DOI:
10.1039/D3TA06113B
(Review Article)
J. Mater. Chem. A, 2023,
11, 26371-26392
A review on photochemical sensors for lithium ion detection: relationship between the structure and performance
Received
8th October 2023
, Accepted 16th November 2023
First published on 16th November 2023
Abstract
The application of lithium in portable electronic devices, medical field, catalysts, and so on has increased in recent years because of the unique properties of lithium. Therefore, detection and sensing of lithium ions are very important in chemical, environmental and biological processes. Sensors as high-rate detecting tools have been widely used for the detection of lithium ions under a variety of conditions. Among different kinds of sensors, photochemical sensors with easy control capabilities have been used as smart systems with rapid response to light as a physical stimulus. Photochemical sensors have attracted much attention due to their advantages such as real-time, fast, cost-effective, and optical detection. Photochromic and fluorescent compounds in contact with the stimulus, with changes in optical properties such as color or fluorescence intensity, can be used as optical sensors with a high detection speed and accuracy. Many fluorescent compounds have been used to prepare photochemical sensors of lithium such as spiropyran, nitrobenzoxazole, coumarin, porphyrin, etc. Herein, we provide an overview of the mechanisms of lithium ion adsorption and desorption by these optical sensors. We have discussed the performance of photochemical sensors considering their structural properties. Moreover, the issue of lithium ion storage is highlighted according to the chemical structure of the probes.
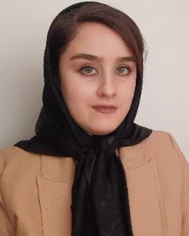 Fatemeh Javanbakht | Fatemeh Javanbakht received her BSc degree in Polymer Engineering from Tabriz Payame Noor University in 2016. She received her M.Sc. degree in Polymer Engineering from the Sahand University of Technology. She is currently a PhD student of Polymer Engineering at the Sahand University of Technology. She is working on Li-ion sensors. |
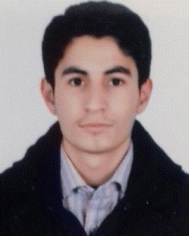 Hossein Najafi | Hossein Najafi received his bachelor's degree in Polymer Engineering from Urmia University in 2019. He started pursuing his master's degree in Polymer Engineering at the Sahand University of Technology in 2020. Since 2020, he has been working as a researcher in the field of smart polymers and sensors. |
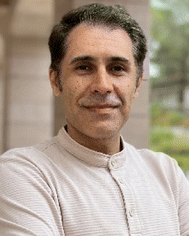 Kiyumars Jalili | Dr Kiyumars Jalili, PhD, is an Associate Professor at the Sahand University of Technology (SUT) and serves as the principal investigator-scientist at the Institute of Polymeric Materials (IPM). He established and directs the BioIntegrated and NanoAssembly Lab (JrG Lab) at IPM and is also the CEO of the Iranian Mana Polymer Inventors (MAPA) company. Dr Jalili's expertise lies in the design of composite polymeric materials with unique properties, primarily for applications in drug delivery, tissue engineering, and enhanced oil recovery, with a special focus on cancer theranostics and regenerative medicine. His research interests encompass various areas, including microfluidic-assisted self-assembly of polymers for drug delivery systems, regenerative medicine, BioMEMS, and neural implants. His recent work in synthetic chemistry focuses on replicating the mechanical properties of biological tissues using specialized molecular structures in synthetic polymers. |
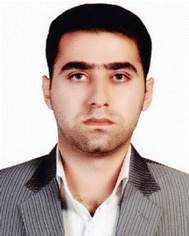 Mehdi Salami-Kalajahi | Mehdi Salami-Kalajahi is a professor of polymer engineering at the Sahand University of Technology (SUT). He received his BSc and MSc degrees in polymer engineering from the Amirkabir University of Technology (AUT). He was selected as a recognized PhD student by the National Elite Foundation in 2009–2011 and also Iran's recognized PhD student by the Ministry of Science in 2010. He was nominated as the entrepreneur student of Iran by the Association of Polymer and Chemical Engineers of Iran in 2010. He was selected as the recognized researcher at SUT in 2018–2023 and recognized engineering researcher at East Azerbaijan province in 2021. He was selected as the outstanding young researcher in chemical engineering by the Iran Academy of Sciences in 2022. His research interest is in the area of polymer chemistry, sensors, and polymer-based storage devices. |
1. Introduction to photochromic compounds and fluorescent sensors
Lithium cations (Li+) have a small ionic diameter and a charge density of (1.47 q. Å−1), and are coordinated by electron donor nitrogen and oxygen atoms.1,2 The specific physical, chemical, and electrochemical properties of lithium and its compounds have made it attractive in many fields. For example, lithium-ion batteries have received great attention due to their unique properties such as high energy capacity, light weight, fast charging, low self-discharge, and good chemical stability.3–5 On the other hand, lithium-based compounds have been used more widely in clinical applications.6–8 They are used in a common treatment for psychosis9,10 and bipolar disorders,11,12 skin diseases,13 and Alzheimer's disease.14,15
Lithium is very reactive and does not exist in nature in its pure form. Primary sources for lithium extraction are obtained from spodumene ores16 and some salt lakes.17,18 The increase in mining activity and the extraction of lithium from lithium mines in the last few decades has caused environmental destruction and endangered the health of wildlife and local populations.19,20 Thus, the detection of lithium in environmental samples is required to control each step of the extraction processes. In the applied form, excessive consumption of Li+ drugs causes harmful effects on the nervous system, brain, and liver.21,22 Therefore, due to side effects and toxicity, the development of suitable methods such as optical sensors with fast and reliable determination of Li+ ion concentration in biofluids such as blood and saliva is required. Moreover, lithium resources in the world are limited and non-renewable. This increases the price of lithium and faces serious problems in the development of different industries.23 Also, the process of extracting raw materials and producing technologies has created many problems and contamination of the environment.24 In such cases, the detection of lithium in environmental samples is required to fully control the recycling process of lithium-based products.25
In the last two decades, the use of sensors to improve the quality of human life has increased significantly. Scientists have always been trying to improve the quality of sensors in terms of their lifetime, sensitivity, and accuracy for chosen applications.26–28 There are many factors to consider when choosing a sensor. However, the selection of the sensor begins with the physical parameter to be measured. After determining the measurement parameter, several important factors such as the sensor performance range, accuracy level, and cost should be considered.29–31 Sensors can be classified from different perspectives such as the type of output signal, type of function, type of measured physical parameters, power source, technology used for their operation, type of building, and the installation location.31
Photochromic compounds are able to show the color change and fluorescence emission simultaneously between two states of OFF and ON in response to light in one place and time.32 When an interaction between the target analyte and the sensor element occurs, photometric sensors are used to instantly record this change in color and fluorescence intensity.33,34 Detection with the naked eye, short analysis time, low detection cost (cheapness), simplicity of the system, selectivity, reversibility, and no need for complex tools are the advantages of these sensors.35 The structure and isomerization of the most important and widely used photochromic compounds such as spiropyran, spirooxazine,36 azobenzene37 and diarylethene38 are shown in Fig. 1, which can be used in the design of optical detectors.
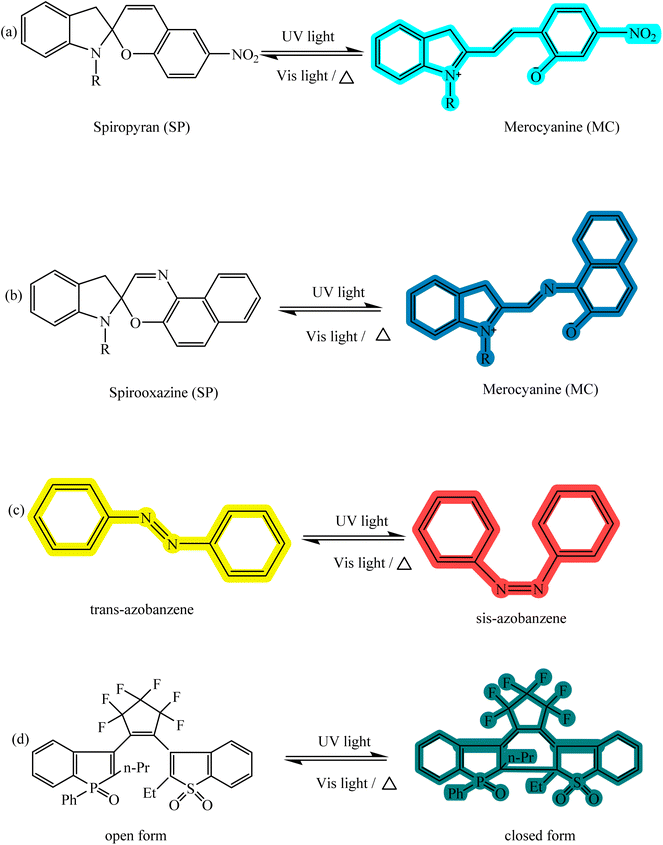 |
| Fig. 1 Chemical structure and response to the stimulus of (a) spiropyran, (b) spirooxazine, (c) azobenzene, and (d) diarylethene. | |
The important subject in optical sensors is the reversible, very fast, and sensitive response of these compounds to stimuli. Variation in functional groups affects the interaction of these compounds with their surroundings (including metal ions) in terms of rate and accuracy in detecting the analyte.39 The response of photochromic compounds in molecules before applying the stimulus is often observed in the form of a non-polar structure, unconjugated structure, and colorless form, and after induction of the external stimulus, it leads to the creation of a polar isomer, conjugated structure, and colored form. Isomerization between two states with different electron structures is done by bond breaking (ring opening) or bond formation (ring closing). According to the structure of photochromic compounds, these materials have various applications in drug delivery systems, colorimetric sensors, optical memories, and photo-patterning.40,41
Fluorescent compounds contain special chemical structures that immediately show fluorescence emission resulting from electron transfers between different energy levels after absorbing light radiation, and when the light source is cut off, fluorescence emission is also turned off due to the end of electron transfer.42 It should be noted that conjugated chemical structures (substituted with electron-withdrawing and electron-donating groups) with appropriate electron density and resonance are required to observe fluorescence emission. Fluorescence emission can be affected by a wide range of chemical or physical properties such as the length of the conjugated structure, the nature of substituted groups (electron donating or withdrawing power), intensity and wavelength of radiation, transparency or turbidity in colloidal solutions, size in the system colloids, concentration, and polarity of the surrounding environment (interaction between fluorescent molecules with solvent molecules or polar groups of the polymer matrix).43 Fluorescent compounds are classified into organic dyes,44 rare earth metal complexes,45 quantum dots,46 polymer dots,47 and supramolecular structures,48 and each of them has a potential application in the preparation of chemical sensors.
In recent years, the use of photochromic and fluorescent compounds in a single (unit) system has led to advanced materials that can be the beginning of new fields of study for the design and construction of chemical sensors. In fact, the use of compounds containing smart organic materials can be more economical and more accessible than complex polymer synthetic systems such as polymer dots, carbon dots,49 quantum dots, and in general nanoscale materials.50,51 Organic fluorescent compounds are considered as a significant group of fluorescent materials that have some special properties such as a conjugated chemical structure, electronic resonance, and electron-withdrawing or electron-donating groups in the form of substituted functional groups. Changing any of the mentioned factors can significantly affect the optical properties of the fluorescence compound, especially the fluorescence emission in solution. The fluorescence emission and its color are a function of the energy absorbed by the electrons and the transitions that take place between energy levels, all of which depend on electron resonance and conjugation. On the other hand, all the mentioned factors can be affected by intramolecular and intermolecular interactions of fluorescence compounds, solvent molecules, and also the surrounding environment. Therefore, a wide range of fluorescent compounds that emit different colors can be developed based on the chemical structure as well as the polarity of the environment, which have potential applications in technologies related to the preparation of fluorescence chemical sensors.51,52 Some of the most important fluorescent organic compounds including rhodamine,53 fluorescein,54 naphthalimide,55 boron dipyrromethene,56 perylene diimide,57 4-hydroxycoumarin58, nitrobenzoxadiazole,59 and pyrene60 are shown in Fig. 2. In recent years, studies have been conducted in the field of preparing chemical sensors using fluorescent compounds and investigating their effect on the performance or improvement of chemical detectors. Similar to photochromic compounds, these materials can be chemically or physically incorporated into the polymer substrates.
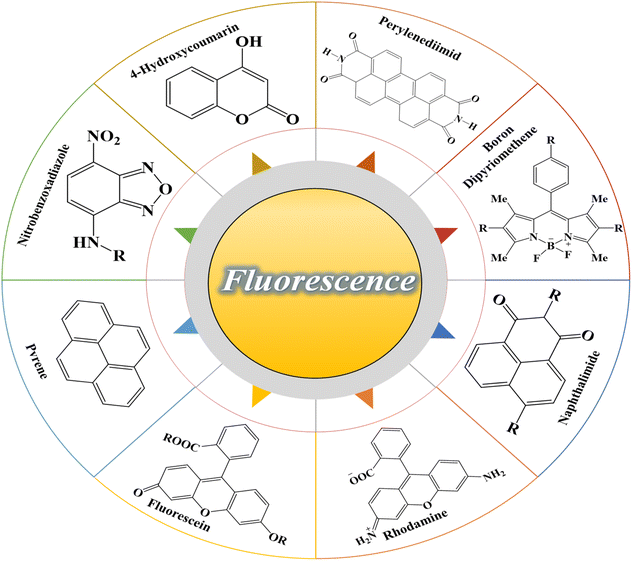 |
| Fig. 2 Chemical structure of the most important fluorescence compounds: rhodamine, fluorescein, naphthalimide, boron dipyrromethene, perylene diimide, 4-hydroxycoumarin, nitrobenzoxadiazole, and pyrene. | |
Fluorescent sensors have high accuracy and selectivity and are widely used in clinical diagnosis,61,62 food safety,63 and detection of heavy metal ions.64,65 Fluorescent sensors have been proven to be the most suitable option for the detection of metal ions.66 A fluorescent sensor consists of a fluorophore moiety and a guest acceptor in a spaced or integrated scaffold (Fig. 3). When the analyte binds to the guest acceptor, the photophysical properties of the sensor change through mechanisms such as photo-induced electron transfer (PET), intramolecular charge transfer (ICT), fluorescence resonance energy transfer (FRET), etc.67–69
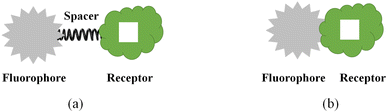 |
| Fig. 3 Fluorescent sensor model (a) with and (b) without a spacer. | |
Photochromic and fluorescence compounds that have been used as lithium ion sensors have different adsorption mechanisms in the presence of lithium ions. These sensors have shown different behaviors according to their chemical structures. In ongoing sections, different compounds with simultaneous abilities of fluorescence emission and lithium ion adsorption–desorption are surveyed.
2. Spiropyran-based chemical sensors
In recent years, studies have been conducted in the field of preparing optical sensors using fluorescent and photochromic compounds and examining them in terms of performance or improving chemical detectors. Spiropyran is one of the most widely used photochromic compounds, which has been welcomed by many researchers due to its quick response (in polar environments) to temperature,70 pH,71 and light.72 The change in the isomerization states of spiropyran is accompanied by the observation of a relatively broad partial peak in the range of 450–750 nm in the ultraviolet-visible (UV-vis) region.73 Before applying the desired stimulus, the structure is a non-polar, colorless, and closed spiro form. After applying the stimulus, the ring-opening isomerization reaction occurs with the breaking of the spiro bond between carbon and oxygen atoms, which turns into the highly polar and colored structure of merocyanine (MC).74 For example, Kang et al.75 synthesized a fluorescent probe for lithium ions by using spiropyran derivatives as fluorophores and combining them with azo-12-crown ether as a lithium recognition site. Lithium ions caused isomerization of spiropyran from a colorless spiro form to a colored merocyanine, to release oxygen negative ions, which were combined with crown ether under the coordination effect. An increase in UV-vis absorption was observed between 450 and 600 nm. The limit of detection was calculated to be 4.67 μM. This system was used for biological imaging in living cells. The results of several other similar structures are given in Table 1. The mechanism of lithium ion adsorption and desorption in crown ether modified spiropyran sensors regardless of crown ether size is shown in Fig. 4. The important point is that spiropyran with the crown ether part in the presence of lithium ions with or without UV irradiation tends to isomerization to the merocyanine form.74,76,77 After adding Li+ ions, the crown ether part in the sensor structure chelates with Li+ ions to form the corresponding complex.21,75–79
Table 1 Spiropyran derivatives as lithium ion adsorbents
Structure |
Derivatives |
λ
em (nm) |
λ
ex (nm) |
LOD (μM) |
Application |
pH |
Solvent |
Concentration (μM) |
Temperature (°C) |
Ref. |
|
|
620 |
550 |
4.67 |
Medical |
7.4 |
PBS : CH3CN (1 : 1 v/v) |
0.250 |
— |
75
|
|
R
1 = 627 |
532 |
— |
Sensor |
— |
Acetonitrile |
0–500 |
25 |
21
|
R
2 = 647 |
R
3 = 637 |
|
630 |
532 |
— |
Sensor |
— |
Acetonitrile, H2O:acetonitrile |
0.1–1 |
— |
79
|
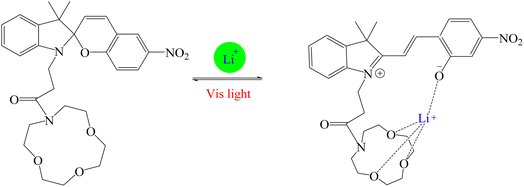 |
| Fig. 4 General mechanism in crown ether-modified spiropyran-based sensors (Reproduced with permission from ref. 75. Copyright 2023, Elsevier). | |
3. Nitrobenzoxazole-based chemical sensors
Qin and coworkers80 synthesized a special fluorescent compound for the detection of lithium ions based on 2-(2-hydroxyphenyl)benzoxazole (HPBO). Based on the coordination reaction between HPBO and lithium ions (Fig. 5), the fluorescence intensity increased dramatically with the addition of lithium ions, which is consistent with the lowest excited singlet changing from n–π* to π–π* and the increase in the rigidity of the molecule. The detection limit of lithium ions by this fluorophore was calculated to be 0.1 μM. In structures like 2-(2-hydroxyphenyl)-naphthoxazole (HPNO), the mechanism of lithium ion adsorption is the same.81,82
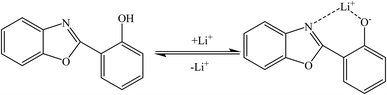 |
| Fig. 5 Behavior of HPBO in the presence of lithium ions (Reproduced with permission from ref. 81. Copyright 2023, Royal Society of Chemistry). | |
4. Coumarin-based chemical sensors
Kumari et al.83 synthesized a coumarin-indole dyad, N-((7-hydroxy-2-oxo-2H-chromen-4-yl)methyl)-1H-indole-2-carboxamide sensor. Lithium ions were added to the synthesized compound and the results showed the binding of lithium to amidic N–H and indolic N–H. The binding energy (BE) calculation showed that the binding of lithium ions to amidic N–H is more thermodynamically preferable than indolic N–H. When the coumarin-indole dyad sensor was coordinated with Li+ through the amide–N and indolic–N moieties, the fluorescence intensity increased due to the inhibition of PET. In the absence of Li+, the lone pair electrons on the amide–N in the coumarin ring were transferred. This led to the non-radiative decay of the excited state and the fluorescence was turned off.
5. Chemosensors with anhydride functional groups
Other common fluorescent compounds are perylenes. Perylene tetracarboxylic diimide/dianhydride (PDI or PTCDI/PTCDA) and their derivatives are suitable molecular structures for use in sensors to detect alkali metal cations, inorganic anions, etc.84 due to excellent optical and photochemical stability, high absorption of visible light, and fluorescence properties.85,86 PTCDA is a 5-ring aromatic structure with a perylene core and two anhydride groups.87 PTCDA was investigated as a model system to explain the mechanism of alkali metal storage by anhydride functional groups. It is reported that lithium can react with carbonyl oxygen and increase the electron density around the carboxylic oxygen (Fig. 6a). Therefore, the electron density of the perylene core increases and due to the interaction with lithium, features such as strong bond bending are observed.88,89 This mechanism is true for naphthalene tetracarboxylic dianhydride (NTCDA) (Fig. 6b), which has an anhydride functional group.89
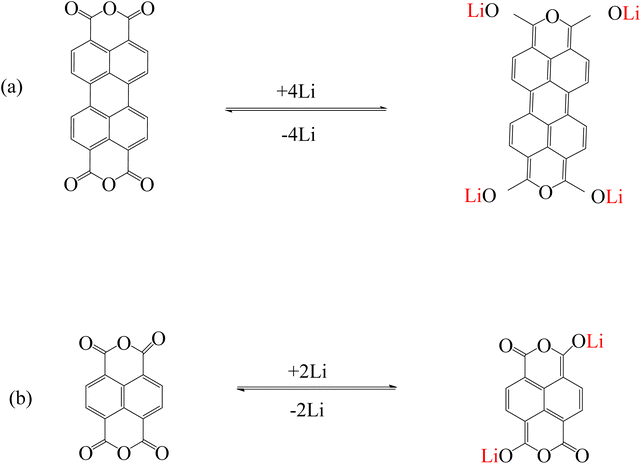 |
| Fig. 6 Mechanism of lithium ion adsorption by (a) PTCDA (Reproduced with permission from ref. 87. Copyright 2023, Elsevier) and (b) NTCDA (Reproduced with permission from ref. 89. Copyright 2023, Royal Society of Chemistry). | |
6. Chemosensors with imide functional groups
PTCDI was investigated as a model system to explain the mechanism of alkali metal adsorption by imide functional groups. After adding lithium, Li+ replaced the hydrogen atoms in the imide group and interacted with the imide nitrogen of PDI. Unlike PTCDA, Li+ showed a weak interaction with oxygen of the carbonyl group90–92 (Fig. 7a). Structures such as naphthalene diimide (Fig. 7b) and pyromellitic diimide (Fig. 7c) that have an imide functional group follow this mechanism.93,94 A number of similar structures are given in Tables 2 and 3.
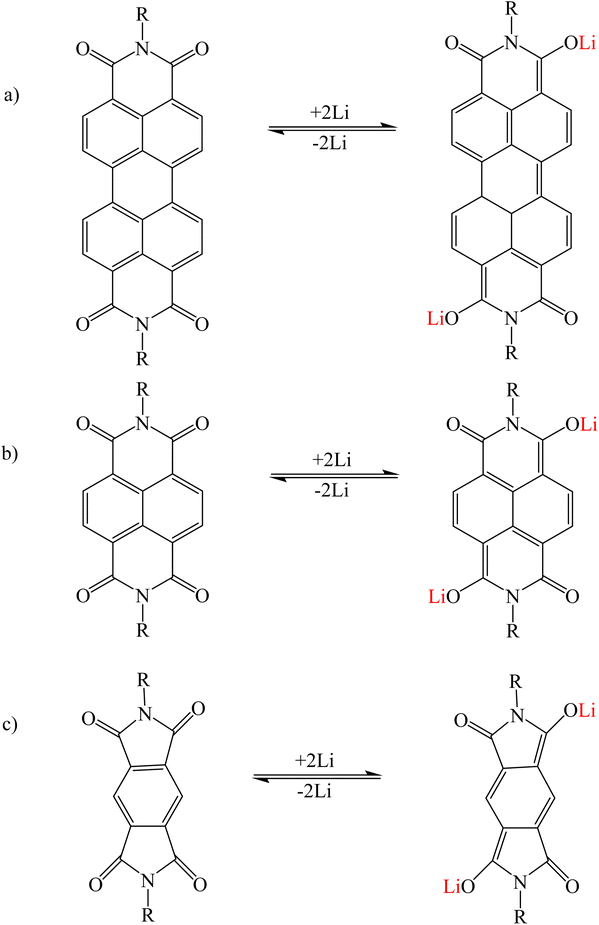 |
| Fig. 7 Mechanism of lithium ion adsorption by (a) PTCDI, (b) naphthalene diimide, and (c) pyromellitic diimide (Reproduced with open access permission from ref. 94. Copyright 2023, European Chemical Societies Publishing). | |
Table 2 Naphthalene diimide derivatives as lithium ion adsorbents
Structure |
|
Application |
Solvent |
Ref. |
|
|
Batteries |
N-Methyl-2-pyrrolidone (NMP) |
94
|
|
Batteries |
EC:DMC, DOL:DME |
92
|
R1 = Me |
R1 = H |
Batteries |
— |
91
|
R2 = H |
R2 = H |
Table 3 PTCDI derivatives as lithium ion adsorbents
Structure |
|
Application |
Solvent |
Temperature (°C) |
Ref. |
|
R1 = H |
Batteries |
— |
— |
90
|
|
Batteries |
DCM |
25 |
97
|
|
Batteries |
EC/DMC (1 : 1 molar ratio) |
— |
95
|
|
Batteries |
NMP |
— |
94
|
In multi-carbonyl perylene diimide derivatives, during lithiation/delithiation processes, the carbonyl groups in the perylene core and other carbonyl groups from the moieties connected to the imide and bay regions (such as 2,6-diaminoanthraquinone) are suitable places for Li+ ions storage. As a result, carbonyl groups (C
O) are converted into lithium enolate (C–O–Li) groups (Fig. 8a).95,96 In polycarbonyl naphthalene diimide derivatives, the mechanism of lithium adsorption is the same (Fig. 8b).92
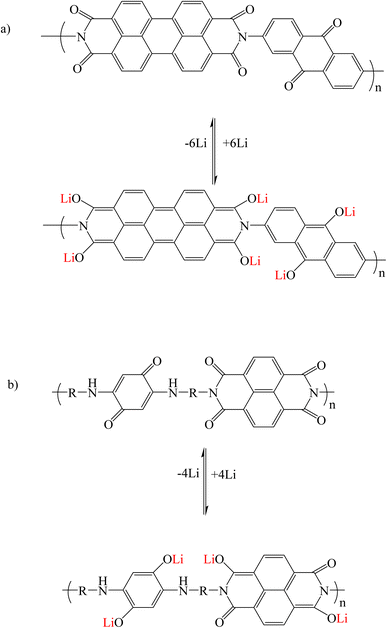 |
| Fig. 8 Mechanism of lithium ion adsorption by multi-carbonyl (a) perylene diimide and (b) naphthalene diimide derivatives (Reproduced with permission from ref. 95. Copyright 2023, Royal Society of Chemistry). | |
7. Cyclic 1,2-diketone-based chemical sensors
In order to investigate the lithiation/delithiation behavior of cyclic 1,2-diketones, pyrene-4,5,9,10-tetraone, which contains two 1,2-diketone units, was studied as a model system. By reduction of two electrons of a 1,2-diketone unit, two negatively charged oxygen atoms were created and then a carbon–carbon double bond was formed. These two oxygen atoms with negative charge are suitable active sites for coordination with Li+ ions. In fact, each carbonyl group (C
O) has the ability to adsorb one lithium ion (Fig. 9).98–102
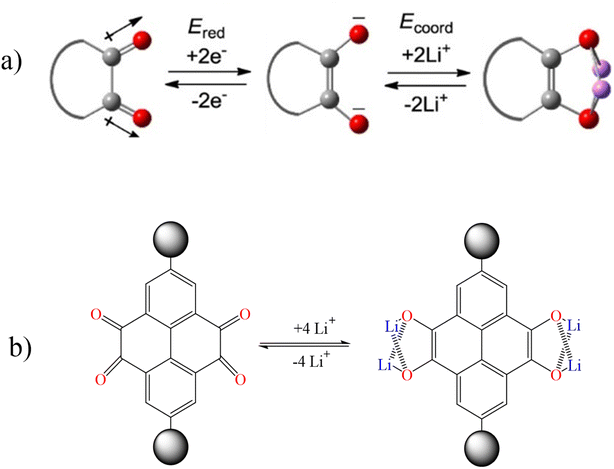 |
| Fig. 9 Lithiation/delithiation behavior of (a) cyclic 1,2-diketones and (b) pyrene-4,5,9,10-tetraone (Reproduced with permission from ref. 98. Copyright 2023, American Chemical Society). | |
8. BODIPY-based chemical sensors
In boron dipyrromethane (BODIPY)-based sensors, crown ether and aza crown ether are attached to BODIPY as ionophores. Two examples of these structures are given in Table 4. These sensors were designed based on the ICT process. After photoexcitation, electron transfer from the electron donor (amino group or oxygen) to the electron withdrawing group (BODIPY) took place under the ICT process. The interaction of the cation (Li+) with the electron donor (nitrogen atom of aza crown ether or oxygen atom of crown ether) inhibited the charge transfer (CT) process and caused a change in the fluorescence intensity. In this sensor, lithium ions caused spectral changes in the range 0–5 mM.56,103
Table 4 BODIPY derivatives as lithium ion adsorbents
Structure |
λ
em (nm) |
λ
ex (nm) |
Mechanism |
LOD (mM) |
Application |
pH |
Solvent |
Concentration (mM) |
Temperature (°C) |
Ref |
|
|
700 |
589 |
ICT |
0–5 |
Sensor |
— |
CH3CN |
0–5 |
25 |
103
|
|
— |
525 |
ICT |
0.1–100 |
Sensor |
7.4 |
Water |
0.1–100 |
— |
104
|
9. Porphyrin-based chemical sensors
In porphyrins, hydrogen atoms attached to the nitrogen in the porphyrazine ring are hydrolyzed by the solvent. Then, nitrogen atoms contain non-bonding electron pairs due to losing the attached hydrogen. When the lithium ion enters the ring, this pair of non-bonding electrons forms a dative bond with the lithium ion. Finally, a coordination structure is formed and nitrogen atoms act as ligands (Fig. 10).105–110
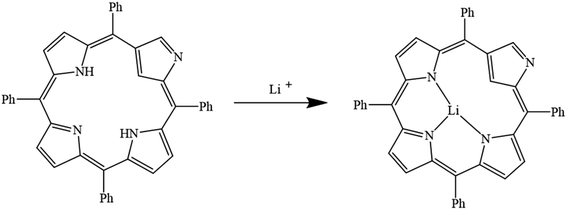 |
| Fig. 10 Mechanism of lithium ion adsorption by porphyrins (Reproduced with permission from ref. 109. Copyright 2023, Elsevier). | |
10. Calix[4]arene-based chemical sensors
Gulino et al. synthesized a (4-pyridylazo)-25,26,27-tris(ethoxycarbonylmethoxy)-28-hydroxycalix[4]arene immobilized monolayer (PyAzoC4-IM) (Fig. 11a). This optical chemosensor detected lithium ions at the ppm level (2.5–33.0 ppm) even in the presence of other cations (Fig. 11b). Among other cations, lithium ions were placed as a guest inside the calix[4] arene cavity.111,112
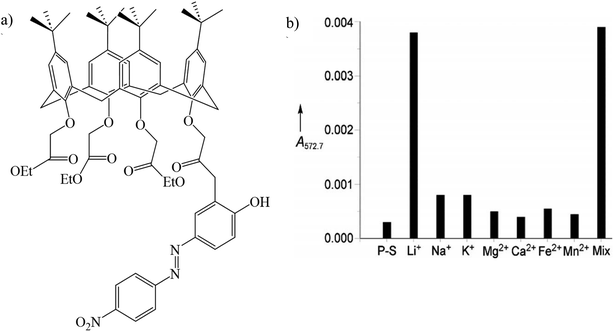 |
| Fig. 11 (a) Structure of PyAzoC4-IM and (b) absorbance of PyAzoC4-IM in the presence of various cations (Reproduced with permission from ref. 112. Copyright 2023, European Chemical Societies Publishing). | |
11. 1,10-Phenanthroline-based chemical sensors
Another type of photochemosensor for lithium ions is based on 1,10-phenanthroline derivatives. The groups at 2 and 9 positions affect coordination ability with lithium ions. These substitutions provided the possibility of selective coordination for lithium ions by forming a small cavity, where a strong interaction between nitrogen atoms and lithium ions was established (Fig. 12).113,114
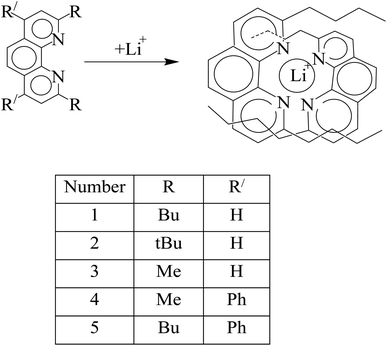 |
| Fig. 12 Mechanism of Li+ ion adsorption by 1,10-phenanthroline derivatives (Reproduced with permission from ref. 113. Copyright 2023, Royal Society of Chemistry). | |
12. Other photochemical sensors
Hangarge and coworkers93 synthesized the chemical sensor of aza-12-crown-4 ether-substituted naphthalene diimide (NDI-12-C-4). The aza-12-crown-4 moiety was used for selective binding of lithium ions (Li+). The fluorophore naphthalene diimide (NDI) has caused significant optical changes in the presence of monovalent lithium ions. UV-vis absorption, emission fluorescence, and naked eye-detection clearly confirmed the selective and sensitive binding of lithium ions. The detection limit for Li+ ions was calculated to be 5.0 μM. The increase in fluorescence emission indicated that in the absence of lithium ions, photoinduced electron transfer (PET) occurred from aza-crown to the NDI fluorophore, which resulted in fluorescence quenching. On the other hand, in the presence of Li+, the suppression of PET or in other words “PET-OFF” from aza-crown ether to NDI was occurred, which increased the fluorescence emission.93,115 Grabchev et al.116 synthesized a polyamidoamine dendrimer modified with 4-hexylamino-1,8-napthalimide as a fluorescent and photochromic sensor for the detection of lithium ions.117 In the alkaline media (in the presence of NaOH), deprotonation of the hexylamino groups at the C-4 position of the 1,8-naphthalimide structure was performed. Then, the lithium ion attacked the negative charge created in the nitrogen atom, which led to its neutralization and as a result the color changed from red to yellow and increased fluorescence intensity (Fig. 13). It is noteworthy that only in the alkaline medium (in the presence of NaOH) the dendrimer core was coordinated with the Li+ cations. The detection range of this sensor for Li+ ions was 1–30 μM. The increase in fluorescence intensity after this concentration was very negligible.
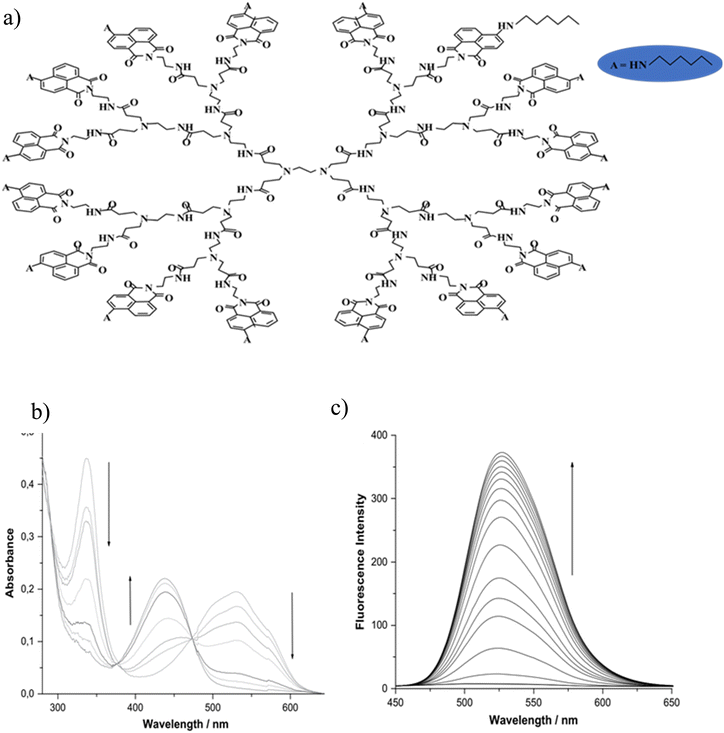 |
| Fig. 13 Mechanism of lithium ion adsorption by polyamidoamine dendrimer modified with 4-hexylamino-1,8-napthalimide (a), change of the absorption spectra (b), and fluorescence spectra (c) of dendrimer + NaOH upon addition of Li+ cations (C = 0–30 μM) (Reproduced with permission from ref. 116. Copyright 2023, Elsevier). | |
Li et al.118 synthesized two organic molecules 1,3,5-tri(9,10-anthraquinonyl)benzene (TAQB) (Fig. 14a) and 5,7,12,14-pentacenetetrone (PT) (Fig. 14b) as cathode electrodes used in lithium ion batteries. The adsorption mechanism of lithium ions in these two organic molecules was conducted by the coordination between lithium ions and the oxygen of the carbonyl group. During the redox reactions, the C
O bonds in TAQB and PT became the = C–O bonds of enolate groups. This process was repeated continuously during the charging and discharging cycle of the battery. The color change in both organic molecules was observed with the naked eye and the intensity of the absorption peaks in the UV-vis diagrams increased with the binding of lithium ions to the desired molecules.118
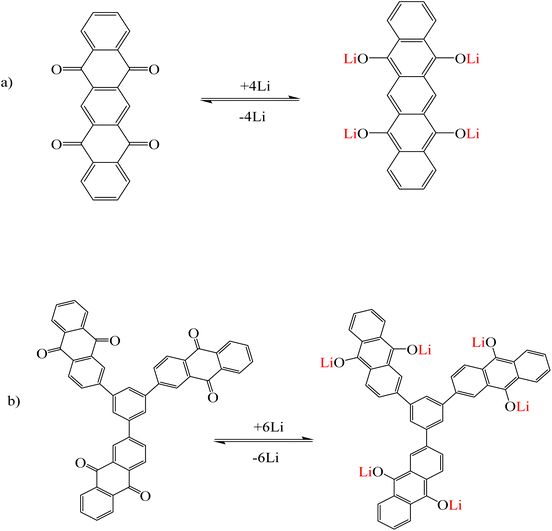 |
| Fig. 14 Mechanism of lithium ion adsorption by (a) PT and (b) TAQB (Reproduced with permission from ref. 118. Copyright 2023, Wiley). | |
Due to their small size and strong hydration in aqueous environments, lithium alkali metals exhibit weak coordination ability in aqueous environments.119 Also, common sensors for lithium ion detection, which are based on cryptands,120 crown ethers121 and other organic chromophores, show poor bonding properties in aqueous environments due to their insolubility in water.122 Therefore, they are only used in organic phases. Kaur and coworkers synthesized an optical sensor based on fluorescent organic nanoparticles. This sensor successfully detected lithium ions in aqueous environments. The synthesized sensor was based on the Biginelli compound which was made in the form of organic nanoparticles (ONPs) through a “bottom-up” technique. In binding Li+ ions with the receptor, the fluorescence intensity increased due to strong interactions between Li+ ions and ONPs. Li+ ions interacted with the nitro group of the 2-nitrophenyl moiety and by suppressing the PET, the fluorescence was switched on (Fig. 15). As the amount of Li+ increased, the fluorescence intensity increased linearly. The detection limit of this sensor for Li+ ions was calculated to be 122 nM.123
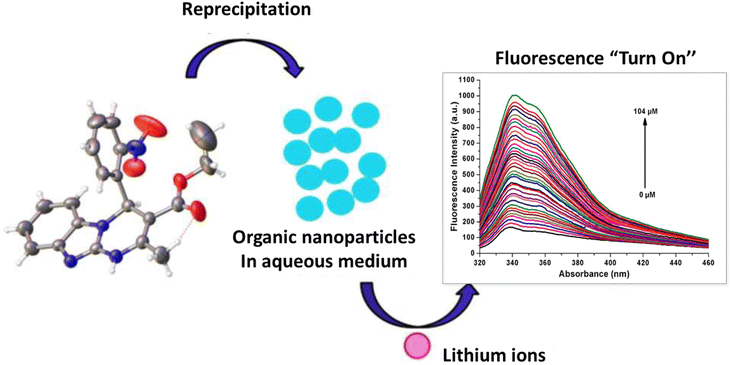 |
| Fig. 15 Mechanism of lithium ion adsorption by ONPs (Reproduced with permission from ref. 123. Copyright 2023, Royal Society of Chemistry). | |
Li et al. synthesized two stable bifunctional perylene imide radicals for use in organic lithium batteries in order to increase capacity and high energy efficiency. Perylene diimide (PDI) was modified from bay and imide positions and two compounds perylene-1-(4-hydroxy-2,6-di-tert-butylphenyl)-3,4:9,10-tricarboxylic-3,4:9,10-bis(2,6-diisopropylphenyl)imide (PTBI) and benzo[ghi]perylene-1-mono(4-hydroxyphenyl)-3,4,6,7,9,10-hexacarboxylic3,4:6,7:9,10-tri(2,6-diisopropylphenyl)imide (BPHI) were obtained and bifunctional radicals (PTBI)˙ and (BPHI)˙ were prepared through chemical/electrochemical reactions. The proposed mechanism for PTBI and BPHI is that first the pure phenoxyl radical (PTBI˙) was transformed into its lithium phenolate salt by taking a lithium ion and an electron. Then, the two carbonyl groups in the PDI skeleton are coordinated with lithium ions. In BPHI, in addition to these reactions, due to the presence of maleimide units and as a result two other active carbonyl groups in the bay position, another reaction was performed with lithium ion s(Fig. 16).124
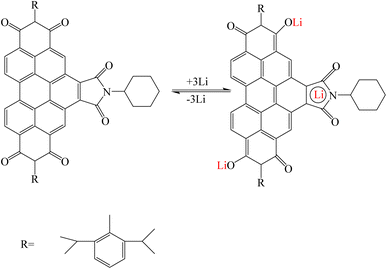 |
| Fig. 16 Mechanism of lithium ion adsorption by bifunctional perylene imide (Reproduced with permission from ref. 124. Copyright 2023, European Chemical Societies Publishing). | |
Tarazi et al.125 modified a heptamethine cyanine dye (IR-786) with crown ether (15-crown-5) (JCM-15C5) and investigated its spectral properties. By complexing lithium ions with the ionophore (crown ether) moiety of the dye, its photophysical properties changed. In fact, lithium ions decreased the fluorescence intensity of JCM-15C5. It was proved by Barzykin that Li+ ions formed complexes with benzocrown-5-ether moieties of the ligand.126 Intramolecular charge transfer (ICT) from the donor moiety (amino group) to the acceptor moiety (N+ of the pyridymerine ring) caused the fluorescence emission of JCM-15C5. The detection range of this sensor for Li+ ions was 7.43 × 10−2 ppb.125 Hiratani and coworkers127 synthesized rotaxane through covalent bond formation. In this compound, a small 3D cavity is created by the ring and chain connected to the macrocycle. This cavity had several ether oxygen atoms and amide groups. Lithium ions with the smallest ionic radius among other alkali metal ions were placed inside the formed 3D cavity as a guest species. Lithium ions reacted with polyether and carbonyl amide oxygen atoms and the fluorescence intensity increased greatly (Fig. 17).
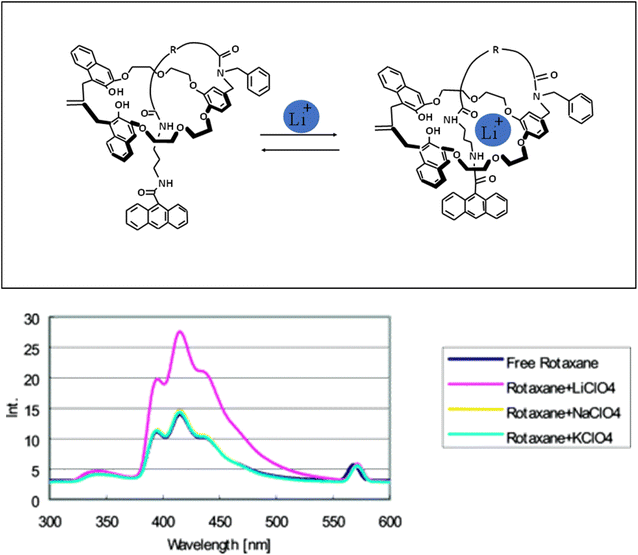 |
| Fig. 17 Behavior of rotaxane in the presence of lithium ions (Reproduced with permission from ref. 127. Copyright 2023, American Chemical Society). | |
Gunnlaugsson et al. synthesized a fluorescent chemosensor for lithium ion detection, which consists of moieties such as diaza-9-crown-3 as an acceptor, naphthalene as a fluorophore, and a chiral methyl substituted spacer. With Li+ ion complexation, protons in crown ether, α-CH2 (present in pendent arms), protons of chiral centers, and amide were also affected. Most likely, the lithium ion was placed in the lower part of the crown ether after binding, where the amide also participated in the binding.128 In optical chemosensors based on thiacalix[4] crown,129 salophen-UO2 homodimeric complex,130 and ruthenium-based metallacrown complexes,131 according to the position of the oxygen atom in the crown ether ring, two covalent bonds were observed by connecting to the surrounding atoms. However, it still has 2 pairs of non-bonding electrons. When Li+ entered this ring, due to higher electronegativity of the oxygen atom, it shared its non-bonding electron pair as a dative bond with Li+ (Fig. 18).
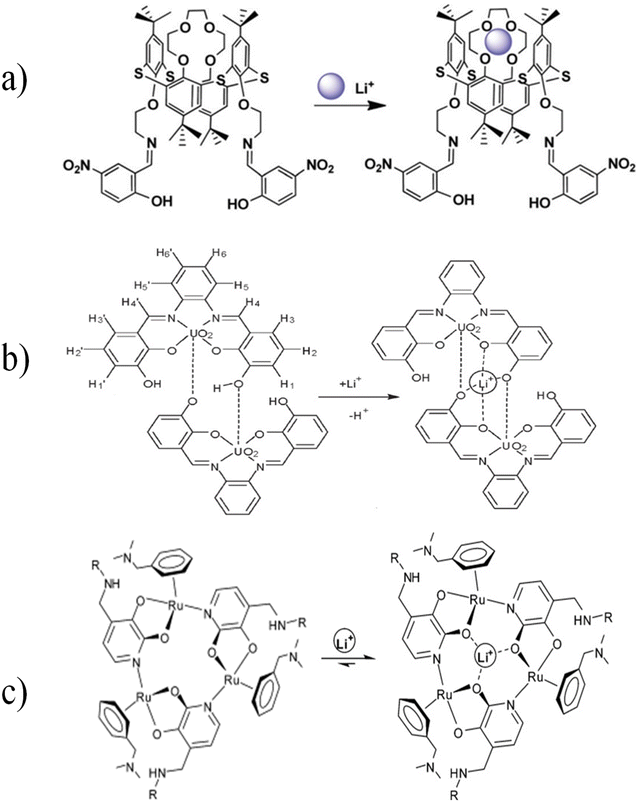 |
| Fig. 18 Mechanism of Li+ ion adsorption by (a) thiacalix[4]crown (Reproduced with permission from ref. 129. Copyright 2023, Royal Society of Chemistry), (b) salophen-UO2 homodimeric complex (Reproduced with permission from ref. 130. Copyright 2023, American Chemical Society), and (c) ruthenium-based metallacrown complex (Reproduced with permission from ref. 131. Copyright 2023, Royal Society of Chemistry). | |
In optical chemosensors based on derivatives of 14-crown-4 containing chromogens such as nitrophenol and azophenol, lithium ions were placed inside the crown ether cavity (Fig. 19). After the formation of the lithium ion complex with the nitrophenol crown, a color change from pale yellow to dark yellow was observed, and with the azophenol crown, a color change from yellow to orange was observed.132
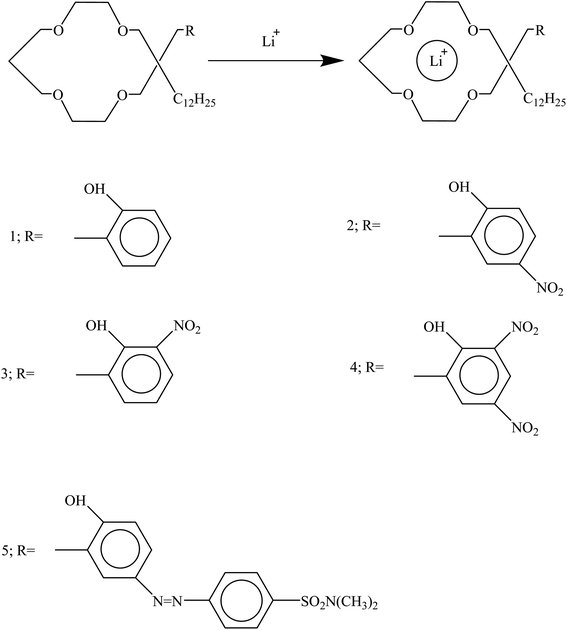 |
| Fig. 19 Mechanism of Li+ ion adsorption by derivatives of 14-crown-4 (Reproduced from ref. 132, Copyright 2023, Chemical Society of Japan). | |
Trautman et al. introduced the arsenic salt Thoron for the detection of lithium in blood serum. Lithium ions were coordinated with the nitrogen atom of the azobenzene group as well as the oxygen atoms of the phenolate and arsenate groups (Fig. 20), which led to a bathochromic shift at 480 nm.133
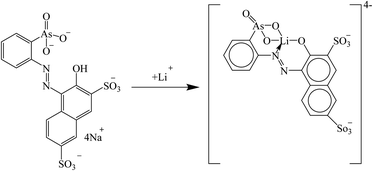 |
| Fig. 20 Mechanism of Li+ ion adsorption by the arsenic salt Thoron (Reproduced with permission from ref. 133. Copyright 2023, Elsevier). | |
13. Conclusions
Herein, photochemical sensors of lithium ion detection have been surveyed considering the effect of the chemical structure on the performance of sensors. A number of chemical sensors for lithium ion detection based on crown ethers and organic chromophores are surveyed. Crown ether derivatives containing a fluorescent moiety are attractive tools for optical sensing of metal ions. In the ionophore section of all chemical sensors, one of the two electron-donating atoms of oxygen and nitrogen is necessarily present, which has caused a change in the optical properties of the sensor through coordination with lithium ions. In the presence of both electron-donating atoms, the nitrogen atom has a greater tendency to coordinate with the lithium ion. The presence of nitrogen-containing functional groups such as amides, imides, amines, azo compounds, etc. or oxygenated functional groups such as aldehydes, ketones, quinones, esters, anhydrides, carbonyls, etc. makes suitable active sites for coordination with lithium ions. In conclusion, the use of lithium ion sensors for the detection of ion concentration in different systems makes it able to predict the system performance in the fields of application such as lithium ion batteries, medical and pharmaceutical applications, etc. This can be performed successfully using oxygen- and nitrogen-containing functional groups in the structure of sensors that help coordination of lithium ions with the sensor structure.
Abbreviations
(LiAlSi4O10) | Petalite |
(PET) | Photo-induced electron transfer |
(ICT) | Intramolecular charge transfer |
(FRET) | Fluorescence resonance energy transfer |
(SP) | Spiropyran |
(MC) | Merocyanine |
(UV-vis) | Ultraviolet-visible |
(HPBO) | 2-(2-Hydroxyphenyl)benzoxazole |
(HPNO) | 2-(2-Hydroxyphenyl)-naphthoxazole |
(BE) | Binding energy |
(PDI or PTCDI) | Perylene-3,4,9,10-tetracarboxylic diimide |
(PTCDA) | Perylene-3,4,9,10-tetracarboxylic dianhydride |
(NTCDA) | Naphthalenetetracarboxylic dianhydride |
(PMDA) | Pyromellitic dianhydride |
(NDI-12-C-4) | Aza-12-crown-4 ether-substituted naphthalene diimide |
(NDI) | Naphthalene diimide |
(TAQB) | 1,3,5-Tri(9,10-anthraquinonyl)benzene |
(PT) | 5,7,12,14-Pentacenetetrone |
(ONP) | Organic nanoparticles |
(PTBI) | Perylene-1-(4-hydroxy-2,6-di-tert-butylphenyl)-3,4:9,10-tricarboxylic-3,4:9,10-bis(2,6 diisopropylphenyl)imide |
(BPHI) | Benzo[ghi]perylene-1-mono(4-hydroxyphenyl)-3,4,6,7,9,10-hexacarboxylic3,4:6,7:9,10-tri(2,6-diisopropylphenyl)imide |
(IR-786) | Heptamethine cyanine dye |
(JCM-15C5) | (IR-786) modified with 15-crown-5 |
(BODIPY) | Boron dipyrromethane |
(CT) | Charge transfer |
(PyAzoC4-IM) | (4-Pyridylazo)-25,26,27-tris(ethoxycarbonylmethoxy)-28-hydroxycalix[4]arene immobilized monolayer |
Data availability
No data were used for the research described in the article.
Author contributions
Fatemeh Javanbakht: writing – original draft. Hossein Najafi: resources. Kiyumars Jalili: validation. Mehdi Salami-Kalajahi: conceptualization, validation, resources, writing – review & editing, visualization, and supervision.
Conflicts of interest
The authors declare that they have no conflict of interest.
Acknowledgements
This research did not receive any specific grant from funding agencies in the public, commercial, or not-for-profit sectors.
References
- Y. Marcus, Ionic radii in aqueous solutions, Chem. Rev., 1988, 88, 1475–1498, DOI:10.1021/cr00090a003.
- U. Olsher, R. M. Izatt and J. S. Bradshaw,
et al., Coordination chemistry of lithium ion: a crystal and molecular structure review, Chem. Rev., 1991, 91, 137–164, DOI:10.1021/cr00002a003.
- A. E. Gerdroodbar, H. Alihemmati, S.-A. Safavi-Mirmahaleh, M. Golshan, R. Damircheli, S. N. Eliseeva and M. Salami-Kalajahi, A review on ion transport pathways and coordination chemistry between ions and electrolytes in energy storage devices, J Energy Storage., 2023, 74, 109311, DOI:10.1016/j.est.2023.109311.
- A. E. Gerdroodbar, R. Damircheli, S. N. Eliseeva and M. Salami-Kalajahi, Janus structures in energy storage systems: advantages and challenges, J. Electroanal. Chem., 2023, 948, 117831, DOI:10.1016/j.jelechem.2023.117831.
- A. Enayati-Gerdroodbar, S. N. Eliseeva and M. Salami-Kalajahi, A review on the effect of nanoparticles/matrix interactions on the battery performance of composite polymer electrolytes, J Energy Storage., 2023, 68, 107836, DOI:10.1016/j.est.2023.107836.
- J. Mertens, Q.-W. Wang and Y. Kim,
et al., Differential responses to lithium in hyperexcitable neurons from patients with bipolar disorder, Nature, 2015, 527, 95–99, DOI:10.1038/nature15526.
- E. Y. Villegas-Vázquez, L. I. Quintas-Granados and H. Cortés,
et al., Lithium: A Promising Anticancer Agent, Life, 2023, 13, 537, DOI:10.3390/life13020537.
- G. Natale, E. Fini, P. F. Calabrò, M. Carli, M. Scarselli and G. Bocci, Valproate and lithium: Old drugs for new pharmacological approaches in brain tumors?, Cancer Lett., 2023, 216125, DOI:10.1016/j.canlet.2023.216125.
- P. E. Keck, S. L. McElroy and S. M. Strakowski,
et al., Antipsychotics in the treatment of mood disorders and risk of tardive dyskinesia, J. clin. psychiatry., 2000, 61, 33–38 CrossRef PubMed.
- C. Lennkh and C. Simhandl, Current aspects of valproate in bipolar disorder, Int. Clin. Psychopharmacol., 2000, 15, 1–11, DOI:10.1097/00004850-200015010-00001.
- R. Jope, Anti-bipolar therapy: mechanism of action of lithium, Mol. Psychiatry., 1999, 4, 117–128, DOI:10.1038/sj.mp.4000494.
- J. K. Rybakowski, Response to lithium in bipolar disorder: clinical and genetic findings, ACS Chem. Neurosci., 2014, 5, 413–421, DOI:10.1021/cn5000277.
- N. J. Birch, Inorganic pharmacology of lithium, Chem. Rev., 1999, 99, 2659–2682, DOI:10.1021/cr9804240.
- J. Zhong and W.-H. Lee, Lithium: a novel treatment for Alzheimer's disease?, Expert Opin. Drug Saf., 2007, 6, 375–383, DOI:10.1517/14740338.6.4.375.
- C. J. Phiel, C. A. Wilson and V. M.-Y. Lee,
et al., GSK-3α regulates production of Alzheimer's disease amyloid-β peptides, Nature, 2003, 423, 435–439, DOI:10.1038/nature01640.
-
K. Berman, J. Dziuba, C. Hamilton, et al., The Lithium Ion Battery and the EV Market: the Science behind what You Can't See. BMO Capital Markets Report. 2018 Search PubMed.
- S. E. Kesler, P. W. Gruber and P. A. Medina,
et al., Global lithium resources: Relative importance of pegmatite, brine and other deposits, Ore Geol. Rev., 2012, 48, 55–69, DOI:10.1016/j.oregeorev.2012.05.006.
- J. F. Song, L. D. Nghiem and X.-M. Li,
et al., Lithium extraction from Chinese salt-lake brines: opportunities, challenges, and future outlook, Environ. Sci.: Water Res. Technol., 2017, 3, 593–597, 10.1039/C7EW00020K.
-
T. G. Goonan, Lithium Use in Batteries, 2012, DOI:10.3133/cir1371.
- H. Ambrose and A. Kendall, Understanding the future of lithium: Part 2, temporally and spatially resolved life-cycle assessment modeling, J. Ind. Ecol., 2020, 24, 90–100, DOI:10.1111/jiec.12942.
- D. Stubing, S. Heng and A. Abell, Crowned spiropyran fluoroionophores with
a carboxyl moiety for the selective detection of lithium ions, Org. Biomol. Chem., 2016, 14, 3752–3757, 10.1039/C6OB00468G.
- A. Besekar and R. J. Immanuel, Detection of Lithium Carbonate: A Deadly Medicines and its Effects in Human Body, International Journal of Novel Research and Development, 2023, 8(8), e51–e85, DOI:10.1729/Journal.35942.
- J. R. Geddes and D. J. Miklowitz, Treatment of bipolar disorder, The lancet, 2013, 381, 1672–1682, DOI:10.1016/S0140-6736(13)60857-0.
-
M. Dry, Extraction of lithium from brine—old and new chemistry, Extraction, 2018: Proceedings of the First Global Conference on Extractive Metallurgy, Springer, 2018, DOI:10.1007/978-3-319-95022-8_187.
- L. Zhang, Z. Xu and Z. He, Electrochemical relithiation for direct regeneration of LiCoO2 materials from spent lithium-ion battery electrodes, ACS Sustain. Chem. Eng., 2020, 8, 11596–11605, DOI:10.1021/acssuschemeng.0c02854.
- A. Zardehi-Tabriz, Y. Ghayebzadeh, A. E. Gerdroodbar, M. Golshan, H. Roghani-Mamaqani and M. Salami-Kalajahi, Polyampholyte polymers-based sensors: a review on stimuli and applications, Macromol. Mater. Eng., 2023, 2300179, DOI:10.1002/mame.202300179.
- S.-A. Safavi-Mirmahalleh, M. Golshan, B. Gheitarani, M. S. Hosseini and M. Salami-Kalajahi, A review on applications of coumarin and its derivatives in preparation of photo-responsive polymers, Eur. Polym. J., 2023, 198, 112430, DOI:10.1016/j.eurpolymj.2023.112430.
- B. Gheitarani, M. Golshan, S.-A. Safavi-Mirmahalleh, M. Salami-Kalajahi, M. S. Hosseini and A. A. Alizadeh, Fluorescent polymeric sensors based on N-(rhodamine-6G) lactam-N'-allyl-ethylenediamine and 7-(allyloxy)-2H-chromen-2-one for Fe3+ ion detection, Colloids Surf., A, 2023, 656, 130473, DOI:10.1016/j.colsurfa.2022.130473.
- A. Nag, R. B. Simorangkir and D. R. Gawade,
et al., Graphene-based wearable temperature sensors: A review, Mater. Des., 2022, 110971, DOI:10.1016/j.matdes.2022.110971.
- J. R. Stetter, W. R. Penrose and S. Yao, Sensors, chemical sensors, electrochemical sensors, J. Electrochem. Soc., 2003, 150, S11, DOI:10.1149/1.1539051.
- R. Paolesse, S. Nardis and D. Monti,
et al., Porphyrinoids for chemical sensor applications, Chem. Rev., 2017, 117, 2517–2583, DOI:10.1021/acs.chemrev.6b00361.
- H. Jiang, S. Kelch and A. Lendlein, Polymers move in response to light, Adv. Mater., 2006, 18, 1471–1475, DOI:10.1002/adma.200502266.
- P. Shende, B. Prabhakar and A. Patil, Color changing sensors: A multimodal system for integrated screening, TrAC, Trends Anal. Chem., 2019, 121, 115687, DOI:10.1016/j.trac.2019.115687.
- A. P. VS, P. Joseph and K. D. SCG,
et al., Colorimetric sensors for rapid detection of various analytes, Mater. Sci. Eng. C., 2017, 78, 1231–1245, DOI:10.1016/j.msec.2017.05.018.
- Z. Gerdan, Y. Saylan and A. Denizli, Recent Advances of Optical Sensors for Copper Ion Detection, Micromachines, 2022, 13, 1298, DOI:10.3390/mi13081298.
- H. Xia, K. Xie and G. Zou, Advances in spiropyrans/spirooxazines and applications based on fluorescence resonance energy transfer (FRET) with fluorescent materials, Molecules, 2017, 22, 2236, DOI:10.3390/molecules22122236.
- L. Xue, Y. Pan and S. Zhang,
et al., Fluorescent azobenzene-containing compounds: from structure to mechanism, Crystals, 2021, 11, 840, DOI:10.3390/cryst11070840.
- T. Ichikawa, M. Morimoto and H. Sotome,
et al., Photochromism of diarylethene derivatives having benzophosphole and benzothiophene groups, Dyes Pigm., 2016, 126, 186–193, DOI:10.1016/j.dyepig.2015.11.023.
-
S. Oak, Mechanical Properties of PDMS and the Use of Hybrid Polymer-Grafted Carbon Microspheres as Stimuli-Responsive Lubricating Particles, Tulane University School of Science and Engineering, 2020 Search PubMed.
-
K. Ichimura, Photochromic polymers, Physicochemical Studies, Biological Applications, and Thermochromism, Organic Photochromic and Thermochromic Compounds, 2002, vol 2, pp. 9–63 Search PubMed.
- M. Golshan, B. Gheitarani, M. Salami-Kalajahi and M. S. Hosseini, Synthesis and characterization of fluorescence poly(amidoamine) dendrimer-based pigments, Sci. Rep., 2022, 12, 15180, DOI:10.1038/s41598-022-19712-5.
- T. Rasheed, M. Bilal and F. Nabeel,
et al., Fluorescent sensor based models for the detection of environmentally-related toxic heavy metals, Sci. Total Environ., 2018, 615, 476–485, DOI:10.1016/j.scitotenv.2017.09.126.
- D. Wu, A. C. Sedgwick and T. Gunnlaugsson,
et al., Fluorescent chemosensors: the past, present and future, Chem. Soc. Rev., 2017, 46, 7105–7123, 10.1039/C7CS00240H.
- N. Marmé, J.-P. Knemeyer and M. Sauer,
et al., Inter-and intramolecular fluorescence quenching of organic dyes by tryptophan, Bioconjug. Chem., 2003, 14, 1133–1139, DOI:10.1021/bc0341324.
- K. Krishna Rao, H.-G. Liu and Y.-I. Lee, Fluorescence spectroscopy of polymer systems doped with rare-earth metal ions and their complexes, Appl. Spectrosc. Rev., 2010, 45, 409–446, DOI:10.1080/05704921003718991.
- U. Resch-Genger, M. Grabolle and S. Cavaliere-Jaricot,
et al., Quantum dots versus organic dyes as fluorescent labels, Nat., 2008, 5, 763–775, DOI:10.1038/nmeth.1248.
- M. Verma, Y.-H. Chan and S. Saha,
et al., Recent developments in semiconducting polymer dots for analytical detection and NIR-II fluorescence imaging, ACS Appl. Bio Mater., 2020, 4, 2142–2159, DOI:10.1021/acsabm.0c01185.
- H. Wang, X. Ji and Z. Li,
et al., Fluorescent supramolecular polymeric materials, Adv. Mater., 2017, 29, 1606117, DOI:10.1002/adma.201606117.
- F. Yan, Y. Hou and C. Yi,
et al., Carbon dots modified/prepared by supramolecular host molecules and their potential applications: A review, Anal. Chim. Acta, 2022, 340475, DOI:10.1016/j.aca.2022.340475.
-
H. Tian and J. Zhang, Photochromic Materials: Preparation, Properties and Applications, John Wiley & Sons, 2016 Search PubMed.
- M. E. Genovese, E. Colusso and M. Colombo,
et al., Acidochromic fibrous polymer composites for rapid gas detection, J. Mater. Chem. A, 2017, 5, 339–348, 10.1039/C6TA08793K.
- M.-Q. Zhu, L. Zhu and J. J. Han,
et al., Spiropyran-based photochromic polymer nanoparticles with optically switchable luminescence, J. Am. Chem. Soc., 2006, 128, 4303–4309, DOI:10.1021/ja0567642.
- N. Choudhury and P. De, Recent progress in pendant rhodamine-based polymeric sensors for the detection of copper, mercury and iron ions, J. Macromol. Sci. A., 2021, 58, 835–848, DOI:10.1080/10601325.2021.1960172.
- B. Sam, L. George and A. Varghese, Fluorescein based fluorescence sensors for the selective sensing of various analytes, J. Fluoresc., 2021, 31, 1251–1276, DOI:10.1007/s10895-021-02770-9.
- H.-Q. Dong, T.-B. Wei and X.-Q. Ma,
et al., 1, 8-Naphthalimide-based fluorescent chemosensors: recent advances and perspectives, J. Mater. Chem. C, 2020, 8, 13501–13529, 10.1039/D0TC03681A.
- V.-N. Nguyen, J. Ha and M. Cho,
et al., Recent developments of BODIPY-based colorimetric and fluorescent probes for the detection of reactive oxygen/nitrogen species and cancer diagnosis, Coord. Chem. Rev., 2021, 439, 213936, DOI:10.1016/j.ccr.2021.213936.
- P. Singh, A. Hirsch and S. Kumar, Perylene diimide-based chemosensors emerging in recent years: From design to sensing, TrAC, Trends Anal. Chem., 2021, 138, 116237, DOI:10.1016/j.trac.2021.116237.
- I. Manolov, C. Maichle-Moessmer and N. Danchev, Synthesis, structure, toxicological and pharmacological investigations of 4-hydroxycoumarin derivatives, Eur. J. Med. Chem., 2006, 41, 882–890, DOI:10.1016/j.ejmech.2006.03.007.
- V. Kumar, P. Kumar and R. Gupta, Detection of Al 3+ and Fe 3+ ions by nitrobenzoxadiazole bearing pyridine-2, 6-dicarboxamide based chemosensors: effect of solvents on detection, New J. Chem., 2020, 44, 13285–13294, 10.1039/D0NJ00517G.
- Z. Kowser, U. Rayhan and T. Akther,
et al., A brief review on novel pyrene based fluorometric and colorimetric chemosensors for the detection of Cu 2+, Mater. Chem. Front., 2021, 5, 2173–2200, 10.1039/D0QM01008A.
- J. Chen, D. Huang and M. She,
et al., Recent progress in fluorescent sensors for drug-induced liver injury assessment, ACS Sens., 2021, 6, 628–640, DOI:10.1021/acssensors.0c02343.
- A. D. Kurdekar, L. Avinash Chunduri and C. S. Manohar,
et al., Streptavidin-conjugated gold nanoclusters as ultrasensitive fluorescent sensors for early diagnosis of HIV infection, Sci. Adv., 2018, 4, eaar6280, DOI:10.1126/sciadv.aar6280.
- N.-R. Ha, I.-P. Jung and I.-J. La,
et al., Ultra-sensitive detection of kanamycin for food safety using a reduced graphene oxide-based fluorescent aptasensor, Sci. Rep., 2017, 7, 40305, DOI:10.1038/srep40305.
- N. De Acha, C. Elosúa and J. M. Corres,
et al., Fluorescent sensors for the detection of heavy metal ions in aqueous media, Sensors, 2019, 19, 599, DOI:10.3390/s19030599.
- A. G. Melnikov, D. A. Bykov and A. S. Varezhnikov,
et al., Toward a Selective Analysis of Heavy Metal Salts in Aqueous Media with a Fluorescent Probe Array, Sensors, 2022, 22, 1465, DOI:10.3390/s22041465.
- B. Zhang, H. Liu and F. Wu,
et al., A dual-response quinoline-based fluorescent sensor for the detection of Copper (II) and Iron (III) ions in aqueous medium, Sens. Actuators B Chem., 2017, 243, 765–774, DOI:10.1016/j.snb.2016.12.067.
- M. Chemchem, A. Chemchem and B. Aydıner,
et al., Recent advances in colorimetric and fluorometric sensing of neurotransmitters by organic scaffolds, Eur. J. Med. Chem., 2022, 244, 114820, DOI:10.1016/j.ejmech.2022.114820.
- A. Kumar, M. Saini and B. Mohan,
et al., Colorimetric and fluorescent Schiff base sensors for trace detection of pollutants and biologically significant cations: A review (2010-2021), Microchem. J., 2022, 181, 107798, DOI:10.1016/j.microc.2022.107798.
-
R. Misra and S. P. Bhattacharyya, Intramolecular Charge Transfer: Theory and Applications, John Wiley & Sons, 2018, DOI:10.1016/j.ejmech.2022.114820.
- A. Abdollahi, Z. Alinejad and A. R. Mahdavian, Facile and fast photosensing of polarity by stimuli-responsive materials based on spiropyran for reusable sensors: a physico-chemical study on the interactions, J. Mater. Chem. C, 2017, 5, 6588–6600, 10.1039/C7TC02232H.
- A. Abdollahi, A. Mouraki and M. H. Sharifian,
et al., Photochromic properties of stimuli-responsive cellulosic papers modified by spiropyran-acrylic copolymer in reusable pH-sensors, Carbohydr. Polym., 2018, 200, 583–594, DOI:10.1016/j.carbpol.2018.08.042.
- A. Radu, R. Byrne and N. Alhashimy,
et al., Spiropyran-based reversible, light-modulated sensing with reduced photofatigue, J. Photochem. Photobiol., A, 2009, 206, 109–115, DOI:10.1016/j.jphotochem.2009.05.022.
- Y. Xue, J. Tian and W. Tian,
et al., Spiropyran based recognitions of amines: UV-vis spectra and mechanisms, Spectrochim. Acta–A: Mol. Biomol. Spectros., 2021, 250, 119385, DOI:10.1016/j.saa.2020.119385.
- L. Wang, Y. Yao and J. Wang,
et al., Selective sensing Ca2+ with a spiropyran-based fluorometric probe, Luminescence, 2019, 34, 707–714, DOI:10.1002/bio.3656.
- J. Kang, E. Li, L. Cui, Q. Shao, C. Yin and F. Cheng, Lithium ion specific fluorescent reversible extraction-release based on spiropyran isomerization combining crown ether coordination and its bioimaging, Sens. Actuators B Chem., 2021, 327, 128941, DOI:10.1016/j.snb.2020.128941.
- J. K. Rad, Z. Balzade and A. R. Mahdavian, Spiropyran-based advanced photoswitchable materials: A fascinating pathway to the future stimuli-responsive devices, J. Photochem. Photobiol. C., 2022, 51, 100487, DOI:10.1016/j.jphotochemrev.2022.100487.
- D. Zhang, Y. Qi, Y. Li and P. Cong, A New Spiropyran-Based Fluorescent Probe for Dual Sensing of Ferrous Ion and pH, J. Fluoresc., 2021, 31, 1133–1141, DOI:10.1007/s10895-021-02741-0.
- E. Li, J. Kang, P. Ye, W. Zhang, F. Cheng and C. Yin, A prospective material for the highly selective extraction of lithium ions based on a photochromic crowned spirobenzopyran, J. Mater. Chem. B, 2019, 7, 903–907, 10.1039/C8TB02906G.
- S. Heng, M.-C. Nguyen, R. Kostecki, T. M. Monro and A. D. Abell, Nanoliter-scale, regenerable ion sensor: sensing with a surface functionalized microstructured optical fibre, RSC Adv., 2013, 3, 8308–8317, 10.1039/C3RA40321A.
- W. Qin, S. O. Obare, C. J. Murphy and S. M. Angel, Specific fluorescence determination of lithium ion based on 2-(2-hydroxyphenyl) benzoxazole, Analyst, 2001, 126, 1499–1501, 10.1039/B104216P.
- S. O. Obare and C. J. Murphy, Selective blue emission from an HPBO–Li+ complex in alkaline media, New J. Chem., 2001, 25, 1600–1604, 10.1039/B103562M.
- N. A. Padilla, M. T. Rea, M. Foy, S. P. Upadhyay, K. A. Desrochers, T. Derus, K. A. Knapper, N. H. Hunter, S. Wood and D. A. Hinton, Tracking lithium ions via widefield fluorescence microscopy for battery diagnostics, ACS Sens., 2017, 2, 903–908, DOI:10.1021/acssensors.7b00087.
- S. Kumari, S. Joshi and A. Sarmah,
et al., Highly Selective Sensing of Li+ in H2O/CH3CN via Fluorescence ‘Turn-on’Response of a Coumarin-Indole Linked Dyad: an Experimental and Theoretical Study, J. Fluoresc., 2016, 26, 2177–2185, DOI:10.1007/s10895-016-1913-1.
- G. Valente, M. Esteve-Rochina and S. P. Alves,
et al., Perylene-Based Coordination Polymers: Synthesis, Fluorescent J-Aggregates, and Electrochemical Properties, Inorg. Chem., 2023, 62, 7834–7842, DOI:10.1021/acs.inorgchem.3c00540.
- S. Chen, Z. Xue and N. Gao,
et al., Perylene diimide-based fluorescent and colorimetric sensors for environmental detection, Sensors, 2020, 20, 917, DOI:10.3390/s20030917.
- L. Yan, L. Yang and J. Lan,
et al., A new perylene diimide-based colorimetric and fluorescent sensor for selective detection of Cu2+ cation, Sci. China, Ser. B: Chem., 2009, 52, 518–522, DOI:10.1016/j.dyepig.2016.07.028.
- D. Sriramulu and S. Valiyaveettil, Perylene derivatives as a fluorescent probe for sensing of amines in solution, Dyes Pigm., 2016, 134, 306–314, DOI:10.1016/j.apsusc.2020.146396.
- X. Lian, Z. Ma and Z. Zhang,
et al., Alkali metal storage mechanism in organic semiconductor of perylene-3, 4, 9, 10-tetracarboxylicdianhydride, Appl. Surf. Sci., 2020, 524, 146396, DOI:10.1016/j.apsusc.2020.146396.
- J. Xie and Q. Zhang, Recent progress in rechargeable lithium batteries with organic materials as promising electrodes, J. Mater. Chem. A, 2016, 4, 7091–7106, 10.1039/C6TA01069E.
- X. X. Lian, Z. Ma, Z. Zhang, J. Yang, S. Sun, C. Gu, Y. Liu, H. Ding, J. Hu and X. Cao, An in-situ spectroscopy investigation of alkali metal interaction mechanism with the imide functional group, Nano Res., 2020, 13, 3224–3229, DOI:10.1007/s12274-020-2991-6.
- D. J. Kim, S. H. Je, S. Sampath, J. W. Choi and A. Coskun, Effect of N-substitution in naphthalenediimides on the electrochemical performance of organic rechargeable batteries, RSC Adv., 2012, 2, 7968–7970, 10.1039/C2RA21239K.
- Z. Ba, Z. Wang, M. Luo, H.-b. Li, Y. Li, T. Huang, J. Dong, Q. Zhang and X. Zhao, Benzoquinone-based polyimide derivatives as high-capacity and stable organic cathodes for lithium-ion batteries, ACS Appl. Mater. Interfaces, 2019, 12, 807–817, DOI:10.1021/acsami.9b18422.
- R. V. Hangarge, D. D. La, M. Boguslavsky, L. A. Jones, Y. S. Kim and S. V. Bhosale, An Aza-12-crown-4 Ether-Substituted Naphthalene Diimide Chemosensor for the Detection of Lithium Ion, ChemistrySelect, 2017, 2, 11487–11491, DOI:10.1002/slct.201702085.
- J. Aher, A. Graefenstein, G. Deshmukh, K. Subramani, B. Krueger, M. Haensch, J. Schwenzel, K. Krishnamoorthy and G. Wittstock, Effect of aromatic rings and substituent on the performance of lithium batteries with rylene imide cathodes, ChemElectroChem, 2020, 7, 1160–1165, DOI:10.1002/celc.202000118.
- M. R. Raj, N. Kim and G. Lee, A perylene-based aromatic polyimide with multiple carbonyls enabling high-capacity and stable organic lithium and sodium ion batteries, Sustain. Energy Fuels., 2021, 5, 175–187, 10.1039/D0SE01246G.
- X. Zhu, X. Liu, W. Deng, L. Xiao, H. Yang and Y. Cao, Perylenediimide dyes as a cheap and sustainable cathode for lithium ion batteries, Mater. Lett., 2016, 175, 191–194, DOI:10.1016/j.matlet.2016.04.038.
- L. Li, Y. J. Hong, D. Y. Chen and M. J. Lin, Molecular Engineering of Perylene Imides for High-Performance Lithium Batteries: Diels–Alder Extension and Chiral Dimerization, Eur. J. Chem., 2017, 23, 16612–16620, DOI:10.1002/chem.201703823.
- T. Nokami, T. Matsuo, Y. Inatomi, N. Hojo, T. Tsukagoshi and H. Yoshizawa, Polymer-bound pyrene-4, 5, 9, 10-tetraone for fast-charge and-discharge lithium-ion batteries with high capacity, J. Am. Chem. Soc., 2012, 134, 19694–19700, DOI:10.1021/ja306663g.
- S. Zheng, L. Miao, T. Sun, L. Li, T. Ma, J. Bao, Z. Tao and J. Chen, An extended carbonyl-rich conjugated polymer cathode for high-capacity lithium-ion batteries, J. Mater. Chem. A, 2021, 9, 2700–2705, 10.1039/D0TA11648C.
- Y. Gao, T. Ma, Z. Ou, W. Cai, G. Yang, Y. Li, M. Xu and Q. Li, Highly sensitive and selective turn-on fluorescent chemosensors for Hg2+ based on thioacetal modified pyrene, Talanta, 2018, 178, 663–669, DOI:10.1016/j.talanta.2017.09.089.
- S. Sarkar, S. Roy, A. Sikdar, R. Saha and S. S. Panja, A pyrene-based simple but highly selective fluorescence sensor for Cu2+ ions via a static excimer mechanism, Analyst, 2013, 138, 7119–7126, 10.1039/C3AN00928A.
- X. Feng, X. Wang and C. Redshaw,
et al., Aggregation behaviour of pyrene-based luminescent materials, from molecular design and optical properties to application, Chem. Soc. Rev., 2023, 52, 6715–6753, 10.1039/D3CS00251A.
- W. Qin, M. Baruah, M. Sliwa, M. Van der Auweraer, W. M. De Borggraeve, D. Beljonne, B. Van Averbeke and N. B. Ratiometric, fluorescent BODIPY dye with aza crown ether functionality: synthesis, solvatochromism, and metal ion complex formation, J. Phys. Chem. A, 2008, 112, 6104–6114, DOI:10.1021/jp800261v.
- Y. Ando, Y. Hiruta, D. Citterio and K. Suzuki, A highly Li+-selective glass optode based on fluorescence ratiometry, Analyst, 2009, 134, 2314–2319, 10.1039/B912756A.
- D. Tzeli, I. D. Petsalakis and G. Theodorakopoulos, Theoretical study on the electronic structure, formation and absorption spectra of lithium, sodium and potassium complexes of N-confused tetraphenylporphyrin, Comput. Theor. Chem., 2013, 1020, 38–50, DOI:10.1016/J.COMPTC.2013.07.014.
- M. P. Militello, R. E. H. Ramírez, I. V. Lijanova, C. M. Previtali, S. G. Bertolotti and E. M. Arbeloa, PAMAM dendrimers with a porphyrin core as highly selective binders of Li+ in an alkaline mixture. A spectroscopic study, New J. Chem., 2019, 43, 16246–16254, 10.1039/C9NJ04088A.
- D. Tzeli, I. D. Petsalakis and G. Theodorakopoulos, Computational insight into the electronic structure and absorption spectra of lithium complexes of N-confused tetraphenylporphyrin, J. Phys. Chem. A, 2011, 115, 11749–11760, DOI:10.1021/jp204298q.
- P. A. Stuzhin, S. S. Ivanova, M. Hamdoush, G. A. Kirakosyan, A. Kiselev, A. Popov, V. Sliznev and C. Ercolani, Tetrakis (1, 2, 5-thiadiazolo) porphyrazines. 9. Synthesis and spectral and theoretical studies of the lithium (i) complex and its unusual behaviour in aprotic solvents in the presence of acids, Dalton Trans., 2019, 48, 14049–14061, 10.1039/C9DT02345C.
- X. Lian-Cai, L. Zun-Yun, H. Tian-Jing, L. Fan-Chen and C. Dong-Ming, Density functional theory studies of lithium porphyrin radicals, Chem. Phys., 2004, 305, 165–174, DOI:10.1016/j.chemphys.2004.06.044.
- M. Tabata, J. Nishimoto and T. Kusano, Spectrophotometric determination of lithium ion using a water-soluble octabromoporphyrin in aqueous solution, Talanta, 1998, 46, 703–709, DOI:10.1016/S0039-9140(97)00327-5.
- M. McCarrick, S. J. Harris and D. Diamond, Assessment of three azophenol calix [4] arenes as chromogenic ligands for optical detection of alkali metal ions, Analyst, 1993, 118, 1127–1130, 10.1039/AN9931801127.
- A. Gulino, F. Lupo, D. A. Cristaldi, S. Pappalardo, C. Capici, G. Gattuso, A. Notti and M. F. Parisi, A viable route for lithium ion detection, Eur. J. Inorg. Chem., 2014, 2014, 442–449, DOI:10.1002/ejic.201301213.
- K. Hiratani, M. Nomoto, H. Sugihara and T. Okada, Behaviour of 2, 9-disubstituted 1, 10-phenanthroline derivatives as specific lithium ion fluorophores, Analyst, 1992, 117, 1491–1495, 10.1039/AN9921701491.
- S. O. Obare and C. J. Murphy, A two-color fluorescent lithium ion sensor, Inorg. Chem., 2001, 40, 6080–6082, DOI:10.1021/ic010271q.
- H. Soni, J. Prasad, A. Pandya, S. S. Soni and P. G. Sutariya, Disposable paper-based PET fluorescence probe linked with calix [4] arene for lithium and phosphate ion detection, New J. Chem., 2022, 46, 21115–21123, 10.1039/D2NJ04536B.
- I. Grabchev, S. Dumas and J.-M. Chovelon, A polyamidoamine dendrimer as a selective colorimetric and ratiometric fluorescent sensor for Li+ cations in alkali media, Dyes Pigm., 2009, 82, 336–340, DOI:10.1016/j.dyepig.2009.02.003.
- I. Grabchev, V. Bojinov and J.-M. Chovelon, Synthesis, photophysical and photochemical properties of fluorescent poly (amidoamine) dendrimers, Polymer, 2003, 44, 4421–4428, DOI:10.1016/S0032-3861(03)00407-5.
- M. Li, J. Yang, Y. Shi, Z. Chen, P. Bai, H. Su, P. Xiong, M. Cheng, J. Zhao and Y. Xu, Soluble Organic Cathodes Enable Long Cycle Life, High Rate, and Wide-Temperature Lithium-Ion Batteries, Adv. Mater., 2022, 34, 2107226, DOI:10.1002/adma.202107226.
- R. G. Pearson, Recent advances in the concept of hard and soft acids and bases, J. Chem. Educ., 1987, 64, 561, DOI:10.1021/ed064p561.
- M. Kamenica, R. R. Kothur, A. Willows, B. A. Patel and P. J. Cragg, Lithium ion sensors, Sensors, 2017, 17, 2430, DOI:10.3390/s17102430.
- A.-P. Chiriac and M.-D. Damaceanu, A novel approach towards crown-ether modified polyimides with affinity for alkali metal ions recognition, J. Mol. Liq., 2021, 322, 114929, DOI:10.1016/j.molliq.2020.114929.
- A. Tsukanov, A. Dubonosov, V. Bren and V. Minkin, Organic chemosensors with crown-ether groups, Chem. Heterocycl. Compd., 2008, 44, 899–923, DOI:10.1007/s10593-008-0132-3.
- G. Kaur, A. Singh and P. Venugopalan,
et al., Selective recognition of lithium (i) ions using Biginelli based fluorescent organic nanoparticles in an aqueous medium, RSC Adv., 2016, 6, 1792–1799, 10.1039/C5RA16743D.
- L. Li, H. X. Gong, D. Y. Chen and M. J. Lin, Stable Bifunctional Perylene Imide Radicals for High-Performance Organic–Lithium Redox-Flow Batteries, Eur. J. Chem., 2018, 24, 13188–13196, DOI:10.1002/chem.201801443.
- L. Tarazi, H. Choi, J. C. Mason, . J. Sowell, L. Strekowski and G. Patonay, Characterization of a novel crown ether-bearing near-infrared heptamethine cyanine dye. A study of fluorescence quenching by lithium, Microchem. J., 2002, 72, 55–62, DOI:10.1016/S0026-265X(01)00150-3.
- A. Barzykin, M. A. Fox, E. N. Ushakov, O. Stanislavskii, S. Gromov, O. Fedorova and M. Al'fimov, Dependence of metal ion complexation and intermolecular aggregation on photoinduced geometric isomerism in a crown ether styryl dye, J. Am. Chem. Soc., 1992, 114, 6381–6385, DOI:10.1021/ja00042a013.
- K. Hiratani, M. Kaneyama, Y. Nagawa, E. Koyama and M. Kanesato, Synthesis of [1] rotaxane via covalent bond formation and its unique fluorescent response by energy transfer in the presence of lithium ion, J. Am. Chem. Soc., 2004, 126, 13568–13569, DOI:10.1021/ja046929r.
- T. Gunnlaugsson, B. Bichell and C. Nolan, A novel fluorescent photoinduced electron transfer (PET) sensor for lithium, Tetrahedron Lett., 2002, 43, 4989–4992, DOI:10.1016/S0040-4039(02)00895-X.
- M. Kumar, N. Kumar and V. Bhalla, Thiacalix [4] crown based optical chemosensor for Fe3+, Li+ and cysteine: a Fe3+/Li+ ion synchronized allosteric regulation, Dalton Trans., 2013, 42, 981–986, 10.1039/c2dt31940c.
- M. Cametti, L. Ilander and K. Rissanen, Recognition of Li+ by a Salophen− UO2 Homodimeric Complex, Inorg. Chem., 2009, 48, 8632–8637, DOI:10.1021/ic901136b.
- S. S. Rochat, Z. Grote and K. Severin, Ruthenium-based metallacrown complexes for the selective detection of lithium ions in water and in serum by fluorescence spectroscopy, Org. Biomol. Chem., 2009, 7, 1147–1153, 10.1039/B820592B.
- K. Kimura, M. Tanaka, S. Kitazawa and T. Shono, Highly lithium-selective crown ether dyes for extraction photometry, Chem. Lett., 1985, 14, 1239–1240, DOI:10.1246/cl.1985.1239.
- J. K. Trautman, V. P. Gadzekpo and G. D. Christian, Spectrophotometric determination of lithium in blood serum with thoron, Talanta, 1983, 30, 587–591, DOI:10.1016/0039-9140(83)80137-4.
|
This journal is © The Royal Society of Chemistry 2023 |