DOI:
10.1039/D2TA09699D
(Review Article)
J. Mater. Chem. A, 2023,
11, 3753-3770
Cyano-capped molecules: versatile organic materials
Received
13th December 2022
, Accepted 18th January 2023
First published on 18th January 2023
Abstract
Conjugated small molecules bearing the cyano group were reviewed. These organic compounds are extensively used as active layers of solar cells and light-emitting diodes as well as materials with high second- and third-order nonlinearities. Typical organic light conversion agents are also based on CN-capped π-conjugated scaffolds that, in addition, are also increasingly popular for building various organic sensors and probes. More recently, cyanoarenes and dicyanopyrazines were identified as efficient photoredox catalysts. Fundamental structure–property relationships of CN-capped molecules used across the aforementioned areas were elucidated and discussed. The cyano group possessing electron-withdrawing character and linear arrangement is capable of significantly altering electronic and optical properties, and supramolecular arrangement. Hence, extensive property tuning/regulation through the CN group has been demonstrated.
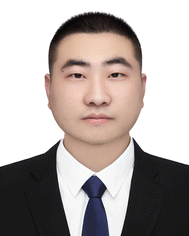 Hongyang Wang | Hongyang Wang received his B.S. degree in 2017 and M.S. degree in 2021 from the Hebei Normal University of Science & Technology. Currently, he is pursuing a PhD degree under the supervision of Prof. Jialei Liu at the Chinese Academy of Agricultural Sciences (CAAS), Beijing, China. His research interests include the synthesis of specifically functionalized luminescence materials and their application in green agriculture. He is conducting research on new foliar light fertilizers and light conversion agents for agricultural films. |
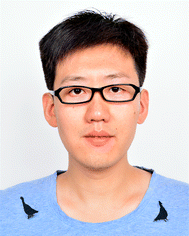 Chipeng Zhao | Chipeng Zhao received his M.S. degree from the Chinese Academy of Agricultural Sciences (CAAS) in 2022. From 2019 to 2022, he conducted his graduate study under the supervision of Prof. Jialei Liu. His research interests involve the synthesis and application of versatile organic light conversion agents in agriculture. |
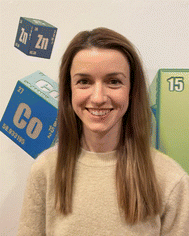 Zuzana Burešová | Zuzana Burešová studied organic chemistry at the University of Pardubice, where she finished her master's and doctoral studies in 2016 and 2019, respectively. In 2018, she pursued a three-month internship at the FAU (Erlangen, Germany) under the guidance of Prof. M. Kivala. Her current research activities focus mainly on the design and synthesis of organic π-systems and visible light-initiated organic transformations via photoredox catalysis. |
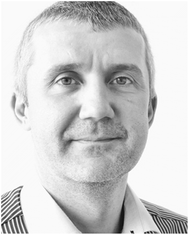 Filip Bureš | Filip Bureš finished his master's and doctoral studies in organic chemistry at the University of Pardubice in 2002 and 2005, respectively. Subsequently, he pursued studies at the LMU (Germany) and a 14 months postdoctoral fellowship at the ETH Zűrich (Switzerland) under the guidance of Prof. P. Knochel and Prof. F. Diederich. After his return to Pardubice, he was habilitated in 2010 and has subsequently been a full professor since December 2017. His current scientific interest includes advanced organic and organometallic materials with manifold applications. |
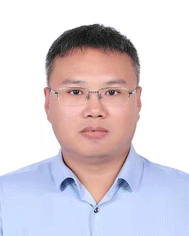 Jialei Liu | Dr. Jialei Liu is an experienced researcher with a history of research in the field of organic chemistry and optical materials. He is skilled in organic synthesis, functional films, and optical modulation. He has focused on extensive fields of nonlinear optical materials, biodegradable plastic films, and natural products. He obtained his PhD degree (2010) in organic chemistry from the University of Chinese Academy of Sciences, Beijing, China. He is now working as a Professor at the Institute of Environment and Sustainable Development in Agriculture, Chinese Academy of Agricultural Sciences. So far, he has published more than 100 SCI academic peer-reviewed papers. |
Cyano group as a part of small conjugated molecules
The cyano (C
N) group bearing both C and N atoms in sp-hybridization is a popular substituent considered a powerful electron-withdrawing moiety with high substituent constants (Hammett σp = 0.66; Pytela σi = 0.525).1 The cyano group possesses negative inductive and mesomeric effects, and the C
N bond is highly polarized, which is sensitively reflected by its characteristic stretching vibration in the infrared spectra (∼2200 cm−1). Hence, the CN group is tremendously used as a linearly-arranged electron acceptor capable of (i) polarizing π-conjugated systems, (ii) imparting intramolecular charge-transfer (ICT) when interconnected to an electron donor, (iii) creating an electron rich part of the molecule (due to −I/M effects), (iv) reducing the LUMO level without altering the HOMO, (v) narrowing the HOMO–LUMO gap, (vi) red-shifting the longest-wavelength absorption maxima, (vii) providing a coordination site for cations and electrophiles (via nitrogen's lone pair), while (viii) the carbon is prone to a nucleophilic attack.2 These fundamental properties impart organic CN-functionalized semiconductors various exploitable features such as red-shifted/wide absorption, intense and narrow luminescence, charge-transfer (CT) excited states with reduced energy, low energy losses during CT, reduced electron trapping effect, increased charge-mobility, etc.3–6 Hence, cyano-capped compounds find wide applications across organic electronics and related areas, including organic solar cells (OSCs), organic light-emitting diodes (OLEDs), nonlinear optics (NLO), light conversion agents, organic fluorescent probes, and photoredox catalysis (Scheme 1). Fundamental structure–property relationships of CN-capped organic materials used across these application areas will be discussed. The review does not intend to exhaustively summarize all recent contributions but rather demonstrate the property tuning on suitable examples.
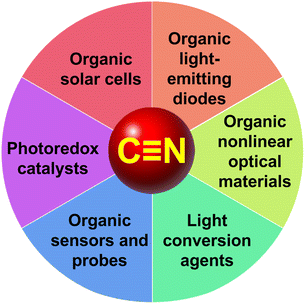 |
| Scheme 1 The main application areas of CN-capped organic molecules covered in this review. | |
Organic solar cells
In view of the current worldwide situation, energy seems to be a very critical issue. Nowadays, about 85% of the energy is produced from fossil fuels, whereas tidal, wind, biomass, hydroelectric, and solar energy harvesting have undergone rapid development.7–9 Despite their lower efficiency as compared to inorganic counterparts, organic solar cells (OSCs) represent an alternative technology with reduced material costs/environmental pollution and facilitated manufacturing process.10,11 In principle, organic photovoltaic cells are capable of converting photons into electrons and supplying electricity due to an active organic layer. The used organic π-conjugated materials are generally lightweight, low-cost, flexible, and can be easily adjusted to absorb in the Vis region (HOMO–LUMO gap tuning).2,12–16 Bulk heterojunction (BHJ) solar cells utilizing a polymeric donor and a fullerene acceptor have been the main research focus in the recent period.17–22 Fullerene acceptors possess extraordinary charge transport but also low absorption coefficient, difficult purification, and limited property tuning. Hence, non-fullerene acceptors (NFAs) are currently a rapidly growing area of research with very promising preliminary results, reaching power conversion efficiency (PCE) of up to 19%.23–28 Small conjugated molecules that are easy to synthesize and purify at a low cost, possessing tunable HOMO–LUMO gap and high molar extinction coefficient, are a potential substitute for fullerene acceptors. These organic semiconductors containing CN group(s) will be the main focus of this review.
The simplest cyano-capped semiconductors used in OSCs possess a terminal cyano group appended in a conjugated position, acting as an electron acceptor. These push–pull molecules showed pronounced optical properties and charge mobilities. For instance, Ie et al. designed a series of simple π-conjugated compounds 1a–e based on CN-substituted benzothiadiazole interconnected to variously N-substituted phthalimide moieties (Fig. 1, Table 1). It has been shown that a combination of electron-deficient aromatic cycle and CN group leads to a low-lying LUMO level, which is essential for photovoltaic application.29 Shibata et al. synthesized two benzothiadiazole-derived n-type materials 2a,b (Fig. 1) with two terminal CN or CF3 groups. Both derivatives were utilized in organic photovoltaics, whereas the performance of a device based on cyano-capped 2a was significantly enhanced. Derivative 2a bearing two CN groups showed improved crystallinity and diminished carrier recombination and resistance.30
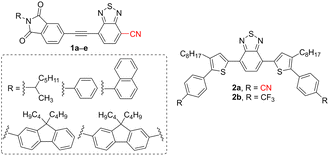 |
| Fig. 1 Small CN-substituted benzothiadiazole derivatives for application in OSCs. | |
Table 1 Optoelectronic parameters of NFAs 1–5 based on cyano(hetero)arene/olefin
Acceptor |
λ
max
A (nm eV−1) |
E
g (eV) |
E
LUMO (eV) |
Donor |
PCE (%) |
Ref. |
1a
|
ca. 375/3.31 |
2.97 |
−3.48 |
P3HT |
0.19 |
29
|
1b
|
ca. 375/3.31 |
2.97 |
−3.47 |
P3HT |
0.12 |
29
|
1c
|
ca. 375/3.31 |
2.98 |
−3.48 |
P3HT |
— |
29
|
1d
|
ca. 310/4.00 |
2.95 |
−3.48 |
P3HT |
0.32 |
29
|
1e
|
ca. 315/3.94 |
2.94 |
−3.48 |
P3HT |
0.28 |
29
|
2a
|
350/3.54 |
— |
−3.33 |
P3HT |
0.86 |
30
|
2b
|
335/3.70 |
— |
−3.30 |
P3HT |
0.09 |
30
|
3
|
655/1.89 |
1.72 |
−3.90 |
P3HT |
3.17 |
32
|
4
|
410/3.02 |
— |
−3.48 |
P3HT |
1.86 |
33
|
5
|
693/1.79 |
1.66 |
−3.65 |
P3HT |
2.37 |
35
|
Sharma et al. prepared a symmetrical perylene bisimide (PBI) derivative 3 with two cyano/nitro-substituted stilbene moieties (Fig. 2). Compared with the devices based on five perylene bisimide derivatives without CN groups as acceptor (they displayed a PCE of 1.92%, 3.11%, 2.54%, 1.54%, and 1.36%, respectively),31 its electrochemical investigation indicated that a device with a thermally annealed active layer of P3HT:PBI blend possessed an enhanced PCE of 3.17%. Hence, the PBI 3 bearing the CN electron acceptor can effectively improve the PCE by balancing the absorbance and the charge mobility of the blend.32 Fullerene-free small molecule based on fused acenaphthoisoindoledione 4 (Fig. 2) has been constructed by Zhou et al.33 A blend of 4 as acceptor and P3HT donor provided the PCE up to 1.86% in a solution-processed BHJ solar cell. Therein, the introduction of electron-withdrawing CN group on the fluoranthene-fused imide skeleton effectively tuned its LUMO levels (around −3.5 eV), and optimized the open circuit voltage (Voc) of the photovoltaic devices, making 4 a promising candidate as a new electron acceptor in BHJ photovoltaic cells. Most notably, when the CN group in 4 was substituted for an analogical and simple electron-withdrawing group (a methyl-ester group), a new acceptor was yielded. Its blend with P3HT only exhibited a relatively low PCE of 1.61% in the BHJ solar cells.34
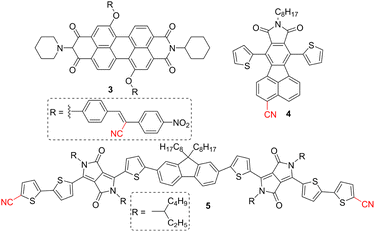 |
| Fig. 2 CN-capped perylene bisimide (3), acenaphthoisoindoledione (4) and fluorene-DPP-CN (5) acceptors for OSCs. | |
Fluorene can be combined with diketopyrrolopyrrole (DPP) units via 2,5-thienylene linkers as demonstrated by Li et al. in oligomeric 5 (Fig. 2). As compared to previous small cyano-capped molecules 1–4 (Table 1), this designed acceptor possesses red-shifted absorption maxima, significantly reduced HOMO–LUMO gap, deepened LUMO level, and good electron mobility (up to 10−3 cm2 V−1 s−1). A BHJ solar cell built on 5 and P3HT showed PCE of 2.37%.35
Two geminal cyano groups attached to a π-conjugated backbone via olefinic linker is well-known as dicyanovinyl (DCV) moiety.36 It is a tremendously popular electron acceptor across organic dyes, chromophores, semiconductors, etc., mostly due to its easy introduction via Knoevenagel condensation of an aldehyde and malononitrile. As compared to single CN-substituted molecules, DCV-functionalization brings about an enlarged π-system by an additional olefinic linker end-capped by two CN groups. Hence, DCV-substituted compounds possess bathochromically and hyperchromically shifted absorption spectra, an important requirement for photovoltaic devices.37 Central electron releasing carbazole decorated by two benzothiadiazole acceptors further supported by terminal DCV moieties allowed the construction of (A–A)2–D derivatives 6 (Fig. 3). Its use as an acceptor along with PCE10 polymeric donor in BHJ solar cell resulted in a device efficiency reaching 5.3%. DCV-capped semiconductor 6 showed low-lying LUMO (Table 2) and efficient ICT, while its rigid-planar structure enhanced intermolecular interactions and pronounced carrier mobilities.38
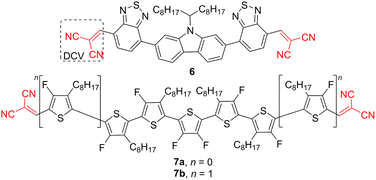 |
| Fig. 3 Two representative examples of DCV-capped semiconductors having (A–A)2–D (6) and oligomeric (7) arrangement. | |
Table 2 Optoelectronic parameters of DCV-capped semiconductors 6, 7
Acceptor |
λ
max
A (nm eV−1) |
E
g (eV) |
E
LUMO (eV) |
Donor |
PCE (%) |
Ref. |
Calculated LUMO levels of 7a and 7b with B3LYP/6-31G(d,p).
Calculated LUMO levels of 7a and 7b with ωB97XD, respectively.
|
6
|
517/2.40 |
2.02 |
−3.64 |
PCE10 |
5.30 |
38
|
7a
|
ca. 540/2.30 |
1.93 |
−3.48a/−1.93b |
PCE10 |
4.50 |
39
|
7b
|
ca. 555/2.23 |
1.81 |
−3.31a/−1.76b |
PCE10 |
1.80 |
39
|
Duan et al. reported two analogous perfluorinated oligothiophene derivatives 7a, 7b bearing five or seven thiophene rings (Fig. 3). Despite Duan's research being primarily focused on the fluorination effect, two terminal DCV moieties affected the HOMO/LUMO levels of 7 and imparted general electron deficiency. Hence, oligomers 7a, 7b were used as NFAs and achieved PCEs of 4.5 and 1.8%, respectively (Table 2).39
Dicyanovinyl can be combined with other carbonyl-based acceptors, such as indan-1,3-dione, to gain a strong electron-withdrawing moiety – 1,1-dicyanomethylene-3-indanone (IC). Besides the parent IC, 2-(5,6-difluoro-3-oxo-2,3-dihydro-1H-inden-1-ylidene)malononitrile (FIC) is also often employed in semiconductors. These planar acceptors red-shift the absorption maxima, reduce band gap, increase electron deficiency, and enhance photovoltaic performance.40–42 Triphenylamine (TPA) is very often used to construct star-shaped systems such as 8a, 8b (Fig. 4) bearing three IC terminal acceptors and thiophene/bithiophene linkers. Their LUMO levels (−3.6 eV; Table 3) are well-suited to accept electrons from P3HT donor (−2.8 eV), and the 8a, 8b:P3HT blend showed promising performance in flexible organic photovoltaic devices with PCE up to 1.13% (8a). Furthermore, 8a has been reported to promote an efficient charge extraction process while limiting charge recombination at the interface.43
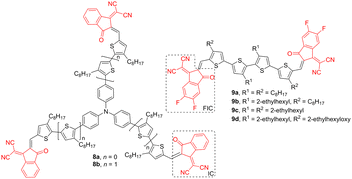 |
| Fig. 4 The molecular structures of TPA (8)- and oligothiophene (9)-based semiconductors bearing peripheral IC and FIC acceptors. | |
Table 3 (F)IC-capped semiconductors and their fundamental properties
Acceptor |
λ
max
A (nm eV−1) |
E
g (eV) |
E
LUMO (eV) |
Donor |
PCE (%) |
Ref. |
8a
|
575/2.16 |
1.93 |
−3.60 |
P3HT |
1.13 |
43
|
8b
|
665/1.86 |
1.71 |
−3.60 |
P3HT |
0.86 |
43
|
9a
|
704/1.76 |
1.47 |
−4.09 |
PBDB-T |
5.53 |
44
|
9b
|
654/1.90 |
1.54 |
−4.02 |
PBDB-T |
9.09 |
44
|
9c
|
667/1.86 |
1.52 |
−3.98 |
PBDB-T/D18 |
10.15/12.04 |
44
|
9d
|
651/1.90 |
1.60 |
−3.86 |
PBDB-T |
8.27 |
44
|
In contrast to odd-membered oligothiophenes 7 (Fig. 3), Zhou et al. developed tetrathiophene (4T) semiconductors 9a–d with two FIC acceptors (Fig. 4). Whereas the FICs affect mostly optical properties, R1 and R2 substituents along the 4T backbone allowed to effectively tune solubility, packing mode, film morphology, and charge mobility. Derivatives 9a–d used as acceptors along with PBDB-T/D18 donors afforded PCE up to 12.04% (Table 3).44
Subphthalocyanine core decorated with three peripheral (F)IC acceptors (Fig. 5) with PBDB-T donor have been utilized in OSCs by Hang et al., achieving the PCE of 4.69 and 3.80%, respectively.45 Compounds 10a, 10b showed extraordinary broad absorption with the full width at half maximum exceeding 260 nm and narrow optical bandgaps (∼1.6 eV; Table 4). When comparing both derivatives, 10b bearing FIC acceptor possesses deepened LUMO level and higher open-circuit voltage (Voc = 0.81 V). Thiophene and selenophene were utilized as linkers in ladder-type derivatives 11 (Fig. 5) bearing two IC acceptors. A solar cell based on the 11:J51 blend afforded PCE of 8.6%.46
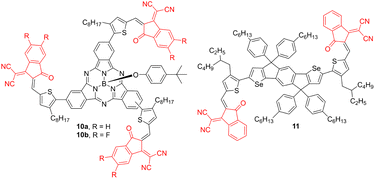 |
| Fig. 5 The molecular structures of subphalocyanine and thiophene-selenophene cores decorated with IC acceptor. | |
Table 4 Optoelectronic parameters of semiconductors bearing the IC acceptors
Acceptor |
λ
max
A (nm eV−1) |
E
g (eV) |
E
LUMO (eV) |
Donor |
PCE (%) |
Ref. |
10a
|
629/1.97 |
1.68 |
−3.90 |
PBDB-T |
4.69 |
45
|
10b
|
634/1.96 |
1.66 |
−4.06 |
PBDB-T |
3.80 |
45
|
11
|
ca. 740/1.68 |
1.52 |
−3.79 |
J51 |
8.60 |
46
|
12
|
702/1.77 |
1.59 |
−3.83 |
PTB7-TH |
6.80 |
47
|
13a
|
700/1.77 |
1.60 |
−3.98 |
PBDB-T |
12.05 |
48
|
13b
|
692/1.79 |
1.63 |
−3.93 |
PBDB-T |
11.29 |
48
|
14
|
ca. 740/1.68 |
1.46 |
−3.82 |
PBDB-T1 |
9.12 |
51
|
15
|
ca. 700/1.77 |
1.58 |
−3.82 |
J61 |
10.57 |
52
|
16
|
722/1.72 |
1.60 |
−3.91 |
See text |
8.06–9.73 |
56–58
|
Enlarged seven-ring fused core of indacenodithieno[3,2-b]thiophene end-capped with two IC acceptors is known as ITIC 12 (Fig. 6). It showed wide absorption, reduced energies of the frontier molecular orbitals, good n-type charge mobility, and miscibility with low band gap polymeric donors such as PTB7-TH as well as suppressed self-aggregation of the central core due to a twisted arrangement of the peripheral substituents. A solar cell with 12:PTB7-TH blended film showed PCE of 6.80% (Table 4).47
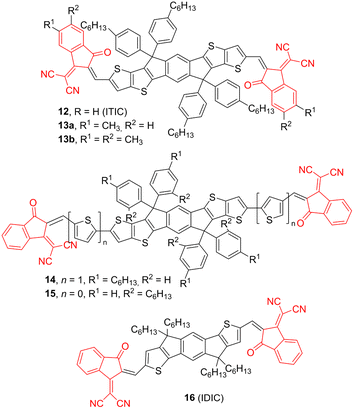 |
| Fig. 6 ITIC family of small acceptors for OSCs. | |
In order to broaden its light absorption and enhance the photocurrent, the parent ITIC (12) has been subjected to various further structural modifications. For example, Lu et al. have prepared two new ITICs 13a, 13b (Fig. 6) bearing (di)methyl-substituted IC units with slightly increased LUMO levels (Table 4). This relatively minor structural tuning improved the PCE of the OSC using 13a, 13b:PBDB-blend up to 12.05/11.29%.48 Further synthetic attempts (Fig. 6) were directed towards the extension of the π-conjugated core by 2,5-thienylene units (14) or introducing hexyl substituents in the position 2 of the appended phenyl rings (15), making the whole acceptor more nonplanar. These structural features allowed obtaining OSCs with the PCE around 10% (Table 4).49–55
Lin et al.56 reported a new ITIC-inspired molecular acceptor 16 named IDIC (Fig. 6), which strongly absorbs within the region of 500–780 nm, possesses a narrow optical bandgap (1.60 eV), and high electron mobility (1.1 × 10−3 cm2 V−1 s−1). It has been used to construct OSCs along with various (polymeric) donors (namely PDBT-T1, PTFBDT-BZS, PTZ1, and H11) with the PCEs within the range of 8.06–9.73% (Table 4).56–58
Indan-1,3-dione (IO) and 1,1-dicyanomethylene-3-indanone (IC) were further utilized as parent scaffolds to study the influence of end groups on NFA's performance (CN vs. CO). For instance, Lin et al. have compared perylene diimide-fused dithiophenepyrroles with the IO and IC end groups bearing additional fluorine/chlorine atoms. The prepared large A–D–A–D–A acceptors bearing peripheral IC groups afforded significantly enhanced PCE up to 9.0%.59 Huang et al. have extensively varied the IC moiety focusing on fused-ring electron acceptors (FREAs). A large series of ITIC derivatives bearing variously modified IC revealed facile modification of the HOMO/LUMO levels and band gap as well as the realization of higher PCE in organic photovoltaic cells with CN-substituted molecules.60 In contrast, dichloroindan-1,3-dione IO4Cl, similar to 12 without CN groups (Fig. 6), showed an outstanding PCE of 26.1% as measured under indoor illumination conditions (2700 K LED at 1000 lux).61 However, a measurement at standard AM 1.5 G conditions revealed diminished PCE = 9.80%. In summary, IC-terminated NAFs may benefit from higher absorption coefficients, tailored HOMO/LUMO levels/band gaps, lower energy loss, and effective charge transfer.
Dicyano rhodanine (mostly N-substituted) is another widely used electron-withdrawing moiety with two geminal cyano groups (DCV) and easy synthesis. As compared to the parent rhodamine, dicyanorhodanine imparts higher ICT and makes the π-conjugated system more electron deficient. Furthermore, the dicyanorhodanine-based semiconductors show small crystal domain size, uniform morphology, and smoother blend film surface, which is advantageous for efficient charge transport and improved efficiency of organic photovoltaic devices.37 For instance, a quadrupolar system 17 (Fig. 7) based on a fluorene core, two benzothiadiazole linkers, and two peripheral dicyanorhodanines was investigated.62 It showed pronounced absorption and lowered LUMO energy, and its blend with polymeric donor (PffBT4T-2OD) afforded the OSC with the PCE of 8.4%, FF of 70.7%, and external quantum efficiency (EQE) of 84% (Table 5). The π-conjugated backbone of 17 has further been extended by two furan units, as shown for 18. Its film with PTB7-Th polymeric donor has been used in OSC with an improved PCE of 10.7%.63 IDT core, similar to 16, flanked by two thiophene-fused benzothiadiazole units and terminal dicyanorhodanines was used as molecular acceptor 19 by Xu et al. (Fig. 7).64 This derivative possesses a reduced band gap (1.92 eV) because of efficient ICT between the dicyanorhodamine and the central auxiliary thiophene donors, and its photovoltaic performance with PTB7-Th donor reached 9.07% (PCE) and short circuit current density (Jsc) over 20.33 mA cm−2.
 |
| Fig. 7 Dicyano rhodamine derived molecular acceptors. | |
Table 5 Optoelectronic parameters of dicyano rhodanine derivatives 17–19
Acceptor |
λ
max
A (nm eV−1) |
E
g (eV) |
LUMO (eV) |
Donor |
PCE (%) |
Ref. |
17
|
498/2.49 |
2.16 |
−3.55 |
PffBT4T-2OD |
8.40 |
62
|
18
|
522/2.38 |
2.07 |
−3.81 |
PTB7-TH |
10.70 |
63
|
19
|
770/1.61 |
1.92 |
−3.78 |
PTB7-TH |
9.07 |
64
|
Organic light-emitting diodes
The recent rapid development of organic electronics is also due to remarkable progress in the design, preparation, and understanding of organic light-emitting semiconductors. Organic semiconducting materials possess a variety of advantageous properties, including low density, easy and inexpensive preparation and structural tuning and solution processibility that were successfully utilized in organic solid-state lasers,65 organic light-emitting diodes (OLED),66 organic field-effect transistors,67 smart materials,68 biological imaging,69 biochemical sensing70 and many others. Since the first OLED reported by Kodak in 1987,71 this sandwich technology has evolved into commercially very successful and popular devices. The OLED utilizes the principle of electroluminescence – a radiative recombination of electrons and holes in a semiconducting material, releasing the excess of energy as photons. The organic materials used in OLEDs involve hole/electron-injection/transport layers, electron/hole-blocking layers, and most importantly, organic emitting layers. According to the exciton harvesting mechanism, the current organic emitters can be divided into three main generations, including fluorescent, phosphorescent, and thermally-activated delayed fluorophores (TADF).72 CN-substituted small-molecules with D–A arrangement and their TADF properties were reviewed by Cao et al.73 Mono/multicyano-substituted benzenes, mono/dicyano-derived aromatic N-heterocycles, and acrylonitriles were covered, and their structure–property relationships were systematically discussed. This work provides an important reference and guidance for the current development of TADF materials containing CN group(s) and their manifold applications. A typical organic emitter consists of a π-conjugated backbone functionalized with both electron-withdrawing/releasing substituents and moieties, allowing precise color-tuning. In this respect, the cyano group proved to be a very useful linear acceptor. N,N-Diphenylamino and stilbene moieties are very common structural features of OLED's fluorescent materials, e.g., see derivative 20 in Fig. 8. This CN-functionalized red-emitting dye has been revealed as a bipolar material allowing efficient charge injection/transport as well as recombination. A double-layered OLED using 20 showed a luminance of 3290 cd m−2 at the current density of 100 mA cm−2 and EQE of 1.1%.74 A very similar quadrupolar (D–π–)2A derivative 21 based on central dicyanonaphthalene core has been investigated by Hung et al.75 An OLED based solely on 21 displayed luminous efficiency of 2.8 cd A−1 and EQE of 0.80%. Biphenylene scaffold 22 decorated by N,N-diphenylamino donor and phenanthroimidazole acceptor has been investigated by Zhang et al.76 The imidazole has been further N-substituted by 4-cyanophenyl moiety, which significantly improved EQE up to 7.8% (Table 6) by modulating the emissive state. The OLED based on 22 showed luminous efficiency of 10.5 cd A−1 and emitted light with the CIE coordinates (0.16, 0.16) corresponding to a pure blue. Anthracene is another prominent π-conjugated scaffold used in the construction of fluorophores. D–π–A derivative 23 (Fig. 8) utilizing methoxy donor and cyano acceptor showed the highest luminous efficiency (6.1 cd A−1 at 2 mA cm−1) across related derivatives. Moreover, the CIE coordinates (0.15, 0.06; Table 6) of 23 match well with the HDTV standard blue.77 Spiro compounds are another popular group of sterically demanding emitters, and derivative 24 combining benzanthrone and fluorene moieties and two peripheral CN groups is a typical example. It has been shown that its LUMO occupies both the central π-system and the cyanophenyl moieties, and 24 possesses CT character. The OLED based on 24 showed luminous efficiency of 10.3 cd A−1 and an EQE of 10.2% at a constant luminance of 1000 cd m−2. Compound 24 is a highly efficient deep blue emitter with the CIE coordinates (0.14, 0.11).78 DCV moiety is less employed in organic emitters as compared to single-CN derivatives; cyclohexane- and pyran-4-one-derived compounds 25 and 26 represent rather simple push–pull molecules.79,80 For instance, an OLED device built on 26 showed luminous efficiency of 4.4 cd A−1 and CIE coordinates (0.65, 0.35) (Table 6).
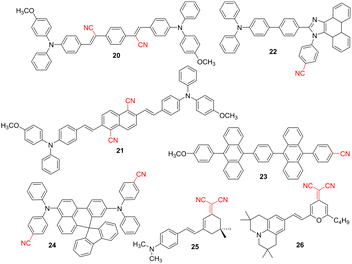 |
| Fig. 8 The molecular structure of representative CN-substituted OLED materials. | |
Table 6 Fundamental parameters of CN-capped OLED materials 20–26
Emitter |
λ
max
A (nm eV−1) |
λ
max
E (nm eV−1) |
CIEx;y |
EQE (%) |
Ref. |
20
|
— |
598/2.07 |
— |
1.10 |
74
|
21
|
507/2.45 |
630/1.97 |
(0.63, 0.37) |
0.80 |
75
|
22
|
ca. 360/3.44 |
445/2.79 |
(0.16, 0.16) |
7.80 |
76
|
23
|
405/3.06 |
452/2.74 |
(0.15, 0.06) |
12.00 |
77
|
24
|
400/3.10 |
450/2.76 |
(0.14, 0.11) |
10.20 |
78
|
25
|
500/2.48 |
650/1.91 |
— |
— |
79
|
26
|
520/2.38 |
615/2.02 |
(0.65, 0.35) |
— |
80
|
Organic nonlinear-optical materials
Since the discovery of laser in 1960, various nonlinear optical (NLO) phenomena, such as second/third-harmonic generation, two-photon absorption, amplification, scattering, conversion, oscillation, modulation, etc., have been experimentally observed.81–95 Along with these fundamental discoveries, the NLO community also became very interested in organic materials with pronounced NLO activity both at the molecular and supramolecular levels.96,97 It has been realized shortly that traditional inorganic crystalline materials can be completed/substituted by organic molecules with distinct properties such as flexibility, facile property tuning, solution processability, and inexpensive preparation. A general drawback of organic molecules is their centrosymmetric supramolecular arrangement, which, however, can be suppressed by proper structural tuning.2,98,99 Due to its negative mesomeric effect, the CN group shows strong D–A interaction if interconnected to an electron-donor via a π-conjugated system (D–π–A system). Hence, CN-capped push–pull chromophores have been tremendously investigated as active NLOphores. D–π–A arrangement brings pronounced ICT, dipolar character, and red-shifted absorption – fundamental aspects of an NLO active substance. The current trends in developing new NLO-active organic molecules involve multichromophoric compounds having extraordinary arrangements inspired by the alphabet.100 Hence, various H-, L-, T-, V-, X-, and Y-shaped NLOphores were developed to date. The most popular tripodal Y-shaped molecules can be built either on imidazole101 or triphenylamine (TPA) cores terminated by three acceptors. For instance, we have decorated TPA core by systematically evaluated CN-acceptors to tailor absorption/emissive maxima, HOMO–LUMO gap, two-photon absorption cross-section as well as thermal robustness (Table 7).102 Hence, tripodal Y-shaped molecules 27a–l (Fig. 9) bearing peripheral single CN-substituted, dicyanovinyl, and dicyanobenzene/imidazole/thiophene moieties and varied π-linkers proved to be tunable NLOphores allowing elucidation of fundamental structure–property relationships. 4-Cyanostyryl (e) and 2,6-dicyanophenylethynyl (i) units turned out to be the most efficient peripheral acceptors in terms of the achieved two-photon absorption cross-sections.
Table 7 Fundamental properties of CN-capped TPA chromophores 27a–l102
NLOphore |
λ
max
A
(nm eV−1) |
λ
max
E
(nm eV−1) |
Φ
F
(%) |
HOMO–LUMOd gap (eV) |
δ
2PA
(GM) |
The longest-wavelength absorption maxima measured in THF.
The emission maxima measured in THF.
Fluorescence quantum yield in THF.
The electrochemically determined HOMO–LUMO gap in acetonitrile.
Two-photo absorption cross-section (the wavelength of the maximum 2 PA cross-section is shown in parenthesis).
|
27a
|
339/3.66 |
377/3.29 |
0.62 |
3.50 |
— |
27b
|
394/3.15 |
460/2.70 |
0.48 |
2.86 |
260 (770) |
27c
|
372/3.33 |
448/2.77 |
0.64 |
3.04 |
566 (760) |
27d
|
387/3.20 |
458/2.71 |
0.59 |
2.89 |
785 (780) |
27e
|
403/3.08 |
510/2.43 |
0.51 |
2.65 |
1100 (810) |
27f
|
453/2.74 |
549/2.26 |
0.29 |
— |
757 (830) |
27g
|
470/2.64 |
611/2.03 |
0.29 |
— |
667 (810) |
27h
|
413/3.00 |
497/2.49 |
0.59 |
2.63 |
620 (810) |
27i
|
420/2.95 |
533/2.33 |
0.69 |
2.71 |
852 (810) |
27j
|
414/3.00 |
499/2.48 |
0.55 |
2.59 |
631 (810) |
27k
|
397/3.12 |
475/2.61 |
0.46 |
2.54 |
148 (750) |
27l
|
412/3.01 |
516/2.40 |
0.49 |
2.58 |
375 (830) |
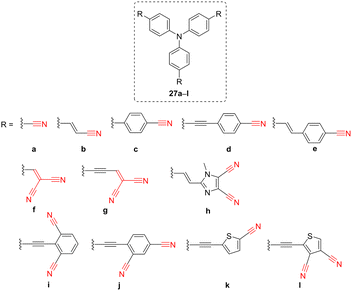 |
| Fig. 9 Property tuning in CN-capped tripodal NLOphores based on triphenylamine. | |
 |
| Fig. 10 Dicyanoimidazole, dicyanobenzene and dicyanopyrazine-derived Y- and X-shaped chromophores. | |
Dicyanoimidazole (DCI), dicyanobenzene (DCB), and dicyanopyrazine (DCP) are other popular aromatic (hetero)cyclic electron-withdrawing moieties of NLO active compounds 28–30. DCI has been used in the construction of Y-shaped imidazoles 28 bearing various electron donors D such as methoxy, N,N-dimethylamino or ferrocenyl and systematically enlarged π-linker.103–107 These structural changes allowed to shift the longest-wavelength absorption maxima by up to 70 nm, narrowed the HOMO–LUMO gap by more than 1.1 eV, and tuned the second-order NLO response within the range of 19–40 × 10−30 esu as measured by hyper-Rayleigh scattering at 1064 in dichloromethane. Isolobal X-shaped derivatives 29 and 30 were built on DCB/DCP cores by attaching various π-linkers, which tuned their optical and electrochemical gaps within the range of 335–458/354–499 nm and 2.54–1.87/2.26–1.50 eV, respectively.108,109 The measured second-order polarizabilities β were generally larger for pyrazines but the effect of stronger acceptor diminishes with gradual π-linker extension.
2-(3-Cyano-4,5,5-trimethylfuran-2(5H)-ylidene)malononitrile (TCF) is another very powerful and popular acceptor combining three CN groups. It has been utilized in representative Y-shaped TPA chromophores 31a, 31b (Fig. 11). Further property tuning has been achieved by furan or thiophene linkers, and both chromophores showed absorption within the region of 610–630 nm, good optical nonlinearity, and thermal stability (Table 8).110
 |
| Fig. 11 Representative TCF-functionalized NLOphores. | |
Table 8 Fundamental properties of NLOphores 31–34
NLOphore |
λ
max
A (nm eV−1) |
HOMO–LUMO gap (eV) |
β
max/(10−30 esu) |
r
33
/(pm V−1) |
Ref. |
λ
max
A was measured in CHCl3.
λ
max
A was measured in the solution of THF.
β values were measured by Hyper-Rayleigh Scattering technique in CHCl3 using the fundamental excitation wavelength of 1064 nm.
β values were calculated using Gaussian 09 at the CAM-B3LYP/6-31+G* level and the direction of the maximum value is directed along the charge transfer axis of the chromophores.
The β components were calculated using Gaussian 09 and dynamic (at the 1310 nm) average β values were measured.
β values were calculated using Gaussian 03 at the B3LYP/6-31 g(d) level and the direction of the maximum value is directed along the charge transfer axis of the chromophores.
r
33 values were measured at a wavelength of 1310 nm.
|
31a
|
621a/2.00 |
— |
1023.00c |
— |
110
|
31b
|
618a/2.01 |
— |
1091.00c |
— |
110
|
32a
|
599a/2.07 |
2.39 |
329.90d |
31.00 |
111
|
32b
|
659a/1.88 |
2.17 |
458.87d |
47.00 |
111
|
32c
|
659a/1.88 |
1.86 |
962.94d |
72.00 |
111
|
32d
|
730a/1.70 |
1.68 |
1561.80d |
95.00 |
111
|
33a
|
309b/4.01 |
— |
106.40e |
10.60 |
112
|
33b
|
550b/2.25 |
— |
798.80e |
23.50 |
112
|
34a
|
725a/1.71 |
1.963 |
713.00f |
149.00 |
113
|
34b
|
750a/1.65 |
1.766 |
995.00f |
143.00 |
113
|
Yang et al. designed a series of linear push–pull molecules 32 with either bridged diaminophenothiazine-π-TCF (a/c) or diaminophenyl-π-TCF (b/d) arrangement (Fig. 11). It has been revealed that how the bridging and extension of the π-system affect absorption maxima, NLO and thermal properties (Table 8). Based on these findings, derivatives 32a–d proved to be promising high-performance organic electro-optic and photorefractive materials.111 TCF is also a very popular acceptor unit of small indole-derived push–pull molecules such as 33a, 33b. It has been shown that further substitution of the indole by pyrrolidine donor red-shifts the absorption maxima and drastically enhances the second-order NLO response (Table 8) as well as increases charge mobility.112 Yang et al. interconnected Michler's ketone moiety to TCF to gain simple push–pull chromophores 34a, 34b (Fig. 11). Along with planar and polarizable olefinic/2,5-thienylene π-backbone; these chromophores possess enhanced macroscopic electro-optic coefficients (Table 8).113
TCF-functionalized molecules were used to construct anionic polymethine salts 35a–c (Fig. 12) bearing phosphonium counterions.114 Whereas (non)linear optical properties of mono- and dicationic compounds 35a, 35b were strongly dependent on the concentration (due to aggregation), hexacationic complex 35c significantly resisted intermolecular interaction at higher concentrations. Its macroscopic third-order nonlinearity measured by the Z-scan technique (1.55 μm) in the neat film is 3.6 × 10−11 esu, which is larger than that measured for 35a, 35b (2.3 and 3.4 × 10−11 esu). Hence, a strong intramolecular anion–cation interaction in 35c provides a steric repulsive effect diminishing intermolecular interactions, which results in an efficient translation between the microscopic and macroscopic polarizabilities.
 |
| Fig. 12 Chemical structure of anionic TCF-polymethine salts 35a–c, including the corresponding phosphonium cations. | |
Light conversion agents
CN-capped molecules can also be utilized as light conversion agents that are especially useful to convert unproductive yellow-green (510–580 nm) and ultraviolet light (280–380 nm) into red-orange (600–700 nm) and blue-violet light (400–480 nm) that are essential wavelengths for photosynthesis. There are three types of light conversion agents,115–122 including (i) rare-earth sulfides, (ii) rare-earth complexes bearing organic ligands (mostly Eu3+ and Sm3+ complexes), and (iii) pure organic fluorescent dyes. Rare-earth sulfides possess very good stability, but they also tend to deliquescence and disperse, and their fabrication requires high-temperature sintering. Rare-earth complexes possess stronger fluorescence and generally better compatibility with a polymer matrix, but these complexes fail to convert yellow-green into red-orange light. In principle, organic fluorescent dyes are well-soluble in organic resins and possess fluorescence emission bands easily adjustable to an absorption spectrum of different crops. Hence, inexpensive and easy-to-obtain agricultural films can be produced. The organic dye used as a light conversion agent should possess pronounced emissive properties and high fluorescence quantum yield along with none or minor quenching upon increasing its concentration/embedding in a matrix. The organic dye should also withstand long-term exposure to solar radiation and not undergo degradation and oxidation. Moreover, molecular optical properties measured in a solution are often not fully mirrored in a polymeric matrix, which may also result from diminished mechanical and chemical stability upon doping with the selected light conversion agent. Aggregation-induced emission (AIE) and TADF were utilized as two leading mechanisms in the design of organic light conversion agents.123 Variety of π-conjugated organic scaffolds were already examined, with triphenylacrylonitrile proving to be a benchmark compound. For instance, Wang et al. have optimized the parent triphenylacrylonitrile backbone by attaching additional aryls at positions 3 and 4 leading to derivatives 36a–e (Fig. 13). These highly efficient blue-violet light conversion agents, biphenyl derivative 36e in particular, exhibited crystallization-induced emission accompanied by a strong fluorescence (ΦF of 0.833 and 0.107 in the solid state and film, respectively; Table 9). Ring-closing oxidation has been disclosed to be the main mechanism, reducing the fluorescence intensity, especially during summer.124
 |
| Fig. 13 Triphenylacrylonitrile family of light conversion agents. | |
Table 9 Emissive properties of light conversion agents 36–38 for agricultural films
Light conversion agent |
λ
max
E (nm) |
Φ
F (%) (solid state) |
Φ
F (%) (film) |
Ref. |
36a
|
ca. 385 |
57.60 |
5.40 |
124
|
36b
|
ca. 389 |
0.50 |
2.70 |
124
|
36c
|
ca. 375 |
0.40 |
2.90 |
124
|
36d
|
ca. 410 |
30.80 |
4.20 |
124
|
36e
|
ca. 400 |
83.30 |
10.70 |
124
|
Triphenylacrylonitrile |
430 |
22.40 |
1.90 |
124/125 |
37a
|
454 |
— |
15.00 |
126
|
37b
|
457 |
— |
50.00 |
126
|
37c
|
455 |
— |
18.00 |
126
|
37d
|
— |
— |
— |
126
|
37e
|
449 |
— |
65.00 |
126
|
TPE
|
478 |
25.32 |
— |
125
|
38a
|
493 |
80.00 |
55.00 |
128
|
38b
|
485 |
83.00 |
52.00 |
128
|
Tetraphenylethene (TPE) is another prominent scaffold used to construct organic light conversion agents. For instance, Qi et al. compared parent triphenylacrylonitrile with three TPE derivatives bearing carbazole peripheral donors, and it turned out that triphenylacrylonitrile outperformed all these derivatives in terms of excellent photostability and ultraviolet light conversion properties.125
Carbazole-functionalized cyanostilbene 37a–d (Fig. 14) and structurally related phenanthrenecarbonitrile 37e were investigated by Wang.126 These luminophores showed various extents of AIE and E/Z isomerization of the central double bond. It was revealed that stilbenes 37a–d are prone to Michael addition and intramolecular photocyclization reactions. In contrast, phenanthrenecarbonitrile 37e does not undergo photocyclization, shows strong fluorescence in both the solid state and the film, and has high light-conversion efficiency (Table 9). Its high photostability is ascribed to a reduced electron density over the π-system due to the attached CN group, which decreases the electrophilicity of the whole system and its capability to accept electrons (an opposite effect of the carbazole moiety).127 Anthracene-functionalized cyanostilbenes 38a, 38b were investigated for their polymorphism and potential application as light conversion agents for agricultural films. Especially isomer 38b showed excellent matching of its fluorescence maxima (485 nm, Table 9), high fluorescence efficiency, and excellent photostability. The films doped with 38b retained 94% of their initial intensity upon UV irradiation for 20 h.128
 |
| Fig. 14 Cyanostilbene and phenanthrenecarbonitrile light conversion agents. | |
Supramolecular arrangement is another aspect to be considered when designing a light conversion agent. For instance, Yang et al. studied simple phenothiazine N-substituted benzonitrile, whose molecular packing significantly affected its room temperature phosphorescence (RTP). This simple luminogen crystallizes in three different crystalline polymorphs possessing different RTP lifetimes and photoluminescence quantum yields.129 The molecular packing and AIE theory should be considered more broadly in the design of organic CN-functionalized molecules, as these may significantly affect general optoelectronic performance.130,131
Organic sensors and probes
Accurate, quick, and easy detection of various potentially hazardous analytes is of utmost importance to analytical and materials chemistry and imaging/biomedical techniques. In general, the detection should be sensitive with a low limit of detection (LOD), specific, non-invasive, low-cost, and should use non-ionizing irradiation. In this respect, colorimetric and fluorometric methods are well-established and widely used even for real-time monitoring. Fluorescence sensing technology has developed rapidly both in vivo and in vitro, and various fluorescent probes were recently developed, with many of them being biocompatible. Organic fluorescent probes are being routinely used as a general means to monitor molecular interactions across biochemistry, medicine, environmental science and industry.132 These probes are usually composed of a prominent π-conjugated backbone/chromophore such as coumarin, xanthene (e.g., fluorescein, eosin Y or rhodamine), porphyrin, phthalocyanine, cyanine, naphthalimide, fluoropyrrole, etc. along with a recognition acceptor and a spacer.133–135 Cyano group connected to a π-conjugated system transmits its negative inductive and mesomeric effects that significantly influence absorption and emission properties. Hence, CN-capped colorimetric and fluorometric probes constitute another interesting class of organic molecules covered in this review. In principle, both electrophilic and nucleophilic species can be detected by CN-capped probes. For instance, Mu et al. developed a dual-response fluorescent probe 39 (Scheme 2) based on a rhodamine B scaffold functionalized by DCV recognition moiety. This probe can selectively detect hydrazine and cyanide, both environmentally and biologically relevant analytes. Whereas hydrazine detection is based on a replacement of malononitrile and the formation of hydrazone, cyanide adds to the DCV moiety. Both chemistries are accompanied by specific and easy-to-recognize changes in the emissive properties of the originally red-fluorescent probe 39. Yellow or blue fluorescent products are formed upon the reaction with hydrazine or cyanide.136
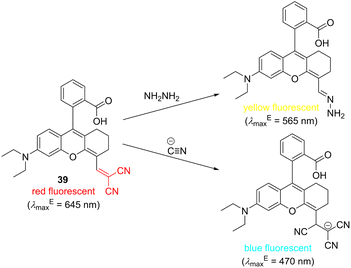 |
| Scheme 2 Dual-response probe 39 based on rhodamine B equipped with DCV moiety. | |
Chemically identical detection mechanism of cyanide anions has also been utilized in D–π–A probe 40 (Scheme 3), utilizing both colorimetric and turn-on fluorescent sensing. The originally violet (amaranth) probe changes to yellow, allowing naked-eye detection of cyanide ions with the LOD of 1.4 × 10−8 M (Table 10). Moreover, the nucleophilic attack on the DCV moiety interrupts efficient ICT from the N,N-dimethylamino donor and turns on fluorescence appearing at 551 nm. A high selectivity and sensitivity towards cyanide ions over a wide range of competitive anions have been demonstrated. DCV-derivative 40 possesses low toxicity and has also been successfully applied in the bioimaging of living cells.137
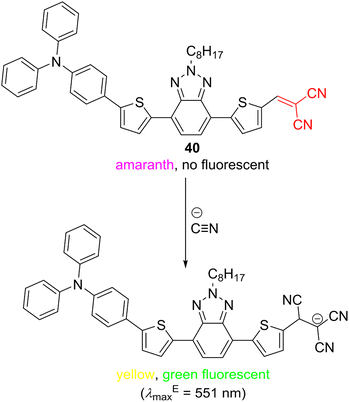 |
| Scheme 3 Colorimetric and fluorometric detection of cyanide ions using probe 40. | |
Table 10 Overview of sensed species and LODs of organic fluorescent probes 39–44
Probe |
Sensed species 1 |
LOD (μM) |
Sensing species 2 |
LOD (μM) |
Ref. |
39
|
N2H4 |
0.08 |
N |
0.33 |
136
|
40
|
N |
0.014 |
— |
— |
137
|
41
|
N |
1.44 |
— |
— |
139
|
42
|
N |
0.0424 |
— |
— |
143
|
43
|
Fe3+ |
0.17 |
N |
0.39 |
146
|
44
|
Cu2+ |
0.03 |
Hg2+ |
0.16 |
147
|
45
|
DCP |
0.20 |
DCNP |
0.26 |
148
|
Dicyanomethylene-4H-pyran (DCMP) is another widely employed moiety in organic fluorescent probes due to its peculiar optical properties, often reaching NIR region, large Stokes shifts, and photostability.138 DCMP functionalized with thiophen-2-yl styrylcarboxylate 41 (Scheme 4) has been used to selectively detect cyanide ions even in the presence of interfering ions and small molecules. Whereas the original yellow probe 41 is not emissive, hydroxy derivative formed upon a hydrolytic cleavage of the ester function mediated by cyanide ions is green and possesses emission in the NIR region. Sensor 41 was found to be efficient in detecting cyanide in the intracellular region of MCF-7 cells139 with the LOD of 1.44 × 10−6 M (Table 10).
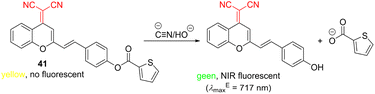 |
| Scheme 4 DCMP-derived sensor of cyanide ions utilizing cleavage of ester function. | |
Sensing methodologies of cyanide ions based on their nucleophilic addition reactions are significantly facilitated by the CN-functionalization of the probe structure.140–142 Besides popular DCV-derived sensors, cyanovinyl analogue such as 42 are also feasible (Scheme 5). This push–pull molecule undergoes a nucleophilic addition solely with cyanide ions (LOD = 4.24 × 10−8 M, Table 10), leading to an interrupted ICT (decolorization) and pronounced emission at 560 nm. Furthermore, the detection of cyanide ions in living cells was possible due to low cytotoxicity of 42.143
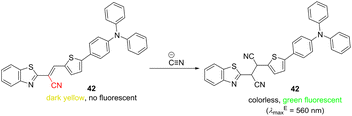 |
| Scheme 5 Single cyano-capped probe 42 for detection of cyanide ions. | |
Besides nucleophiles, various electrophiles, mostly metal cations, can also be sensed by CN-capped organic probes. AIE-active molecules are increasingly popular in this respect.144,145 For instance, simple DCV derivative 43, prepared by Knoevenagel condensation of malononitrile and N,N-diethylaminobenzaldehyde, can be used for the detection of either cyanide anions or Fe3+ cations (Scheme 6). The original aggregated orange and fluorescent 43 was selectively decolorized by both analytes, accompanied by quenching/changing of the original emissive properties. The latter is due to the destruction of the original emissive aggregates either by producing a less rigid structure upon attaching the third CN group or by coordinating Fe3+ ions via DCV moiety and thus altering its electronic properties. It should be noted that the probe did not respond to Fe2+ ions.146
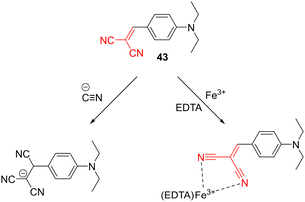 |
| Scheme 6 Dual responsive probe 43 utilizing quenching/reduction of AIE as a detection mechanism. | |
We have screened push–pull compound 44 for sensing various metal cations (Scheme 7), and it turned out that only Hg2+ and Cu2+ ions induce spectral changes. The original yellow color of 44 is decolorized/red-shifted in the presence of Hg2+/Cu2+. Whereas fluorescence properties persisted upon treatment with Hg2+, no emission was observed for 44 and Cu2+. Investigation of the sensing mechanism revealed N–S coordination of Hg2+ to imidazole/thiophene donor part, whereas Cu2+ in aqueous media induced hydrolysis of one of the CN groups present at the DCI moiety and subsequent formation of an imino derivative (IPIMO). In contrast to Cu2+, Cu+ was inefficient in inducing such transformation. Hence, interrupting/enhancing efficient ICT in 44 resulted in either hypsochromic or bathochromic shifts of the absorption spectra accompanied by persisted or diminished emission. The LODs towards both ions are 0.16 and 0.03 × 10−6 M (Table 10). Moreover, paper strips coated with 44 were developed for a fast track detection of both ions in real samples with the visualization either by naked-eye or handheld UV-lamp.147
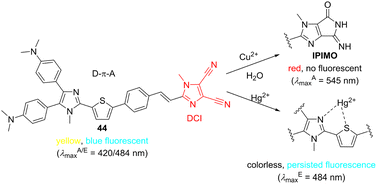 |
| Scheme 7 Dicyanoimidazole D–π–A system capable of selectively detecting Cu2+ and Hg2+ ions. | |
Sensing of nerve gases is another important task to be addressed by organic probes. Y-shaped CN-capped NH-imidazole push–pull derivative 45 (Scheme 8) was revealed to detect sarin and tabun mimics DCP and DCNP very selectively. The originally weakly emissive aggregates of 45 react quickly with DCP/DCNP in terms of nucleophilic substitution, affording highly emissive N-phosphorylated adduct, which underwent slow hydrolysis in aqueous media to 45H+ and nontoxic phosphoric acid derivative. Hence, 45 is able to detect and detoxify both mimics of nerve gases with the LODs of 0.20 and 0.26 × 10−6 M (Table 10), respectively.148
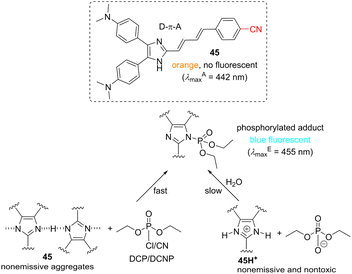 |
| Scheme 8 Molecular structure of sensor 45 and its mechanism of detection and detoxification of nerve gases. | |
Photoredox catalysis
The use of visible light in organic synthesis is considered a green and sustainable source of energy, allowing radical processes that recently came back to the foreground as photoredox catalysis. Its renaissance has been due to synthetically attractive visible light-initiated radical transformations that can be accomplished at very mild reaction conditions. The current photoredox catalysis relies on a suitable catalyst, which undergoes facile excitation by visible light to yield a strong one-electron reducing or oxidizing reagent. Ru- and Ir-polypyridyl complexes are traditional and efficient photoredox catalysts but their use can be, in particular cases, limited by the presence of heavy metals and their high costs.149–151 In this respect, organic photoredox catalysis seems to be a greener way of utilizing purely organic catalysts such as xanthene dyes (e.g., Eosin Y, Fluorescein, Rose Bengal), flavins, perylenediimide, acridiniums, and last but not the least dicyano(hetero)arenes. The latter cyano-capped synthetic dyes attract particular attention due to their easily tunable properties.152–154Fig. 15 shows the molecular structure of typical CN-substituted aromatic (hetero)cycles, including two major groups – dicyanobenzene/naphthalene/anthracene 46–50 and dicyanopyrazines 51. Their fundamental photophysical properties are summarized in Table 11. The absorption maxima are important parameters used to fit the catalyst with a suitable light source, whereas the ground- and excited-state electrochemical properties are crucial to determine the fundamental redox potentials and subsequently estimate the feasibility of a desired photoredox process.
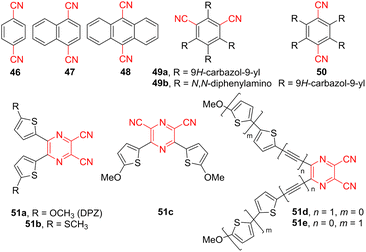 |
| Fig. 15 The molecular structure of CN-capped photoredox catalysts – cyanoarenes 46–50 and DPZs 51. | |
Table 11 Fundamental photophysical properties of photoredox catalysts 46–51
Catalysts |
λ
max
A (nm eV−1) |
E
0,0 (eV) |
Ground state redox potentials (V vs. SCE) |
Excited state redox potentialsa (V vs. SCE) |
Ref. |
E
1/2(red)
|
E
1/2(ox)
|

|

|
Singlet excited state redox potentials.
|
46
|
290/4.28 |
4.01 |
−1.46 |
— |
+2.55 |
— |
153
|
47
|
325/3.82 |
3.57 |
−1.27 |
— |
+2.30 |
— |
153
|
48
|
422/2.94 |
2.90 |
−0.91 |
— |
+1.99 |
— |
153
|
49a
|
435/2.85 |
2.56 |
−1.04 |
+1.35 |
+1.52 |
−1.21 |
153
|
49b
|
425/2.92 |
2.62 |
−1.28 |
+1.10 |
+1.34 |
−1.52 |
153
|
50
|
463/2.68 |
2.43 |
−0.99 |
+1.41 |
+1.44 |
−1.02 |
153
|
51a
|
440/2.82 |
2.50 |
−1.14 |
+1.32 |
+1.36 |
−1.18 |
160
|
51b
|
443/2.79 |
2.22 |
−1.01 |
+1.32 |
+1.21 |
−0.90 |
160
|
51c
|
389/3.19 |
2.70 |
−1.15 |
+1.57 |
+1.55 |
−1.13 |
160
|
51d
|
459/2.70 |
2.59 |
−0.80 |
+1.46 |
+1.82 |
−1.16 |
161
|
51e
|
511/2.43 |
— |
−0.95 |
+1.11 |
— |
— |
161
|
Photophysics of cyanoarenes is well investigated, and compounds 46–48 represent photoredox catalysts enabling electron transfer from the singlet excited state. Due to their high ground state reduction potentials ranging from −1.46 to −0.91 V (Table 11), these simple dinitriles also possess high singlet excited energies E0,0. Extended cyanoarenes (e.g., 46vs.48) are better suited to photoredox catalysis due to their red-shifted absorption maxima and longer lifetimes of the singlet excited state. Amino-substituted dicyanobenzenes 49 and 50 bearing either 9H-carbazol-9-yl or N,N-diphenylamino donors are relatively new D–π–A photoredox catalysts.155 Especially catalyst 49a (4CzIPN) has been thoroughly explored in a plethora of photoredox transformations.156 Its further structural tuning by replacing the donor or isomerization afforded derivatives 49b and 50.157 A replacement of carbazole by N,N-diphenylamino group (49a→49b) led to lowered redox potentials (−1.28 and +1.10 V, Table 11), whereas isomerization of 49a to 50 slightly increased the redox potentials and red-shifted the absorption maxima to 463 nm. 5,6-Disubstituted pyrazine-2,3-dicarbonitriles 51a–e (DPZ, Fig. 15) represent the family of heteroaromatic photoredox catalysts with two cyano acceptors and two methoxy/methylthiothiophene donors recently developed in our group.158 These catalysts evolved from dicyanopyrazine NLOphores 30 (Fig. 10) by further structural tuning. Especially the parent DPZ catalyst 51a showed very broad application potential across various photochemical transformations, including C–C bond formation,159–163 enantioselective photoreduction,164,165 pH controlled photooxygenation of indoles,166etc. Its structure has been further systematically modified by varying the peripheral donor (OMe → SMe, 51a→51b), which affected the reduction/oxidation potentials of the excited state, and subsequently, also the excited state energy. Isomerization of substituents along the pyrazine core (51a→51c) significantly influenced the chromophore arrangement resulting in the merging of two original absorption bands (λmaxA = 440 and 360 nm) seen for 51a into a single band of 51c appearing at 389 nm. Further extension and planarization of the π-system by additional acetylenic spacers, as in 51d resulted in slightly red-shifted absorption and increased E0,0. The attachment of methoxy-substituted bithiophene linker (51e) significantly supported the ICT, suppressed emissive properties, and red-shifted the absorption maxima to 511 nm. Hence, structural variation of CN-capped aromatic (hetero)cycles 46–51 allowed extensive tuning of their photoredox properties.
Conclusions
Due to its negative inductive and mesomeric effects, the cyano group can significantly alter the electronic properties of π-conjugated systems and CN can be denoted as a linear and increasingly popular electron acceptor. Six fundamental groups of CN-capped small organic materials were reviewed, including materials applicable in OSCs, OLEDs, NLO, light conversion agents, organic sensors and probes, and photoredox catalysis. Semiconductors used as acceptors in solar cells may contain either single-CN group(s) or DCV moiety. The latter is often part of larger scaffolds such as (F)IC or dicyanorhodanine with pronounced electron-withdrawing properties. The cyano-capped semiconductors usually possess reduced LUMO level, narrowed bandgap, and red-shifted absorption and thus enhanced device efficiency with the PCE reaching up to 12%. Organic emitters for OLEDs can be easily tuned by attaching CN groups, either single DCV moiety, and achieve a device with improved luminance, well-tailored color, and EQE up to 12%. Cyano-substituted push–pull molecules possess long-lasting tradition in nonlinear optics; a variety of novel and complex CN-based acceptors were recently developed, including cyanoaryls, dicyanoimidazole, (di)cyanothiophene, dicyanopyrazine/benzene, tricyanofuran, etc. These moieties allow the construction of D–π–A systems with the ICT of a large extent and thus of different NLO responses. Nowadays, organic light conversion agents are a burgeoning area of π-conjugated compounds used to produce agricultural films affecting plant growth. Triphenylacrylonitrile and related cyanostilbenes are the most popular CN-capped molecules capable of converting unproductive ultraviolet and yellow-green wavelengths into red-orange and blue-violet light. Organic sensors and probes are being used to detect various analytes. For instance, DCV-substituted molecules undergo facile addition of cyanide ions resulting in a product with different optical properties. Hence, colorimetric and fluorometric detection of this environmentally important analyte is possible. DCI-based push–pull molecules proved to be very selective detectors of Cu2+ ions, and CN-capped imidazoles also detect and detoxify nerve gases. The group of cyano-substituted compounds is cyanoarenes and X-shaped dicyanopyrazines, which were recently applied as very efficient and pure organic photoredox catalysts. These synthetic dyes possess tunable structures and properties, and their visible light-induced catalytic activity has been demonstrated in various organic transformations.
The aforementioned examples clearly revealed organic CN-capped compounds as versatile organic materials, and attaching the cyano group represents well-established structural tuning used across various branches of the current materials chemistry.
Author contributions
H. W., C. Z. and J. L.: conceptualization. H. W., C. Z. and Z. B.: data curation. H. W., F. B. and J. L.: formal analysis. F. B. and J. L.: funding acquisition. H. W., C. Z., Z. B. and F. B.: investigation. F. B. and J. L.: project administration. J. L.: supervision. H. W. and F. B.: writing-original draft. H. W., C. Z., Z. B., F. B. and J. L.: writing-review & editing.
Conflicts of interest
There are no conflicts to declare.
Note added after first publication
This article replaces the version published on 31st January 2023, which contained an incorrect version of Fig. 10.
Acknowledgements
This work was supported by the Science and technology project of Inner Mongolia Autonomous Region (2021GG0063), Finance Science and Technology Project of Hainan Province (No. ZDYF2020084), Central Public-interest Scientific Institution Basal Research Fund (No. BSRF202105), Key scientific and technological projects of Xinjiang Production and Construction Corps (No. 2021AB007), Science and Technology Project of the Ninth Agricultural Division of Xinjiang Production and Construction Corps (2020JS015), and Changzhou Science and Technology Program (No. CE20202022). ZB and FB are indebted to the Czech Science Foundation (22-14988S).
Notes and references
- F. Bureš, Chem. Listy, 2013, 107, 834–842 Search PubMed.
- F. Bureš, RSC Adv., 2014, 4, 58826–58851 RSC.
- Y. Wu and W. Zhu, Chem. Soc. Rev., 2013, 42, 2039–2058 RSC.
- H. Cha, H. N. Kim, T. K. An, M. S. Kang, S.-K. Kwon, Y.-H. Kim and C. E. Park, ACS Appl. Mater. Interfaces, 2014, 6, 15774–15782 CrossRef CAS PubMed.
- H. Li, M. Fang, Y. Hou, R. Tang, Y. Yang, C. Zhong, Q. Li and Z. Li, ACS Appl. Mater. Interfaces, 2016, 8, 12134–12140 CrossRef CAS PubMed.
- X. Tang, X.-L. Li, H. Liu, Y. Gao, Y. Shen, S. Zhang, P. Lu, B. Yang, S.-J. Su and Y. Ma, Dyes Pigm., 2018, 149, 430–436 CrossRef CAS.
- Y. Rahmawati, A. Afandi, D. Arengga, S. Sendari, W. Agustin, T. Matsumoto and I. Rahman, IOP Conf. Ser. Earth Environ. Sci., 2018, 105, 012079 CrossRef.
- D. M. Kammen, Sci. Am., 2006, 295, 84–93 CrossRef PubMed.
- V. Balzani, A. Credi and M. Venturi, ChemSusChem, 2008, 1, 26–58 CrossRef CAS PubMed.
- C. J. Brabec, N. S. Sariciftci and J. C. Hummelen, Adv. Funct. Mater., 2001, 11, 15–26 CrossRef CAS.
- S. Günes, H. Neugebauer and N. S. Sariciftci, Chem. Rev., 2007, 107, 1324–1338 CrossRef PubMed.
- Y.-J. Cheng, S.-H. Yang and C.-S. Hsu, Chem. Rev., 2009, 109, 5868–5923 CrossRef CAS PubMed.
- A. W. Hains, Z. Liang, M. A. Woodhouse and B. A. Gregg, Chem. Rev., 2010, 110, 6689–6735 CrossRef CAS PubMed.
- S. Dey, Small, 2019, 15, 1900134 CrossRef PubMed.
- C. W. Tang, Appl. Phys. Lett., 1986, 48, 183–185 CrossRef CAS.
- A. Shah, P. Torres, R. Tscharner, N. Wyrsch and H. Keppner, Science, 1999, 285, 692–698 CrossRef CAS PubMed.
- G. Dennler, M. C. Scharber and C. J. Brabec, Adv. Mater., 2009, 21, 1323–1338 CrossRef CAS.
- D. Gendron and M. Leclerc, Energy Environ. Sci., 2011, 4, 1225–1237 RSC.
- C. Duan, F. Huang and Y. Cao, J. Mater. Chem., 2012, 22, 10416–10434 RSC.
- J. You, L. Dou, K. Yoshimura, T. Kato, K. Ohya, T. Moriarty, K. Emery, C.-C. Chen, J. Gao and G. Li, Nat. Commun., 2013, 4, 1–10 Search PubMed.
- Y. Liang, Z. Xu, J. Xia, S. T. Tsai, Y. Wu, G. Li, C. Ray and L. Yu, Adv. Mater., 2010, 22, E135–E138 CrossRef CAS PubMed.
- T. Taima, M. Chikamatsu, Y. Yoshida, K. Saito and K. Yase, Appl. Phys. Lett., 2004, 85, 1832–1834 CrossRef CAS.
- Y. Zhang, B. Kan, Y. Sun, Y. Wang, R. Xia, X. Ke, Y. Q. Q. Yi, C. Li, H. L. Yip and X. Wan, Adv. Mater., 2018, 30, 1707508 CrossRef PubMed.
- F. Zhao, S. Dai, Y. Wu, Q. Zhang, J. Wang, L. Jiang, Q. Ling, Z. Wei, W. Ma and W. You, Adv. Mater., 2017, 29, 1700144 CrossRef PubMed.
- L. Meng, Y. Zhang, X. Wan, C. Li, X. Zhang, Y. Wang, X. Ke, Z. Xiao, L. Ding and R. Xia, Science, 2018, 361, 1094–1098 CrossRef CAS PubMed.
- W. Zhao, S. Li, H. Yao, S. Zhang, Y. Zhang, B. Yang and J. Hou, J. Am. Chem. Soc., 2017, 139, 7148–7151 CrossRef CAS PubMed.
- S. Zhang, Y. Qin, J. Zhu and J. Hou, Adv. Mater., 2018, 30, 1800868 CrossRef PubMed.
- K. Jiang, J. Zhang, C. Zhong, F. R. Lin, F. Qi, Q. Li, Z. Peng, W. Kaminsky, S.-H. Jang and J. Yu, Nat. Energy, 2022, 7, 1076–1086 CrossRef.
- Y. Ie, S. Jinnai, M. Karakawa and Y. Aso, Chem. Lett., 2015, 44, 694–696 CrossRef CAS.
- Y. Shibata, T. Kono, H. Usui and Y. Yoshida, Chem. Lett., 2015, 44, 680–682 CrossRef CAS.
- W. Jiang, L. Ye, X. Li, C. Xiao, F. Tan, W. Zhao, J. Hou and Z. Wang, Chem. Commun., 2014, 50, 1024–1026 RSC.
- G. Sharma, M. Roy, J. Mikroyannidis and K. J. Thomas, Org. Electron., 2012, 13, 3118–3129 CrossRef CAS.
- Y. Zhou, L. Ding, K. Shi, Y. Z. Dai, N. Ai, J. Wang and J. Pei, Adv. Mater., 2012, 24, 957–961 CrossRef CAS PubMed.
- Y.-Q. Zheng, Y.-Z. Dai, Y. Zhou, J.-Y. Wang and J. Pei, Chem. Commun., 2014, 50, 1591–1594 RSC.
- S. Li, J. Yan, C.-Z. Li, F. Liu, M. Shi, H. Chen and T. P. Russell, J. Mater. Chem. A, 2016, 4, 3777–3783 RSC.
- M. Klikar, V. Jelínková, Z. Růžičková, T. Mikysek, O. Pytela, M. Ludwig and F. Bureš, Eur. J. Org. Chem., 2017, 2017, 2764–2779 CrossRef CAS.
- S. P. Singh, J. Mater. Chem. A, 2019, 7, 22701–22729 RSC.
- K. Wang, Y. Firdaus, M. Babics, F. Cruciani, Q. Saleem, A. El Labban, M. A. Alamoudi, T. Marszalek, W. Pisula and F. Laquai, Chem. Mater., 2016, 28, 2200–2208 CrossRef CAS.
- T. Duan, M. Babics, A. Seitkhan, Y. Firdaus, R.-Z. Liang, F. Cruciani, S. Liu, S. Lopatin and P. M. Beaujuge, J. Mater. Chem. A, 2018, 6, 9368–9372 RSC.
- W. Zhao, S. Li, H. Yao, S. Zhang, Y. Zhang, B. Yang and J. Hou, J. Am. Chem. Soc., 2017, 139, 7148–7151 CrossRef CAS PubMed.
- S. j. Xu, Z. Zhou, W. Liu, Z. Zhang, F. Liu, H. Yan and X. Zhu, Adv. Mater., 2017, 29, 1704510 CrossRef PubMed.
- J. Sun, X. Ma, Z. Zhang, J. Yu, J. Zhou, X. Yin, L. Yang, R. Geng, R. Zhu and F. Zhang, Adv. Mater., 2018, 30, 1707150 CrossRef PubMed.
- B. Al-Anesi, S. Revoju, A. Hiltunen, R. Suhonen, T. M. Kraft, M. Liu, H. Zhang, Z. Deng, C. Fedele and A. Berdin, Energy Technol., 2022, 10, 2200264 CrossRef CAS.
- Y. Zhou, M. Li, H. Lu, H. Jin, X. Wang, Y. Zhang, S. Shen, Z. Ma, J. Song and Z. Bo, Adv. Funct. Mater., 2021, 31, 2101742 CrossRef CAS.
- H. Hang, X. Wu, Q. Xu, Y. Chen, H. Li, W. Wang, H. Tong and L. Wang, Dyes Pigm., 2019, 160, 243–251 CrossRef CAS.
- Y. Li, L. Zhong, F.-P. Wu, Y. Yuan, H.-J. Bin, Z.-Q. Jiang, Z. Zhang, Z.-G. Zhang, Y. Li and L.-S. Liao, Energy Environ. Sci., 2016, 9, 3429–3435 RSC.
- Y. Lin, J. Wang, Z. G. Zhang, H. Bai, Y. Li, D. Zhu and X. Zhan, Adv. Mater., 2015, 27, 1170–1174 CrossRef CAS PubMed.
- S. Li, L. Ye, W. Zhao, S. Zhang, S. Mukherjee, H. Ade and J. Hou, Adv. Mater., 2016, 28, 9423–9429 CrossRef CAS PubMed.
- Y. Li, X. Liu, F.-P. Wu, Y. Zhou, Z.-Q. Jiang, B. Song, Y. Xia, Z.-G. Zhang, F. Gao and O. Inganäs, J. Mater. Chem. A, 2016, 4, 5890–5897 RSC.
- C. e. Zhang, S. Feng, Y. Liu, R. Hou, Z. Zhang, X. Xu, Y. Wu and Z. Bo, ACS Appl. Mater. Interfaces, 2017, 9, 33906–33912 CrossRef CAS PubMed.
- Z. Zhang, W. Liu, T. Rehman, H.-X. Ju, J. Mai, X. Lu, M. Shi, J. Zhu, C.-Z. Li and H. Chen, J. Mater. Chem. A, 2017, 5, 9649–9654 RSC.
- Y. Yang, Z.-G. Zhang, H. Bin, S. Chen, L. Gao, L. Xue, C. Yang and Y. Li, J. Am. Chem. Soc., 2016, 138, 15011–15018 CrossRef CAS PubMed.
- W. T. Hadmojo, F. T. A. Wibowo, D. Y. Ryu, I. H. Jung and S.-Y. Jang, ACS Appl. Mater. Interfaces, 2017, 9, 32939–32945 CrossRef CAS PubMed.
- B. Xiao, J. Song, B. Guo, M. Zhang, W. Li, R. Zhou, J. Liu, H.-B. Wang, M. Zhang and G. Luo, J. Mater. Chem. A, 2018, 6, 957–962 RSC.
- X. Xu, T. Yu, Z. Bi, W. Ma, Y. Li and Q. Peng, Adv. Mater., 2018, 30, 1703973 CrossRef PubMed.
- Y. Lin, F. Zhao, Y. Wu, K. Chen, Y. Xia, G. Li, S. K. Prasad, J. Zhu, L. Huo, H. Bin, Z. G. Zhang, X. Guo, M. Zhang, Y. Sun, F. Gao, Z. Wei, W. Ma, C. Wang, J. Hodgkiss, Z. Bo, O. Inganäs, Y. Li and X. Zhan, Adv. Mater., 2017, 29, 1604155 CrossRef PubMed.
- B. Guo, W. Li, X. Guo, X. Meng, W. Ma, M. Zhang and Y. Li, Adv. Mater., 2017, 29, 1702291 CrossRef PubMed.
- H. Bin, Y. Yang, Z.-G. Zhang, L. Ye, M. Ghasemi, S. Chen, Y. Zhang, C. Zhang, C. Sun and L. Xue, J. Am. Chem. Soc., 2017, 139, 5085–5094 CrossRef CAS PubMed.
- Y.-C. Lin, N.-Z. She, C.-H. Chen, A. Yabushita, H. Lin, M.-H. Li, B. Chang, T.-F. Hsueh, B.-S. Tsai and P.-T. Chen, ACS Appl. Mater. Interfaces, 2022, 14, 37990–38003 CrossRef CAS PubMed.
- J. Huang, H. Tang, C. Yan and G. Li, Cell Rep. Phys. Sci., 2021, 2, 100292 CrossRef CAS.
- Y. Cui, Y. Wang, J. Bergqvist, H. Yao, Y. Xu, B. Gao, C. Yang, S. Zhang, O. Inganäs and F. Gao, Nat. Energy, 2019, 4, 768–775 CrossRef CAS.
- V. Gupta, A. Bagui and S. P. Singh, Adv. Funct. Mater., 2017, 27, 1603820 CrossRef PubMed.
- A. Bagui, A. Garg, B. Tyagi, V. Gupta and S. P. Singh, Chem. Commun., 2018, 54, 4001–4004 RSC.
- H. Xu, Y. Yang, C. Zhong, X. Zhan and X. Chen, J. Mater. Chem. A, 2018, 6, 6393–6401 RSC.
- I. D. W. Samuel and G. A. Turnbull, Chem. Rev., 2007, 107, 1272–1295 CrossRef CAS PubMed.
- Y. Shao, G. C. Bazan and A. J. Heeger, Adv. Mater., 2008, 20, 1191–1193 CrossRef CAS.
- C. R. Newman, C. D. Frisbie, D. A. da Silva Filho, J.-L. Brédas, P. C. Ewbank and K. R. Mann, Chem. Mater., 2004, 16, 4436–4451 CrossRef CAS.
- Z. Chi, X. Zhang, B. Xu, X. Zhou, C. Ma, Y. Zhang, S. Liu and J. Xu, Chem. Soc. Rev., 2012, 41, 3878–3896 RSC.
- L. Yan, Y. Zhang, B. Xu and W. Tian, Nanoscale, 2016, 8, 2471–2487 RSC.
- X. Li, K. Ma, S. Zhu, S. Yao, Z. Liu, B. Xu, B. Yang and W. Tian, Anal. Chem., 2014, 86, 298–303 CrossRef CAS PubMed.
- C. W. Tang and S. A. VanSlyke, Appl. Phys. Lett., 1987, 51, 913–915 CrossRef CAS.
- M. Y. Wong and E. Zysman-Colman, Adv. Mater., 2017, 29, 1605444 CrossRef PubMed.
- X. Cao, D. Zhang, S. Zhang, Y. Tao and W. Huang, J. Mater. Chem. C, 2017, 5, 7699–7714 RSC.
- D. U. Kim, S. H. Paik, S.-H. Kim and T. Tsutsui, Synth. Met., 2001, 123, 43–46 CrossRef CAS.
- L. Hung and C. Chen, Mater. Sci. Eng., R, 2002, 39, 143–222 CrossRef.
- S. Zhang, L. Yao, Q. Peng, W. Li, Y. Pan, R. Xiao, Y. Gao, C. Gu, Z. Wang and P. Lu, Adv. Funct. Mater., 2015, 25, 1755–1762 CrossRef CAS.
- J. Y. Hu, Y. J. Pu, F. Satoh, S. Kawata, H. Katagiri, H. Sasabe and J. Kido, Adv. Funct. Mater., 2014, 24, 2064–2071 CrossRef CAS.
- S. J. Cha, N. S. Han, J. K. Song, S.-R. Park, Y. M. Jeon and M. C. Suh, Dyes Pigm., 2015, 120, 200–207 CrossRef CAS.
- X. Tao, S. Miyata, H. Sasabe, G. Zhang, T. Wada and M. Jiang, Appl. Phys. Lett., 2001, 78, 279–281 CrossRef CAS.
- T.-H. Liu, C.-Y. Iou and C. H. Chen, Appl. Phys. Lett., 2003, 83, 5241–5243 CrossRef CAS.
- P. Franken, A. E. Hill, C. e. Peters and G. Weinreich, Phys. Rev. Lett., 1961, 7, 118 CrossRef.
- R. W. Hellwarth, Phys. Rev., 1963, 130, 1850 CrossRef.
- R. Chiao, C. Townes and B. Stoicheff, Phys. Rev. Lett., 1964, 12, 592 CrossRef.
-
H. Durr and H. Bouas-Laurent, Photochromism: Molecules and Systems, Elsevier, 2003 Search PubMed.
- J. A. Delaire and K. Nakatani, Chem. Rev., 2000, 100, 1817–1846 CrossRef CAS PubMed.
- D. R. Kanis, M. A. Ratner and T. J. Marks, Chem. Rev., 1994, 94, 195–242 CrossRef CAS.
-
M. Gimeno, A. Laguna and T. Meyer, Comprehensive Coordination Chemistry II, Elsevier, Oxford, 2004, vol. 6, p. 1 Search PubMed.
-
J. Zyss, Molecular Nonlinear Optics: Materials, Physics, and Devices, Academic press, 2013 Search PubMed.
-
C. Bosshard, K. Sutter, P. Prêtre, J. Hulliger, M. Flörsheimer, P. Kaatz and P. Günter, Organic Nonlinear Optical Materials, CRC press, 2020 Search PubMed.
- S. R. Marder, Chem. Commun., 2006, 131–134 RSC.
-
M. G. Papadopoulos, A. J. Sadlej and J. Leszczynski, Non-linear Optical Properties of Matter, Springer, 2006 Search PubMed.
- W. Gong, Q. Li, Z. Li, C. Lu, J. Zhu, S. Li, J. Yang, Y. Cui and J. Qin, J. Phys. Chem. B, 2006, 110, 10241–10247 CrossRef CAS PubMed.
- J. L. Bredas, C. Adant, P. Tackx, A. Persoons and B. Pierce, Chem. Rev., 1994, 94, 243–278 CrossRef CAS.
- S. D. Bella, Chem. Soc. Rev., 2001, 30, 355–366 RSC.
- V. Guieu, C. Payrastre, Y. Madaule, S. Garcia-Alonso, P. G. Lacroix and K. Nakatani, Chem. Mater., 2006, 18, 3674–3681 CrossRef CAS.
- L. R. Dalton, P. A. Sullivan and D. H. Bale, Chem. Rev., 2010, 110, 25–55 CrossRef CAS PubMed.
- P. A. Sullivan and L. R. Dalton, Acc. Chem. Res., 2010, 43, 10–18 CrossRef CAS PubMed.
- B. H. Robinson, L. E. Johnson, D. L. Elder, A. A. Kocherzhenko, C. M. Isborn, C. Haffner, W. Heni, C. Hoessbacher, Y. Fedoryshyn and Y. Salamin, J. Lightwave Technol., 2018, 36, 5036–5047 CAS.
- J. Liu, C. Ouyang, F. Huo, W. He and A. Cao, Dyes Pigm., 2020, 181, 108509 CrossRef CAS.
- M. Klikar, P. Solanke, J. Tydlitát and F. Bureš, Chem. Rec., 2016, 16, 1886–1905 CrossRef CAS PubMed.
- J. Kulhánek and F. Bureš, Beilstein J. Org. Chem., 2012, 8, 25–49 CrossRef PubMed.
- D. Cvejn, E. Michail, I. Polyzos, N. Almonasy, O. Pytela, M. Klikar, T. Mikysek, V. Giannetas, M. Fakis and F. Bureš, J. Mater. Chem. C, 2015, 3, 7345–7355 RSC.
- J. Kulhánek, F. Bureš, O. Pytela, T. Mikysek, J. Ludvík and A. Růžička, Dyes Pigm., 2010, 85, 57–65 CrossRef.
- A. Plaquet, B. Champagne, J. Kulhánek, F. Bureš, E. Bogdan, F. Castet, L. Ducasse and V. Rodriguez, ChemPhysChem, 2011, 12, 3245–3252 CrossRef CAS PubMed.
- J. Kulhánek, F. Bures, A. Wojciechowski, M. Makowska-Janusik, E. Gondek and I. Kityk, J. Phys. Chem. A, 2010, 114, 9440–9446 CrossRef PubMed.
- F. Bureš, J. Kulhánek, T. Mikysek, J. Ludvík and J. Lokaj, Tetrahedron Lett., 2010, 51, 2055–2058 CrossRef.
- J. Kulhánek, F. Bureš, W. Kuznik, I. V. Kityk, T. Mikysek and A. Růžička, Chem.–Asian J., 2013, 8, 465–475 CrossRef PubMed.
- L. Dokládalová, F. Bureš, W. Kuznik, I. V. Kityk, A. Wojciechowski, T. Mikysek, N. Almonasy, M. Ramaiyan, Z. Padělková and J. Kulhánek, Org. Biomol. Chem., 2014, 12, 5517–5527 RSC.
- F. Bureš, H. Čermáková, J. Kulhánek, M. Ludwig, W. Kuznik, I. V. Kityk, T. Mikysek and A. Růžička, Eur. J. Org. Chem., 2012, 2012, 529–538 CrossRef.
- W. Gong, Q. Li, S. Li, C. Lu, Z. Li, J. Zhu, Z. Zhu, Q. Wang, Y. Cui and J. Qin, Mater. Lett., 2007, 61, 1151–1153 CrossRef CAS.
- Y. Yang, H. Xiao, H. Wang, F. Liu, S. Bo, J. Liu, L. Qiu, Z. Zhen and X. Liu, J. Mater. Chem. C, 2015, 3, 11423–11431 RSC.
- J. Liu, M. Zhang, W. Gao, A. Fedorchuk and I. Kityk, J. Mol. Struct., 2018, 1165, 223–227 CrossRef CAS.
- Y. Yang, H. Xu, F. Liu, H. Wang, G. Deng, P. Si, H. Huang, S. Bo, J. Liu and L. Qiu, J. Mater. Chem. C, 2014, 2, 5124–5132 RSC.
- Z. a. Li, H. Kim, S.-H. Chi, J. M. Hales, S.-H. Jang, J. W. Perry and A. K.-Y. Jen, Chem. Mater., 2016, 28, 3115–3121 CrossRef CAS.
- W. Wang, X. Yang and S. Xiao, J. Lumin., 2020, 225, 117406 CrossRef CAS.
- H. Wang, S. Yang, X. Li, F. Yang, X. Sun, W. Li and Z. Yao, Polym.-Plast. Technol. Mater., 2020, 59, 1875–1886 Search PubMed.
- Y. Yu, P. Xu, S. Jia, H. Pan, H. Zhang, D. Wang and L. Dong, Int. J. Biol. Macromol., 2019, 127, 210–221 CrossRef CAS PubMed.
- H. I. Yoon, J. H. Kim, K. S. Park, J. W. Namgoong, T. G. Hwang, J. P. Kim and J. E. Son, Hortic., Environ. Biotechnol., 2020, 61, 999–1009 CrossRef CAS.
- Y. Jiang, C. Yan, H. Zhang, M. Wu, S. Zheng, Y. Zhang and L. Xu, Coatings, 2021, 11, 139 CrossRef CAS.
- D. Wang, Y. Yu, X. Ai, H. Pan, H. Zhang and L. Dong, Polym. Adv. Technol., 2019, 30, 203–211 CrossRef CAS.
- T. G. Hwang, J. Y. Kim, J. W. Namgoong, J. M. Lee, S. B. Yuk, S. H. Kim and J. P. Kim, Photochem. Photobiol. Sci., 2019, 18, 1064–1074 CrossRef CAS PubMed.
- Y. Liu, J. Liu, Q. Liu, W. He and I. Kityk, J. Lumin., 2020, 218, 116852 CrossRef CAS.
- Y. Liu, Z. Gui and J. Liu, Polymers, 2022, 14, 851 CrossRef CAS PubMed.
- Y. Wang, Y. Yu, W. Liu, L. Ren and G. Ge, J. Agric. Food Chem., 2018, 66, 13295–13302 CrossRef CAS PubMed.
- Y. Qi, Y. Wang, Y. Yu, Z. Liu, Y. Zhang, Y. Qi and C. Zhou, J. Mater. Chem. C, 2016, 4, 11291–11297 RSC.
- Y. Wang, Z. Qian, X. Li, A. Qin, Y. Guo and B. Tang, J. Mater. Chem. C, 2021, 9, 12681–12693 RSC.
- M. L. Kadam, D. Patil and N. Sekar, Opt. Mater., 2018, 85, 308–318 CrossRef CAS.
- Y. Wang, Z. Qian, X. Li, A. Qin, Y. Guo and B. Tang, Dyes Pigm., 2022, 197, 109888 CrossRef CAS.
- J. Yang, Z. Ren, B. Chen, M. Fang, Z. Zhao, B. Z. Tang, Q. Peng and Z. Li, J. Mater. Chem. C, 2017, 5, 9242–9246 RSC.
- Q. Li and Z. Li, Acc. Chem. Res., 2020, 53, 962–973 CrossRef CAS PubMed.
- J. Mei, N. L. Leung, R. T. Kwok, J. W. Lam and B. Z. Tang, Chem. Rev., 2015, 115, 11718–11940 CrossRef CAS PubMed.
- H. Zhang, D. C. Salo, D. M. Kim, S. Komarov, Y.-C. Tai and M. Y. Berezin, J. Biomed. Opt., 2016, 21, 126006 CrossRef PubMed.
- L. Yuan, W. Lin, K. Zheng, L. He and W. Huang, Chem. Soc. Rev., 2013, 42, 622–661 RSC.
- H. Kobayashi, Y. Koyama, T. Barrett, Y. Hama and P. L. Choyke, Molecular Probes for Biomedical Applications II, Proc. SPIE, 2008, 6867, 157–164 CrossRef.
- J. Chan, S. C. Dodani and C. J. Chang, Nat. Chem., 2012, 4, 973–984 CrossRef CAS PubMed.
- S. Mu, H. Gao, C. Li, S. Li, Y. Wang, Y. Zhang, C. Ma, H. Zhang and X. Liu, Talanta, 2021, 221, 121606 CrossRef CAS PubMed.
- Q. Li, Z. Wang, W. Song, H. Ma, J. Dong, Y.-Y. Quan, X. Ye and Z.-S. Huang, Dyes Pigm., 2019, 161, 389–395 CrossRef CAS.
- X. Yang, Y. Liu, Y. Wu, X. Ren, D. Zhang and Y. Ye, Sens. Actuators, B, 2017, 253, 488–494 CrossRef CAS.
- B.-B. Wang, Y. Wang, W.-N. Wu, Z.-H. Xu, X.-L. Zhao, Z.-Q. Xu and Y.-C. Fan, Inorg. Chem. Commun., 2020, 122, 108245 CrossRef CAS.
- Q. Wu, S. Wang, E. Hao and L. Jiao, Spectrochim. Acta, Part A, 2021, 247, 119102 CrossRef CAS PubMed.
- A. Tigreros and J. Portilla, Eur. J. Org. Chem., 2022, e202200249 CAS.
- S. Munusamy, S. Swaminathan, D. Jothi, V. P. Muralidharan and S. K. Iyer, RSC Adv., 2021, 11, 15656–15662 RSC.
- Y. Zhao, L. Feng, X. Meng and J. Guan, Dyes Pigm., 2020, 183, 108713 CrossRef CAS.
- X. Wen, Q. Wang and Z. Fan, J. Lumin., 2018, 194, 366–373 CrossRef CAS.
- Q. Wang, X. Wen and Z. Fan, J. Photochem. Photobiol., A, 2018, 358, 92–99 CrossRef CAS.
- X. Wen, L. Yan and Z. Fan, J. Photochem. Photobiol., A, 2021, 405, 112969 CrossRef CAS.
- N. Dey, J. i. Kulhánek, F. Bureš and S. Bhattacharya, J. Org. Chem., 2018, 84, 1787–1796 CrossRef PubMed.
- N. Dey, J. Kulhanek, F. Bureš and S. Bhattacharya, J. Org. Chem., 2021, 86, 14663–14671 CrossRef CAS PubMed.
- X. Zhang and D. W. MacMillan, J. Am. Chem. Soc., 2016, 138, 13862–13865 CrossRef CAS PubMed.
- Z. Zuo, H. Cong, W. Li, J. Choi, G. C. Fu and D. W. MacMillan, J. Am. Chem. Soc., 2016, 138, 1832–1835 CrossRef CAS PubMed.
- C. K. Prier, D. A. Rankic and D. W. MacMillan, Chem. Rev., 2013, 113, 5322–5363 CrossRef CAS PubMed.
- V. Srivastava, P. K. Singh and P. P. Singh, J. Photochem. Photobiol., C, 2022, 100488 CrossRef CAS.
- N. A. Romero and D. A. Nicewicz, Chem. Rev., 2016, 116, 10075–10166 CrossRef CAS PubMed.
- Y. Lee and M. S. Kwon, Eur. J. Org. Chem., 2020, 2020, 6028–6043 CrossRef CAS.
- J. Luo and J. Zhang, ACS Catal., 2016, 6, 873–877 CrossRef CAS.
- T.-Y. Shang, L.-H. Lu, Z. Cao, Y. Liu, W.-M. He and B. Yu, Chem. Commun., 2019, 55, 5408–5419 RSC.
- P. P. Singh and V. Srivastava, Org. Biomol. Chem., 2021, 19, 313–321 RSC.
- Y. Zhao, C. Zhang, K. F. Chin, O. Pytela, G. Wei, H. Liu, F. Bureš and Z. Jiang, RSC Adv., 2014, 4, 30062–30067 RSC.
- Z. Burešová, V. Jandová, M. Klikar, M. Grygarová and F. Bureš, Org. Biomol. Chem., 2022, 20, 9378–9384 RSC.
- Z. Hloušková, M. Klikar, O. Pytela, N. Almonasy, A. Růžička, V. Jandová and F. Bureš, RSC Adv., 2019, 9, 23797–23809 RSC.
- Z. Hloušková, J. Tydlitát, M. Kong, O. Pytela, T. Mikysek, M. Klikar, N. Almonasy, M. Dvořák, Z. Jiang and A. Růžička, ChemistrySelect, 2018, 3, 4262–4270 CrossRef.
- K. Cao, S. M. Tan, R. Lee, S. Yang, H. Jia, X. Zhao, B. Qiao and Z. Jiang, J. Am. Chem. Soc., 2019, 141, 5437–5443 CrossRef CAS PubMed.
- G. Wei, C. Zhang, F. Bureš, X. Ye, C.-H. Tan and Z. Jiang, ACS Catal., 2016, 6, 3708–3712 CrossRef CAS.
- L. Lin, X. Bai, X. Ye, X. Zhao, C. H. Tan and Z. Jiang, Angew. Chem., 2017, 129, 14030–14034 CrossRef.
- B. Qiao, C. Li, X. Zhao, Y. Yin and Z. Jiang, Chem. Commun., 2019, 55, 7534–7537 RSC.
- C. Zhang, S. Li, F. Bureš, R. Lee, X. Ye and Z. Jiang, ACS Catal., 2016, 6, 6853–6860 CrossRef CAS.
Footnote |
† The two authors contributed equally to this work. |
|
This journal is © The Royal Society of Chemistry 2023 |
Click here to see how this site uses Cookies. View our privacy policy here.