) Conversion Phosphor for White LED Applications, J. Electrochem. Soc., 2006, 153(4), G278, DOI:10.1149/1.2167950.
- Y. Suda, Y. Kamigaki and H. Yamamoto, Blue Emission in Photoluminescence Spectra of the Red Phosphor CaAlSiN3:Eu2+ at Low Eu2+ Concentration, J. Appl. Phys., 2018, 123(16), 161542, DOI:10.1063/1.5004481.
- Y. Wang, F. Y. Zhao, X. Q. Piao, Z. Sun, T. Horikawa and K. Machida, Synthesis and Photoluminescence Properties of Divalent Europium Doped-CaAlSi1+2xN3+2xOx (x = 0–1) Solid Solution Phosphors, ECS J. Solid State Sci. Technol., 2013, 2(7), R131–R134, DOI:10.1149/2.014307jss.
- D. D. Jia, J. Zhu and B. Q. Wu, Trapping Centers in CaS:Bi3+ and CaS:Eu2+,Tm3+, J. Electrochem. Soc., 2000, 147(1), 386, DOI:10.1149/1.1393205.
- X. X. Wang, Z. T. Zhang, Z. L. Tang and Y. H. Lin, Characterization and Properties of a Red and Orange Y2O2S-Based Long Afterglow Phosphor, Mater. Chem. Phys., 2003, 80(1), 1–5, DOI:10.1016/S0254-0584(02)00097-4.
- B. Wang, H. Lin, J. Xu, H. Chen, Z. B. Lin, F. Huang and Y. S. Wang, Design, Preparation, and Characterization of a Novel Red Long-Persistent Perovskite Phosphor: Ca3Ti2O7:Pr3+, Inorg. Chem., 2015, 54(23), 11299–11306, DOI:10.1021/acs.inorgchem.5b01894.
- Y. Y. Zhang, R. Pang, C. Y. Li, C. Y. Zang and Q. Su, Reddish Orange Long Lasting Phosphorescence of Sm3+ in Sr2ZnSi2O7:Sm3+ Phosphors, J. Rare Earths, 2010, 28(5), 705–708, DOI:10.1016/S1002-0721(09)60184-8.
- J. Ueda, S. Tanabe, K. Takahashi, T. Takeda and N. Hirosaki, Thermal Quenching Mechanism of CaAlSiN3:Eu2+ Red Phosphor, Bull. Chem. Soc. Jpn., 2018, 91(2), 173–177, DOI:10.1246/bcsj.20170307.
- K. Uheda, N. Hirosaki and H. Yamamoto, Host Lattice Materials in the System Ca3N2-AlN-Si3N4 for White Light Emitting Diode, Phys. Status Solidi A, 2006, 203(11), 2712–2717, DOI:10.1002/pssa.200669576.
- Z. Zhang, O. M. ten Kate, A. Delsing, E. van der Kolk, P. H. L. Notten, P. Dorenbos, J. Zhao and H. T. Hintzen, Photoluminescence Properties and Energy Level Locations of RE3+ (RE = Pr, Sm, Tb, Tb/Ce) in CaAlSiN3 Phosphors, J. Mater. Chem., 2012, 22(19), 9813, 10.1039/c2jm30220a.
- H. Watanabe, H. Wada, K. Seki, M. Itou and N. Kijima, Synthetic Method and Luminescence Properties of SrxCa1−xAlSiN3:Eu2+ Mixed Nitride Phosphors, J. Electrochem. Soc., 2008, 155(3), F31, DOI:10.1149/1.2829880.
- Y. Q. Li, J. E. J. van Steen, J. W. H. van Krevel, G. Botty, A. C. A. Delsing, F. J. DiSalvo, G. de With and H. T. Hintzen, Luminescence Properties of Red-Emitting M2Si5N8:Eu2+ (M=Ca, Sr, Ba) LED Conversion Phosphor, J. Alloys Compd., 2006, 417(1–2), 273–279, DOI:10.1016/j.jallcom.2005.09.041.
- O. M. ten Kate, Z. Zhang, P. Dorenbos, H. T. Hintzen and E. van der Kolk, 4f and 5d Energy Levels of the Divalent and Trivalent Lanthanide Ions in M2Si5N8 (M=Ca, Sr, Ba, J. Solid State Chem., 2013, 197, 209–217, DOI:10.1016/j.jssc.2012.08.029.
- R.-J. Xie, N. Hirosaki, N. Kimura, K. Sakuma and M. Mitomo, 2-Phosphor-Converted White Light-Emitting Diodes Using Oxynitride/Nitride Phosphors, Appl. Phys. Lett., 2007, 90(19), 191101, DOI:10.1063/1.2737375.
- X. Q. Piao, K. Machida, T. Horikawa and H. Hanzawa, Synthesis of Nitridosilicate CaSr1−xEuxSi5N8 (x=0–1) Phosphor by Calcium Cyanamide Reduction for White Light-Emitting Diode Applicationss, J. Electrochem. Soc., 2008, 155(1), J17, DOI:10.1149/1.2801380.
- X. Q. Piao, T. Horikawa, H. Hanzawa and K. Machida, Preparation of (Sr1−xCax)2Si5N8/Eu2+ Solid Solutions and Their Luminescence Properties, J. Electrochem. Soc., 2006, 153(12), H232, DOI:10.1149/1.2360775.
- C. C. Lin, Y.-T. Tsai, H. E. Johnston, M.-H. Fang, F. J. Yu, W. Z. Zhou, P. Whitfield, Y. Li, J. Wang, R.-S. Liu and J. P. Attfield, Enhanced Photoluminescence Emission and Thermal Stability from Introduced Cation Disorder in Phosphors, J. Am. Chem. Soc., 2017, 139(34), 11766–11770, DOI:10.1021/jacs.7b04338.
- N. C. George, A. Birkel, J. Brgoch, B.-C. Hong, A. A. Mikhailovsky, K. Page, A. Llobet and R. Seshadri, Average and Local Structural Origins of the Optical Properties of the Nitride Phosphor La3–xCexSi6N11 (0 < x ≤ 3), Inorg. Chem., 2013, 52(23), 13730–13741 CrossRef CAS PubMed.
- S. H. You, S. X. Li, Y. C. Jia and R.-J. Xie, Interstitial Site Engineering for Creating Unusual Red Emission in La3Si6N11:Ce3+, Chem. Mater., 2020, 32(8), 3631–3640 CrossRef CAS.
- V. Bachmann, C. Ronda, O. Oeckler, W. Schnick and A. Meijerink, Color Point Tuning for (Sr,Ca,Ba)Si2O2N2:Eu2+ for White Light LEDs, Chem. Mater., 2009, 21(2), 316–325, DOI:10.1021/cm802394w.
- Y. X. Zhuang, Y. Lv, L. Wang, W. W. Chen, T.-L. Zhou, T. Takeda, N. Hirosaki and R.-J. Xie, Trap Depth Engineering of SrSi2O2N2:Ln2+,Ln3+(Ln2+ = Yb, Eu; Ln3+ = Dy, Ho, Er) Persistent Luminescence Materials for Information Storage Applications, ACS Appl. Mater. Interfaces, 2018, 10(2), 1854–1864, DOI:10.1021/acsami.7b17271.
- X. J. Zhang, M.-H. Fang, Y.-T. Tsai, A. Lazarowska, S. Mahlik, T. Lesniewski, M. Grinberg, W. K. Pang, F. J. Pan, C. L. Liang, W. Z. Zhou, J. Wang, J.-F. Lee, B.-M. Cheng, T.-L. Hung, Y.-Y. Chen and R.-S. Liu, Controlling of Structural Ordering and Rigidity of β-SiAlON:Eu through Chemical Cosubstitution to Approach Narrow-Band-Emission for Light-Emitting Diodes Application, Chem. Mater., 2017, 29(16), 6781–6792, DOI:10.1021/acs.chemmater.7b01697.
- S. X. Wang, X. L. Liu, B. Qu, Z. Song, Z. Z. Wang, S. Y. Zhang, F. X. Wang, W.-T. Geng and Q. L. Liu, Green Persistent Luminescence and the Electronic Structure of β-Sialon:Eu2+, J. Mater. Chem. C, 2019, 7(40), 12544–12551, 10.1039/C9TC03833G.
- L. Z. He, Z. Song, X. H. Jia, Z. G. Xia and Q. L. Liu, Consequence of Optimal Bonding on Disordered Structure and Improved Luminescence Properties in T-Phase (Ba,Ca)2SiO4:Eu2+ Phosphor, Inorg. Chem., 2018, 57(7), 4146–4154, DOI:10.1021/acs.inorgchem.8b00362.
- M. K. Chhina and K. Singh, Dy3+ and Inherent Ti4+ Activated Ca2SiO4 near White Light Emitting Phosphors Synthesized from Agro-Food Waste Ashes, Ceram. Int., 2020, 46(7), 9370–9379, DOI:10.1016/j.ceramint.2019.12.195.
- D. S. Jo, J. Kim, K. Senthil, H.-J. Lee, H. K. Chung, M. Kakihana, T. Kenji, T. Masaki and D. H. Yoon, Influence of Amorphous and Crystalline Type Precursor Intermediates on the Morphology and Luminescence Properties of Green Emitting β-Ca2SiO4:Eu2+ Phosphor Synthesized Using a Liquid Phase Precursor Method, J. Alloys Compd., 2014, 608, 311–317, DOI:10.1016/j.jallcom.2014.04.133.
- M. Mangalagowri, R. B. Basavaraj, G. P. Darshan, M. S. Raju, Y. V. Naik, D. Kavyashree, H. K. Inamdar, S. C. Sharma and H. Nagabhushana, Sonochemical Synthesis of Green Emitting Ca2SiO4:Er3+ Nanopowders: Promising Applications in Optical Thermometry and Radiation Dosimeter, Opt. Mater., 2019, 92, 125–135, DOI:10.1016/j.optmat.2019.04.005.
- K. Mondal, D. K. Singh and J. Manam, Spectroscopic Behavior, Thermal Stability and Temperature Sensitivity of Ca2SiO4:Eu3+ Red Emitting Phosphor for Solid State Lighting Application, J. Alloys Compd., 2018, 761, 41–51, DOI:10.1016/j.jallcom.2018.05.161.
- A. G. Bispo-Jr, S. A. M. Lima, L. D. Carlos, A. M. Pires and R. A. S. Ferreira, Eu(II)-Activated Silicates for UV Light-Emitting Diodes Tuning into Warm White Light, Adv. Eng. Mater., 2020, 22(8), 2000422, DOI:10.1002/adem.202000422.
- M. Somani, M. Saleem, M. Mittal and P. K. Sharma, Structural, Photoluminescent and Thermoluminescent Studies of Rare Earth Ion (RE = Eu3+) Doped Sr2SiO4 Phosphor, Optik, 2019, 182, 839–847, DOI:10.1016/j.ijleo.2019.01.091.
- H. Nagabhushana, D. V. Sunitha, S. C. Sharma, B. Daruka Prasad, B. M. Nagabhushana and R. P. S. Chakradhar, Enhanced Luminescence by Monovalent Alkali Metal Ions in Sr2SiO4:Eu3+ Nanophosphor Prepared by Low Temperature Solution Combustion Method, J. Alloys Compd., 2014, 595, 192–199, DOI:10.1016/j.jallcom.2014.01.094.
- M. Zhang, J. Wang, Q. H. Zhang, W. J. Ding and Q. Su, Optical Properties of Ba2SiO4:Eu2+ Phosphor for Green Light-Emitting Diode (LED), Mater. Res. Bull., 2007, 42(1), 33–39, DOI:10.1016/j.materresbull.2006.05.011.
- C. L. Wang, Y. H. Jin, Y. Lv, G. Ju, D. Liu, L. Chen, Z. Z. Li and Y. H. Hu, Trap Distribution Tailoring Guided Design of Super-Long-Persistent Phosphor Ba2SiO4:Eu2+,Ho3+ and Photostimulable Luminescence for Optical Information Storage, J. Mater. Chem. C, 2018, 6(22), 6058–6067, 10.1039/C8TC01722K.
- S. Tezuka, Y. Sato, T. Komukai, Y. Takatsuka, H. Kato and M. Kakihana, Eu2+-Activated CaSrSiO4: A New Red-Emitting Oxide Phosphor for White-Light-Emitting Diodes, Appl. Phys. Express, 2013, 6(7), 072101, DOI:10.7567/APEX.6.072101.
- J. K. Park, C. H. Kim, S. H. Park, H. D. Park and S. Y. Choi, Application of Strontium Silicate Yellow Phosphor for White Light-Emitting Diodes, Appl. Phys. Lett., 2004, 84(10), 1647–1649, DOI:10.1063/1.1667620.
- E.-H. Kang, S.-W. Choi, S. E. Chung, J. Jang, S. Kwon and S.-H. Hong, Photoluminescence Characteristics of Sr3SiO5:Eu2+ Yellow Phosphors Synthesized by Solid-State Method and Pechini Process, J. Electrochem. Soc., 2011, 158(11), J330, DOI:10.1149/2.016111jes.
- H. M. Liu, H. W. Liang, W. Y. Zhang, Q. G. Zeng and D. W. Wen, Improving the Thermal Stability and Luminescent Efficiency of (Ba,Sr)3SiO5:Eu2+ Phosphors by Structure, Bandgap Engineering and Soft Chemistry Synthesis Method, Chem. Eng. J., 2021, 410, 128367, DOI:10.1016/j.cej.2020.128367.
- J. K. Park, M. A. Lim, K. J. Choi and C. H. Kim, Luminescence Characteristics of Yellow Emitting Ba3SiO5:Eu2+ Phosphor, J. Mater. Sci., 2005, 40(8), 2069–2071, DOI:10.1007/s10853-005-1237-z.
- Y. J. Hua, H. P. Ma, D. G. Deng, S. L. Zhao, L. H. Huang, H. P. Wang and S. Q. Xu, Enhanced Photoluminescence Properties of Orange Emitting Sr2.96−xBaxSiO5:Eu2+ Phosphors Synthesized with Sr2SiO4 as Precursor, J. Lumin., 2014, 148, 39–43, DOI:10.1016/j.jlumin.2013.11.056.
- H. S. Jang and D. Y. Jeon, Yellow-Emitting Sr3SiO5:Ce3+,Li+ Phosphor for White-Light-Emitting Diodes and Yellow-Light-Emitting Diodes, Appl. Phys. Lett., 2007, 90(4), 041906, DOI:10.1063/1.2432947.
- J. Brgoch, S. P. DenBaars and R. Seshadri, Proxies from Ab Initio Calculations for Screening Efficient Ce3+ Phosphor Hosts, J. Phys. Chem. C, 2013, 117(35), 17955–17959, DOI:10.1021/jp405858e.
- H. D. Luo, J. Liu, X. Zheng, L. X. Han, K. X. Ren and X. B. Yu, Enhanced Photoluminescence of Sr3SiO5:Ce3+ and Tuneable Yellow Emission of Sr3SiO5:Ce3+,Eu2+ by Al3+ Charge Compensation for W-LEDs, J. Mater. Chem., 2012, 22(31), 15887, 10.1039/c2jm32293e.
- K. Van den Eeckhout, P. F. Smet and D. Poelman, Luminescent Afterglow Behavior in the M2Si5N8:Eu Family (M = Ca, Sr, Ba), Materials, 2011, 4(6), 980–990, DOI:10.3390/ma4060980.
- S. X. Wang, Z. Song, Y. W. Kong, S. Y. Zhang, Z. G. Xia and Q. L. Liu, Enhanced Performance of Sr2Si5N8:Eu2+ Red Afterglow Phosphor by Co-Doping with Boron and Oxygen, J. Lumin., 2018, 204, 36–40, DOI:10.1016/j.jlumin.2018.07.045.
- X. M. Teng, Y. H. Liu, Y. Z. Liu, Y. S. Hu, H. Q. He and W. D. Zhuang, Luminescence Properties of Tm3+ Co-Doped Sr2Si5N8:Eu2+ Red Phosphor, J. Lumin., 2010, 130(5), 851–854, DOI:10.1016/j.jlumin.2009.12.012.
- J. Wang, H. R. Zhang, B. F. Lei, H. W. Dong, H. Zhang, Y. L. Liu, N. L. Lai, Y. Fang and Z. J. Chen, Red Persistent and Photo-Stimulated Luminescence Properties of SrCaSi5N8:Eu2+,Tm3+ Solid Solution, Opt. Mater., 2014, 36(11), 1855–1858, DOI:10.1016/j.optmat.2014.04.048.
- D. Dutczak, A. Milbrat, A. Katelnikovas, A. Meijerink, C. Ronda and T. Jüstel, Yellow Persistent Luminescence of Sr2SiO4:Eu2+,Dy3+, J. Lumin., 2012, 132(9), 2398–2403, DOI:10.1016/j.jlumin.2012.03.055.
- D. Verma, R. P. Patel and M. L. Verma, Optical Properties of Sr2SiO4:Eu2+,Dy3+ Phosphors Prepared by Combustion Method, Mater. Sci.-Pol., 2018, 36(3), 387–396, DOI:10.1515/msp-2018-0029.
- B. H. Zhang, X. Yu, T. Wang, S. Cheng, J. B. Qiu and X. H. Xu, Photostimulated and Long Persistent Luminescence Properties from Different Crystallographic Sites of β-Sr2SiO4:Eu2+,R3+ (R = Tm, Gd), J. Am. Ceram. Soc., 2015, 98(1), 171–177, DOI:10.1111/jace.13272.
- K. Asami, J. Ueda, K. Yasuda, K. Hongo, R. Maezono, M. G. Brik and S. Tanabe, Development of Persistent Phosphor of Eu2+ Doped Ba2SiO4 by Er3+ Codoping Based on Vacuum Referred Binding Energy Diagram, Opt. Mater., 2018, 84, 436–441, DOI:10.1016/j.optmat.2018.07.021.
- X. Y. Sun, J. H. Zhang, X. Zhang, Y. S. Luo and X.-J. Wang, Long Lasting Yellow Phosphorescence and Photostimulated Luminescence in Sr3SiO5:Eu2+ and Sr3SiO5:Eu2+,Dy3+ Phosphors, J. Phys. D: Appl. Phys., 2008, 41(19), 195414, DOI:10.1088/0022-3727/41/19/195414.
- Z. Z. Wang, Z. Song, L. X. Ning and Q. L. Liu, Sunlight-Activated Yellow Long Persistent Luminescence from Nb-Doped Sr3SiO5:Eu2+ for Warm-Color Mark Applications, J. Mater. Chem. C, 2020, 8(3), 1143–1150, 10.1039/C9TC05880J.
- Z. Z. Wang, Z. Song, L. X. Ning, Z. G. Xia and Q. L. Liu, Enhanced Yellow Persistent Luminescence in Sr3SiO5:Eu2+ through Ge Incorporation, Inorg. Chem., 2019, 58(13), 8694–8701, DOI:10.1021/acs.inorgchem.9b01020.
- Z. Z. Wang, Z. Song and Q. L. Liu, Orange Super-Long Persistent Luminescent Materials: (Sr1−xBax)3SiO5:Eu2+,Nb5+, Mater. Chem. Front., 2021, 5(1), 333–340, 10.1039/D0QM00488J.
- T. Schlieper, W. Nitrido-Silicate Schnick and I. Hochtemperatur-Synthese, und Kristallstruktur von Ca2Si5N8, Z. Anorg. Allg. Chem., 1995, 621(6), 1037–1041, DOI:10.1002/zaac.19956210624.
- T. Schlieper and W. Milius, Schnick, W. Nitrido-silicate. II [1]. Hochtemperatur-Synthesen und Kristallstrukturen von Sr2Si5N8 und Ba2Si5N8, Z. Anorg. Allg. Chem., 1995, 621(8), 1380–1384, DOI:10.1002/zaac.19956210817.
-
J. W. H. van Krevel, PhD thesis, Eindhoven University of Technology, Eindhoven, Netherlands, 2000.
- X. Q. Piao, T. Horikawa, H. Hanzawa and K. Machida, Characterization and Luminescence Properties of Sr2Si5N8:Eu2+ Phosphor for White Light-Emitting-Diode Illumination, Appl. Phys. Lett., 2006, 88(16), 161908, DOI:10.1063/1.2196064.
- O. M. Ten Kate, Z. Zhang and H. T. (Bert) Hintzen, On the Relations between the Bandgap, Structure and Composition of the M–Si–N (M = Alkali, Alkaline Earth or Rare-Earth Metal) Nitridosilicates, J. Mater. Chem. C, 2017, 5(44), 11504–11514, 10.1039/C7TC04259K.
- J. W. H. van Krevel, H. T. Hintzen, R. Metselaar, L. Le Gendre and R. Marchand, Oxidation Resistance of Ln–Si–O-N Powders (Ln=Y, Gd and La), Solid State Sci., 2001, 3(1–2), 49–56, DOI:10.1016/S1293-2558(00)01120-1.
- Y. Zhao, L. Yin, O. M. ten Kate, B. Dierre, R. Abellon, R.-J. Xie, J. R. van Ommen and H. T. Hintzen, Enhanced Thermal Degradation Stability of the Sr2Si5N8:Eu2+ Phosphor by Ultra-Thin Al2O3 Coating through the Atomic Layer Deposition Technique in a Fluidized Bed Reactor., J. Mater. Chem. C, 2019, 7(19), 5772–5781, 10.1039/C9TC01063G.
- C.-W. Yeh, W.-T. Chen, R.-S. Liu, S.-F. Hu, H.-S. Sheu, J.-M. Chen and H. T. Hintzen, Origin of Thermal Degradation of Sr2–xSi5N8:Eux Phosphors in Air for Light-Emitting Diodes, J. Am. Chem. Soc., 2012, 134(34), 14108–14117, DOI:10.1021/ja304754b.
- K. Van den Eeckhout, P. F. Smet and D. Poelman, Persistent Luminescence in Rare-Earth Codoped, J. Lumin., 2009, 129(10), 1140–1143, DOI:10.1016/j.jlumin.2009.05.007.
- H. R. Zhang, H. W. Dong, B. F. Lei, P. Wang, J. Li, Y. L. Liu, J. Wang, Y. Xiao, M. T. Zheng and J. X. Meng, Enhanced Performance of Ca2Si5N8:Eu2+,Tm3+ Reddish-Orange Afterglow Phosphor by Co-Doping with Dy3+, Opt. Mater., 2014, 36(11), 1846–1849, DOI:10.1016/j.optmat.2014.02.029.
- T. Maldiney, G. Sraiki, B. Viana, D. Gourier, C. Richard, D. Scherman, M. Bessodes, K. Van den Eeckhout, D. Poelman and P. F. Smet, In Vivo Optical Imaging with Rare Earth Doped Ca2Si5N8 Persistent Luminescence Nanoparticles, Opt. Mater. Express, 2012, 2(3), 261, DOI:10.1364/OME.2.000261.
- F. Du, W. D. Zhuang, R. H. Liu, Y. H. Liu, W. Gao, X. Zhang, Y. Xue and H. Hao, Synthesis, Structure and Luminescent Properties of Yellow Phosphor La3Si6N11:Ce3+ for High Power White-LEDs, J. Rare Earths, 2017, 35(11), 1059–1064 CrossRef CAS.
- M. Woike and W. Jeitschko, Preparation and Crystal Structure of the Nitridosilicates Ln3Si6N11 (Ln = La, Ce, Pr, Nd, Sm) and LnSi3N5 (Ln = Ce, Pr, Nd), Inorg. Chem., 1995, 34(21), 5105–5108 CrossRef CAS.
- C. M. Fang, H. T. Hintzen, R. A. de Groot and G. de With, First-Principles Electronic Structure Calculations of Ba5Si2N6 with Anomalous Si2N6 Dimeric Units, J. Alloys Compd., 2001, 322(1–2), L1–L4, DOI:10.1016/S0925-8388(01)01204-X.
- C. M. Fang, H. T. Hintzen and G. de With, First-Principles Electronic Structure Calculations of BaSi7N10 with Both Corner- and Edge-Sharing SiN4 Tetrahedra, J. Alloys Compd., 2002, 336(1–2), 1–4, DOI:10.1016/S0925-8388(01)01866-7.
- C. M. Fang, Y. Q. Li, H. T. Hintzen and G. de With, Crystal and Electronic Structure of the Novel Nitrides MYSi4N7 (M = Sr, Ba) with Peculiar NSi4 Coordination, J. Mater. Chem., 2003, 13(6), 1480, 10.1039/b211813k.
- C. M. Fang, H. T. Hintzen, G. With and R. A. de Groot, Electronic Structure of the Alkaline-Earth Silicon Nitrides M2Si5N8 (M = Ca and Sr) Obtained from First-Principles Calculations and Optical Reflectance Spectra, J. Phys.: Condens. Matter, 2001, 13(1), 67–76, DOI:10.1088/0953-8984/13/1/307.
- O. M. Ten Kate, Z. Zhang, J. R. van Ommen and H. T. (Bert) Hintzen, Dependence of the Photoluminescence Properties of Eu2+ Doped M–Si–N (M = Alkali, Alkaline Earth or Rare Earth Metal) Nitridosilicates on Their Structure and Composition, J. Mater. Chem. C, 2018, 6(21), 5671–5683, 10.1039/C8TC00885J.
- F. Du, W. D. Zhuang, R. Liu, Y. H. Liu, J. Y. Zhong, W. Gao, K. Chen, L. Chen, K. Kato and K. Lin, Effect of Y3+ on the Local Structure and Luminescent Properties of La3−xYxSi6N11:Ce3+ Phosphors for High Power LED Lighting, RSC Adv., 2016, 6(80), 77059–77065 RSC.
- Y. Jia, A. Miglio, S. Poncé, X. Gonze and M. Mikami, First-Principles Study of Ce3+ -Doped Lanthanum Silicate Nitride Phosphors: Neutral Excitation, Stokes Shift, and Luminescent Center Identification, Phys. Rev. B, 2016, 93(15), 155111 CrossRef.
- W. B. Park, T. H. Kwon and K.-S. Sohn, Decay Behavior in Ce3+-Doped La3Si6N11 and Lu3Al5O12 Phosphors, J. Am. Ceram. Soc., 2015, 98(2), 490–494 CrossRef CAS.
- J. Y. Zhong, W. R. Zhao, F. Du, J. Wen, W. D. Zhuang, R. H. Liu, C.-K. Duan, L. G. Wang and K. Lin, Identifying the Emission Centers and Probing the Mechanism for Highly Efficient and Thermally Stable Luminescence in the La3Si6N11: Ce3+ Phosphor, J. Phys. Chem. C, 2018, 122(14), 7849–7858 CrossRef CAS.
- F. Du, W. D. Zhuang, R. H. Liu, J. Zhong, Y. H. Liu, Y. S. Hu, W. Gao, X. Zhang, L. Chen and K. Lin, Site Occupancy and Photoluminescence Tuning of La3Si6−xAlxN11−x/3:Ce3+ Phosphors for High Power White Light-Emitting Diodes, CrystEngComm, 2017, 19(21), 2836–2843 RSC.
- Z. H. Chen, Q. H. Zhang, Y. G. Li, H. Z. Wang and R.-J. Xie, A Promising Orange-Yellow-Emitting Phosphor for High Power Warm-Light White LEDs: Pure-Phase Synthesis and Photoluminescence Properties, J. Alloys Compd., 2017, 715, 184–191 CrossRef CAS.
- T. Suehiro, N. Hirosaki and R.-J. Xie, Synthesis and Photoluminescent Properties of (La,Ca)3Si6N11: Ce3+ Fine Powder Phosphors for Solid-State Lighting, ACS Appl. Mater. Interfaces, 2011, 3(3), 811–816 CrossRef CAS PubMed.
- M. Nitta, N. Nagao, Y. Nomura, T. Hirasawa, Y. Sakai, T. Ogata, M. Azuma, S. Torii, T. Ishigaki and Y. Inada, High-Brightness Red-Emitting Phosphor La3(Si,Al)6(O,N)11: Ce 3+ for Next-Generation Solid-State Light Sources, ACS Appl. Mater. Interfaces, 2020, 12(28), 31652–31658, DOI:10.1021/acsami.0c09342.
- Z. Song and Q. L. Liu, Understanding the Abnormal Lack of Spectral Shift with Cation Substitution in Highly Efficient Phosphor La3Si6N11:Ce3+, Phys. Chem. Chem. Phys., 2020, 22(25), 14162–14168, 10.1039/D0CP01445A.
- P. Pust, A. S. Wochnik, E. Baumann, P. J. Schmidt, D. Wiechert, C. Scheu and W. Schnick, Ca[LiAl3N4]:Eu2+ —A Narrow-Band Red-Emitting Nitridolithoaluminate, Chem. Mater., 2014, 26(11), 3544–3549, DOI:10.1021/cm501162n.
- P. Pust, F. Hintze, C. Hecht, V. Weiler, A. Locher, D. Zitnanska, S. Harm, D. Wiechert, P. J. Schmidt and W. Schnick, Group (III) Nitrides M[Mg2Al2N4] (M = Ca, Sr, Ba, Eu) and Ba[Mg2Ga2N4 ]—Structural Relation and Nontypical Luminescence Properties of Eu2+ Doped Samples, Chem. Mater., 2014, 26(21), 6113–6119, DOI:10.1021/cm502280p.
- S. Schmiechen, H. Schneider, P. Wagatha, C. Hecht, P. J. Schmidt and W. Schnick, Toward New Phosphors for Application in Illumination-Grade White Pc-LEDs: The Nitridomagnesosilicates Ca[Mg3SiN4]:Ce3+, Sr[Mg3SiN4]:Eu2+, and Eu[Mg3SiN4], Chem. Mater., 2014, 26(8), 2712–2719, DOI:10.1021/cm500610v.
- D. Cui, Q. Xiang, Z. Song, Z. Xia and Q. Liu, The Synthesis of Narrow-Band Red-Emitting SrLiAl3N4:Eu2+ Phosphor and Improvement of Its Luminescence Properties, J. Mater. Chem. C, 2016, 4(30), 7332–7338, 10.1039/C6TC02093C.
- M.-H. Fang, Y.-T. Tsai, H.-S. Sheu, J.-F. Lee and R.-S. Liu, Pressure-Controlled Synthesis of High-Performance SrLiAl3N4:Eu2+ Narrow-Band Red Phosphors, J. Mater. Chem. C, 2018, 6(38), 10174–10178, 10.1039/C8TC03025A.
- T. M. Tolhurst, T. D. Boyko, P. Pust, N. W. Johnson, W. Schnick and A. Moewes, Investigations of the Electronic Structure and Bandgap of the Next-Generation LED-Phosphor Sr[LiAl3N4]:Eu2+-Experiment and Calculations, Adv. Opt. Mater., 2015, 3(4), 546–550, DOI:10.1002/adom.201400558.
- S. Zhang, Z. Song, S. Wang, Z. Wang, F. Wang and Q. Liu, Red Persistent and Photostimulable Phosphor SrLiAl3N4:Eu2+, J. Mater. Chem. C, 2020, 8(14), 4956–4964, 10.1039/D0TC00277A.
-
M. Seibald, D. Baumann, T. Fiedler, S. Lange, H. Huppertz, D. Dutzler, T. Schroder, D. Bichler, G. Plundrich, S. Peschke, H. Gregor, G. Achrainer and K. Wurst, Lighting Devices Using Phosphors and Their Use as Backlights, WO 2018/029304 A1, 2018 Search PubMed.
- D. Dutzler, M. Seibald, D. Baumann and H. Huppertz, Alkali Lithosilicates: Renaissance of a Reputable Substance Class with Surprising Luminescence Properties, Angew. Chem., Int. Ed., 2018, 57(41), 13676–13680, DOI:10.1002/anie.201808332.
- M. Zhao, H. Liao, L. Ning, Q. Zhang, Q. Liu and Z. Xia, Next-Generation Narrow-Band Green-Emitting RbLi(Li3SiO4)2:Eu2+ Phosphor for Backlight Display Application, Adv. Mater., 2018, 30(38), 1802489, DOI:10.1002/adma.201802489.
- H. Liao, M. Zhao, Y. Zhou, M. S. Molokeev, Q. Liu, Q. Zhang and Z. Xia, Polyhedron Transformation toward Stable Narrow-Band Green Phosphors for Wide-Color-Gamut Liquid Crystal Display, Adv. Funct. Mater., 2019, 7 Search PubMed.
- H. Liao, M. Zhao, M. S. Molokeev, Q. Liu and Z. Xia, Learning from a Mineral Structure toward an Ultra-Narrow-Band Blue-Emitting Silicate Phosphor RbNa3(Li3SiO4)4:Eu2+, Angew. Chem., 2018, 130(36), 11902–11905, DOI:10.1002/ange.201807087.
- M. Zhao, H. Liao, M. S. Molokeev, Y. Zhou, Q. Zhang, Q. Liu and Z. Xia, Emerging Ultra-Narrow-Band Cyan-Emitting Phosphor for White LEDs with Enhanced Color Rendition, Light: Sci. Appl., 2019, 8(1), 38, DOI:10.1038/s41377-019-0148-8.
- M. Zhao, K. Cao, M. Liu, J. Zhang, R. Chen, Q. Zhang and Z. Xia, Dual-Shelled RbLi(Li3SiO4)2:Eu2+@Al2O3@ODTMS Phosphor as a Stable Green Emitter for High-Power LED Backlights, Angew. Chem., Int. Ed., 2020, 6 Search PubMed.
- H. Zhong, J.-W. Wang, B.-B. Dong, L. Li, M.-Y. Yang, J.-L. Yu, X. Xin and S. Agathopoulos, A Simple Way to Prepare a Hydrophobic Sr[LiAl3N4]:Eu2+Phosphor with Improved Moisture Resistance, Mater. Res. Bull., 2018, 105, 260–264, DOI:10.1016/j.materresbull.2018.05.009.
- H. A. Höppe, F. Stadler, O. Oeckler and W. Schnick, Ca[Si2O2N2]-A Novel Layer Silicate, Angew. Chem., Int. Ed., 2004, 43(41), 5540–5542, DOI:10.1002/anie.200460098.
- O. Oeckler, F. Stadler, T. Rosenthal and W. Schnick, Real Structure of SrSi2O2N2, Solid State Sci., 2007, 9(2), 205–212, DOI:10.1016/j.solidstatesciences.2006.11.009.
- J. A. Kechele, O. Oeckler, F. Stadler and W. Schnick, Structure Elucidation of BaSi2O2N2 – A Host Lattice for Rare-Earth Doped Luminescent Materials in Phosphor-Converted (Pc)-LEDs, Solid State Sci., 2009, 11(2), 537–543, DOI:10.1016/j.solidstatesciences.2008.06.014.
- Y. Q. Li, A. C. A. Delsing, G. de With and H. T. Hintzen, Luminescence Properties of Eu2+-Activated Alkaline-Earth Silicon-Oxynitride MSi2O2−δN2+2/3δ (M = Ca, Sr, Ba): A Promising Class of Novel LED Conversion Phosphors, Chem. Mater., 2005, 17(12), 3242–3248, DOI:10.1021/cm050175d.
- G. G. Li, C. C. Lin, W.-T. Chen, M. S. Molokeev, V. V. Atuchin, C.-Y. Chiang, W. Z. Zhou, C.-W. Wang, W.-H. Li, H.-S. Sheu, T.-S. Chan, C. Ma and R.-S. Liu, Photoluminescence Tuning via Cation Substitution in Oxonitridosilicate Phosphors: DFT Calculations, Different Site Occupations, and Luminescence Mechanisms, Chem. Mater., 2014, 26(9), 2991–3001, DOI:10.1021/cm500844v.
- H. R. Zhang, B. F. Lei, J. L. Qin, J. F. Li, Y. L. Liu, X. T. Liu, M. T. Zheng, Y. Xiao and H. W. Dong, Preparation of SrSi2O2N2:Eu2+ Phosphor by SrSi Alloy Precursor and Its Long-Lasting Phosphorescence Properties, J. Am. Ceram. Soc., 2013, 96(6), 1810–1814, DOI:10.1111/jace.12253.
- G. Z. Cao and R. Metselaar, α’-Sialon Ceramics: A Review, Chem. Mater., 1991, 3(2), 242–252, DOI:10.1021/cm00014a009.
- S. Hampshire, H. Park, D. Thompson and K. Jack, α′-Sialon Ceramics, Nature, 1978, 274(5674), 880–882 CrossRef CAS.
- J. Van Krevel, J. Van Rutten, H. Mandal, H. Hintzen and R. Metselaar, Luminescence Properties of Terbium-, Cerium-, or Europium-Doped α-Sialon Materials, J. Solid State Chem., 2002, 165(1), 19–24, DOI:10.1006/jssc.2001.9484.
- R.-J. Xie, M. Mitomo, K. Uheda, F.-F. Xu and Y. Akimune, Preparation and Luminescence Spectra of Calcium-and Rare-Earth (R= Eu, Tb, and Pr)-Codoped α-SiAlON Ceramics, J. Am. Ceram. Soc., 2002, 85(5), 1229–1234, DOI:10.1111/j.1151-2916.2002.tb00250.x.
- R.-J. Xie, N. Hirosaki, M. Mitomo, Y. Yamamoto, T. Suehiro and K. Sakuma, Optical Properties of Eu2+ in α-SiAlON, J. Phys. Chem. B, 2004, 108(32), 12027–12031 CrossRef CAS.
- Y. Li, N. Hirosaki, R. Xie, T. Takeda and M. Mitomo, Crystal and Electronic Structures, Luminescence Properties of Eu2+-Doped Si6−zAlzOzN8−z and MySi6−zAlz−yOz+yN8−z−y(M= 2Li, Mg, Ca, Sr, Ba), J. Solid State Chem., 2008, 181(12), 3200–3210 CrossRef CAS.
- J. J. Yang, Z. Song, L. Bian and Q. L. Liu, An Investigation of Crystal Chemistry and Luminescence Properties of Eu-Doped Pure-Nitride α--Sialon Fabricated by the Alloy-Nitridation Method, J. Lumin., 2012, 132(9), 2390–2397, DOI:10.1016/j.jlumin.2012.04.006.
- F. Wang, J. Guo, S. Wang, B. Qu, Z. Song, S. Zhang, W.-T. Geng and Q. Liu, Yellow Persistent Luminescence and Electronic Structure of Ca-α-Sialon: Eu2+, J. Alloys Compd., 2020, 821, 153482, DOI:10.1016/j.jallcom.2019.153482.
- J. Brgoch, M. W. Gaultois, M. Balasubramanian, K. Page, B.-C. Hong and R. Seshadri, Local Structure and Structural Rigidity of the Green Phosphor β-SiAlON:Eu2+, Appl. Phys. Lett., 2014, 105(18), 181904, DOI:10.1063/1.4901104.
- Y. Oyama and O. Kamigaito, Solid Solubility of Some Oxides in Si3N4, Jpn. J. Appl. Phys., 1971, 10(11), 1637, DOI:10.1143/JJAP.10.1637.
- N. Hirosaki, R.-J. Xie, K. Kimoto, T. Sekiguchi, Y. Yamamoto, T. Suehiro and M. Mitomo, Characterization and Properties of Green-Emitting β-SiAlON:Eu2+ Powder Phosphors for White Light-Emitting Diodes, Appl. Phys. Lett., 2005, 86(21), 211905, DOI:10.1063/1.1935027.
- R.-J. Xie, N. Hirosaki, H.-L. Li, Y. Q. Li and M. Mitomo, Synthesis and Photoluminescence Properties of β-Sialon:Eu2+ (Si6−zAlzOzN8−z:Eu2+), J. Electrochem. Soc., 2007, 154(10), J314, DOI:10.1149/1.2768289.
- Y. Q. Li, N. Hirosaki, R. J. Xie, T. Takeda and M. Mitomo, Crystal and Electronic Structures, Luminescence Properties of Eu2+-Doped Si6−zAlzOzN8−z and MySi6−zAlz−yOz+yN8−z−y (M=2Li, Mg, Ca, Sr, Ba, J. Solid State Chem., 2008, 181(12), 3200–3210, DOI:10.1016/j.jssc.2008.08.012.
- S. X. Li, L. Wang, D. M. Tang, Y. Cho, X. J. Liu, X. T. Zhou, L. Lu, L. Zhang, T. Takeda, N. Hirosaki and R.-J. Xie, Achieving High Quantum Efficiency Narrow-Band β-Sialon:Eu2+ Phosphors for High-Brightness LCD Backlights by Reducing the Eu3+ Luminescence Killer, Chem. Mater., 2018, 30(2), 494–505, DOI:10.1021/acs.chemmater.7b04605.
- Z. B. Wang, W. K. Ye, I.-H. Chu and S. P. Ong, Elucidating Structure–Composition–Property Relationships of the β-SiAlON:Eu2+ Phosphor, Chem. Mater., 2016, 28(23), 8622–8630, DOI:10.1021/acs.chemmater.6b03555.
- L.-C. Ju, X. Xu, L.-Y. Hao, Y. Lin and M.-H. Lee, Modification of the Coordination Environment of Eu2+ in Sr2SiO4:Eu2+ Phosphors to Achieve Full Color Emission, J. Mater. Chem. C, 2015, 3(7), 1567–1575, 10.1039/C4TC01435A.
- H. P. Grosse and E. Tillmanns, Bariumorthosilicate, Ba2SiO4, Cryst. Struct. Commun., 1974, 3(4), 599–602 CAS.
- M. Catti, G. Gazzoni, G. Ivaldi and G. Zanini, The β <-> α’ Phase Transition of Sr2SiO4. I. Order–Disorder in the Structure of the α’ Form at 383 K, Acta Crystallogr., Sect. B: Struct. Sci., 1983, 39(6), 674–679, DOI:10.1107/S0108768183003213.
- C. M. Midgley, The Crystal Structure of β Dicalcium Silicate, Acta Crystallogr., 1952, 5(3), 307–312, DOI:10.1107/S0365110X52000964.
- J. K. Park, M. A. Lim, C. H. Kim, H. D. Park, J. T. Park and S. Y. Choi, White Light-Emitting Diodes of GaN-Based Sr2SiO4:Eu and the Luminescent Properties, Appl. Phys. Lett., 2003, 82(5), 683–685, DOI:10.1063/1.1544055.
- J. S. Kim, Y. H. Park, S. M. Kim, J. C. Choi and H. L. Park, Temperature-Dependent Emission Spectra of M2SiO4:Eu2+ (M=Ca, Sr, Ba) Phosphors for Green and Greenish White LEDs, Solid State Commun., 2005, 133(7), 445–448, DOI:10.1016/j.ssc.2004.12.002.
- H. G. Jenkins and A. H. Mckeag, Some Rare Earth Activated Phosphors, J. Electrochem. Soc., 1950, 97(12), 415, DOI:10.1149/1.2777902.
- T. L. Barry, Fluorescence of Eu2+-Activated Phases in Binary Alkaline Earth Orthosilicate Systems, J. Electrochem. Soc., 1968, 115(11), 1181, DOI:10.1149/1.2410935.
- G. Blasse, W. L. Wanmaker, J. W. Ter Vrugt and A. Bril, Fluorescence of Eu2+-Activated Alkaline-Earth Aluminates, Philips Res. Rept., 1968, 23, 189 CAS.
- Y. Sato, H. Kuwahara, H. Kato, M. Kobayashi, T. Masaki and M. Kakihana, Large Redshifts in Emission and Excitation from Eu2+-Activated Sr2SiO4 and Ba2SiO4 Phosphors Induced by Controlling Eu2+ Occupancy on the Basis on Crystal-Site Engineering, Opt. Photonics J., 2015, 05(11), 326–333, DOI:10.4236/opj.2015.511031.
- K. Fukuda, M. Ito and T. Iwata, Crystal Structure and Structural Disorder of (Ba0.65Ca0.35)2SiO4, J. Solid State Chem., 2007, 180(8), 2305–2309, DOI:10.1016/j.jssc.2007.06.001.
- N. Lakshminarasimhan and U. V. Varadaraju, Luminescence and Afterglow in Sr2SiO4:Eu2+,RE3+ [RE=Ce, Nd, Sm and Dy] Phosphors—Role of Co-Dopants in Search for Afterglow, Mater. Res. Bull., 2008, 43(11), 2946–2953, DOI:10.1016/j.materresbull.2007.12.005.
- L. S. D. Glasser, Silicates M3SiO5. II. Relationships between Sr3SiO5, Cd3SiO5, and Ca3SiO5, Acta Crystallogr., 1965, 18(3), 455–457, DOI:10.1107/S0365110X65001007.
- L. S. D. Glasser and F. P. Glasser, Silicates M3SiO5. I. Sr3SiO5, Acta Crystallogr., 1965, 18(3), 453–454, DOI:10.1107/S0365110X65000993.
- J. K. Park, K. J. Choi, J. H. Yeon, S. J. Lee and C. H. Kim, Embodiment of the Warm White-Light-Emitting Diodes by Using a Ba2+ Codoped Sr3SiO5:Eu Phosphor, Appl. Phys. Lett., 2006, 88(4), 043511, DOI:10.1063/1.2166471.
- X. Y. Sun, J. H. Zhang, X. Zhang, Y. S. Luo, Z. D. Hao and X. Wang, Effect of Retrapping on Photostimulated Luminescence in Sr3SiO5:Eu2+,Dy3+ Phosphor, J. Appl. Phys., 2009, 105(1), 013501, DOI:10.1063/1.3050330.
- K. Dong, J. J. Liao, S. G. Xiao, X. L. Yang and J. W. Ding, Enhancement of Yellow Emission and Afterglow in Sr3SiO5:Eu2+,Dy3+ by Adding Alkaline Earth Metal Fluorides, J. Mater. Res., 2012, 27(19), 2535–2539, DOI:10.1557/jmr.2012.291.
- Y. Li, B. H. Li, C. C. Ni, S. X. Yuan, J. Wang, Q. Tang and Q. Su, Synthesis, Persistent Luminescence, and Thermoluminescence Properties of Yellow Sr3SiO5:Eu2+,RE3+ (RE=Ce, Nd, Dy, Ho, Er, Tm, Yb) and Orange-Red Sr3−xBaxSiO5:Eu2+,Dy3+ Phosphor, Chem. Asian J., 2014, 9(2), 494–499, DOI:10.1002/asia.201301045.
|
This journal is © The Royal Society of Chemistry 2023 |
Click here to see how this site uses Cookies. View our privacy policy here.