DOI:
10.1039/D3SU00065F
(Critical Review)
RSC Sustain., 2023,
1, 763-787
A review of past promises, present realities and a vibrant future for wound dressing from naturally occurring to sustainable materials
Received
22nd February 2023
, Accepted 9th May 2023
First published on 10th May 2023
Abstract
In the contemporary world, evolution of the scientific field has reached an elevation but has its own intricacies. It is necessary to use naturally occurring materials, which are often incorporated into modern therapeutics and have made major contributions. Generated medical waste needs to be processed to ensure that clean-up is not messy. In many cases, the cost of waste disposal is greater than the cost of the starting materials. It is important to prevent, or at least minimize, the formation of hazardous products that are environmentally harmful. In this study, we review the various applications of phytochemicals, such as curcumin, EGCG, tannic acid, cellulose, ascorbic acid, aloe vera and quercetin, for the healing of chronic wounds (e.g., incisional, diabetic and burn wounds), along with antiviral and antibacterial properties, with an emphasis on hydrogels fabricated from biopolymers.
Sustainability spotlight
Hydrogels derived from natural (phytochemicals) and sustainable materials (bio derived and biopolymers) are promising in the biomedical field in general, and in wound dressings in particular, for faster and better healing due to their excellent antimicrobial properties.
|
1. Introduction
Natural products are compounds produced by living organisms and can be either primary or secondary metabolites. They are distinct due to the presence of aromaticity in the rings, hetero atoms, chiral centers and stereoselectivity.1 The different classes include alkaloids, terpenoids, phenolics, and saponins.2 They have always had enormous significance as far as medicine and health are concerned, and herbs have long been used for pain relief, wound treatment, etc. In recent years, the use of natural compounds in biomedical fields has seen a surge. Technological capabilities now allow the biological effects of natural compounds on viruses and bacteria to be studied. Viruses are sub-microscopic non-cellular organisms that require a host cell to survive; they use their machinery to produce viral proteins and their viral coat encloses DNA or RNA as the genetic material.3 The mutation rates of viruses are very high, and therefore the best chance to fight them is during the period of attachment to the host cell. The life cycle of a virus involves attachment, viral entry (endocytosis), the release of the viral genome, replication of the viral genome (reverse transcription), synthesis and processing of viral proteins, and assembly and release of the virus from the host cell.4 Most antiviral drugs work by disrupting the critical stages of the life cycle and the synthesis of viral-specific nucleic acids.
Bacteria are microscopic single-celled organisms that are for the most part useful and harmless; they do not necessarily require a host to survive and DNA is usually the genetic material. Some are infectious and the life cycle involves a lag phase, exponential phase, stationary phase, and death phase.
Antibacterial agents are successful because they can act selectively against bacterial cells rather than animal cells due to differences in structure and biosynthetic pathways. Bacteria are characterized as Gram-positive and Gram-negative based on the staining technique. Bacteria that lack an outer membrane but have thick cell walls that stain purple are called Gram-positive bacteria. Bacteria with thin peptidoglycan cell walls that stain pink and are surrounded by an outer membrane that contains lipopolysaccharides are called Gram-negative bacteria.5 The different mechanisms by which antibacterial agents act are: the inhibition of cell metabolism, inhibition of bacterial cell wall synthesis, plasma membrane interaction, protein synthesis disruption, nucleic acid replication, and transcription inhibition.6 Applications, such as wound dressings and hydrogels, are collaboratively approached herein by reviewing the established methodologies and pooling the obtained knowledge to reach the solution. In this study, only compounds sourced from plants are being considered because they can be regenerated in the time frame of a human lifetime; NPs produced as a result of metabolic pathways are not considered. In addition, few approaches demonstrating sustainability (self-healing and durability) are emphasized. External stresses and pressure result in damage to wound dressings. Self-healing capability is preferred in the case of wound dressings to spontaneously heal themselves similar to biological tissues and hence prolong the lifetime of the material.7
The conservation of resources and reduction of waste is made feasible by employing durable materials. This review extends to multifunctional antimicrobial dressings and hydrogels that extend the service life of the dressings and prevent the drying out of newly formed granulation tissue.8 Consequently, the raw materials employed for wound dressings for burn, diabetic and incisional wounds are lint, gauze, cotton, alginate, plaster and non-biodegradable polymeric resources. The concerns put forward by medical professionals over the materials used both in biomedical commodities and non-medical items make it imperative to use biocompatible compounds due to the unfolding of hazardous impacts on the ecosystem. Here, hydrogels that are incorporated into the wound dressings, prepared for faster and better healing due to their excellent mechanical and biochemical properties,9 are also reviewed.
2. Methods of fabrication of wound dressings and hydrogels
Various materials for the fabrication of hydrogels and wound dressings are chosen based on the extremity of the wounds developed. The following qualities are mandatory in a material for it to be sustainable. (i) It should decay after its lifetime without any residual by-products. (ii) Good adhesion of the material due to its superior mechanical properties on the wound site to avoid further infections. (iii) The material must be capable of assisting in the clotting of blood at the wound site and should be involved in activating factors for maintaining homeostasis to facilitate faster wound healing. (iv) It must possess excellent moisture retention so that it can deliver moisture to the wound site, which is required for cell proliferation.10 The integration of a biodegradable polymer with the plant source to achieve the said properties can be done by various methods as follows:
Electrospinning
Traditional dressings have not been able to satisfy the demands of the current market due to their limited antibacterial qualities and other drawbacks, despite the rising need for wound care and treatment globally. Researchers are becoming more and more interested in electrospinning technology as a straightforward and adaptable production technique. The electrospun nanofiber membranes have both distinctive structures and biological functions that make them suitable for use as advanced wound dressings. Based on this idea, it is possible to modify the electrospinning process to alter the morphology and size of nanofibers by adjusting system parameters such as polymer type, molecular weight, viscosity, the conductivity of the solution, and surface tension, as well as process parameters such as voltage, flow rate, and receiving distance.11 Electrospinning is an adaptable and practical method for creating ultrathin fibres. There have been remarkable strides in the advancement of electrospinning techniques and the creation of electrospun nanofibers to suit or enable diverse applications.12
An electrospinning fabrication process is voltage driven in which nanofibers are yielded from polymers. Solutions with low surface tension are usually preferred. Typically, a needle with a high voltage positive charge and a negative voltage at the collector is the key to the formation of Taylor cones and sample deposition. The voltage applied at the needle causes charges to accumulate and overcome the surface tension of the solution. The needle-to-collector distance is reduced for less travel time and this induces small fiber–fiber bonding and prevents delamination. Increasing the solution flow rate increases the diameter of the developing fibers.12
Spin coating
Spin coating is a frequently used method for producing thin-film coatings with uniform, smooth surfaces over huge regions. Through the development of techniques involving the interface between the substrate and the deposited solution, research has recently expanded the use of spin coating to produce self-supported films, also known as freestanding films. As a result, those structures have been created for a variety of regions. Spin coated freestanding films are used for biosensing, medication administration, and wound dressing in the field of medicine.13 Four phases can efficiently be used to separate the spin-coating procedure, namely, (i) the dispensing stage, (ii) substrate acceleration stage, (iii) stable fluid outflow, and (iv) solvent evaporation. For a variety of spin coated systems, a wide range of spin-coating process factors have been thoroughly investigated.
It is well known that the spin speed, fluid viscosity, solvent evaporation rate, diffusivity, molecular weight, and concentration of the solutes have a significant impact on the film thickness, morphology, and surface topography. During the spin coating, a solution is dispensed onto a rotating substrate and is cast as an evenly spread thin film. The centripetal force in combination with the surface tension of the solution pulls the liquid into an even coating.14
Spun bond technique
The clinical options for managing chronic wounds are currently limited, particularly in terms of reducing pain and promoting quick wound healing. Therefore, the creation of alternate therapeutic strategies is critical. Recent studies on the creation of wound healing dressings have been considerably aided by the use of multi-dimensional, multi-pore, and multi-functional electrospun nanofiber scaffolds, which have been fabricated using this method.15 Fabric manufacture and filament production are combined in the process of creating spun bond textiles. These textiles are now accepted in a variety of application fields including civil engineering, medicine, and hygiene because of their exceptional characteristics and high process efficiencies.16 Non-woven fabrics are formed by an integrated process of fiber spinning, web formation and bonding. The molten polymer is spun to form filaments, which are stretched. The extruded spun fibers are deposited under the influence of air jets or electrostatic charges onto a collecting belt, which is perforated to prevent the air stream from forming uncontrolled, deflected fibers. On increasing the polymer melt temperature, the filament diameter decreases.17
Atomic layer deposition and molecular layer deposition
High-quality nanometer-scale thin films may be made atomically, layer by layer, using the atomic layer deposition (ALD) process. ALD provides several key benefits over other cutting-edge gas-phase thin-film deposition methods as follows. The films are symmetrical even when deposited on intricate three-dimensional (3D) structures and may be placed with excellent control over the film thickness. They are also dense, uniform, and pinhole-free. Due to its unique capabilities, atomic layer deposition (ALD), an ultra-thin film deposition technology, has found several uses. It can increase the effectiveness of electrical devices and include the uniform deposition of conformal films with adjustable thickness, even on intricate three-dimensional surfaces. Both the fundamental knowledge of how novel functional materials may be created by ALD and the many practical uses of this technique, notably in advanced nanopatterning for microelectronics, catalysis, and medical disciplines, have generated a great deal of attention.18 The research using an ALD version that produced organic polymers in the 1990s, now more frequently known as molecular layer deposition (MLD), named after the molecular layer-by-layer manner the film builds during the deposition, has significantly expanded the variety of materials. Interestingly, in the late 2000s, the two methods, ALD and MLD, were merged to create inorganic–organic hybrid materials. This allowed for the synthesis of entirely new material families with a variety of properties that were not feasible with any other method at the time.19 This is a vapour phase technique based on the sequential use of gas-phase chemical processes in which the thin film growth is governed by the self-limiting reactions of reactant molecules with the substrate to form films, which ensure that the reaction stops once all the reactive sites on the substrate are used up. ALD is limited to inorganic coatings and MLD uses small organic molecules as well, therefore enabling the growth of organic layers similar to polymerisation.19,20
Hydrothermal synthesis
In the twenty-first century, there is the recurrent use of nanoparticles that were created through nanotechnology. Since nanotechnology's invention in the late 1950s, its immense promise has been realised via a rapid rise in its applications in practically all spheres of life, and in particular, in the realms of medicine and health.21 This method has been used to successfully synthesise a wide variety of nanomaterials. The hydrothermal synthesis approach has several benefits as compared to other ones. Nanomaterials produced by hydrothermal synthesis may not be stable at high temperatures. The hydrothermal approach allows for the production of nanomaterials with high vapour pressures while minimising material loss. Through liquid phase or multiphase chemical processes, the compositions of the nanomaterials to be synthesised may be tightly regulated during hydrothermal synthesis. Moreover, it is a solution reaction-based approach in which nanomaterials are formed in wide temperature and pressure range conditions. Multiphase or liquid phase chemical reactions can be used to control the composition of the nanomaterials.22
Supercritical CO2-assisted phase inversion technique
The utilisation of supercritical CO2 processes holds promise for creating goods with applications in biomedical engineering. The end results of these processes, such as aerogels, foams, membranes, fibres, liposomes, particles, and impregnated polymers, are not frequently employed in hospitals or during surgical operations.23 SC-CO2 acts as the solvent for the liquid to dissolve the polymer and as the non-solvent for the polymer. This results in the formation of a phase inversion process with the formation of supercritical discontinuous and continuous polymer phases to produce a porous interconnected structure. SC-CO2 dries the polymer membrane without allowing the collapse of the structure due to the absence of a liquid–vapour interface. No pot treatment is required because there is no trace of organic solvent. It is also easy to recover the solvent because the separator located downstream of the vessel for membrane formation can remove the solvent dissolved in supercritical CO2 from gaseous CO2.24–27
Hydrogel preparation
Presently, wounds are a leading cause of mortality. There is currently no effective and commonly used wound dressing that can result in significant relief, especially in chronic wounds that cause patients a considerable deal of discomfort. As such, new, multipurpose wound dressings must be created. Because of their 3D structure, superior permeability, outstanding biocompatibility, and capacity to create a moist environment for wound healing, which solves the drawbacks of conventional dressings, hydrogels are a suitable dressing choice.10
They are degradable polymeric networks that are formed and degraded as a result of crosslinking and this leads to the multidimensional extension of polymeric chains. These natural hydrogels have a good water absorption capacity, long service life, and high gel strength. The classical chemical approaches to preparation include one-step procedures like parallel cross-linking of monomers and polymerisation.28,29
One pot radical polymerisation
Different polymers may be used to provide the best materials for making wound dressings. They fall within the categories of synthetic polymers and biopolymers. A chain polymerization is initiated by an initiator to form a reactive species such as a cation or anion and many monomer molecules are added to continuously propagate the reactive center.30
Freeze gelation technique
The freeze gelation process has been used to fabricate three-dimensional porous scaffolds. Due to their distinctive adjustable features, the construction of scaffolds utilising polymer mixes has recently become popular for numerous tissue engineering applications. The most widely used, yet inefficient and expensive way of creating porosity in scaffolds is freeze-drying.31 It involves a sol–gel process to induce the gelation of the polymer, using chitosan or gelatin as the bio-polymers, and is used for biomedical applications without high-temperature sintering.32
Successive ionic layer absorption and reaction deposition technique
One of the simplest techniques in terms of better substrate flexibility, large-area fabrication capability, deposition of a stable and adherent film, low processing temperature for the fabrication of the film, and reproducibility is the successive ionic layer absorption and reaction (SILAR). This method saves a lot of money because it doesn't need expensive equipment. Because different fabrication parameters, including solution concentration, precursors, the number of cycles during immersion, pH, annealing, doping, and growth temperature, impact the rate of fabrication and the structural, optical, and electrical properties of the thin films produced, it has become necessary to conduct a thorough analysis of this special fabrication method.33
This is based on the deposition of ionic species on a surface, which is followed by a reaction triggered by the successive adsorption of other ionic species for the formation of an insoluble product, which accounts for the film coating usually at room temperature and pressure.34
Solvent casting method
The most used technique for creating wound dressing films is solvent casting. Enhanced gas permeability and wound monitoring are provided by wound dressing films, which are adaptable and simple to use.35 This is a process of forming a thermoplastic polymer by dipping a mold into a polymer solution and removing the solvent to leave a film of the polymer on the mold to form a membrane or scaffold.
Force spinning method
A new approach called force spinning reduces the drawbacks of electrospinning and gives it more benefits. Drug delivery issues including instability and hydrophobicity are solved using polymer-based nanofibers whose benefits include stability, solubility, and drug storage.36 This method uses solid materials or solutions that are melted into spinnerets and spun into nanofibers by centripetal forces. There is no need for solvent recovery because no solvent is used. The parameters that control the fiber diameter include spinneret selection, rheological properties, rotation speed, temperature and environment.37
Ionic gelation method
Calvo et al. (1997) developed the ionic gelation approach, a chemical procedure for creating microparticles or NPs, which relies on electrostatic interactions between ions of various charges. Alginate and polymeric materials, typically CS, are needed for this method.38 The ionic gelation procedures may be carried out easily, gently, and rapidly in typical laboratories since it just needs inexpensive, basic, and readily available ingredients and equipment. Additionally, because the method relies on electrostatic contact rather than a chemical reaction, organic solvents are not required, and potentially harmful substances or reagents are also avoided. This method's drawbacks include the difficulty in producing evenly sized NPs and the paucity of research on different polymers (other than CS).39 It is a process for the synthesis of nanoparticles in which polyelectrolytes crosslink with counter ions. Chitosan is a polysaccharide that has a positive charge and undergoes ion gelation to form spherical particles.40
Solvent exchange method
This is a novel strategy for creating hybrid alginate–chitosan aerogel fibres and assessing their potential for use in wound healing. The advantage of chitosan's composition and molecular weight regarding fibres has led to adapting emulsion-gelation to create polyelectrolyte complex hydrogels from both polymers to create the fibres. Alcogels were created by converting hydrogels by solvent exchange and then drying them with supercritical CO2.41 A solid polymer forms at the interface between an aqueous solution and a solution of the water-insoluble polymer. A micro-dispenser system consists of two ink jet nozzles that continuously produce reservoir-type microcapsules that consist of an aqueous core surrounded by a thin polymer membrane (Fig. 1).42
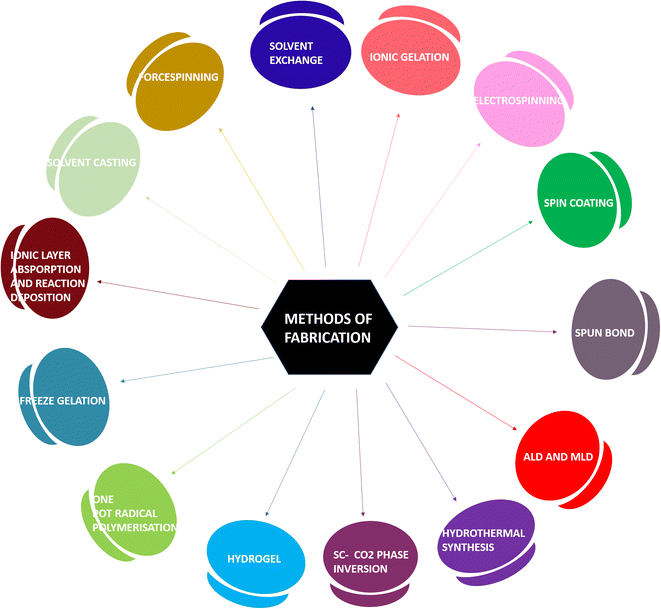 |
| Fig. 1 Different strategies for the fabrication of wound dressings and hydrogels. | |
3. Phytochemicals: natural occurrence, source and chemistry
Curcumin (1,7-bis(4-hydroxy-3-methoxy)-1,6-heptadiene-3,5-dione), also called diferuloylmethane, is an active ingredient (polyphenol) derived from turmeric, the rhizome of Curcuma longa and other Curcuma species.43
EGCG (−)-epigallocatechin-3-gallate is a major polyphenolic compound (catechin) found in green tea, Camellia sinensis.44
Tannic acid is a naturally occurring water-soluble polyphenol that consists of the central glucose molecule and one or more galloyl residues derivatizing the hydroxyl groups. It is found with other condensed tannins in beverages such as green tea, black tea, coffee, beer, and red wine.45
Cellulose is a polysaccharide comprised of a linear chain of units of β(1,4)D-glucose units and is an important structural component of the cell wall of algae, green plants and oomycetes.46 Bacterial cellulose is an extracellular polysaccharide produced by Acetobacter xylinum bacteria into long ribbon-like microfibrils.47 Ascorbic acid is a water-soluble vitamin found in citrus fruits, oranges, strawberries and peppers. The keto lactone with two ionisable hydroxyl groups usually exists as the ascorbate monoanion at physiological pH. It is an excellent reducing agent and undergoes one-electron oxidation to form the ascorbate radical and dehydroascorbic acid.48
Aloe vera consists of phytochemical classes such as phenolic acids (caffeoylquinic acid hexoside and 3,4-O-(E) caffeoyl feruloyl quinic acid), anthraquinones (aloeresin E, isoaloeresin D and 2′-O-feruloyl aloesin), and flavonoids (lucenin II, vicenin II, and orientin).49
Quercetin (5,7,3′,4′-flavan-3-ol), a pentahydroxyflavone with five hydroxyl groups, is an abundant flavonoid found in apples, onions, red wine and berries.50 It has four active groups: a dihydroxy group in the A ring, o-dihydroxy group B, C ring C2, C3 double and carbonyl groups. The phenolic group and double bonds present rationales for the biological properties of quercetin.51
The plant kingdom has exuberant flora and medicinal plants have been known to provide a secure, accelerated recovery with minimal side effects from ailments due to the active compounds in them and are a substantial resource for constituents that are extensively used for drug development. The organic constituents are typically the secondary metabolites produced by the plants as a consequence of physiological stress and are not primarily involved in the normal growth, maturation and reproduction of the life form. The phytochemicals are known to have antioxidant, anticarcinogenic, antifungal, antibacterial, anticonvulsant, anti-inflammatory, and analgesic properties that are beneficial to both humans and animals. Accordingly, the functional groups in the mentioned phytochemicals play a vital role in wound healing. It is imperative to scrutinize the active compounds that naturally occur in plants for the development of dressings (Fig. 2).
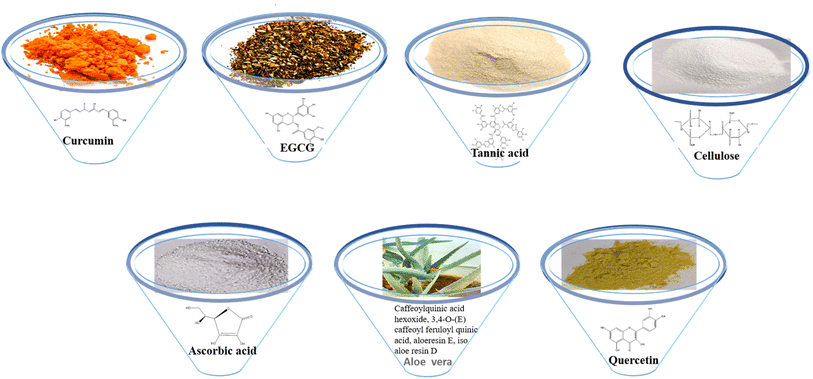 |
| Fig. 2 Phytochemicals: source, occurrence and chemical structure. | |
4. Naturally occurring materials having antiviral and antibacterial potential and their mechanisms of action
Curcumin
Curcumin inhibited haemagglutination in H1N1 and H6N1 subtypes, which developed resistance to amantadine. The synthesis of viral proteins like neuraminidase (NA), matrix protein (M1), and haemagglutinin (HA) was delayed in MDCK cells by curcumin. Incubation of the IAV (influenza A virus) with curcumin prior to viral attachment completely terminated plaque formation. The haemagglutinating (HA) interference assays showed the compounds lacking one methoxyl group (curcumin II–demethoxycurcumin), or both methoxyl groups (curcumin III–bisdemethoxycurcumin) exhibited similar strength to curcumin. This indicates that the presence of the methoxyl groups does not play a significant role.52 Curcumin showed antibacterial activity against Gram-positive bacteria (Enterococcus faecalis and Staphylococcus aureus) and Gram-negative (Pseudomonas aeruginosa and Escherichia coli). There was membrane leakage in the bacteria and this was exhibited using fluorescent probes, calcein and propidium iodide. Curcumin, being lipophilic, enters the bilayer organization and this is due to the interaction of curcumin with the polar head group of the lipid bilayer.53,54
EGCG
The 3-galloyl group and 5-OH group of the catechin skeleton in EGCG play a role in the antiviral activity and the antiviral effect on the influenza virus is due to its capacity to alter the physical properties of the viral membrane.55 It interferes during the early stages of infection such as the attachment of the virus, its entry and membrane fusion with the host cell. Its virucidal activity can be increased by the incorporation of fatty acids as this would increase the phenolic hydroxyl groups, which in turn increases viral and cellular membrane permeability.56 The recovery acceleration during wound healing is due to the antioxidant activity for speeding up the vessel formation on the skin and its anti-inflammatory activity.57 The galloyl moieties are efficient as they can partition into biological membranes of the bacteria, perturb the membrane and reduce free radical diffusion and affect enzyme activities, and regulate the functions of membranes and protein in membranes.58 Hydrogen peroxide generated by a one-electron reduction to dissolved oxygen from catechin has a bactericidal effect; catechin also has physical binding properties to lipid bilayers and also cell membrane proteins, thereby enhancing the bactericidal activity.59
Tannic acid
Tannic acid's activity against viruses is related to its interference with the viral adsorption onto the cell membrane, and might be effective in blocking the NoV P protein of the norovirus binding to HBGA receptors.60 The polyphenolic hydroxyl groups with one or more galloyl residues of tannic acid and their hydrophobic ‘core’ and hydrophilic ‘shell’ are also responsible for the antioxidant activity, which might contribute to the inhibitory effect on replication.61 Tannic acid acts as an inhibitor of the NorA efflux pump and this is considered as the main mechanism that is responsible for resistance against S. aureus.62 The inhibition of bacterial growth observed in E. coli, P. aeruginosa, Y. enterocolitica, S. aureus, B. cereus and L. innocua might be due to the inhibition of sugar and amino acid uptake by tannic acid.63
Cellulose
The antiviral or antibacterial properties can be developed by the incorporation or coating of antimicrobial phytochemicals. Bacterial cellulose dressings were made antibacterial by loading with amoxicillin and bacitracin, which are antibacterial agents, and showed activity against S. aureus and E. coli.64 DAC (dialdehyde cellulose)/GO (graphene oxide)/amino acid (cysteine (Cys) and methionine (Meth)) nanocomposites were prepared in which both DAC/GO/Cys and DAC/GO/Meth showed good antimicrobial activity against E. coli, P. aeruginosa, B. subtilis and S. aureus.65 This shows that cellulose, unfortunately, does not possess any antiviral or antibacterial activity but can be modified by incorporating biocompatible compounds that possess these activities.
Ascorbic acid
Redox-active metals are reduced by ascorbate and on further reaction with oxygen produce superoxide; superoxide dismutase produces hydrogen peroxide and its increased levels afford the antimicrobial activity.66 In the HSV1 virus, dehydroascorbic acid (oxidized form of ascorbic acid) affects the virus during the maturation stage after the completion of viral replication and so, prevents the formation of infectious virus progeny in the infected cells.67 Vitamin C is acidic and its inhibitory activity can be altered by altering the pH; it inhibits S. aureus at a pH close to neutral. There are two possible mechanisms for this growth inhibitory activity. (1) When vitamin C is oxidized, the bacteria are exposed to oxidative stress. (2) The formation of acetic acid and lactic acid from vitamin C.68
Aloe vera
The aloe polysaccharides from aloe vera inhibit the attachment of the influenza virus to host cells and also reduce the viral shedding and clinical symptoms in influenza-affected mice. Since the inhibition effect was observed in the early stages of infection, nasal administration is also effective.69 The main mechanism of antiviral action was during the time of DNA synthesis, especially protein synthesis during the cytomegalovirus infection.70
AVG has antimicrobial activity against cariogenic and periodontopathic bacteria and it shows stronger activity against Gram-positive as compared to Gram-negative bacteria.71 This is due to the presence of pharmacologically active compounds such as aloin A (10-C-beta-D-glucopyranosyl-1,8-dihydroxy-3-hydroxymethyl-9-anthracenone), and aloe-emodin (1,8-dihydroxy-3-hydroxymethyl-9,10-anthracene dione), which are polyphenols that can inhibit protein synthesis by bacterial cells.72
Quercetin
Quercetin modulates HCV replication through its antioxidant activity as it inhibits HCV-induced ROS and RNS generation, lipid peroxidation, and accumulation.73 Quercetin interacts with glycoprotein hemagglutinin 2 (HA2) and plays a role in the initial stage of viral infection by inhibiting viral entry into the host cell.74
Quercetin strongly inhibited E. coli, S. enterica, S. typhimurium, S. aureus, and P. aeruginosa and the bacteriostatic effect was greater for Gram-positive than Gram-negative bacteria due to the difference in the cell wall. Quercetin changed the permeability of the membrane of S. aureus and increased ATP activity. It also decreased the synthesis of bacterial proteins, deterred the expression of proteins in the cell and ultimately led to cell lysis and death.75 Using the scratch wound in vitro assay, it was proved that quercetin inhibited the scratch wound closure of primary astrocytes by two pathways: (i) inhibition of G1 to S phase cell cycle transition, which further inhibits cell proliferation; (ii) the extracellular-signal-regulated kinase (ERK) pathway (Tables 1 and 2).76
Table 1 Structures of the natural phytochemicals having antiviral and antibacterial potential
Curcumin |
|
EGCG |
|
Tannic acid |
|
Cellulose |
|
Ascorbic acid |
|
Aloe vera |
Caffeoylquinic acid hexoside and 3,4-O-(E) caffeoyl feruloyl quinic acid, aloeresin E, iso aloe resin D and 2′-O-feruloyl aloesin |
Lucenin II, vicenin II, and orientin |
Quercetin |
|
Table 2 The compositions of the wound dressings and hydrogels with their antibacterial activities
Phytochemical |
Bacteria type |
Material and composition |
References |
Curcumin |
E. coli and S. aureus |
Polycaprolactone + curcumin on polypropylene spun bound membrane |
77
|
E. coli and S. aureus |
Cu(II) curcumin complex coated cotton |
78
|
EGCG |
E. coli, P. aeruginosa, B. subtilis and S. aureus |
HG–Ag–EGCG |
79
|
Bare hydrogel + Ag nanoparticles + EGCG |
E. coli and S. aureus |
CMCS (carboxymethyl chitosan)–PBA (4-formylphenylboronic acid) + EGCG + PEG–glu (gluconate-terminated polyethylene glycol) to form hydrogel |
80
|
Tannic acid |
S. aureus, B. subtilis, E. coli and P. aeruginosa |
Cotton/GPTMS (3-glycidoxypropyl trimethoxysilane)/tannic acid/MWCNT |
81
|
S. aureus, E. coli and P. aeruginosa |
CA60/TA/Zn (CTZ) hydrogel |
82
|
S. aureus and E. coli |
TA-reinforced CSMA/SFMA hydrogels |
83
|
S. aureus and E. coli |
QT hydrogel |
84
|
S. aureus and E. coli |
TA@bilayer hydrogel |
85
|
Cellulose |
E. coli and B. subtilis |
Diclofenac containing cellulose film |
86
|
S. aureus
|
Lysostaphin (Lst), a cell lytic enzyme functionalized cellulose |
87
|
E. coli, S. aureus and P. aeruginosa |
AgNP-bacterial cellulose hybrid gel membranes |
88
|
E. coli and S. aureus |
Bacterial cellulose dressings loaded with amoxicillin and bacitracin |
63
|
Ascorbic acid |
E. coli and S. aureus |
ZnO–ascorbic acid |
89
|
Aloe vera |
E. coli and S. aureus |
SH (sodium hyaluronate), CS (chitosan), and DA (dopamine) in natural DES (deep eutectic solvent) + aloe vera gel |
90
|
Quercetin |
E. coli and S. aureus |
Quercetin–chitosan–fibrin scaffold |
91
|
E. coli and S. aureus |
Poly lactic acid, graphene oxide, quercetin scaffold |
92
|
S. aureus
|
Polycaprolactone nanofibers was fabricated with graphene oxide/quercetin nanocomposites |
93
|
Open wounds are common, as a result of cuts, burns and surgical incisions. Environmental conditions or improper care result in infection. Gram-negative bacteria, such as S. aureus and Gram-positive bacteria, namely, E. coli and P. aeruginosa, are predominantly responsible for wound infections and contaminations.94 Wound healing is delayed due to the viral alteration of macrophages, especially in second-degree wounds.95 Numerous strategies can be adopted to incorporate antimicrobial agents into the dressings as they behave as biocides for killing and inhibiting microorganisms that are intact on the wounds during wound healing.96 This amalgamation of antimicrobial agents with the dressings and hydrogels results in an increased therapeutic outcome and assists in the healing of the infected wound.
5. Wound healing activities: mechanism of wound healing
Wound healing occurs in four phases:
Homeostasis
Once the skin is injured, the exposure of collagen initiates a clotting cascade in which additional platelets are recruited by the activated platelets to the homeostatic plug. These activated platelets cause the negatively charged phospholipid to move from the inner to the outer leaflet of the membrane bilayer and vesicles containing anionic phospholipids are released. These vesicles and activated platelets provide a binding site for enzymes and cofactors and thrombin is generated.97 Thrombin binds to procoagulant substrates such as fibrinogen to activate them to convert them into fibrin, which further initiates the process of activating factor XIII (FXIII), which is crucial for efficient homeostasis.98 The fibrin forms a mesh to trap the blood cells and platelets.
Inflammation
A localized swelling is observed due to the leaking of blood vessels. Neutrophils and monocytes rapidly migrate to this site, which stimulates the secretion of growth factors and proteins. This controls bleeding and prevents any infection. Vascular endothelial growth factor, which is secreted from the monocyte-derived macrophages, plays an important role in the inflammatory response during the healing of the wound because its release and angiogenesis are important.99
Inflammation is accompanied by redness, heat and pain.
Proliferative phase
At the beginning of this phase, fibroblasts start to appear at the site of injury under the influence of some chemoattractants that are released or produced from the matrix of macrophages and platelets, such as platelet-derived growth factor (PDGF), interleukin-1 beta (IL-1β) and tumor necrosis factor-alpha (TNF-α) as part of the inflammatory response where it penetrates the fibrin and degrades it.100,101 This is replaced by an extracellular matrix that is composed of components such as collagen, glycoproteins, glycosoaminoglycans, and hyaluronic acid.102
The myofibroblasts (activated fibroblasts), which are contractile, smooth muscle-activating cells also help in the contraction of the granulation tissue. The tissue also receives oxygen and nutrients with the help of blood vessels built.
Keratinocytes usually contribute to the production of cytokines and help in the pathogenesis of wound healing. They produce antimicrobial peptides that kill invading pathogens.103 They are also responsible for restoring the epidermal layer by a process called re-epithelialization and the failure of keratinocytes to maintain the barrier can lead to wound recurrence.104
Remodeling/maturation phase
In this phase, the granulation tissue matures into a scar and the tissue tensile strength is increased. This scar is then remodeled by the ECM, which is similar to skin, and this occurs through the action of different classes of proteins produced during wound healing such as matrix metalloproteinases and serine proteases (Fig. 3).105
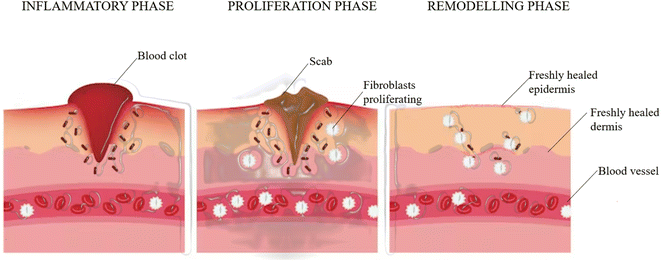 |
| Fig. 3 Phases of wound healing. | |
Wound healing activity of the considered phytocompounds
Cost-effective therapeutic agents are essential in comparison to reference drugs due to their availability. The active phytochemicals fabricated into the wound dressings play a pivotal role in accelerated wound healing and optimising the formulation assists in the multifunctional application. The salient features responsible for this are the astringent, free radical scavenging, anti-inflammatory, anti-microbial and anti-oxidative properties. The mechanisms of wound healing aided by the active components are as follows:
Curcumin.
It stimulates the production of growth factors and accelerates healing and ability to reduce the body's response such as inflammation and oxidation and enhance granulation, collagen synthesis and wound contraction.106
EGCG.
The recovery acceleration during wound healing is due to the antioxidant activity of EGCG, which is beneficial for speeding up vessel formation on the skin and its anti-inflammatory activity.57
Tannic acid.
This is a natural polyphenol astringent that can accelerate wound healing by modulating the inflammatory growth factors and cytokines and activating the Erk ½ (extracellular signal-regulated kinase ½) cascade by increasing the cell growth by stimulating the bFGF (basic fibroblast growth factor).107
Cellulose.
Its wound healing capacity can be attributed to its high tensile strength, strong water-holding capacity, and compatibility with tissues. It also allows the absorption and evaporation of exudate from the wound and allows oxygen exchange for better healing.108
Ascorbic acid.
In wound healing, ascorbic acid is necessary for collagen synthesis, angiogenesis and for promoting collagen cross-linking. In its deficiency, there is reduced collagen deposition and delayed wound healing is likely.109
Aloe vera.
Its anti-inflammatory, antiviral, and antioxidant properties, its ability to decrease histamine-induced acid secretion,110 the stimulatory effect on cell proliferation, and migration of fibroblasts and keratinocytes make it an ideal candidate for a dressing.111
Quercetin.
Its wound healing activity is demonstrated in its ability to promote proliferation and the migration of fibroblasts, reduce the level of inflammatory factors and also increase the level of growth factor through the Wnt/β-catenin pathway. Also, due to the intervention of quercetin, the expression of TERT protein can be increased.76
6. Naturally occurring materials fabricated into wound dressings and hydrogels
Curcumin
It was used as a nanocoating on a polypropylene (PP) spun bond membrane, which influenced the contact-killing efficiency against bacteria and bacteriophages.77 Curcumin was added to biobased PU in DMF and was electrospun to form fibrous scaffolds for wound dressing. The effects of the concentration of the polymer and contact angles were investigated.112 Cu(II) curcumin complex-coated cotton prepared by treating CuO cotton or pristine cotton with an ethanolic solution of curcumin showed better antimicrobial properties than curcumin or cotton against E. coli and S. aureus.78 An ethanolic solution of curcumin was incorporated into a collagen solution, which was then filtered through muslin cloth and dried in the dark under a laminar fume hood in Teflon trays and the obtained collagen films were applied in excised wounds on rats and wound healing took place.113 Electrospun curcumin-loaded mesoporous silica nanofiber mats (CCM-MSNs) prepared by the incorporation of polyvinyl pyrrolidone (as depicted in Fig. 4) can be used in the biomedical field to construct homeostatic materials for in vivo and in vitro studies of animal livers.114 A dermal wound dressing was developed, which was made up of a nano-composite hydrogel composed of curcumin, N,O-carboxymethyl chitosan and oxidized alginate. The CCS–OA hydrogel has properties like exudate absorption, immobilization, and non-cytotoxicity, and is also biodegradable and bioabsorbable. Nano-curcumin was prepared via a thin-film evaporation method using methoxypoly(ethylene glycol)-b--poly(ε-caprolactone) copolymer (MPEG–PCL) as a carrier. In an adult male SD of 10 weeks, a full-thickness dorsal wound was created. The nano-curcumin/CCS–OA hydrogel was found to have the best potential in wound healing.115 The effects of the curcumin nanoparticles-hydrogel on the full-thickness excisional skin wounds on the backs of rats in a rat model of diabetes mellitus type 1 were studied. Curcumin and Cur-NP were loaded into the N,O-carboxymethyl CS/oxidized alginate hydrogel, forming a gel-forming composite, and the rats with Cur-NP/HG showed the highest rate of wound closure, which was confirmed by the re-epithelization of epidermal cells, indicating that the hydrogel played a very important role in wound healing.116 Wound healing in adult male BALB/C mice was analyzed using dextran hydrogel and curcumin-encapsulated PEG–PLA [poly(lactide)-block-poly(ethylene glycol)] nanomicelles incorporated into dextran hydrogel to study the role of the hydrogel as a wound dressing and its capacity to control the release of curcumin. The blank dextran hydrogel was less effective as compared to the curcumin nanomicelle-loaded dextran hydrogel; due to the presence of curcumin in the hydrogel, it was released slowly during the healing and contributed to the growth of epithelial cells.117 Consequently, the approaches undertaken to fabricate the polyphenol curcumin into the dressings assisted in accomplishing a multifunctional sustainable material. Curcumin, as indicated above, protects wounds against microbial infection, reduces inflammation and induces cell epithelialization due to its anti-infectious, anti-inflammatory and antioxidant properties during various wound healing stages. Electrospinning is specifically beneficial for constructing nanofibers and these nanocomponents provide a large surface area for the incorporation of an excessive amount of polyphenol to substantiate the uniform release, which is important for durable dressings.
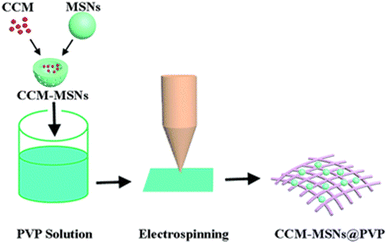 |
| Fig. 4 Fabrication of curcumin-loaded mesoporous silica nanofiber mats.114 | |
EGCG
Aminated guar gum was used to produce silver nanoparticles (Ag@AGG) from silver nitrate by reduction chemistry. Bare hydrogels were prepared using aminated GG, sodium alginate, gelatin solutions, glycerol and calcium chloride followed by lyophilization. To prepare HG–Ag–EGCG, sodium alginate, gelatin solutions, glycerol, CaCl2, Ag@AGG and EGCG were added instead of AGG before lyophilization. It showed good anti-inflammatory and antibacterial properties and demonstrated good cytocompatibility towards normal keratinocyte MSC P5 cells. This formulation also promotes rapid wound healing.79 CMCS–PBA is phenylboronic acid-grafted carboxymethyl chitosan prepared using carboxymethyl chitosan and 4-formylphenylboronic acid. Gluconate-terminated polyethylene glycol (PEG–glu) was prepared according to a previous report,118 where CMCS–PBA, EGCG and PEG–glu were dissolved in deionized water in different proportions to form different hydrogels by borate ester crosslinking between diol groups of EGCG and PEG–glu and phenylboronic acid (PBA) groups of phenylboronic acid-grafted carboxymethyl chitosan (CMCS–PBA). The hydrogels were desirable because EGCG provides antioxidant properties by scavenging free radicals and has excellent antibacterial capability against S. aureus and E. coli. As such, the hydrogels were good wound dressings for female Kunming rats employed in the experiment.80 Poly(lactic-co-glycolic acid) (PLGA) in DMF and THF and EGCG were electrospun into membranes that were then sterilized with 25 kGy gamma irradiation. The fibre diameter was decreased by increasing the concentration of EGCG due to its increased molecular weight. The EGCG helped in wound healing in adult human dermal fibroblasts (HDFs) but on increasing the concentration (i.e. 5% EGCG–PLGA), it showed cytotoxicity because HDFs lost their spindle shape and exhibited a round shape.119 EGCG–NapFFY supramolecular hydrogel was prepared using a 0.01 M phosphate-buffered saline (PBS, pH 7.4) solution of the hydrogelator NapFFY and a PBS solution of EGCG. The 1
:
2 EGCG–NapFFY helped in decreasing the wound area, which was made by making an incision of 1 cm in mice that were anesthetized by intraperitoneal injection of 0.5% pentobarbital sodium and proved that this hydrogel can be used for wound healing.120 Fiber matrices of HA/PLGA-E core/shell prepared by electrospinning hyaluronic acid (HA), poly(lactic-co-glycolic acid (PLGA) and EGCG were used to explore wound healing in streptozotocin-induced diabetic rats and normal rats and the rate was accelerated with the HA/PLGA-E matrices and can be considered as a novel scaffold for diabetic wound healing.121 3-Acrylamido phenylboronic acid (APBA) with EGCG formed boronic esters as crosslinkers to form the E–A complex and this was further used to prepare the EACPA E–A complex-based polyacrylamide hydrogel by a one-pot radical copolymerization with acrylamide (AM) in the presence of N,N,N,N-tetramethyl ethylene-1,2-diamine (TEMED) and ammonium persulfate (APS) (as depicted in Fig. 5a). Streptozotocin (STZ)-induced diabetic C57BL/6 mice with wounds had a better healing efficiency and avoided secondary injury of the wound site (as depicted in Fig. 5d) due to the presence of EGCG.122 A green tea ointment was made using a hydro-alcoholic extract of green tea and the yield was mixed with distilled water, hydrocarbon bases and Eucerin to yield a 1% green tea ointment. Ulcer healing was monitored for leprosy patients using the ointment and control (FGD – framycetin gauze dressing) and it was confirmed that the EGCG 1% ointment improved the healing rates as compared to FGD.123 Glycol chitosan was combined with EGCG to form the EGCG–chitosan hydrogel, which showed good antibacterial activity toward Escherichia coli and Staphylococcus aureus. On increasing the EGCG concentration, the wound closure was accelerated.124 As indicated above, EGCG functions as a powerful antioxidant and also inhibits the early stages of infections, which is critical to avoid delayed wound healing. It also plays an important role in providing a microenvironment that is essential for better functioning of the key factors involved in improved rates of wound healing.
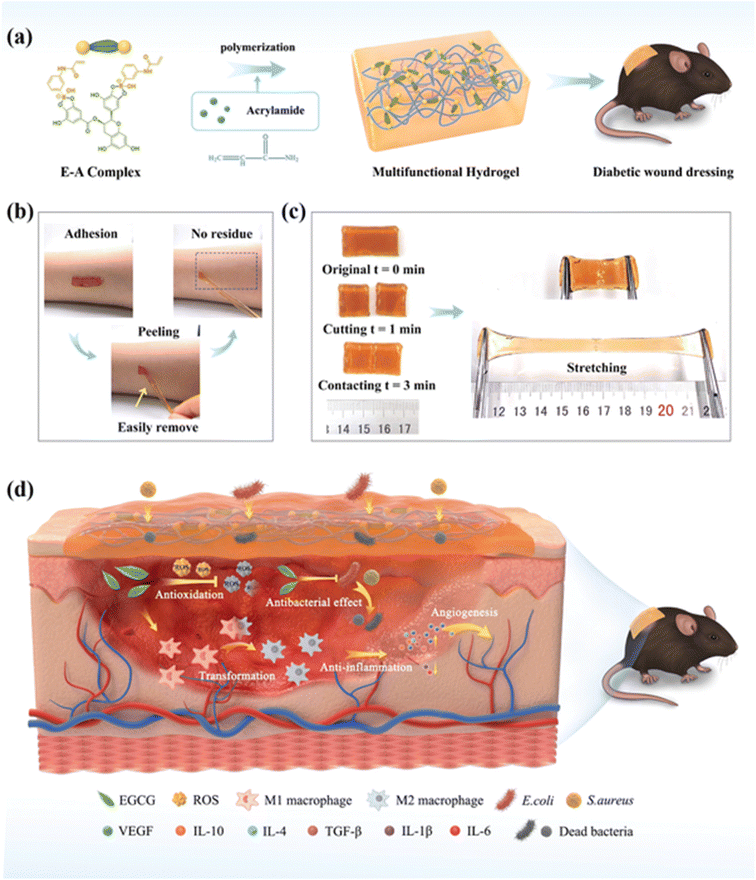 |
| Fig. 5 (a) Preparation of the EACPA hydrogel. (b) Depiction of no residue after adhesion and peeling. (c) Depiction of the ability of the self-healed hydrogel to withstand external force. (d) The mechanism involved in chronic wound treatment in streptozotocin (STZ)-induced diabetic C57BL/6 mice by the hydrogel.122 | |
Tannic acid
An acetic acid solution of chitosan and tannic acid was treated with an iron(III) solution. The material obtained after evaporation with chitosan and the TA–Fe(III) complex was subjected to contact angle and maximum tensile strength measurements. The rate of haemolysis was also measured using sheep blood. Tannic acid can improve the mechanical properties and degradation of chitosan fibers and can, therefore, be used as a wound dressings.125 The medical adhesive TASK (prepared as depicted in Fig. 6) is composed of tannic acid and silk fibroin, which was used as a lyophilized powder. Wet tissue adhesion, and self-healing properties of TASK were provided by SF, and tannic acid provided its wet-resistant adhesion resulting from the abundant anchor moieties in its structure.126 The CA60/TA/Zn (CTZ) hydrogel prepared from carboxylated agarose, CA60, TA and ZnCl2. Zn2+ ions provided the best control over the release of TA. The antibacterial activity of hydrogel CTZ was observed against E. coli, S. aureus, and P. aeruginosa using an agar disc diffusion test. E. coli was used because it is one of the causative agents of burn wound infections, and the hydrogel exhibited remarkable activity. This makes it a suitable candidate to be used for wound dressings.82 The TATA supramolecular hydrogel was formed by copolymerization between the crosslinker, tannic acid and thioctic acid monomer, followed by freeze-thawing to produce hydrogen bond cross-linking for hydrogel formation. The amount of tannic acid determines the state (amorphous) of the hydrogel formed. The wound healing property of TATA hydrogel was checked on a rat model with an incision. On treatment with the TATA hydrogel, the incision was restored by the basement membrane and, therefore, no deep opening could be observed.127 TA-reinforced CSMA (methacrylated chitosan)/SFMA (methacrylated silk fibroin) hydrogels were prepared and they showed wound healing ability that was evaluated using the ICR mice model. The wound area had drastically decreased in the case of hydrogels with increasing TA percentage concentration. The antibacterial activity of the hydrogel was demonstrated against S. aureus and E. coli. TA-reinforced hydrogels performed significantly better in comparison with hydrogel without TA, which is mostly because of the combined antibacterial ability of chitosan and TA.83 QT hydrogels were prepared by introducing tannic acid (TA) into a quaternized chitosan (QCS) matrix. A full-thickness STZ diabetic wounded rat was used to evaluate the wound-healing effect of the QT hydrogel. The QT hydrogel could promote wound healing and skin tissue regeneration. It also showed antibacterial activity against S. aureus and E. coli.84 Bilayer hydrogel was prepared (as depicted in Fig. 7) in which the bottom layer was composed of carboxylated chitosan (CCS), poly(vinyl alcohol) (PVA) and polyethylene glycol (PEG) and the top layer was composed of hyaluronic acid (HA), PVA, and PEG, which was further cast in a mold. This bilayer hydrogel was immersed in a TA aqueous solution to acquire the TA@bilayer hydrogel. The TA@bilayer hydrogel inhibited the growth of E. coli and S. aureus.
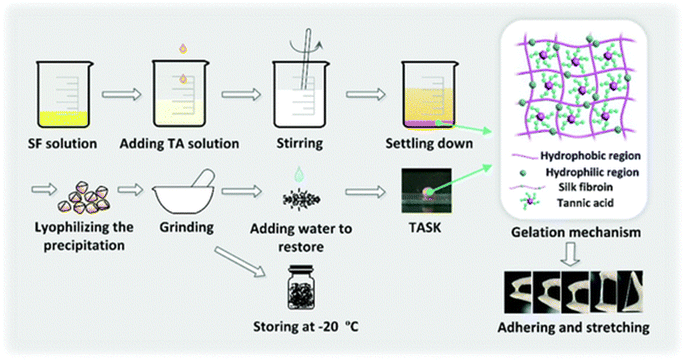 |
| Fig. 6 Preparation of TASK.128 | |
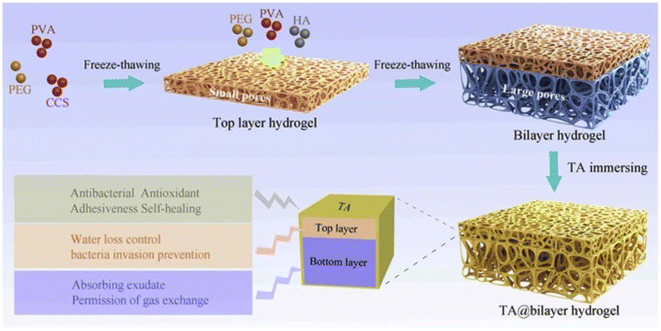 |
| Fig. 7 Preparation process of TA@bilayer hydrogel.85 | |
Homeostatic performance was evaluated in rabbits with an incision in the liver. The TA@bilayer hydrogel showed less blood loss, which proved the good adhesive property of the hydrogel as it provided a good physical barrier and prevented the outflow of blood from the site of the wound. A cut on the dorsum of the rabbit was treated with the hydrogel and wound repair was observed.85 As visualized earlier, chitosan, PVA, PEG, etc. provide a base for the incorporation of tannic acid to fabricate hydrogels that possess great tissue adhesiveness and decreased water loss, which are essential for rapid wound healing. The incorporated polyphenol tannic acid aids in preventing microbial infections as evidenced by studies that claim it prevents the binding of microbes onto the wound site.60
Cellulose
Silver nanoparticles were impregnated into bacterial cellulose by immersing it in silver nitrate solution and the absorbed silver (Ag+ ions) was reduced to silver nanoparticles by sodium borohydride. This impregnated cellulose as a wound dressing showed antimicrobial activity against E. coli and S. aureus.127 Cellulose was used as the support for the incorporation of analgesics into wound dressings. Trimethylsilyl cellulose and diclofenac dissolved in THF were used to prepare DCF-containing cellulose film; the release of DCF from the film was studied over time and the addition of another layer of cellulose slowed down the release.86 Wood-based (from bleached birch pulp fibers) nanofibrillar cellulose, which was chemically unmodified, was used as a replacement for Suprathel to enhance wound healing in severely burned patients, also it detached from epithelialized skin by itself after the site was renewed.129 Bacterial cellulose was impregnated with alginate (as depicted in Fig. 8) and the efficiency of this dressing was tested on human fibroblast cells. To incorporate antimicrobial activity and for the treatment of wounds, a cationic antimicrobial agent PHMB poly(hexamethylene biguanide) hydrochloride solution was mixed with the BC/alginate dressings.130
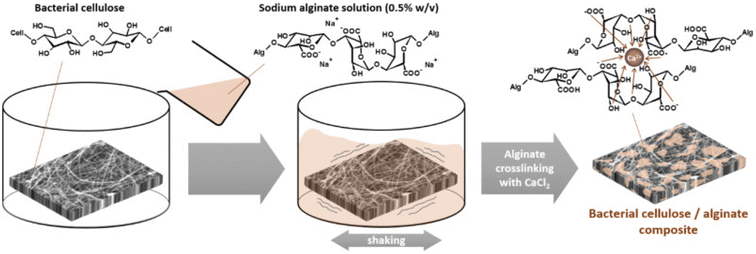 |
| Fig. 8 Fabrication of bacterial cellulose/alginate composite.130 | |
Cation exchange reactions were used to modify montmorillonite and bacterial cellulose was immersed in a homogeneous suspension of different modified MMTs (Na–MMT, Ca–MMT, Cu–MMT). After the removal of the surface suspension, the modified cellulose composites were freeze-dried and their antibacterial activities were investigated against E. coli and S. aureus, which suggested the potential use of these composites in wound dressings.131 Lysostaphin (Lst), a cell lytic enzyme, was immobilized onto biocompatible fibers made by electrospinning homogeneous solutions of cellulose [EMIM][OAc], cellulose–chitosan solution and cellulose–PMMA (poly(methyl methacrylate)) solution. These Lst-functionalized cellulose fibers were processed to obtain bandages that showed antibacterial activity against S. aureus.87 Cellulose nanowhiskers (CNWs) prepared from cellulose pulp and CNW-loaded poly(SA) hydrogel films prepared from monomer sodium acrylate (SA) were dispersed in cellulose nano-whiskers, where N,N′-methylenebis-acrylamide (MB) was used as a crosslinker; the catalyst used was N,N,N,N′-tetramethyl ethylenediamine (TEMED) and the initiator used was potassium persulfate (KPS).132 The antibiotic drug minocycline (Mic) was then loaded to investigate the antifungal and antibacterial activity and this was controlled by varying the cellulose content and amount of cross-linker (MB) in the film. The release of minocyclin was interpreted by the Schott model. These films can be used as a potential wound dressings.133 A cellulose-based super absorbent polymer was formed by combining cellulose + sodium monochloroacetate (MCA) to obtain biodegradable carboxymethyl cellulose (CMC), which was further treated with epichlorohydrin (ECH). Because of its superior swelling properties, it can be used to replace biomedical products and feminine hygiene products. Therefore, it can also be potentially used as wound dressing.134 Cellulose considered here plays a pivotal role in sustainability due to its excellent biodegradable nature, and in most approaches, it is considered as the basal layer for incorporating reference drugs. This provides the advantage of good adhesiveness to maintain water content, which is beneficial for providing an optimum environment for wound closure along with uniform drug release to prevent microbial infections.
Vitamin C
Hydrogen peroxide was generated by adding a copper/ascorbate formulation to hydroentangled nonwoven greige cotton. Each fabric was saturated by submerging it in copper(II) chloride hydrate and either L-sodium ascorbate (Na Asc.) or L-ascorbic acid (Asc. A). Cu/Asc.—analogs of cotton and ascorbic acid alone showed good activity against S. aureus and K. pneumoniae. Treatment of human dermal fibroblasts with Cu/Asc.—analogs of cotton and copper-treated cotton showed attenuated growth in comparison to untreated cotton.135 Super absorbent wound dressing prepared (as depicted in Fig. 9a) with chitosan and agarose provided accelerated healing and also an environment to support skin regeneration. Vitamin C (100/200 μg per 1 mL of prepared homogeneous mass) in the biomaterial CHN/A + vit C enhanced fibroblast proliferation as it reduced matrix metalloproteinase-2 production and promoted platelet-derived growth factor-BB synthesis by fibroblasts, which is desired during chronic wound treatment.109
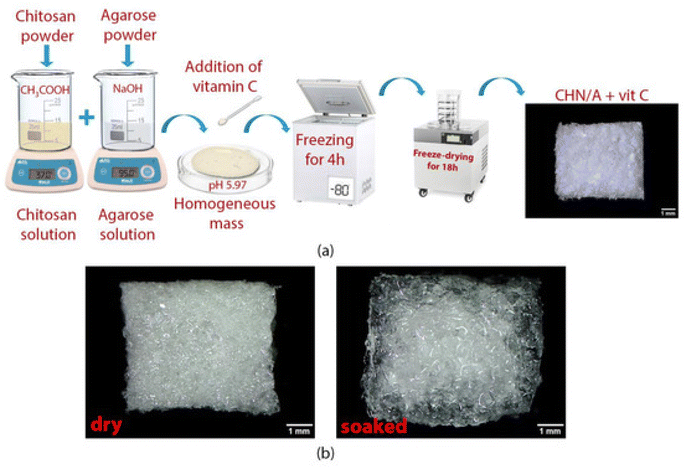 |
| Fig. 9 (a) The preparation of the CHN/A + vit C biomaterial. (b) Dry and hydrated material. (b) The exceptional ability of the material to remain intact in the presence of water makes it beneficial in wound treatment.109 | |
Different quantities of L-ascorbic acid were added to acrylamide to synthesize hydrogel specimens by a γ-ray radiation technique. The gel systems ((P(AAm)/LAA)) were good candidates to be used as wound dressing based on tensile strength, hardness, pH and degree of adhesion. Another application was the drug release of antifungal terbinafine hydrochloride (TER-HCl), which was helpful in skin wound healing.136 The electrospinning of a solution of polyvinyl alcohol and ascorbic acid or caffeine sodium benzoate (a caffeine salt that is water soluble) was carried out to prepare nanofibers. Wounded Wistar rats were treated with nanofiber mats loaded with ascorbic acid and caffeine and maximum wound healing was observed. Both the ascorbic acid and caffeine in the mat also enhanced the antifungal activity against Candida albicans.137 Ascorbic acid solvent was used to dissolve chitosan, which was linked to dialdehyde bacterial cellulose (DABC) nanofibers by a Schiff's base reaction to form an injectable hydrogel that has antibacterial properties.138L-Ascorbic acid (C6H8O6) was used as the reducing agent for the green, effective synthesis of ZnO–AA mats from zinc acetate dihydrate (Zn(CH3COO)2–2H2O) via the SILAR (successive ionic layer adsorption and reaction) technique. S. aureus was more sensitive to the antibacterial activity in comparison to E. coli.139N,O-Carboxymethyl chitosan (CMCS) was synthesized from virgin chitosan, to which polyethylene oxide (PEO) was added to form a polymer solution, to which ascorbic acid was added and the vitamin C and phenytoin sodium (PHT-Na) solutions were electrospun to form a fabricated hybrid of the nanofibrous sample. In the wounds of male Wistar rats, electrospun samples containing vitamin C and PHT-Na (1%) showed an efficient healing process as compared with vitamin C/PHT-Na (50/50) ointment and there was remarkable regeneration of the epidermis without necrosis. The rates of release of vitamin C and PHT-Na from the nanofibrous sample were also similar.140 Chitosan ascorbate is the organic salt prepared from chitosan deacylated amino groups and ascorbic acid in water and the electrospun polycaprolactone membrane was infiltrated with the prepared CA solution to obtain PCL/CA composites, which also improved the hydrophilicity and water uptake capacities of the membranes. The cell compatibility and the adhesion of human mesenchymal stem cells (hMSCs) and human umbilical vein endothelial cell (HUVEC) culture with PCL/CA were better than the pristine; therefore, the PCL/CA membranes are promising for wound healing applications.141 Wound healing in diabetes mellitus was accelerated by cellulose-based films that were prepared by the solvent casting method using cellulose extracted from rice husk and poly(vinyl alcohol) (PVA), and vit C-containing film and propolis (Prop)-containing film were prepared by adding vit C and Prop respectively after the cellulose addition step in the protocol. Diabetes-induced mice treated with streptozotocin showed increased wound healing and closure by Cel-PVA/VitC/Prop films.142 Based on the mentioned approaches, it is evident that vitamin C is crucial for all phases of wound healing. Its deficiency can hinder wound healing as revealed, and hence coupling with other reference drugs enhances the fibroblast proliferation for rapid wound closure.
Aloe vera
A conventional crosslinking method was used to prepare SA/PVA/aloe vera hydrogels using poly(vinyl alcohol), a solution of sodium alginate, ammonium persulfate and a solution of aloe vera, to which glycerine was added143 to ensure the transparency and flexibility of the membrane. The dried hydrogel was used to measure the tensile strength of the dressings and the amount of aloe vera incorporated affected this; no burst effect was observed during the release of aloe vera, which was beneficial during wound healing. No toxicity was observed on the normal human dermal fibroblasts (NHDF) by the fabricated membrane and it actively improved the wound-healing efficiency.144 Periodate-oxidized pectin (OP) was crosslinked with gelatin to prepare OP–gelatin matrices. To this, aloe powder, curcumin and a combination of both aloe and curcumin were added in different matrices and non-woven cotton fabric was dipped in solutions to form three different bio composite dressings (as depicted in Fig. 10). An incision in the NIH3T3 mouse fibroblast cells was used to test the efficiency of the OP–gel–drug dressings and it was found that OP–gel–aloe–curcumin and OP–gel–curcumin dressings induced apoptosis, whereas the OP–gel–aloe dressing exhibited good healing and was a good substrate for cell attachment and proliferation.145
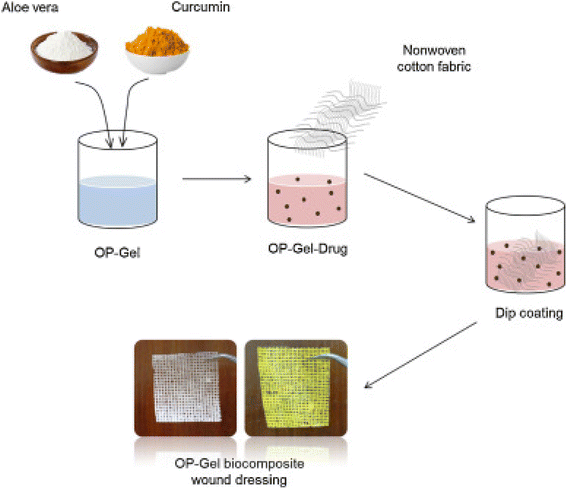 |
| Fig. 10 OP–gel wound dressings preparation.145 | |
The DES–SH–CS/DA/AV hydrogel was prepared using SH (sodium hyaluronate), CS (chitosan), and DA (dopamine) which were first mixed in the natural DES (deep eutectic solvent) with stirring, followed by the addition of aloe vera gel and the hydrogel was formed via hydrogen bonding and molecular crosslinking. Mouse NIH-3T3 fibroblast cells were used as model cells and they showed good cell viability on using the hydrogel, and the cell viability of S. aureus and E. coli colonies decreased. It also promoted good wound healing and wound closure rates.146 The force-spinning process was used to produce nanofibers using aloe vera extract, distilled water, chitosan, citric acid and pullulan, which showed antibacterial activity against E. coli. NIH 3T3 mouse embryonic fibroblast cells were taken as a model and it was observed that the NFs were non-toxic to the cells and their porous and thermal stability made them a potential candidate for wound dressings.90 The electrospinning process was used to fabricate nanofibrous membranes using rhEGF (recombinant human epidermal growth factor), aloe vera, and PLGA (polylactic-co-glycolic acid) to form the PLGA–AV–EGF nanofibrous membrane. In db/db mice, the wound healing and closure were tested via the in vivo full-thickness wound healing assay and PLGA–AV–EGF nanofibers with a high concentration of aloe vera are a suitable dressing for the treatment of chronic wounds.147 Chitosan-based hydrogels were prepared using chitosan, acetic acid, crosslinker (diacrylate (poly(ethylene glycol))), photoinitiator (2-hydroxy-2-methylpropiophenone) and additive aloe vera. The neutral red uptake (NRU) assay and MTT reduction assay on L929 murine fibroblasts showed that the composites did not induce cytotoxicity and increased the viability of the cells, and can find application in wound healing due to the biomedical properties of aloe vera.148 The time taken for partial thickness burns to heal was shortened and this was more effective with aloe gel in comparison to silver sulfadiazine cream; the better healing of burn injuries is due to aloe vera's anti-inflammatory properties.149 Aloe vera is already familiar as a folk remedy for the treatment of minor wounds and burns due to its stimulatory and anti-inflammatory effects in comparison to over-the-counter drugs as it can retain moisture content and the presence of glucomannan assists in the regeneration of cells.150
Quercetin
A chitosan–fibrin (CF) composite scaffold was prepared by mixing an acidic solution of chitosan with an alkaline solution of fibrin, which was then dialyzed, followed by the addition of quercetin and lyophilized then dialyzed to obtain the quercetin–CF scaffold. It showed good activity as a wound dressing on open excision wounds on male albino rats and also showed good antibacterial activity against E. coli and S. aureus. The Q–CF scaffold showed excellent biocompatibility, which was evaluated by the MTT cell proliferation assay.91 Quercetin was incorporated into collagen and it showed a shrinkage temperature of 50 °C, which was greater than the shrinkage temperature of collagen itself (i.e. 40 °C), and QIC had a greater thermal stability. QIC also healed wounds on male albino Wistar rats and scavenged free radicals as compared to a normal collagen matrix.151 Electrospinning (as depicted in Fig. 11) was used to prepare micro-scaffold matrices using polylactic acid, and graphene oxide. Quercetin was loaded onto the fibres to form PLA/GO/Q scaffolds. An electric field was applied and it showed that the rate of quercetin release was much better in comparison to normal drug release. It also showed good antibacterial activity against S. aureus, E. coli and Candida albicans. The scaffold was biocompatible with the L929 fibroblast cell line. On increasing GO, the swelling ability increased for water uptake and the incorporation of GO induced the electric triggering capacity and personalized release.92
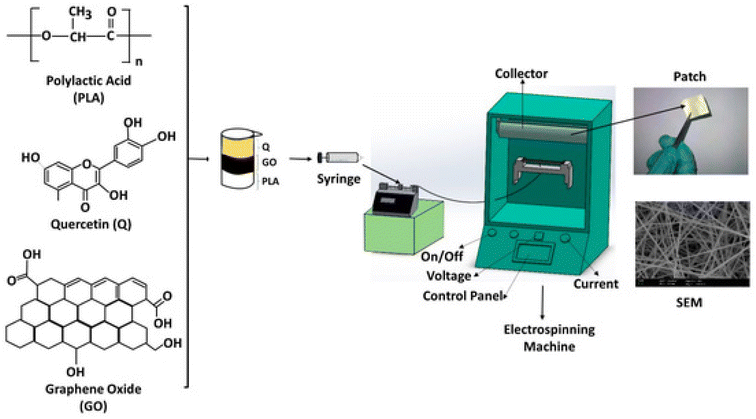 |
| Fig. 11 Fabrication of PLA/GO/Q by electrospinning.92 | |
Sodium tripolyphosphate was added dropwise to a solution of chitosan and quercetin for the adsorption of quercetin on chitosan nanoparticles. The drug-loaded chitosan nanosuspension was coated on alginate for the crosslinking of alginate. A gel formulation of quercetin-loaded chitosan/alginate nanoparticles was prepared using Carbopol 934. An excisional wound developed on Wistar rats received doses of a gel formulation of Q–ALG/CSNPs, and hydroxyproline and hexosamine markers for healing were estimated from the granulation tissue. There was the sustained release of quercetin, which can be correlated with the therapeutic mechanism responsible for enhanced wound healing and so the Q–ALG/CSNPs system is a good polymer system for wound healing applications.152 Quercetin was loaded onto graphene oxide (GO) nanoparticles. Polycaprolactone nanofibers were fabricated with varying concentrations of GO/Q nanocomposites using the electrospinning procedure. The fabricated scaffolds showed good antibacterial activity against S. aureus as quercetin decreased growth by up to 56%. High cell viability, proliferation, and adhesion of NIH/3 T3 fibroblast cells proved that the scaffold has excellent biocompatibility and can be used for wound healing.93 To improve the hydrophilicity and the skin penetration of quercetin, it was added to chitosan, to which sodium tripolyphosphate was added to form chitosan-based quercetin nanoparticles by the ionic gelation method. The application of the prepared nanoparticles (0.03%) to cutaneous wounds on male Wistar rats showed lower cytokine TNF-α (tumor necrosis factor-alpha) levels but IL-10 (interleukin 10) levels were high in comparison to Pluronic F127 (20% w/v) gel. Increased VEGF (vascular endothelial growth factor) and TGF-β1 levels were observed, which were responsible for faster wound healing. A 0.03% quercetin nanoformulation was considered to be novel for wound healing.40 The solvent exchange method was used to make water-soluble quercetin borate nanoparticles by the borate esterification reaction.132 Borate ions of QuB were used as crosslinking agents for the gelation of polyvinyl alcohol to obtain QuB–PVA microgels and a transverse electrospray deposition device was used to fabricate QuB–PVA dressings. The QuB–PVA microgel had a remarkable zone of inhibition for E. coli and S. aureus. A whole cortex injury was induced in male Kunming mice and the QuB–PVA film placed on the injury showed a significant reduction in the wound area on the 7th day and, therefore, could dramatically facilitate the wound healing speed.153 The evaluation of wounded adult male Wistar rats showed that 0.3% quercetin showed enhanced wound healing and the fastest wound closure and increased oxidative stress. Tumor necrosis factor alpha (TNF-α) expression was decreased, and interleukin 10 (IL 10), interleukin-1β (IL-1β), vascular endothelial growth factor (VEGF), and transforming growth factor-beta 1 (TGF-β1) levels were also significantly higher, which were involved in collagen synthesis and angiogenesis. There was enhanced fibroblast proliferation with collagen deposition and increased myofibroblast formation, which played a pivotal role in wound contraction.154 A poly(caprolactone)-based nanofiber membrane functionalized with a combination of an antimicrobial, ciprofloxacin hydrochloride (CHL), and an antioxidant, quercetin, for accelerated wound healing, was prepared by electrospinning (as depicted in Fig. 12). The antimicrobial efficacy of the nanofiber films against S. aureus showed that electrospinning did not alter the loaded CHL activity. PCL/CHL/Que-nanofiber-treated Wistar rats showed superior healing and inhibited excess free radicals in the wound due to the release of the drug in a biphasic manner, with an initial burst and then prolonged release. The low initial burst might be due to the low solubility of quercetin at pH 7.4.155 A nano hydrogel embedded with quercetin and oleic acid showed exceptional wound healing in diabetes mellitus patients with lower limb skin wounds without any adverse drug reactions. To make the treatment much more effective Qu and OA molecules in a nano hydrogel containing hyaluronic acid were used and it was observed that post-treatment pain was reduced and tissue viscoelasticity was also improved.156 Silver nanoparticles were prepared from sodium borohydride and silver nitrate, to which PVP was added for long-term stability and to prevent AgNPs aggregation; quercetin was then added. The prepared quercetin-loaded silver nanoparticles hydrogel matrix was used for an in vivo wound healing study in diabetic-induced Swiss albino mice. It had antimicrobial activity against S. aureus and E. coli.157 The antioxidant quercetin possesses anti-inflammatory, immunomodulatory and antiviral activity. Consequently, it is incorporated into matrices to promote accelerated wound healing without any adverse reactions as it maintains hydration at the wound site and assists in restoring the skin barrier.
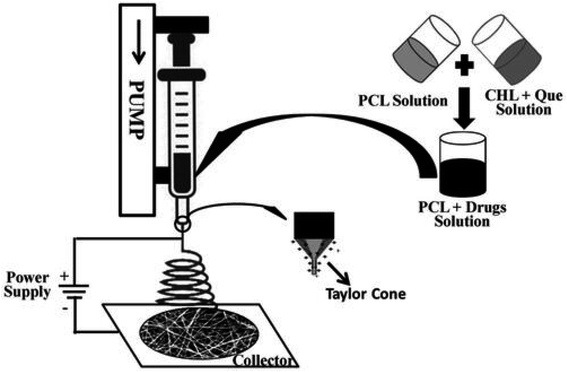 |
| Fig. 12 Fabrication of a poly(caprolactone)-based nanofiber membrane functionalized with CHL and quercetin.155 | |
There is growing evidence of the sustainable multifunctional efficacy of the mentioned dressings fabricated with active components for protection against scarring and infection during wound healing. Considerable applications reviewed here exhibit self-healing ability for extending the lifespan of the material as observed in TASK, where the lyophilized powder was condensed when exposed to water.126 Moreover, shape memory and self-healing properties were exhibited in the case of hydrogels prepared from TA and PVA due to the presence of intramolecular and intermolecular hydrogen bonds formed between the TA and PVA molecules.85 The reversibility of boron ester bonds also assists in the self-healing ability of the fabricated QuB–PVA films.153
CS–DABC hydrogels also exhibit self-healing properties due to the presence of dynamic Schiff base networks and hydrogen bonding.138 The durability of the antimicrobial activity was exhibited by the retention of 50% of the activity on laundering the polyphenolic material-coated dyed cellulose once. Similarly, the durability of the antibacterial activity of MWCNT and tannic acid-treated fabric was exhibited after several washing cycles since tannic acid plays the dual roles of maintaining the functionality of the treated fabric and stabilisation.81 These sustainable approaches are crucial for waste minimization and the preservation of natural resources (Fig. 13).
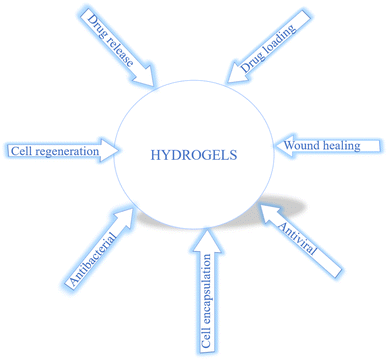 |
| Fig. 13 Application of hydrogels. | |
The reaction of one or more monomers produces a water-swollen, yet water-insoluble crosslinked polymeric network. The potential of hydrogels to absorb water arises due to the functional groups that are attached to the polymeric network. They have a great degree of flexibility, similar to natural tissue because of their water content.29 They can provide a moist environment for wound healing and have control over wound exudates.127
Hydrogels can be stabilized by physical or chemical crosslinking. Physical crosslinking is preferred over the chemical method because it does not involve the use of crosslinking agents or organic solvents, and toxicity issues can be avoided.158 They also provide the flexibility and elasticity required for adapting to wounds in different parts of the body.
They serve as a 3D scaffold that provides temporary support for cell growth and healing.159 It can also modulate wound bed environments to allow keratinocyte migration, which is crucial for wound healing.134 They also provide a source for entrapped molecules such as antibiotics or antiviral or antibacterial compounds to enter the wound (Table 3).
Table 3 Composition of the wound dressings and hydrogels developed for efficient wound healing
Phytochemical |
Material and composition |
References |
Curcumin |
Curcumin was added to bio based PU electrospun to form fibrous scaffolds |
160
|
Nano-curcumin/CCS–OA hydrogel |
115
|
Curcumin nanoparticles–hydrogel (Cur-NP/HG) |
116
|
Curcumin encapsulated PEG–PLA (poly(lactide)-block-poly(ethylene glycol) nanomicelles incorporated into dextran hydrogel |
117
|
EGCG |
HG–Ag–EGCG |
79
|
Bare hydrogel (using aminated guar gum) + Ag nanoparticles + EGCG |
CMCS (carboxymethyl chitosan)–PBA (4-formylphenylboronic acid) + EGCG + PEG–glu (gluconate-terminated polyethylene glycol) to form hydrogels |
80
|
Hyaluronic acid (HA)/poly(lactic-co-glycolic acid (PLGA)/EGCG fiber matrices |
121
|
EGCG–NapFFY supramolecular hydrogel |
120
|
3-Acrylamido phenylboronic acid (APBA) with EGCG to prepare EACPA E–A complex-based polyacrylamide hydrogel |
122
|
EGCG–chitosan hydrogel |
124
|
Tannic acid |
Chitosan and TA–Fe(III) complex |
125
|
TASK was composed of tannic acid and silk fibroin |
126
|
CA60/TA/Zn (CTZ) hydrogel |
82
|
TATA supramolecular hydrogel |
126
|
TA-reinforced CSMA/SFMA hydrogels |
83
|
QT hydrogels |
84
|
TA@bilayer hydrogel |
85
|
Cellulose |
AgNPs impregnated in cellulose |
127
|
DCF containing cellulose film |
69
|
Wood based nanofibrillar cellulose |
129
|
PHMB hydrochloride incorporated into BC/alginate |
130
|
Modified MMTs and bacterial cellulose |
131
|
Lysostaphin (Lst), a cell lytic enzyme functionalized cellulose |
87
|
Minocyclin loaded onto cellulose nanowhiskers |
133
|
BC–SSDs (supernatant of silver sulfadiazine) |
161
|
Cellulose based superabsorbent polymer formed by combining cellulose + sodium monochloroacetate (MCA) and ECH (epichlorohydrin) |
134
|
Ascorbic acid |
Nonwoven greige cotton saturated with Cu/Asc. |
135
|
Chitosan, agarose and vit C |
107
|
(P(AAm)/LAA) hydrogel-acrylamide + L-ascorbic acid |
136
|
DABC (dialdehyde bacterial cellulose) + ascorbic acid + chitosan hydrogel |
162
|
N,O-Carboxymethyl chitosan (CMCS),virgin chitosan, polyethylene oxide (PEO) vitamin C/PHT-Na (phenytoin sodium) |
140
|
PCL (polycaprolactone)/CA (chitosan ascorbate) composites |
141
|
Cellulose based film PVA (poly(vinyl alcohol)), vit C and propolis |
142
|
Aloe vera |
Sodium alginate, polyvinyl alcohol, aloe vera hydrogel |
143 and 144 |
(Periodate oxidized pectin) OP–gel–aloe–curcumin dressing |
145
|
SH (sodium hyaluronate), CS (chitosan), and DA (dopamine) in natural DES (deep eutectic solvent) + aloe vera gel to form hydrogel |
146
|
Chitosan, acetic acid, crosslinker (diacrylate (poly(ethylene glycol))), photo initiator (2-hydroxy-2-methylpropiophenone) and additive aloe vera to form chitosan based hydrogel |
147
|
Quercetin |
Quercetin–CF (chitosan–fibrin) scaffold |
91
|
Quercetin was incorporated into collagen |
151
|
PLA/GO/Q (poly lactic acid, graphene oxide, quercetin) |
92
|
Q–ALG (alginate)/CSNPs (chitosan nanosuspension) |
152
|
PCL (polycaprolactone)/GO (graphene oxide)/quercetin |
93
|
Chitosan based quercetin nanoparticles |
40
|
QuB (quercetin borate nanoparticles)–PVA (polyvinyl alcohol) xerogel film |
153
|
Qu and OA molecules in a nano hydrogel |
156
|
Quercetin loaded silver nanoparticles hydrogel matrix |
157
|
Consequently, hydrogels are crucial to providing a moist environment and in vivo therapeutic effects and the additional underlying antimicrobial activity inhibits infection and prevents the chronic phase of wound healing.163 Multifunctional hydrogels are designed based on the chemical composition of the constituent elements and their modifications, followed by the incorporation of nanocomponents or naturally occurring active agents to provide an underlying molecular mechanism that assists in accelerated wound healing. The contributing factors that influence wound healing and antimicrobial activity are the polymers and active agents incorporated. Acute and chronic wounds are at risk of microbial infections, and hydrogel-based dressings with antimicrobial activity are an approach that provides exceptional outcomes.
7. Discussion
Wound healing is a complex process that requires the synchronization of multiple cell types for distinct purposes such as homeostasis, re-epithelialization, growth, etc. Chronic wounds require attention and improper care can be treacherous.164 Faster and more efficient wound healing has been the objective and modulation is done with various natural developments that focus on the prevention of the dehydration of wounds and prevent further complications due to diseases that arise as a result of the interactions of viruses and bacteria.
An ideal wound dressing should do the following. (1) Maintain or provide moisture. (2) Allow collective epidermal cell migration. (3) Assist in angiogenesis as it is critical for tissue formation. (4). Be capable of providing oxygen and nutrition to the growing tissues during healing. (5) Allow gaseous exchange with the environment as it is vital for wound tissue hypoxia.165 (6) Prevent bacterial infection during healing. (7) Be non-adherent so that it can be removed without causing pain and prevent the removal of the stratum corneum from the newly formed epithelium. (8) Be capable of absorbing excess wound exudates to avoid leakage into surrounding healthy tissues. (9) Be non-toxic, non-allergic and non-septic.166
Presently, there are innumerable naturally occurring and synthetic materials utilized in the biomedical field but the concern of biodegradability needs to be addressed. Developing a dressing that can alter the duration and efficiency of healing will greatly benefit the patients. Hydrogels are a great alternative to synthetic wound dressings due to their high water content, porous nature, biodegradability, and adaptive nature, and the preparation method is inexpensive and eco-friendly. Hydrogels can be incorporated with naturally occurring phytocompounds that can act against infection-causing viruses or bacteria, which is a coupled approach, and these developments have been reviewed herein. Multifunctional wound dressings possess antimicrobial, anti-inflammatory, cell synthesis, modulating properties and wound healing agents that are of natural origin provide minimal side effects, accelerate epithelialization and prevent infection. This coupled approach provides tremendous progress in preventing broad-spectrum microbial infections in addition to stimulating wound healing. The self-healing ability and durability are also beneficial for elongating the lifetime of the dressings for continued wound healing. The natural agents incorporated may be the core constituents or can also be supporting constituents in the development of these dressings. Being environmentally friendly is a huge upside to the use of these substances. The procurement of materials is much easier because they are naturally available and can be regenerated within the human lifetime. This direction of research and development to incorporate natural products in wound dressings is overall beneficial to all involved, including the developers, consumers and the environment.
8. Conclusions
Despite being a potentially coupled approach, multifunctional wound dressings and hydrogels pose several challenges during wound healing. Though several approaches are being developed by incorporating natural active agents as mentioned in the review, the fabrication of smarter dressings utilising corn protein zein, shellac, keratin, etc. will be an interesting sustainable approach to developing dressings with improved mechanical properties, assisting in accelerated cell proliferation and superior tissue adhesiveness. More studies need to be carried out for the fabrication of multi-functional wound dressings that have durable antimicrobial activity coupled with drug release at the wound site. Moreover, carrying out more trials in clinical environments would be well appreciated along with the marketing of the developed approaches for advanced wound healing. In the next few years, mathematical models describing wound healing should also be developed.
Conflicts of interest
The authors declare that they have no competing interests that could influence the work done in the paper.
References
- K. Grabowski, K.-H. Baringhaus and G. Schneider, Nat. Prod. Rep., 2008, 25, 892–904, 10.1039/b715668p.
-
A. Osbourn and V. Lanzotti, Plant-derived Natural Products: Synthesis, Function and Application, Springer, 2009, DOI:10.1007/978-0-387-85498-4.
-
V. Chaitanya, Genome and Genomics: From Archaea to Eukaryotes, 2019, DOI:10.1007/978-981-15-0702-1.
- W.-S. Ryu, Mol. Virol. Hum. Pathog. Viruses, 2017, 31–45, DOI:10.1016/B978-0-12-800838-6.00003-5.
- T. J. Silhavy, D. Kahne and S. Walker, Cold Spring Harbor Perspect. Biol., 2010, 2, a000414, DOI:10.1101/cshperspect.a000414.
-
G. L. Patrick, An introduction to medicinal chemistry, Oxford University Press, 1995, DOI:10.1021/jm800676e.
- M. Tavakolizadeh, A. Pourjavadi, M. Ansari, H. Tebyanian, S. J. S. Tabaei, M. Atarod, N. Rabiee, M. Bagherzadeh and R. S. Varma, Green Chem., 2021, 23, 1312–1329, 10.1039/d0gc02719g.
- H. Liu, Z. Li, Y. Zhao, Y. Feng, A. V. Zvyagin, J. Wang, X. Yang, B. Yang and Q. Lin, ACS Appl. Mater. Interfaces, 2021, 13, 26770–26781, DOI:10.1021/acsami.1c05514.
- Y. Liang, J. He and B. Guo, ACS Nano, 2021, 15, 12687–12722, DOI:10.1021/acsnano.1c04206.
- J. Su, J. Li, J. Liang, K. Zhang and J. Li, Life, 2021, 11(10), 1016, DOI:10.3390/life11101016.
- X. Liu, H. Xu, M. Zhang and D.-G. Yu, Membranes, 2021, 11(10), 770, DOI:10.3390/membranes11100770.
- J. Xue, T. Wu, Y. Dai and Y. Xia, Chem. Rev., 2019, 119, 5298–5415, DOI:10.1021/acs.chemrev.8b00593.
- J. Moreira, A. C. Vale and N. M. Alves, Spin-coated freestanding films for biomedical applications, J. Mater. Chem. B, 2021, 9, 3778–3799, 10.1039/d1tb00233c.
- D. P. Birnie, Spin Coating Technique, 2004, 49–55, DOI:10.1007/978-0-387-88953-5_4.
- C. Gao, L. Zhang, J. Wang, M. Jin, Q. Tang, Z. Chen, Y. Cheng, R. Yang and G. Zhao, J. Mater. Chem. B, 2021, 9, 3106–3130, 10.1039/d1tb00067e.
- V. Midha and D. Arjun, J. Text. Eng. Fash. Technol., 2017, 1(4) DOI:10.15406/jteft.2017.01.00023.
- G. S. Bhat and S. R. Malkan, J. Appl. Polym. Sci., 2002, 83(3), 572–585, DOI:10.1002/app.2259.
- P. Oviroh, R. Akbarzadeh, D. Pan, R. Alfred, M. Coetzee, T.-C. Jen and R. Coetzee, Sci. Technol. Adv. Mater., 2019, 465–496, DOI:10.1080/14686996.2019.1599694.
- M. Karppinen, Beilstein J. Nanotechnol., 2014, 5, 1104–1136, DOI:10.3762/bjnano.5.123.
- W. W. McNeary, S. A. Tacey, G. D. Lahti, D. R. Conklin, K. A. Unocic, E. C. D. Tan, E. C. Wegener, T. E. Erden, S. Moulton, C. Gump, J. Burger, M. B. Griffin, C. A. Farberow, M. J. Watson, L. Tuxworth, K. M. Van Allsburg, A. A. Dameron, K. Buechler and D. R. Vardon, ACS Catal., 2021, 11, 8538–8549 CrossRef CAS.
- S. N. Nandhini, N. Sisubalan, A. Vijayan, C. Karthikeyan, M. Gnanaraj, D. A. M. Gideon, T. Jebastin, K. Varaprasad and R. Sadiku, Heliyon, 2023, 9, e13128, DOI:10.1016/j.heliyon.2023.e13128.
- Y. X. Gan, A. H. Jayatissa, Z. Yu, X. Chen and M. Li, J. Nanomater., 2020, 2020, 8917013, DOI:10.1155/2020/8917013.
- A. Tabernero, Á. González-Garcinuño, S. Cardea and E. Martín del Valle, Chem. Eng. J., 2022, 444, 136615, DOI:10.1016/j.supflu.2020.105129.
- E. Reverchon and S. Cardea, J. Membr. Sci., 2004, 240, 187–195, DOI:10.1016/j.memsci.2004.04.020.
- Y. W. Kho, D. S. Kalika and B. L. Knutson, Polymer, 2001, 42, 6119–6127, DOI:10.1016/s0032-3861(01)00067-2.
- L. Baldino, S. Cardea and E. Reverchon, J. Taiwan Inst. Chem. Eng., 2017, 71, 518–526, DOI:10.1016/j.jtice.2016.12.020.
- P. van de Witte, P. J. Dijkstra, J. W. A. van den Berg and J. Feijen, J. Membr. Sci., 1996, 117, 1–31, DOI:10.1016/0376-7388(96)00088-9.
- L. Lu, S. Yuan, J. Wang, Y. Shen, S. Deng, L. Xie and Q. Yang, Curr. Stem Cell Res. Ther., 2018, 13, 490–496, DOI:10.2174/1574888X12666170612102706.
- E. M. Ahmed, J. Adv. Res., 2015, 6, 105–121, DOI:10.1016/j.jare.2013.07.006.
-
G. Odian, Radical Chain Polymerization, in Principles of Polymerization, John Wiley & Sons, Inc., Hoboken, NJ, USA, 2004, pp. 198–349 Search PubMed.
- H. Afjoul, A. Shamloo and A. Kamali, Mater. Sci. Eng., C, 2020, 113, 110957, DOI:10.1016/j.msec.2020.110957.
- M. Alizadeh, F. Abbasi, A. B. Khoshfetrat and H. Ghaleh, Mater. Sci. Eng., C, 2013, 33, 3958–3967, DOI:10.1016/j.msec.2013.05.039.
-
M. Abdul Majed Patwary, Thin films processed by SILAR method, in Thin Films - Deposition Methods and Applications, Intech Open, 2023, DOI:10.5772/intechopen.100701.
- S. P. Ratnayake, J. Ren, J. van Embden, C. F. McConville and E. D. Gaspera, J. Mater. Chem. A, 2021, 9, 25641–25650, 10.1039/d1ta07710d.
- I. Savencu, S. Iurian, A. Porfire, C. Bogdan and I. Tomuţă, React. Funct. Polym., 2021, 168, 105059, DOI:10.1007/s11837-022-05180-9.
- S. Mitra, T. Mateti, S. Ramakrishna and A. Laha, JOM, 2022, 74, 3392–3407, DOI:10.1007/s11837-022-05180-9.
- K. Sarkar, C. Gomez, S. Zambrano, M. Ramirez, E. de Hoyos, H. Vasquez and K. Lozano, Mater. Today, 2010, 13, 12–14, DOI:10.1016/S1369-7021(10)70199-1.
- P. Calvo, C. Remuñan-López, J. L. Vila-Jato and M. J. Alonso, Pharm. Res., 1997, 14, 1431–1436, DOI:10.1023/a:1012128907225.
- N. H. Hoang, T. Le Thanh, R. Sangpueak, J. Treekoon, C. Saengchan, W. Thepbandit, N. K. Papathoti, A. Kamkaew and N. Buensanteai, Polymers, 2022, 14(4), 662, DOI:10.3390/polym14040662.
- A. Choudhary, V. Kant, B. L. Jangir and V. G. Joshi, Eur. J. Pharmacol., 2020, 880, 173172, DOI:10.1016/j.ejphar.2020.173172.
- M. P. Batista, V. S. S. Gonçalves, F. B. Gaspar, I. D. Nogueira, A. A. Matias and P. Gurikov, Int. J. Biol. Macromol., 2020, 156, 773–782, DOI:10.1016/j.ijbiomac.2020.04.089.
- Y. Yeo and K. Park, J. Controlled Release, 2004, 100, 379–388, DOI:10.1016/j.jconrel.2004.09.012.
- B. B. Aggarwal, A. Kumar and A. C. Bharti, Anticancer Res., 2003, 23, 363–398 CAS.
- D. G. Nagle, D. Ferreira and Y.-D. Zhou, Phytochemistry, 2006, 67, 1849–1855, DOI:10.1016/j.phytochem.2006.06.020.
- İ. Gülçin, Z. Huyut, M. Elmastaş and H. Y. Aboul-Enein, Arab. J. Chem., 2010, 3, 43–53, DOI:10.1016/j.arabjc.2009.12.008.
-
P. Kumar Gupta, S. Sai Raghunath, D. Venkatesh Prasanna, P. Venkat, V. Shree, C. Chithananthan and K. Geetha, An Update on Overview of Cellulose, Its Structure and Applications, Cellulose, 2019, ch. 4, IntechOpen, DOI:10.5772/intechopen.84727.
- R. M. J. Brown, J. H. Willison and C. L. Richardson, Proc. Natl. Acad. Sci. U. S. A., 1976, 73, 4565–4569, DOI:10.1073/pnas.73.12.4565.
- J. Du, J. J. Cullen and G. R. Buettner, Biochim. Biophys. Acta, 2012, 1826, 443–457, DOI:10.1016/j.bbcan.2012.06.003.
- A. M. El Sayed, S. M. Ezzat, M. M. El Naggar and S. S. El Hawary, Rev. Bras. Farmacogn., 2016, 26, 352–362, DOI:10.1016/j.bjp.2016.01.009.
- A. J. Larson, J. D. Symons and T. Jalili, Pharmaceuticals, 2010, 3, 237–250, DOI:10.3390/ph3010237.
- D. Yang, T. Wang, M. Long and P. Li, Oxid. Med. Cell. Longev., 2020, 2020, 8825387, DOI:10.1155/2020/8825387.
- D.-Y. Chen, J.-H. Shien, L. Tiley, S. Chiou, S.-Y. Wang, T.-J. Chang, Y.-J. Lee, K.-W. Chan and W.-L. Hsu, Food Chem., 2010, 119, 1346–1351, DOI:10.1016/j.foodchem.2009.09.011.
- P. Tyagi, M. Singh, H. Kumari, A. Kumari and K. Mukhopadhyay, PLoS One, 2015, 10, e0121313, DOI:10.1371/journal.pone.0121313.
- G. K. Varshney, R. K. Saini, P. K. Gupta and K. Das, Langmuir, 2013, 29, 2912–2918, DOI:10.1021/la304778d.
- J.-M. Song, K.-H. Lee and B.-L. Seong, Antiviral Res., 2005, 68, 66–74, DOI:10.1016/j.antiviral.2005.06.010.
- K. Kaihatsu, M. Yamabe and Y. Ebara, Molecules, 2018, 23(10) DOI:10.3390/molecules23102475.
- S. Y. Asadi, P. Parsaei, M. Karimi, S. Ezzati, A. Zamiri, F. Mohammadizadeh and M. Rafieian-Kopaei, Int. J. Surg., 2013, 11, 332–337, DOI:10.1016/j.ijsu.2013.02.014.
- N. Caturla, E. Vera-Samper, J. Villalaín, C. R. Mateo and V. Micol, Free Radical Biol. Med., 2003, 34, 648–662, DOI:10.1016/s0891-5849(02)01366-7.
- H. Arakawa, M. Maeda, S. Okubo and T. Shimamura, Biol. Pharm. Bull., 2004, 27, 277–281, DOI:10.1248/bpb.27.277.
- X.-F. Zhang, Y.-C. Dai, W. Zhong, M. Tan, Z.-P. Lv, Y.-C. Zhou and X. Jiang, Bioorg. Med. Chem., 2012, 20, 1616–1623, DOI:10.1016/j.bmc.2011.11.040.
- J. C. Isenburg, N. V. Karamchandani, D. T. Simionescu and N. R. Vyavahare, Biomaterials, 2006, 27, 3645–3651, DOI:10.1016/j.biomaterials.2006.02.016.
- S. Amri and M. Bensouilah, S. Afr. J. Bot., 2020, 131, 200–205, DOI:10.1016/j.sajb.2020.02.018.
- A. Pandey and P. S. Negi, Nat. Prod. Res., 2018, 32, 1189–1192, DOI:10.1080/14786419.2017.1323209.
- G.-M. Lemnaru Popa, R. D. Truşcă, C.-I. Ilie, R. E. Ţiplea, D. Ficai, O. Oprea, A. Stoica-Guzun, A. Ficai and L.-M. Diţu, Molecules, 2020, 25(18), 4069, DOI:10.3390/molecules25184069.
- A. H. Hashem, M. Hasanin, S. Kamel and S. Dacrory, Colloids Surf., B, 2022, 209, 112172, DOI:10.1016/j.colsurfb.2021.112172.
- A. Furuya, M. Uozaki, H. Yamasaki, T. Arakawa, M. Arita and A. H. Koyama, Int. J. Mol. Med., 2008, 22, 541–545, DOI:10.3892/ijmm_00000053.
- P. Ghaly, J. Iliopoulos and M. Ahmad, Br. J. Nurs., 2021, 30, S38–S42, DOI:10.12968/bjon.2021.30.5.S38.
- J. Kallio, M. Jaakkola, M. Mäki, P. Kilpeläinen and V. Virtanen, Planta Med., 2012, 78, 1824–1830, DOI:10.1055/s-0032-1315388.
- Z. Sun, C. Yu, W. Wang, G. Yu, T. Zhang, L. Zhang, J. Zhang and K. Wei, Front. Microbiol., 2018, 9, 2338, DOI:10.3389/fmicb.2018.02338.
- K. Saoo, H. Miki, M. Ohmori and W. D. Winters, Phyther. Res., 1996, 10, 348–350, DOI:10.1002/(sici)1099-1573(199606)10:4<348::aid-ptr836>3.0.co;2-2.
- M. Fani and J. Kohanteb, J. Oral Sci., 2012, 54, 15–21, DOI:10.2334/josnusd.54.15.
- R. Coopoosamy and M. Magwa, Afr. J. Biotechnol., 2006, 5(11), 1092–1094, DOI:10.4314/ajb.v5i11.42978.
- W. Wu, R. Li, X. Li, J. He, S. Jiang, S. Liu and J. Yang, Viruses, 2015, 8(1), 6, DOI:10.3390/v8010006.
- Y. Mi, L. Zhong, S. Lu, P. Hu, Y. Pan, X. Ma, B. Yan, Z. Wei and G. Yang, J. Ethnopharmacol., 2022, 290, 115066, DOI:10.3390/ijms24054607.
- S. Wang, J. Yao, B. Zhou, J. Yang, M. T. Chaudry, M. Wang, F. Xiao, Y. Li and W. Yin, J. Food Prot., 2018, 81, 68–78, DOI:10.4315/0362-028X.JFP-17-214.
- Z. Yuan, F. Yao, Z. Hu, S. Sun and B. Wu, NeuroReport, 2015, 26, 387–393, DOI:10.1097/WNR.0000000000000352.
- R. C. Rema, A. Salim, R. Babu, S. Pal, R. Biswas, B. N. Sathy, S. V. Nair and D. Menon, ACS Appl. Polym. Mater., 2022, 4, 4839–4849, DOI:10.1021/acsapm.2c00440.
- I. El-Nahhal, J. Salem, F. Kodeh, R. Anbar and A. Elmanama, J. Nanomed. Nanotechnol., 2021, 12, 568, DOI:10.1016/j.matchemphys.2022.126099.
- A. K. Kar, A. Singh, N. Dhiman, M. P. Purohit, P. Jagdale, M. Kamthan, D. Singh, M. Kumar, D. Ghosh and S. Patnaik, Int. J. Nanomed., 2019, 14, 9837–9854, DOI:10.2147/IJN.S228462.
- Q. Wei, L. Ma, W. Zhang, G. Ma and Z. Hu, J. Mater. Chem. B, 2022, 10(20), 3927–3925, 10.1039/D2TB00074A.
- M. M. Abd El-Hady, A. Farouk and S. Sharaf, Coatings, 2022, 12(2), 178, DOI:10.3390/coatings12020178.
- N. Ninan, A. Forget, V. P. Shastri, N. H. Voelcker and A. Blencowe, ACS Appl. Mater. Interfaces, 2016, 8, 28511–28521, DOI:10.1021/acsami.6b10491.
- X. He, X. Liu, J. Yang, H. Du, N. Chai, Z. Sha, M. Geng, X. Zhou and C. He, Carbohydr. Polym., 2020, 247, 116689, DOI:10.1016/j.carbpol.2020.116689.
- W. Pan, X. Qi, Y. Xiang, S. You, E. Cai, T. Gao, X. Tong, R. Hu, J. Shen and H. Deng, Int. J. Biol. Macromol., 2022, 195, 190–197, DOI:10.1016/j.ijbiomac.2021.12.007.
- Y. Li, R. Fu, C. Zhu and D. Fan, Colloids Surf., B, 2021, 205, 111869, DOI:10.1016/j.colsurfb.2021.111869.
- T. Maver, U. Maver, F. Mostegel, T. Griesser, S. Spirk, D. M. Smrke and K. Stana-Kleinschek, Cellulose, 2015, 22, 749–761, DOI:10.1007/s10570-014-0515-9.
- J. Miao, R. C. Pangule, E. E. Paskaleva, E. E. Hwang, R. S. Kane, R. J. Linhardt and J. S. Dordick, Biomaterials, 2011, 32, 9557–9567, DOI:10.1016/j.biomaterials.2011.08.080.
- J. Wu, Y. Zheng, W. Song, J. Luan, X. Wen, Z. Wu, X. Chen, Q. Wang and S. Guo, Carbohydr. Polym., 2014, 102, 762–771, DOI:10.1016/j.carbpol.2013.10.093.
- J. Lee, M. Yeo, W. Kim, Y. Koo and G. H. Kim, Int. J. Biol. Macromol., 2018, 110, 497–503, DOI:10.1016/j.ijbiomac.2017.10.105.
- R. Barbosa, A. Villarreal, C. Rodriguez, H. De Leon, R. Gilkerson and K. Lozano, Mater. Sci. Eng., C, 2021, 124, 112061, DOI:10.1016/j.msec.2021.112061.
- W. S. Vedakumari, N. Ayaz, A. S. Karthick, R. Senthil and T. P. Sastry, Eur. J. Pharm. Sci., 2017, 97, 106–112, DOI:10.1016/j.ejps.2016.11.012.
- A.-M. Croitoru, Y. Karaçelebi, E. Saatcioglu, E. Altan, S. Ulag, H. K. Aydoğan, A. Sahin, L. Motelica, O. Oprea, B.-M. Tihauan, R.-C. Popescu, D. Savu, R. Trusca, D. Ficai, O. Gunduz and A. Ficai, Pharmaceutics, 2021, 13(7), 957, DOI:10.3390/pharmaceutics13070957.
- S. Faraji, N. Nowroozi, A. Nouralishahi and J. S. Shayeh, Life Sci., 2020, 257, 118062, DOI:10.1016/j.lfs.2020.118062.
- D. Simões, S. P. Miguel, M. P. Ribeiro, P. Coutinho, A. G. Mendonça and I. J. Correia, Eur. J. Pharm. Biopharm., 2018, 127, 130–141, DOI:10.1016/j.ejpb.2018.02.022.
- N. Karsan and R. M. Zuker, Can. J. Plast. Surg., 1998, 6(3), 159–161, DOI:10.1177/229255039800600309.
- A. Paduraru, C. Ghitulica, R. Trusca, V. A. Surdu, I. A. Neacsu, A. M. Holban, A. C. Birca, F. Iordache, B. S. Vasile, F Iordache, A.C. Birca, A.M. Holban, I.A. Neacsu, V.A. Surdu, R Trusca, C Ghitulica and A Paduraru, Materials, 2019, 12(11), 1859, DOI:10.3390/ma12111859.
- I.-C. Coman, M. Al Hammoud, R. Tudosescu, R. Iancu, C. Barac and C. A. Popa, Rom. J. Ophthalmol., 2019, 63, 135–141, DOI:10.22336/rjo.2019.20.
- O. M. Al-Amer, Blood Coagulation Fibrinolysis, 2022, 33, 145–148, DOI:10.1097/MBC.0000000000001130.
- C. Stockmann, S. Kirmse, I. Helfrich, A. Weidemann, N. Takeda, A. Doedens and R. S. Johnson, J. Invest. Dermatol., 2011, 131, 797–801, DOI:10.1038/jid.2010.345.
- W. J. Kim, R. R. Mohan, R. R. Mohan and S. E. Wilson, Investig. Ophthalmol. Vis. Sci., 1999, 40, 1364–1372 CAS.
- P. Bainbridge, J. Wound Care, 2013, 22, 407–412, DOI:10.12968/jowc.2013.22.8.407.
- B. Li and J. H.-C. Wang, J. Tissue Viability, 2011, 20, 108–120, DOI:10.1016/j.jtv.2009.11.004.
- M. Piipponen, D. Li and N. X. Landén, Int. J. Mol. Sci., 2020, 21(22), 8790, DOI:10.3390/ijms21228790.
- I. Pastar, O. Stojadinovic, N. C. Yin, H. Ramirez, A. G. Nusbaum, A. Sawaya, S. B. Patel, L. Khalid, R. R. Isseroff and M. Tomic-Canic, Adv. Wound Care, 2014, 3, 445–464, DOI:10.1089/wound.2013.0473.
-
G. S. Schultz, G. A. Chin, L. Moldawer and R. F. Diegelmann, Principles of Wound Healing, in Mechanisms of Vascular Disease: A Reference Book for Vascular Specialists, ed. R. Fitridge and M. Thompson, University of Adelaide Press, Adelaide (AU), 2011 Search PubMed.
- D. Akbik, M. Ghadiri, W. Chrzanowski and R. Rohanizadeh, Life Sci., 2014, 116, 1–7, DOI:10.1016/j.lfs.2014.08.016.
- Y. Chen, L. Tian, F. Yang, W. Tong, R. Jia, Y. Zou, L. Yin, L. Li, C. He, X. Liang, G. Ye, C. Lv, X. Song and Z. Yin, Adv. Wound Care, 2019, 8, 341–354, DOI:10.1089/wound.2018.0853.
- L. Zheng, S. Li, J. Luo and X. Wang, Front. Bioeng. Biotechnol., 2020, 8, 593768, DOI:10.3389/fbioe.2020.593768.
- V. Vivcharenko, M. Wojcik, K. Palka and A. Przekora, Materials, 2021, 14(5), 1211, DOI:10.3390/ma14051211.
- W. Suvitayavat, C. Sumrongkit, S. S. Thirawarapan and N. Bunyapraphatsara, J. Ethnopharmacol., 2004, 90, 239–247, DOI:10.1016/j.jep.2003.09.044.
- E. Teplicki, Q. Ma, D. E. Castillo, M. Zarei, A. P. Hustad, J. Chen and J. Li, Wounds, 2018, 30, 263–268 Search PubMed.
- D. D. Samarasekera, Adv. Health Sci. Educ. Theory Pract., 2010, 15, 403 CrossRef PubMed.
- D. Gopinath, M. R. Ahmed, K. Gomathi, K. Chitra, P. K. Sehgal and R. Jayakumar, Biomaterials, 2004, 25, 1911–1917, DOI:10.1016/s0142-9612(03)00625-2.
- D. Li, W. Nie, L. Chen, Y. Miao, X. Zhang, F. Chen, B. Yu, R. Ao, B. Yu and C. He, RSC Adv., 2017, 7, 7973–7982, 10.1039/c6ra27319j.
- X. Li, S. Chen, B. Zhang, M. Li, K. Diao, Z. Zhang, J. Li, Y. Xu, X. Wang and H. Chen, Int. J. Pharm., 2012, 437, 110–119, DOI:10.1016/j.ijpharm.2012.08.001.
- S. S. Kamar, D. H. Abdel-Kader and L. A. Rashed, Ann. Anat., 2019, 222, 94–102, DOI:10.1016/j.aanat.2018.11.005.
- M. Alibolandi, M. Mohammadi, S. M. Taghdisi, K. Abnous and M. Ramezani, Int. J. Pharm., 2017, 532, 466–477, DOI:10.1016/j.ijpharm.2017.09.042.
- V. Yesilyurt, M. J. Webber, E. A. Appel, C. Godwin, R. Langer and D. G. Anderson, Adv. Mater., 2016, 28, 86–91, DOI:10.1002/adma.201502902.
- H.-L. Kim, J.-H. Lee, B. J. Kwon, M. H. Lee, D.-W. Han, S.-H. Hyon and J.-C. Park, Artif. Organs, 2014, 38, 411–417, DOI:10.1111/aor.12190.
- J. Zhu, R. Hou, M. Liu, L. Wang, W. Chen, Y. Sun, W. Wei and S. Ye, Mater. Today Sustain., 2022, 18, 100125, DOI:10.1016/j.mtsust.2022.100125.
- Y. C. Shin, S.-J. Song, S. W. Hong, S. J. Jeong, W. Chrzanowski, J.-C. Lee and D.-W. Han, Nanomaterials, 2017, 7(11), 369, DOI:10.3390/nano7110369.
- X. Zhao, D. Pei, Y. Yang, K. Xu, J. Yu, Y. Zhang, Q. Zhang, G. he, Y. Zhang, A. Li, Y. Cheng and X. Chen, Adv. Funct. Mater., 2021, 31(18), 2009442, DOI:10.1002/adfm.202009442.
- C. R. S. Prakoeswa, R. N. Oktaviyanti, D. M. Indramaya, E. Hendradri, S. Sawitri, L. Astari, D. Damayanti and M. Y. Listiawan, J. Dermatol. Treat., 2021, 32, 1026–1030, DOI:10.1080/09546634.2020.1729333.
- B. S. Kim, S.-H. Kim, K. Kim, Y.-H. An, K.-H. So, B.-G. Kim and N. S. Hwang, Mater. Today Bio, 2020, 8, 100079, DOI:10.1016/j.mtbio.2020.100079.
- B. Kaczmarek, O. Mazur, O. Miłek, M. Michalska-Sionkowska, A. Das, A. Jaiswal, J. Vishnu, K. Tiwari, A. Sionkowska, A. M. Osyczka and G. Manivasagam, Prog. Biomater., 2020, 9, 249–257, DOI:10.1007/s40204-020-00146-z.
- C. Chen, X. Yang, S. Li, C. Zhang, Y. Ma, Y. Ma, P. Gao, S. Gao and X. Huang, Green Chem., 2021, 23, 1794–1804, 10.1039/d0gc02909b.
- T. Maneerung, S. Tokura and R. Rujiravanit, Carbohydr. Polym., 2008, 72, 43–51, DOI:10.1016/j.carbpol.2007.07.025.
- X. Gao, Q. Dai, L. Yao, H. Dong, Q. Li and X. Cao, Biomater. Sci., 2020, 8, 2694–2701, 10.1039/d0bm00322k.
- T. Hakkarainen, R. Koivuniemi, M. Kosonen, C. Escobedo-Lucea, A. Sanz-Garcia, J. Vuola, J. Valtonen, P. Tammela, A. Mäkitie, K. Luukko, M. Yliperttula and H. Kavola, J. Controlled Release, 2016, 244, 292–301, DOI:10.1016/j.jconrel.2016.07.053.
- I. Sulaeva, H. Hettegger, A. Bergen, C. Rohrer, M. Kostic, J. Konnerth, T. Rosenau and A. Potthast, Mater. Sci. Eng., C, 2020, 110, 110619, DOI:10.1016/j.msec.2019.110619.
- M. Islam, T. Khan, W. Khattak and J. Park, Cellulose, 2013, 20(2), 589–596, DOI:10.1007/s10570-012-9849-3.
- S. K. Bajpai, V. Pathak, N. Chand and B. Soni, J. Macromol. Sci., Part A: Pure Appl.Chem., 2013, 50, 466–477 CrossRef CAS.
- S. K. Bajpai, V. Pathak and B. Soni, Int. J. Biol. Macromol., 2015, 79, 76–85, DOI:10.1016/j.ijbiomac.2015.04.060.
- A. J. Bullock, P. Pickavance, D. B. Haddow, S. Rimmer and S. MacNeil, Regen. Med., 2010, 5, 55–64, DOI:10.2217/rme.09.67.
- J. V. Edwards, N. T. Prevost, M. Santiago, T. von Hoven, B. D. Condon, H. Qureshi and D. R. Yager, Molecules, 2018, 23(9), 2399, DOI:10.3390/molecules23092399.
- A. Awadallah-F, Des. Monomers Polym., 2014, 17, 466–480, DOI:10.1080/15685551.2013.867578.
- L. Avizheh, T. Peirouvi, K. Diba and A. Fathi-Azarbayjani, Ther. Deliv., 2019, 10, 757–767, DOI:10.4155/tde-2019-0059.
- W. Li, B. Wang, M. Zhang, Z. Wu, J. Wei, Y. Jiang, N. Sheng, Q. Liang, D. Zhang and S. Chen, Cellulose, 2020, 27(5), 2637–2650, DOI:10.1007/s10570-019-02942-8.
- A. Taşdemir, R. Aydin, A. Akkaya, N. Akman, Y. Altınay, H. Çetin, B. Şahin, A. Uzun and E. Ayyıldız, Ceram. Int., 2021, 47, 19362–19373 CrossRef.
- M. A. Zarandi, P. Zahedi, I. Rezaeian, A. Salehpour, M. Gholami and B. Motealleh, IET Nanobiotechnol., 2015, 9, 191–200, DOI:10.1049/iet-nbt.2014.0030.
- R. Augustine, P. Dan, I. Schlachet, D. Rouxel, P. Menu and A. Sosnik, Int. J. Pharm., 2019, 559, 420–426, DOI:10.1016/j.ijpharm.2019.01.063.
- G. T. Voss, M. S. Gularte, A. G. Vogt, J. L. Giongo, R. A. Vaucher, J. V. Z. Echenique, M. P. Soares, C. Luchese, E. A. Wilhelm and A. R. Fajardo, Int. J. Pharm., 2018, 552, 340–351, DOI:10.1016/j.ijpharm.2018.10.009.
- K. Bialik-Wąs, M. Miastkowska, P. Sapuła, K. Pluta, D. Malina, J. Chwastowski and M. Barczewski, Pharmaceutics, 2022, 14(4), 773, DOI:10.3390/pharmaceutics14040773.
- K. Bialik-Wąs, K. Pluta, D. Malina, M. Barczewski, K. Malarz and A. Mrozek-Wilczkiewicz, Mater. Sci. Eng., C, 2021, 120, 111667 CrossRef PubMed.
- M. Tummalapalli, M. Berthet, B. Verrier, B. L. Deopura, M. S. Alam and B. Gupta, Int. J. Biol. Macromol., 2016, 82, 104–113 CrossRef CAS PubMed.
- Y. Wang, Y. Zhang, Z. Lin, T. Huang, W. Li, W. Gong, Y. Guo, J. Su, J. Wang and Q. Tu, Composites, Part B, 2021, 222, 109047 CrossRef CAS.
- I. Garcia-Orue, G. Gainza, F. B. Gutierrez, J. J. Aguirre, C. Evora, J. L. Pedraz, R. M. Hernandez, A. Delgado and M. Igartua, Int. J. Pharm., 2017, 523, 556–566 CrossRef CAS PubMed.
- S. Kudłacik-Kramarczyk, A. Drabczyk, M. Głąb, D. Alves-Lima, H. Lin, T. E. L. Douglas, S. Kuciel, A. Zagórska and B. Tyliszczak, Mater. Sci. Eng., C, 2021, 123, 111977, DOI:10.1016/j.msec.2021.111977.
- M. N. Shahzad and N. Ahmed, J. Pak. Med. Assoc., 2013, 63, 225–230 Search PubMed.
- D. Hekmatpou, F. Mehrabi, K. Rahzani and A. Aminiyan, Iran. J. Med. Sci., 2019, 44, 1–9 Search PubMed.
- K. Gomathi, D. Gopinath, M. R. Ahmed and R. Jayakumar, Biomaterials, 2003, 24, 2767–2772, DOI:10.1016/s0142-9612(03)00059-0.
- T. Nalini, S. K. Basha, A. M. Sadiq and V. S. Kumari, Polym. Bull., 2023, 80, 515–540, DOI:10.1016/j.mtcomm.2022.104172.
- X. Li, X. Yang, Z. Wang, Y. Liu, J. Guo, Y. Zhu, J. Shao, J. Li, L. Wang and K. Wang, Colloids Surf., B, 2022, 209, 112175, DOI:10.1016/j.colsurfb.2021.112175.
- V. Kant, B. L. Jangir, V. Kumar, A. Nigam and V. Sharma, Growth Factors, 2020, 38, 105–119, DOI:10.1080/08977194.2020.1822830.
- G. Ajmal, G. V. Bonde, S. Thokala, P. Mittal, G. Khan, J. Singh, V. K. Pandey and B. Mishra, Artif. Cells, Nanomed., Biotechnol., 2019, 47, 228–240, DOI:10.1080/21691401.2018.1548475.
- G. Gallelli, E. Cione, R. Serra, A. Leo, R. Citraro, P. Matricardi, C. Di Meo, F. Bisceglia, M. C. Caroleo, S. Basile and L. Gallelli, Int. Wound J., 2020, 17, 485–490, DOI:10.1089/ten.teb.2007.0332.
- R. Badhwar, B. Mangla, Y. R. Neupane, K. Khanna and H. Popli, Nanotechnology, 2021, 32(50), 505102, DOI:10.1088/1361-6528/ac2536.
- A. Agostino, A. Gatta, T. Busico, M. Rosa and C. Schiraldi, J. Biotechnol. Biomater., 2012, 4(1) DOI:10.4172/2155-952X.1000140.
- G. D. Nicodemus and S. J. Bryant, Tissue Eng., Part B, 2008, 14, 149–165, DOI:10.1089/ten.teb.2007.0332.
- N. Horzum and N. Arik, Cumhur. Sci. J., 2019, 40(1), 125–135, DOI:10.17776/csj.505746.
- X. Wen, Y. Zheng, J. Wu, L. Yue, C. Wang, J. Luan, Z. Wu and K. Wang, Prog. Nat. Sci.: Mater. Int., 2015, 25(3), 197–203, DOI:10.1016/j.pnsc.2015.05.004.
- J. Xiong, Y. Wang, Q. Xue and X. Wu, Green Chem., 2011, 13, 900–904, 10.1039/C0GC00772B.
- N. Asadi, H. Pazoki-Toroudi, A. R. Del Bakhshayesh, A. Akbarzadeh, S. Davaran and N. Annabi, Int. J. Biol. Macromol., 2021, 170, 728–750, DOI:10.1016/j.ijbiomac.2020.12.202.
- M. Rodrigues, N. Kosaric, C. A. Bonham and G. C. Gurtner, Physiol. Rev., 2019, 99, 665–706, DOI:10.1152/physrev.00067.2017.
- D. M. Castilla, Z.-J. Liu and O. C. Velazquez, Adv. Wound Care, 2012, 1, 225–230, DOI:10.1089/wound.2011.0319.
- H. Matsumura, R. Imai, N. Ahmatjan, Y. Ida, M. Gondo, D. Shibata and K. Wanatabe, Int. Wound J., 2014, 11, 50–54, DOI:10.1111/j.1742-481X.2012.01061.x.
|
This journal is © The Royal Society of Chemistry 2023 |