DOI:
10.1039/D2SU00058J
(Paper)
RSC Sustain., 2023,
1, 282-293
Kinetic and thermodynamic studies of HMF-ester synthesis using Brønsted–Lewis acidic ionic liquid catalyst†
Received
24th September 2022
, Accepted 17th December 2022
First published on 5th January 2023
Abstract
The valorization of 2nd generation biomass-derived 5-HMF into HMF-ester following the esterification reaction is a promising and appealing route for the production of renewable platform chemicals. Herein, we report the thermodynamics and kinetics of the HMF-ester products synthesized using bifunctional IL [SMIM][FeCl4] catalyst. The effects of the various reaction parameters such as reaction temperature and time were studied and optimized for maximum 5-HMF conversion into HMF-levulinate in a batch autoclave. Bifunctional IL [SMIM][FeCl4] catalyst gave 97% 5-HMF conversion with a maximum 78% yield of HMF-levulinate within 5 h reaction time at 378 K. The thermodynamic properties such as critical temperature (Tc), critical volume Vc, critical pressure (Pc), Gibbs free energy of formation (ΔG0f), enthalpy of formation (ΔH0f), and heat capacity (Cp) for the HMF-ester products (HMF-levulinate, HMF-propionate, HMF-lactate, HMF-formate, and HMF-acetate) were estimated following the group contribution methods (GCMs). To elucidate the kinetics for the synthesis of HMF-levulinate, a series of the reaction runs were performed in a batch reactor in the temperature range of 348–393 K. Based on the results obtained by the experimental study, a reaction kinetic model for this HMF-levulinate synthesis reaction was formulated and the activation energy for the first order reaction was found to be 32.78 kJ mol−1.
Sustainability spotlight
HMF-esters are derived from platform chemical HMF and are obtained by lignocellulosic biomass valorization. They find potential applications as fungicides, fuel additives, surfactants, heat-activated crosslinkers for adhesives, coatings, composites, foundry binders, foams/inks, and also for treating sickle cell anemia. The scalability of such valuable HMF esters to industry scale production from the gram-scale laboratory synthesis needs the efficient design of suitable reactors, which is only feasible after establishing the thermodynamics and reaction kinetics, which is achieved in this work. Further, this work aligns with UN SDG 3 (good health and wellbeing), SDG 8 (decent work and economic growth), SDG 9 (industry, innovation, and infrastructure), and SDG 12 (responsible consumption and production).
|
1 Introduction
Th valorization of 2nd generation lignocellulosic waste biomass materials into specialty chemicals, biofuel precursors, and fuel additives has gained extensive attention globally due to the ready availability and depletion of the fossil-based energy sources.1–3 The production of biofuel and the development of biorefineries is the best alternate tool for the transition from fossil-based resources to renewable energy sources.4 Waste biomass (potentially green and renewable) is composed of lignin, hemicellulose, and cellulose, in which cellulose and hemicellulose contain 75% carbohydrates in polymer form, including both the six- and five-membered sugars.5–7 Numerous industrially important products8 and chemicals are being produced in biorefineries from biomass such as the industrial production of ethanol, lactic acid, 5-hydroxymethylfurfural (5-HMF), levulinic acid (LA), succinic acid, 2,5-furandicarboxylic acid, and sorbitol.4,9 Different kinds of homogeneous and heterogeneous catalysts are reported for the conversion of lignocellulosic biomass into industrially important chemicals.10 However, owing to the complex structure of biomass components involving inter- and intramolecular hydrogen bonding activation, the conversion of these components into fine chemicals is still very difficult and challenging.11 Researchers have reported the treatment of waste biomass in different kinds of acid and alkaline environments such as H2SO4, NaOH, and ammonia to break this inter and intramolecular hydrogen bonding but the conversion and recovery of the catalyst is still challenging.12 In this context, ionic liquids (ILs) play an important role for biomass conversion into fine chemicals.11,13
Waste biomass-derived 5-hydroxymethylfurfural (5-HMF)14 and levulinic acid (LA) have been identified as topmost specialty energy-rich chemicals.15,16 The synthesis of these high value-added chemicals using environmentally-benign IL catalysts is reported in earlier literature.17–19 The presence of highly reactive functional groups within 5-HMF and LA make both the chemicals important intermediate precursors for a host of different chemical reactions, such as esterification, acetalization, halogenation, hydrogenation, and condensation.20–24 5-HMF valorization is one of the thrust areas because of the wide application of its products, which include25,26 resins, polymers, pharmaceuticals, antifreezing agents, fuels, and fuel additives. Among these, the upgraded HMF-ester products of 5-HMF following the esterification reaction are recognized as promising biofuel and fuel additive compounds.27,28
Esters are widely used in different industries such as food,29 pharmaceutical,27 and petroleum and petrochemicals.30,31 Esters are usually synthesized by Fischer esterification, which is a classical acid-promoted organic reaction and predominantly catalyzed by mineral acid catalysts such as H2SO4, HF, and H3PO4.32 The use of these mineral acid catalyst is advantageous due to full access to active sites and easy operation in homogeneous reaction conditions. However, mineral acid-catalyzed reactions have some disadvantages, including severe equipment corrosion, neutralization-generated gypsum waste, and problems in product purification, which has high energy consumption, high cost, and is environmentally hazardous. Hence, the synthesis of ester products using more efficient catalysts and green and sustainable process routes is highly demanding and desirable. Recently, the use of IL catalysts has increasingly become a more interesting approach33–36 due to its advantageous properties such as high thermal stability, negligible volatility, selective solubility, and adjustable structural properties.37 The reports on esterification reactions38,39 are sparse; in addition, this reaction of 5-HMF with LA requires further investigation with regards to kinetic and thermodynamic aspects especially.
The thermodynamic physical properties such as liquid vapor pressure and normal boiling point of a compound are indispensable for the development of new processes and the optimization of the existing processes.40–43 For instance, the accurate value of the liquid vapor pressure is required for many tasks in process simulation as well as for the determination of the number of theoretical stages in distillation columns and the calculation of temperature profiles. Similarly, the value of vapor pressure and normal boiling point express the stability and volatility of the fuel and have great importance for safety and environment concern42,43 and required as entry data for the calculation of density, viscosity, surface tension, thermal conductivity, enthalpy of vaporization, critical properties, and acentric factor.41,44,45 On the other hand, critical properties such as critical temperature (Tc) and critical pressure (Pc) of the pure substance (compound) are commonly used in design and operation of the cyclic system.41,46 However, the estimation of the experimental thermodynamic properties of the ester products has been a long-standing goal at the lab scale.47 However, the determination of the thermodynamic properties of the biodiesel or biodiesel/biodiesel blends is impractical and difficult.40 Therefore, thermodynamic models based on the correlations,48 group contribution techniques,49–52 and the method based on the principle of corresponding states are very helpful to estimate these properties with more accurate results.53 Among all these, the GCMs are the most successful predictive methods and can be selected as promising for the estimation of the thermodynamic properties, which were developed in 1950s.54
According to the group contribution approach, the property of a molecule is a function of the structurally-dependent parameter and can be determined by summing the frequency of each group occurring in the molecule times its contribution.46 In addition, the behavior of a substance is strongly dependent over the intermolecular forces and the types of the chemical bonds within the molecule. Following GCMs, thermodynamic properties such as Tc, Pc, normal boiling point (Tb),49,50,55–59 enthalpies of formation, entropies,60 and activity coefficients can be estimated.54 In previous studies, following this approach, Z. Kolska estimated the enthalpy and entropy of vaporization of organic compounds.51 R. Ceriani and coworkers estimated the vapor pressure and heats of vaporization of edible oil/fat compounds.61 B. Moller et al. estimated the vapor pressure of non-electrolyte organic compounds.62 Sales-Cruz and coworkers estimated Tc and Pc for fatty acid alkyl esters compounds.63 Wallek et al. recently reported the Tc, Pc, and Tb of the compounds.64 Similarly, the thermodynamic physical properties of the fatty acid esters and multifunctional organic compounds was estimated by Wang et al. and J. F. Pankow et al., respectively.65,66
On the other hand, a number of the kinetic studies have appeared on the synthesis of the esters, which help us to ascertain the boundaries of feasibility of the reaction investigations and are important to assess the activation energy of the esterification reaction.67 In the earlier reported studies, the kinetics of the esterification reactions have been reported by different research groups.68–70 However, to the best of our knowledge, the thermodynamic properties for various organic compounds and kinetics for different esterification reactions have been reported in the previous studies, whereas the available data does not cover the thermodynamics of HMF-ester products, which are derived by the reaction of 5-HMF with acid components. In addition, the kinetics for the 5-HMF esterification reaction is still missing in the literature. The esterification reaction scheme of the HMF-levulinate with the esterification reaction of 5-HMF and LA is represented in Scheme 1.
 |
| Scheme 1 Esterification reaction scheme of 5-HMF with LA. | |
The objective of the present study is to develop the reaction kinetics for the esterification of HMF into HMF-levulinate using Brønsted–Lewis (bifunctional) acidic IL [SMIM][FeCl4] catalyst. To perform the kinetic study of this reaction, the effect of the reaction kinetic parameters such as time and temperature on the 5-HMF conversion was studied. From the kinetics, the reaction rate constant, activation energy (Ea), and pre-exponential factor (A) were estimated. Similarly, HMF-formate, HMF-lactate, HMF-propionate, and HMF-acetate were synthesized with the reaction of 5-HMF with formic acid, lactic acid, propionic acid, and acetic acid. Our current study is also focussed on predicting the thermodynamic properties of HMF-ester compounds, such as critical temperature, critical pressure, and critical volume based on GCMs. This study also estimates the thermodynamic properties of HMF-ester compounds such as Gibbs free energy (ΔG0f), enthalpy (ΔH0f), and heat capacity (Cp) using the GCMs.
2. Materials and methods
2.1 Materials
HMF (97%), 1-methyl imidazole (99%), LA (99%), and propionic acid (99%) were purchased from Alfa Aesar, China. Lactic acid (LAC) (88%), formic acid (FA) (99%), and acetic acid (85%) were purchased from Fischer Scientific India. Chlorosulfonic acid (98%) was purchased from Spectrochem India Pvt. Ltd. Diethyl ether, n-hexane, anhydrous FeCl3 (97%), ethyl acetate, and 4 Å molecular sieves were purchased from Merck, India. All the other required chemicals from commercial sources used were at high purity level. The solvents were dried with 4 Å molecular sieves by gently shaking overnight prior to use. 4 Å molecular sieves (MS) were dried overnight at 408 K prior to use.
2.2 Synthesis of the Brønsted–Lewis (bifunctional) acidic IL catalysts ([SMIM][FeCl4])
The bifunctionalized IL catalyst, [SMIM][FeCl4], was synthesized as per the previously reported synthetic procedure.71 For the synthesis of the bifunctional IL catalyst [SMIM][FeCl4], first Brønsted acidic IL [SMIM][Cl] was synthesized following the earlier reported procedure. In a typical synthesis, chlorosulfonic acid (SO3HCl) was added dropwise at room temperature in a slight excess of 1-methylimidazole in dry dichloromethane (CH2Cl2) solvent. The resultant reaction mixture was stirred for 20 minutes and after the completion of the reaction, solvent was decanted. The unreacted species were removed by washing the resultant mixture with CH2Cl2, dried using a rotary evaporator, and the product was dried at 353 K. The synthesized Brønsted acidic IL [SMIM][Cl] was characterized following the 1H NMR and 13C NMR spectroscopic techniques. Finally, [SMIM][FeCl4] was synthesized by mixing equimolar amounts of anhydrous FeCl3 within [SMIM][Cl] at room temperature, followed by vigorous stirring, and characterized as reported in the literature.23
2.3 Catalytic activity for 5-HMF conversion into HMF-esters
HMF-ester products were synthesized in a batch reactor; all experiments were conducted in a 25 mL round bottom (RB) flask and heated in an oil bath at the required optimum reaction temperature. The RB flask was connected with a reflux condenser and the inert atmosphere inside the reaction was created by adjusting the nitrogen balloon at the top of the reflux condenser. The reaction was conducted by adding 1.2 mmol 5-HMF and 6 mmol acid (LA, formic acid, lactic acid, propionic acid, and acetic acid) into the flask. The resultant reaction mixture was stirred magnetically under an inert atmosphere at room temperature. Further, 150 mg bifunctional IL catalyst and 150 mg 4 Å dried molecular sieves were added into the reaction mixture, and the reaction temperature was increased to the desired value to attain a certain reaction temperature, which was measured using a thermocouple within the oil bath. After the completion of the reaction, the sample was collected and the quantitative conversion of 5-HMF was analyzed by high pressure liquid chromatography (HPLC).
2.4 HPLC analysis
HPLC was used for detecting the conversion of 5-HMF into HMF-esters, and the separation and purification of the product were done according to the same procedure as described in our previous studies.23,72 Finally, the conversion of 5-HMF was determined according to eqn (1) |  | (1) |
The yield of the product was determined according to eqn (2).
|  | (2) |
The selectivity of the product was determined according to eqn (3)
|  | (3) |
2.5 Estimation of thermodynamic property
The HMF-ester products (synthesized by the reaction of 5-HMF with different acids (formic acid, LA, propionic acid, acitic acid, and lactic acid)) are represented in Scheme 2. The HMF-ester products represented in Scheme 2 were successfully synthesized using a bifunctional IL catalyst developed in the lab and characterized previously.23 To calculate the thermodynamic properties of the HMF-ester products, different GCMs were employed. All the estimated thermodynamic properties such as critical temperature, critical pressure, and critical volume, Gibbs free energy, and enthalpy of the formations are based on the GCMs. In the GCMs, the critical properties of a compound are considered to be the sum of the contribution values of all, i.e., based on the contribution of elements or functional groups, isomers, and chemical bonds. The GCMs, namely, Constantinou and GANI, JOBACK, AMBROSE, FEDORS, LYDERSEN, and BENSON, were employed to determine the thermodynamic properties of HMF-esters.
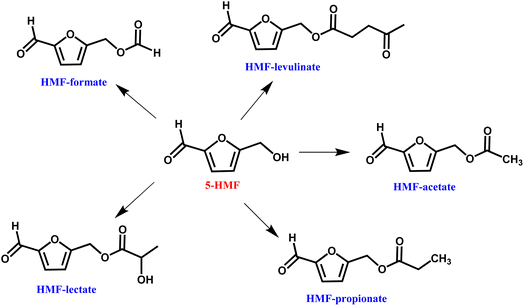 |
| Scheme 2 Synthesized HMF-ester products from 5-HMF and different acids. | |
2.6 Methods used in the estimation of the thermodynamic properties
The thermodynamic parameters such as change in Gibbs free energy (ΔG, J mol−1) and enthalpy change (ΔH, J mol−1) were estimated using ASPEN PLUS. The properties of the ester products (shown in Scheme 2) were estimated using appropriate GCMs.73 Thermodynamic physical property data Tc and (Pc) for the HMF-esters were calculated using the best GCMs, which shows somewhat more accurate results with minimum error value compared to the other GCMs. A comparison of the deviations of the calculated thermodynamic property values for 5-HMF from the experimental and estimated values between the different group contribution methods is summarized in Table S1, ESI.† It gives the number of model parameters and average percent deviations of the thermodynamic property estimation for the GCMs. When the thermodynamic critical property value was estimated using the JOBACK and GANI GCMs, the estimated error value was relatively lower with more accurate predictions as compared with LYDERSEN, BENSON, and FEDORS GCMs. Henceforth, JOBACK method was implemented to estimate (Pc) and (Tc) values for the HMF-ester compounds. On the other hand, GANI GCM used to estimate the normal boiling point (Tb), ΔH0f, and ΔG0f. The GANI method is a combination of two levels of approximation wherein the basic level only used the contribution from the first-order groups while the second level considers the effects among groups and isomers and gives more accurate results. Similarly, the value of heat capacity was calculated using JOBACK and BENSON GCMs. It is seen that the BENSON method showed somewhat more accurate predictions with relatively minimum error value compared with the JOBACK method. The equations used to estimate the thermodynamic properties of pure components are as follows.
The value of Tb, ideal gas heat capacity(Cp), and standard Gibbs free energy (ΔGf298.15 K) were calculated using the JOBACK method.
|  | (4) |
Ideal gas heat capacity:
| Cp(J mol−1 K−1) = ∑ai − 37.93+[∑bi + 0.210]T + [∑ci − 3.91 × 10−4] + [∑di + 2.06 × 10−7]T3 | (5) |
Standard Gibbs free energy:
|  | (6) |
LYDERSEN method was employed for the estimation of Tc followed by the equation as given below.
| 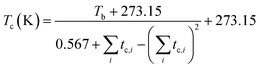 | (7) |
The Pc value of the HMF-esters was estimated, followed by the AMBROSE method, and the used equation is as given below.
| 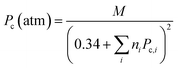 | (8) |
The Vc value of the HMF-ester compounds was calculated using the FEDORS method with the following equation.
| 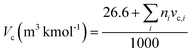 | (9) |
The BENSON method was also employed for the estimation of Gibbs free energy and enthalpy of formation.
The enthalpy of formation was calculated using the following equation.
| 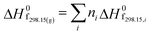 | (10) |
Gibbs free energy was calculated using the given equation.
|  | (11) |
Specific heat capacity was calculated using the following equation.
| 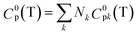 | (12) |
where
M is the molar mass,
ni is the total number of the groups of type-
i in the compound,
tc,i,
pc,i,
tb,i,
vc,i,
hf,i, and
gf,i are the contribution values for each group of type-
i for the estimation of
Tc,
Pc,
Tb,
Vc, Δ
H0f, and Δ
G0f, respectively, and N is the number of atoms in the molecule.
The GANI method was used for the calculation of the following values.
Standard value of vaporization of enthalpy and entropy:
| ΔH0f(l) = ΔH0f(g) − ΔH0v | (13) |
| S0298.15(l) = S0298.15(g) − S0v | (14) |
Calculation of Gibbs free energy and equilibrium constant:
| ΔrG0298.15 = ΔrH0298.15Δf − TΔrS0298.15 | (15) |
| 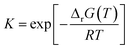 | (16) |
2.7 Kinetics of the esterification reaction of 5-HMF with LA
In the kinetic study, all the experiments for the esterification of 5-HMF with LA were conducted in a batch reactor (25 mL round bottom flask) with 5-HMF as the limiting reactant and LA as the excess reactant to get the maximum conversion of 5-HMF into the desired product in the temperature range of 348–393 K. Based on the experimental data, a reaction kinetic model was developed.
3. Results and discussion
3.1 Characterization of the synthesized Brønsted acidic IL [SMIM][Cl]
The 1H and 13C NMR data of [SMIM][Cl] are as follows: 1H NMR (400 MHz, DMSO-d6): δ (ppm) 3.85 (s, 3H), 7.67 (t, 1H), 9.06 (t, 1H), 12.20 (s, 1H), and 14.51 (s, 1H). 13C NMR: (100 MHz; D2O): δ (ppm) 36.45, 122.02, 123.4, and 137.6.
3.2 Determination of the acidity of the bifunctional [SMIM][FeCl4] IL catalyst
The acidity of the bifunctional IL [SMIM][FeCl4] catalyst was determined using an acid–base titration method according to the procedure reported earlier.36 The desired amount of the bifunctional IL was dissolved in 2-propanol and the mixture was then titrated with 0.1 M NaOH with phenolphthalein as the indicator. When the colorless solution turns pink, it was considered as the end-point of the titration. A blank titration was also performed. Finally, the acid value was calculated, which is defined as the amount of NaOH required to neutralize 1 g bifunctional IL catalyst, using following the equation.
where A is the volume of NaOH required for the titration of the sample (mL), B is the volume of NaOH required for the titration of the blank (mL), M is the molarity of the NaOH solution, and W is the sample mass (g). Finally, the acidity of the bifunctional IL, [SMIM][FeCl4] was found to be 0.92 ± 0.04 g NaOH/g IL.
3.3 Conversion of 5-HMF into HMF-levulinate and the effect of the reaction time and temperature on 5-HMF conversion
The effect of the reaction time and temperature on the 5-HMF conversion and HMF-levulinate yield are represented in Fig. 1. Using the bifunctional [SMIM][FeCl4] IL catalyst, the effect of the reaction time and temperature on the 5-HMF conversion was investigated at 348–393 K up to 6 h reaction time. The conversion of 5-HMF into HMF-levulinate significantly increases with increasing reaction time and temperature. At 348 K, the conversion of 5-HMF into HMF-levuliante significantly increases and reaches 66% within 5 h reaction time, whereas as the reaction temperature increased from 348 K to 363 K, 5-HMF conversion increased from 66% to 79% at 5 h reaction time. Similarly, at 378 K, the conversion of 5-HMF reached up to 97% within 5 h reaction time, and the maximum (78%) yield of HMF-levulinate was obtained. This result confirms that the reactivity of 5-HMF as well as the yield of HMF-levulinate increases till a reaction temperature of up to 378 K. On the contrary, as the reaction time increases at this temperature, the conversion of 5-HMF reached up to 100%, whereas the yield of HMF-levulinate decreased significantly because the longer reaction time increased the 5-HMF conversion into its byproduct humin. 98% 5-HMF conversion was obtained within 4 h as the reaction temperature was increased up to 393 K. However, the high reaction temperature had a negative effect on the HMF-levulinate yield and the product selective yield decreased and reached up to 74% at this time and temperature. At 393 K and 5 h reaction time, 100% conversion of 5-HMF was achieved, whereas no significant improvement in the HMF-levulinate yield was observed because high reaction temperature and long reaction time led to a high polymerization of 5-HMF into a black mass of humin byproduct. Finally, at 378 K and 5 h, the highest yield (78%) of HMF-levulinate and 97% 5-HMF conversion were achieved, making them the best optimized reaction conditions for 5-HMF esterification to HMF-levulinate.
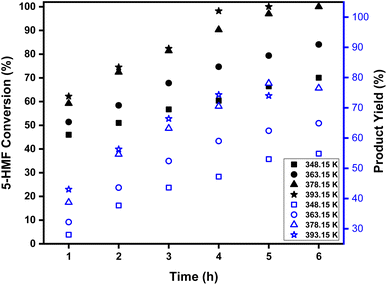 |
| Fig. 1 Conversion of 5-HMF into HMF-levulinate with time and temperature. Reaction conditions: 5 : 1 molar ratio (LA : HMF), 150 mg catalyst, molecular sieves, 300 rpm. | |
3.4 Conversion of the different acids into the HMF-ester product
The esterification reaction of 5-HMF into HMF-ester products was conducted to investigate the possibility of extending the scope of 5-HMF into its different HMF-ester products. The laboratory-synthesized bifunctional IL was tested in the 5-HMF conversion to HMF-ester products such as HMF-formate, HMF-lactate, HMF-propionate, and HMF-acetate at the established optimum reaction conditions for the HMF-levulinate synthesis, except for the HMF-formate synthesis. The conversion of 5-HMF into different HMF-ester products and the product yields are shown in Fig. 2. The proposed reaction method was successfully employed for the conversion of 5-HMF into its ester products. Using the bifunctional [SMIM][FeCl4] IL catalyst, high conversion of 5-HMF was achieved with good yield of the HMF-ester products. As the esterification reaction of 5-HMF was conducted using propionic acid and [SMIM][FeCl4] IL catalyst at 378 K and 5 h reaction time, a maximum 90% 5-HMF conversion with 77% HMF-propionate was obtained. In the case of lactic acid, 60% 5-HMF conversion with 53% HMF-lactate yield was obtained at 378 K and 5 h. On the other hand, when the esterification reaction of 5-HMF was performed with acetic acid under similar reaction conditions, 81% 5-HMF conversion with 72% HMF-acetate was obtained. In the case of formic acid, the esterification reaction of 5-HMF was performed at 363 K, and 68% yield of the HMF-formate was obtained with 80% 5-HMF conversion. The difference in the 5-HMF conversion as well as in the product yield can be attributed to the different acidic strengths of the corresponding acids, which affect the overall acidity of the reaction medium and varied the product yield and 5-HMF conversion. However, good to moderate yield of the HMF-ester products was obtained using the bifunctional [SMIM][FeCl4] IL catalyst. These results confirm that the synthesis of different HMF-ester products is feasible using this bifunctional IL catalyst.
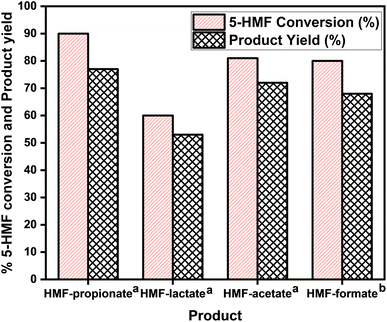 |
| Fig. 2 Synthesis of different HMF-ester products. aReaction conditions: 5 : 1 molar ratio of acid to 5-HMF, 150 mg catalyst, 150 mg molecular sieve, T = 378 K, t = 5 h, 300 rpm. bReaction conditions: 5 : 1 molar ratio of acid and 5-HMF, 150 mg catalyst, 150 mg molecular sieve, T = 363 K, t = 5 h, 300 rpm. | |
3.5 Kinetic study of HMF-levulinate synthesis
As shown in the reaction Scheme 1, the reaction kinetics of HMF-levulinate synthesis are presented in this section from the obtained experimental results with the esterification reaction of 5-HMF and LA using the bifunctional IL catalyst at different times and temperatures. Numerous reaction experiments were performed over different times and temperatures, as represented in Fig. 1, using 1
:
5 molar ratio of 5-HMF and LA in the range of 348–393 K. The rate constant was calculated according to eqn (17). The experimental data obtained from the reaction at different temperatures and times were used to derive the kinetic model for this reaction.
Here, HMF-levulinate synthesis reaction kinetics was determined following the integral method and the rate of the reaction can be written as follows.
|  | (17) |
where
k1 is the rate constant for the forward reaction,
k2 is the reaction rate constant of backward reaction, a, b, c, and d are the number of reaction order of 5-HMF, LA, HMF-levulinate, and water for forward and backward reaction, respectively (
Scheme 1). Here, the LA is present in high concentration (5-HMF
![[thin space (1/6-em)]](https://www.rsc.org/images/entities/char_2009.gif)
:
![[thin space (1/6-em)]](https://www.rsc.org/images/entities/char_2009.gif)
LA (1
![[thin space (1/6-em)]](https://www.rsc.org/images/entities/char_2009.gif)
:
![[thin space (1/6-em)]](https://www.rsc.org/images/entities/char_2009.gif)
5)) compared with 5-HMF in the reaction; therefore, the possibility of backward reaction is considered as negligible and the reaction rate of the HMF-levulinate synthesis can be written as follows.
| 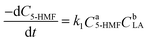 | (18) |
As the concentration of LA is in excess amount,
k1CbLA is constant and
eqn (18) can be transformed into
The esterification rate for 5-HMF with LA is defined as X, the initial concentration is C5-HMF,0, and the concentraton of 5-HMF in the esterification reaction system at any time is C5-HMF; then, C5-HMF = C5-HMF,0(1−X).
|  | (20) |
Then,
| 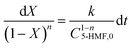 | (21) |
After integration on both sides, eqn (21) can be written as follows.
| 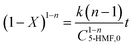 | (22) |
Finally, at different reaction temperatures, a graph between the 5-HMF concentration and reaction were obtained (Fig. 3 (a–c)) using eqn (22). The value of ln(l − X) is linear with t at n = 1, whereas at n ≠ 1, (1 − X)(l−n) and time have a linear relationship. The Arrhenius plot for the HMF-levulinate synthesis is shown in Fig. 4(a–c), and the value of the correlation coefficient can be estimated for different values of n. Accordingly, the variation in the reaction rate with temperature may be expressed by the Arrhenius equation.
| 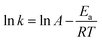 | (23) |
where
T indicates the reaction temperature and
R is the gas constant. Thus, the value of
Ea (activation energy) and
k0 (pre-exponential factor) can be derived from the Arrhenius (ln
k vs. 1/
T) plot. The calculated
Ea for the different reaction orders are shown in
Table 1. The activation energy was in the range of 21.10–41.80 kJ mol
−1 For the first order reaction, the activation energy was 32.78 kJ mol
−1. These results unambiguously provide strong evidence that the used bifunctional catalyst for the HMF-levulinate synthesis is an efficient and active catalyst.
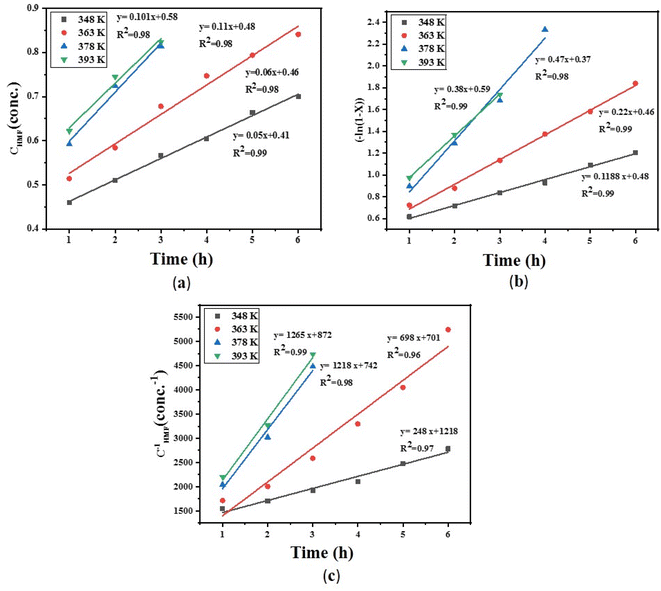 |
| Fig. 3 (a) Conversion of 5-HMF at different reaction times for (a) zero order, (b) first order, (c) second order reaction. | |
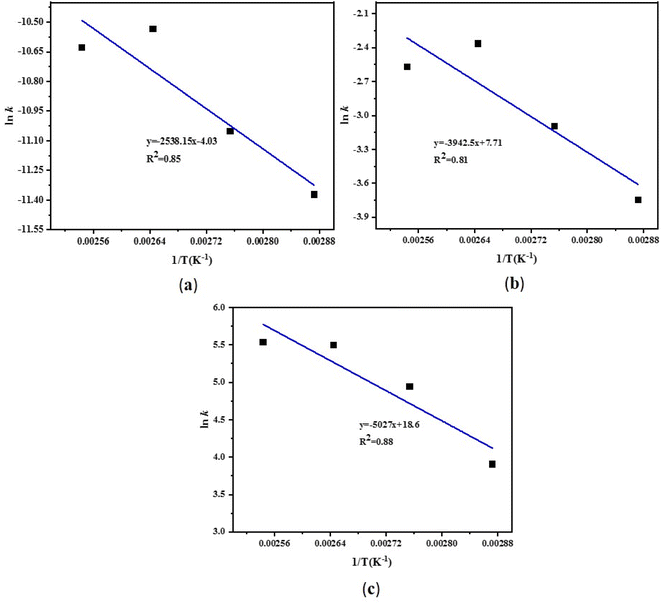 |
| Fig. 4 Arrhenius plot for the esterification of 5-HMF with LA (a) zero order, (b) first order, and (c) second order reaction. | |
Table 1 Reaction order, pre-exponential, and activation energy for the esterification reaction
Reaction order |
Kinetics |
Activation energy(kJ mol−1) |
Pre-exponential factor ((min)−1(mol L−1)1−n) |
R
2
|
0 order reaction |
C
G0 − CG = kt |
21.10 |
56.50 |
0.85 |
1st order reaction |
−ln(1 − X) = kt |
32.78 |
22.4 × 102 |
0.82 |
2nd order reaction |
|
41.80 |
11.50 × 107 |
0.88 |
3.6 Comparison of the kinetics of the previously reported esterification reactions
The comparison of the previously reported kinetics studies for the different reactants (acid and alcohols) is summarized in Table 2. In the earlier studies, several kinetic models are reported for the esterification reaction with the consideration of different types of assumptions. In 1994, H. J. Bart et al. reported the esterification reaction of LA with n-butanol using H2SO4 mineral acid catalyst, and the activation energy for this study was 54.27 kJ mol−1.74 In 1991, S. Goto and coworkers reported the esterification reaction of isobutyl alcohol with palmitic acid using sulfuric acid catalyst and reported 58.0 kJ mol−1 activation energy.75 R. Aafaqi et al. studied the esterification reaction of palmitic acid with isopropanol using p-TSA. This study reported 40.77 kJ mol−1 activation energy for this reaction.76 W. Shi and coworkers reported the esterification reaction of palmitic acid and isobutyl alcohol using a novel composite catalytic membrane (CCM) as a heterogeneous catalyst, which was prepared from sulfonated polyethersulfone (SPES) and polyethersulfone (PES) blend supported by non-woven fabrics. This study reported 35.97 kJ mol−1 activation energy for this reported system.77 S. H. Ali et al. reported the esterification (benzyl alcohol and acetic acid) and hydrolysis (benzyl acetate) process using Dowex 50 Wx8-catalyst with 54.5–57 kJ mol−1 activation energy.69 In 2019, L. J. Wei et al. reported the kinetics and esterification of acrylic acid and ethanol over the solid acid catalyst Amberlyst 35 and reported an activation energy of 59.60 kJ mol−1.68 In 2020, S. Chaemchuen et al. reported the esterification of oleic acid into methyl oleate using UiO-66 MOF catalyst with an activation energy of 54.9 ± 1.8 kJ mol−1.70 Novozyme 435 catalyzed enzymatic esterification of 5-HMF was reported by Y. Z. Qin group and lipase-catalyzed transesterification of 5-HMF esters was reported by M. Krystof and coworkers; however, their kinetic study has not been reported.27,78 Among the reported kinetics for similar esterification reactions, the activation energies ranged from 35 to 60 kJ mol−1 as shown in Table 2. In this study, bifunctional IL catalyst successfully catalyzed the esterification reaction and the activation energy for this reaction was found in the range of 32.78 kJ mol−1 for the first order reaction. Finally, the activation energy is lower than the earlier reported studies compared in Table 2. The obtained results show beneficial results for the esterification reaction of 5-HMF with LA.
Table 2 Comparison of the esterification reactions with the present studya
Reactants |
Catalysts |
Activation energy (kJ mol−1) |
References |
NR = Not reported.
|
n-Butanol and LA |
Sulfuric acid |
54.27 |
74
|
Isobutyl alcohol and palmitic acid |
Sulfuric acid |
58.0 |
75
|
Palmitic acid and isopropanol |
p-TSA |
40.77 |
76
|
Palmitic acid and isobutyl alcohol |
Composite catalytic membrane (CCM) |
35.97 |
77
|
Benzyl alcohol and acetic acid |
Dowex 50 Wx8-catalyst |
54.5–57 |
69
|
Ethanol and acrylic acid |
Amberlyst 35 |
59.60 |
68
|
Methanol and oleic acid |
UiO-66 MOF |
54.9 ± 1.8 |
70
|
5-HMF and LA |
Novozym 435 |
NR |
27
|
5-HMF and LA |
[SMIM][FeCl4] |
32.78 |
This study |
3.7 Estimation of the thermodynamic parameters for HMF-ester products
The value of the critical properties such as Tc, Pc, and Vc for the HMF-ester compounds (HMF-levulinate, HMF-acetate, HMF-propionate, HMF-lactate, and HMF-formate) were estimated, followed by using the most appropriate GCMs, which showed the lowest error values. The JOBACK method was employed to estimate the value of critical properties such as Tc and Pc for the HMF-ester compounds, and their estimated results are shown in Table 3. Similarly, the LYDERSEN method was employed to estimate the Vc value. The esters derived from 5-HMF have different structures, which have different number of atoms such as carbon and oxygen. For the estimated values of Tc and Pc from the JOBACK method, the differences are more noticeable for HMF-levulinate and HMF-lactate, likely due to the different number of the groups (such as –CH2, O–H, ring group, non-ring group, and –O–), which significantly affect the contributions of these groups. The results obtained from this study confirm that the thermodynamic property values of the molecules is strong dependent on the actual structure of the molecule and the specific contribution of the particular group. The GANI method was employed to estimate the value of ΔG0f(298.15) and (ΔfH0298.15(g)) for HMF-esters. The negative value of (ΔfH0298.15(g)) and ΔG0f(298.15) for (HMF-levulinate, HMF-acetate, HMF-propionate, HMF-lactate, and HMF-formate) compounds indicates that the product formation from each reaction step can proceed spontaneously. In this comparison, similarly, enthalpy and Gibbs free energy of formation of HMF-ester compounds at 298 K were also calculated using the GANI method, and the values are shown in Table 3.
Table 3 Estimated thermodynamic properties of the HMF-ester compounds using group contribution methods
Methods |
Properties |
HMF-levulinate |
HMF-lectate |
HMF-propionate |
HMF-Acetate |
HMF-formate |
JOBACK |
T
c(K) |
777 |
799 |
745 |
745 |
744 |
P
c(bar) |
28.11 |
33.1 |
32.4 |
30.6 |
43.2 |
GANI |
T
b(K) |
593.15 |
601.05 |
546.10 |
539.24 |
630.62 |
ΔH0f(J mol−1) |
−681 × 103 |
−664 × 103 |
−541 × 103 |
−471 × 103 |
−459 × 103 |
ΔG0f(J mol−1) |
−446 × 103 |
−459 × 103 |
−459 × 103 |
−341 × 103 |
−332 × 103 |
LYDERSEN |
V
c(m3 kmol−1) |
0.627 |
0.568 |
0.512 |
0.423 |
0.401 |
Following the JOBACK and BENSON GCMs, the estimated value for the idea gas heat capacity for 5-HMF in the temperature range from 273 K to 1273 K is shown in Table 4 and compared in Tables S2 and S3 (ESI).† The estimated physical properties were compared and the percentage error was calculated. Finally, the compared results confirm that the BENSON method fitted well with the experimental findings with minimum percentage error and gave more accurate results, whereas the JOBACK method shows relatively low accuracy. Table 4 shows the predicted values of ideal gas heat capacities in the temperature range from 273 K to 1273 K for HMF-ester products using the BENSON method.
Table 4 Estimated value of ideal gas heat capacity Cp(J mol−1 K−1) for HMF-ester products at constant pressure using the BENSON method
Ester products |
373 (K) |
473 (K) |
573 (K) |
673 (K) |
773 (K) |
873 (K) |
973 (K) |
1073 (K) |
1173 (K) |
1273 (K) |
HMF-levulinate |
299.8 |
348.4 |
390.6 |
428.8 |
457.0 |
481.7 |
500.8 |
514.7 |
523.4 |
527.3 |
HMF-formate |
187.0 |
216.9 |
243.4 |
266.4 |
285.5 |
300.5 |
311.3 |
317.7 |
319.4 |
326.2 |
HMF-lactate |
262.3 |
304.1 |
341.1 |
373.2 |
400.3 |
422.3 |
439.1 |
450.7 |
456.8 |
457.6 |
HMF-propionate |
243.8 |
284.4 |
319.7 |
350.0 |
375.4 |
395.8 |
411.5 |
422.6 |
426.2 |
431.4 |
HMF-acetate |
215.0 |
250.2 |
281.4 |
308.0 |
330.3 |
348.0 |
365.3 |
369.2 |
372.4 |
379.0 |
4. Conclusion
In this study, the laboratory synthesis of the bifunctional IL catalyst [SMIM][FeCl4] was done and characterized using 1H and 13C NMR spectroscopic techniques. The catalytic activity of the laboratory-synthesized bifunctional IL catalyst [SMIM][FeCl4] was investigated for the esterification of 5-HMF with LA to HMF-levulinate. The effect of the reaction time and temperature on the 5-HMF conversion to HMF-levulinate was investigated. Further, the kinetic modelling of this esterification reaction was carried out. To determine the reaction kinetic parameters, the effects of the reaction conditions such as reaction time and temperature on 5-HMF conversion were investigated. A maximum (97%) 5-HMF conversion and 78% yield of HMF-levulinate were achieved at 378.15 K in 5 h. The kinetics of the HMF-levulinate synthesis were investigated in a batch reactor. The activation energy Ea for this reaction was 32.78 kJ mol−1 for first order kinetics. The thermodynamic properties such as Tc, Vc, Pc, ΔG0f, ΔfH0, and Cp for the HMF-ester products (HMF-acetate, HMF-formate, HMF-levulinate, HMF-propionate, and HMF-lactate), which are not reported in the literature so far, were estimated using the GCMs. Overall, these new insights into the reaction thermodynamics and kinetics of the esterification of 5-HMF with biomass-derived acids will be extremely useful for the development of a green process route for the valorization of waste biomass into potentially useful ester products.
Conflicts of interest
There are no conflicts to declare.
Acknowledgements
Komal Kumar thanks University Grants Commission (UGC), India, for a research fellowship. Dr Sreedevi Upadhyayula is thankful to Ministry of Human Resource Development (MHRD), Government of India for financial grant no. SPARC/P1318. The authors are also thankful to NRF (Nanoscale Research Facility) for providing High pressure liquid chromatography (HPLC) for the detection of 5-HMF conversion at IIT Delhi. One of the authors Dr Anthony Halog gratefully acknowledges funding support from the University of Queensland, Australia under the UQ Global Strategy and Partnerships Seed Funding Scheme: Round Two 2022.
References
- V. S. Sikarwar, M. Zhao, P. Clough, J. Yao, X. Zhong, M. Z. Memon, N. Shah, E. J. Anthony and P. S. Fennell, Energy Environ. Sci., 2016, 9, 2939–2977 RSC
.
- X. Zhao, H. Zhou, V. S. Sikarwar, M. Zhao, A. H. A. Park, P. S. Fennell, L. Shen and L. S. Fan, Energy Environ. Sci., 2017, 10, 1885–1910 RSC
.
- A. Saravanan, S. Jeevanantham, P. Senthil Kumar, S. Varjani, P. R. Yaashikaa and S. Karishma, Ind. Crops Prod., 2020, 153, 112613 CrossRef CAS
.
- S. Takkellapati, T. Li and M. A. Gonzalez, Clean Technol. Environ. Policy, 2018, 20, 1615–1630 CrossRef CAS PubMed
.
- M. J. Climent, A. Corma and S. Iborra, Green Chem., 2014, 16, 516–547 RSC
.
- S. Jing, X. Cao, L. Zhong, X. Peng, R. Sun and J. Liu, Ind. Crops Prod., 2018, 126, 151–157 CrossRef CAS
.
- E. C. Nnadozie and P. A. Ajibade, Chem. Data Collect., 2021, 33, 100673 CrossRef CAS
.
- S. Pathak, A. K. Ray, H. Großmann and R. Kleinert, Radiat. Phys. Chem., 2015, 117, 59–63 CrossRef CAS
.
- F. M. Fadul, Acta Innov., 2019, 47–56 Search PubMed
.
- M. Stöcker, Angew. Chem., Int. Ed., 2008, 47, 9200–9211 CrossRef PubMed
.
- S. Kang, J. Fu and G. Zhang, Renewable Sustainable Energy Rev., 2018, 94, 340–362 CrossRef CAS
.
- A. M. Da Costa Lopes, R. M. G. Lins, R. A. Rebelo and R. M. Łukasik, Green Chem., 2018, 20, 4043–4057 RSC
.
- L. Zhou, R. Liang, Z. Ma, T. Wu and Y. Wu, Bioresour. Technol., 2013, 129, 450–455 CrossRef CAS PubMed
.
- A. M. Borrero-López, E. Masson, A. Celzard and V. Fierro, Ind. Crops Prod., 2018, 124, 919–930 CrossRef
.
- I. Delidovich, K. Leonhard and R. Palkovits, Energy Environ. Sci., 2014, 7, 2803–2830 RSC
.
- K. Kumar, F. Parveen, T. Patra and S. Upadhyayula, New J. Chem., 2018, 42, 228–236 RSC
.
- M. E. Zakrzewska, E. Bogel-Lukasik and R. Bogel-Lukasik, Chem. Rev., 2011, 42, 397–417 CrossRef PubMed
.
- A. A. Rosatella, S. P. Simeonov, R. F. M. Frade and C. A. M. Afonso, Green Chem., 2011, 13, 754–793 RSC
.
- Y. S. Qu, Y. L. Song, C. P. Huang, J. Zhang and B. H. Chen, Ind. Eng. Chem. Res., 2012, 51, 13008–13013 CrossRef CAS
.
- D. J. Braden, C. A. Henao, J. Heltzel, C. C. Maravelias and J. A. Dumesic, Green Chem., 2011, 13, 1755–1765 RSC
.
- F. A. Kucherov, L. V. Romashov, K. I. Galkin and V. P. Ananikov, ACS Sustainable Chem. Eng., 2018, 6, 8064–8092 CrossRef CAS
.
- M. Li, G. Li, N. Li, A. Wang, W. Dong, X. Wang and Y. Cong, Chem. Commun., 2014, 50, 1414–1416 RSC
.
- K. Kumar, A. Dahiya, T. Patra and S. Upadhyayula, ChemistrySelect, 2018, 3, 6242–6248 CrossRef CAS
.
- K. Kumar, S. Pathak and S. Upadhyayula, J. Cleaner Prod., 2020, 256, 120292 CrossRef CAS
.
- Z. Zhang, J. Zhen, B. Liu, K. Lv and K. Deng, Green Chem., 2015, 17, 1308–1317 RSC
.
- Y. Zhu, X. Kong, H. Zheng, G. Ding, Y. Zhu and Y. W. Li, Catal. Sci. Technol., 2015, 5, 4208–4217 RSC
.
- Y. Z. Qin, M. H. Zong, W. Y. Lou and N. Li, ACS Sustainable Chem. Eng., 2016, 4, 4050–4054 CrossRef CAS
.
- M. Krystof, M. Pérez-Sánchez and P. Domínguez De María, ChemSusChem, 2013, 6, 630–634 CrossRef CAS PubMed
.
- V. K. Mishra, F. Temelli and B. Ooraikul, J. Food Eng., 1994, 23, 467–480 CrossRef
.
- N. Bureau, J. Jose, I. Mokbel and J. C. De Hemptinne, J. Chem. Thermodyn., 2001, 33, 1485–1498 CrossRef CAS
.
- M. Moosavi, Fluid Phase Equilib., 2012, 316, 122–131 CrossRef CAS
.
- X. Han and L. Zhou, Chem. Eng. J., 2011, 172, 459–466 CrossRef CAS
.
- D. Zhao, M. Liu, J. Zhang, J. Li and P. Ren, Chem. Eng. J., 2013, 221, 99–104 CrossRef CAS
.
- T. Joseph, S. Sahoo and S. B. Halligudi, J. Mol. Catal. A: Chem., 2005, 234, 107–110 CrossRef CAS
.
- J. Gui, X. Cong, D. Liu, X. Zhang, Z. Hu and Z. Sun, Catal. Commun., 2004, 5, 473–477 CrossRef CAS
.
- J. Keogh, M. S. Tiwari and H. Manyar, Ind. Eng. Chem. Res., 2019, 58, 17235–17243 CrossRef CAS
.
- J. P. Hallett and T. Welton, Chem. Rev., 2011, 111, 3508–3576 CrossRef CAS PubMed
.
- D. Yujaroen, M. Goto, M. Sasaki and A. Shotipruk, Fuel, 2009, 88, 2011–2016 CrossRef CAS
.
- W. Sakdasri, R. Sawangkeaw and S. Ngamprasertsith, Asia-Pac. J. Chem. Eng., 2016, 11, 539–548 CrossRef CAS
.
- N. S. Evangelista, F. R. Do Carmo and H. B. De Sant'Ana, Ind. Eng. Chem. Res., 2017, 56, 2298–2309 CrossRef CAS
.
- N. S. Evangelista, F. R. Do Carmo and H. B. De Sant'Ana, Ind. Eng. Chem. Res., 2018, 57, 8552–8565 CrossRef CAS
.
- S. V. D. Freitas, M. B. Oliveira, Á. S. Lima and J. A. P. Coutinho, Energy Fuels, 2012, 26, 3048–3053 CrossRef CAS
.
- W. Cordes and J. Rarey, Fluid Phase Equilib., 2002, 201, 409–433 CrossRef CAS
.
- P. Saxena, J. Patel and M. H. Joshipura, Fuel, 2016, 182, 842–849 CrossRef CAS
.
- O. C. Díaz, F. Schoeggl, H. W. Yarranton, M. A. Satyro, T. M. Lovestead and T. J. Bruno, Int. J. Chem., Mol., Nucl., Mater. Metall. Eng., 2012, 6, 460–470 Search PubMed
.
- W. Su, L. Zhao and S. Deng, Renewable Sustainable Energy Rev., 2017, 79, 984–1001 CrossRef
.
- D. H. Zaitsau, A. A. Pimerzin and S. P. Verevkin, J. Chem. Thermodyn., 2019, 132, 322–340 CrossRef CAS
.
- W. Yuan, A. C. Hansen and Q. Zhang, Fuel, 2005, 84, 943–950 CrossRef CAS
.
- L. Constantinou and R. Gani, AIChE J., 1994, 40, 1697–1710 CrossRef CAS
.
- J. Marrero and R. Gani, Fluid Phase Equilib., 2001, 183–184, 183–208 CrossRef CAS
.
- Z. Kolská, V. Růžička and R. Gani, Ind. Eng. Chem. Res., 2005, 44, 8436–8454 CrossRef
.
- A. M. Benkouider, R. Kessas, S. Guella, A. Yahiaoui and F. Bagui, J. Mol. Liq., 2014, 194, 48–56 CrossRef CAS
.
- I. Cachadiña and A. Mulero, Fluid Phase Equilib., 2009, 287, 33–38 CrossRef
.
- W. L. Kubic, R. W. Jenkins, C. M. Moore, T. A. Semelsberger and A. D. Sutton, Ind. Eng. Chem. Res., 2017, 56, 12236–12245 CrossRef CAS
.
- H. Grensemann and J. rgen Gmehling, Ind. Eng. Chem. Res., 2005, 44, 1610–1624 CrossRef CAS
.
- K. G. Joback and R. C. Reid, Chem. Eng. Commun., 1987, 57, 233–243 CrossRef CAS
.
- J. Marrero-Morejón and E. Pardillo-Fontdevila, AIChE J., 1999, 45, 615–621 CrossRef
.
- X. Wen and Y. Qiang, Ind. Eng. Chem. Res., 2001, 40, 6245–6250 CrossRef CAS
.
- J. Li, L. Xia and S. Xiang, Fluid Phase Equilib., 2016, 417, 1–6 CrossRef CAS
.
- Y. Yoneda, Bull. Chem. Soc. Jpn., 1979, 52, 1297–1314 CrossRef CAS
.
- R. Ceriani, R. Gani and Y. A. Liu, Fluid Phase Equilib., 2013, 337, 53–59 CrossRef CAS
.
- B. Moller, J. Rarey and D. Ramjugernath, J. Mol. Liq., 2008, 143, 52–63 CrossRef CAS
.
-
M. Sales-Cruz, G. Aca-Aca, O. Sánchez-Daza and T. López-Arenas, 20th Eur. Symp. Comput. Aided Process Eng. ESCAPE20, 2010, pp. 1–6 Search PubMed
.
- T. Wallek, K. Knöbelreiter and J. Rarey, Ind. Eng. Chem. Res., 2018, 57, 3382–3396 CrossRef CAS
.
- T. Y. Wang, X. Z. Meng, M. Jia and X. C. Song, Fuel Process. Technol., 2015, 131, 223–229 CrossRef CAS
.
- J. F. Pankow and W. E. Asher, Atmos. Chem. Phys., 2008, 8, 2773–2796 CrossRef CAS
.
- M. M. Zainol, N. A. S. Amin and M. Asmadi, Renewable Energy, 2019, 130, 547–557 CrossRef CAS
.
- J. W. Lin, A. H. Zaki, H. T. Wu, H. mu Lin and M. J. Lee, J. Taiwan Inst. Chem. Eng., 2019, 102, 44–50 CrossRef CAS
.
- S. H. Ali and S. Q. Merchant, Ind. Eng. Chem. Res., 2009, 48, 2519–2532 CrossRef CAS
.
- S. Chaemchuen, P. M. Heynderickx and F. Verpoort, Chem. Eng. J., 2020, 394, 124816 CrossRef CAS
.
- N. A. S. Ramli and N. A. S. Amin, J. Mol. Catal. A: Chem., 2015, 407, 113–121 CrossRef CAS
.
- K. Kumar, V. Khatri, F. Parveen, H. K. Kashyap and S. Upadhyayula, Sustainable Energy Fuels, 2020, 4, 2924–2936 RSC
.
- F. R. Carmo, N. S. Evangelista, F. A. N. Fernandes and H. B. D. S. Ana, J. Chem. Eng. Data, 2015, 60, 3358–3381 CrossRef
.
- H. J. Bart, J. Reidetschläger, K. Schatka and A. Lehmann, Int. J. Chem. Kinet., 1994, 26, 1013–1021 CrossRef CAS
.
- S. Goto, T. Tagawa and A. Yusoff, Int. J. Chem. Kinet., 1991, 23, 17–26 CrossRef CAS
.
- R. Aafaqi, A. R. Mohamed and S. Bhatia, J. Chem. Technol. Biotechnol., 2004, 79, 1127–1134 CrossRef CAS
.
- W. Shi, B. He, Y. Cao, J. Li, F. Yan, Z. Cui, Z. Zou, S. Guo and X. Qian, Bioresour. Technol., 2013, 129, 100–107 CrossRef CAS PubMed
.
- M. Krystof, M. Pérez-Sánchez and P. Domínguez De María, ChemSusChem, 2013, 6, 630–634 CrossRef CAS PubMed
.
|
This journal is © The Royal Society of Chemistry 2023 |