DOI:
10.1039/D3SM01112G
(Paper)
Soft Matter, 2023,
19, 8945-8953
The pH responsiveness of fluorescein loaded in polysaccharide composite films†
Received
24th August 2023
, Accepted 22nd October 2023
First published on 23rd October 2023
Abstract
Stimuli-responsive materials have been used in biomedical applications. Composite films fabricated using polyion complexes comprising anionic and cationic polysaccharides exhibited loading and release abilities for water-soluble molecules, the release ability of which depended on the solution pH. However, the interactions between polysaccharides and loaded molecules in the film have not been evaluated. In this study, polysaccharide composite films loaded with fluorescein (FL) as a probe molecule were fabricated and the film properties, FL ionization, and release behaviour of FL were investigated. FL loading did not significantly affect the mechanical and morphological properties of the films. The release behaviour of FL was determined by the pH of the solution as well as the electrostatic interaction between polysaccharides and FL ionic structures in FL-loaded films. Furthermore, the ionic structure change of FL that remained in the film was suppressed due to interactions with polysaccharides, such as through hydrogen bonding. Additionally, the pH responsiveness of FL in the film in the dried state was evaluated. The result shows that polysaccharide composite films were swollen because of air moisture and that the diffusion of molecules inside the film accelerated. These findings are useful to understand the properties of the loaded molecules such as ionic state and diffusiveness in the films made of polyion complexes.
1. Introduction
The development of stimuli-responsive materials, which exhibit changes in their properties and structures due to external stimuli such as temperature, light, magnetizing, and chemical environments (solution pH), has resulted in more advanced materials that perform the required functions on demand.1–3 In recent years, stimuli-responsive materials have been used for biomedical applications.4–7 In particular, temperature- and solution-pH-responsive materials are beneficial owing to their ease of control.6,7
The use of natural polymers for medical materials can not only reduce adverse effects to the environment, but also provide advantages such as biocompatibility and biodegradability. In particular, natural polysaccharides abound on Earth, and they have been utilized as feedstock for lumber, paper, clothes, etc. Recently, the fabrication of biomaterials composed of polysaccharides for applications such as drug carriers and tissue engineering has received attention.8–11 Among polysaccharides, water-soluble polysaccharides are easier to manage and mold compared with water-insoluble polysaccharides. Chitosan (CHI), hyaluronic acid (HYA), chondroitin sulfate (CS) C sodium salts, and sodium alginate (ALG) are representative water-soluble polysaccharides used for such purposes.12–14 These polysaccharides possess cationic or anionic groups in the sugar unit under an appropriate pH environment, and these groups can form electrostatic interactions with inorganic salts, proteins, and oppositely charged polysaccharides.15–18 The electrostatic interactions depend on the pH of the solution; therefore, these polysaccharides can be used as pH-responsive materials.7 However, materials composed of water-soluble polysaccharides cannot provide sufficient mechanical strength and durability under conditions used in biomedical applications.19,20 One method to insolubilize water-soluble polysaccharides is via the formation of polyion complexes (PICs). CHI, which contains positive charges in acidic solutions, can form water-insoluble PICs with anionic polysaccharides such as HYA, CS, and ALG. One advantage of PIC formation is that no cross-linking agent or chemical modification is required to produce water-insoluble gels.21–24 Furthermore, PICs can be molded into various shapes without losing the natural properties and functions of the polysaccharide.23 In addition, the desired swelling ratio and elastic properties of PICs can be achieved by adjusting the component type, component mixing ratio, and solution pH. Accordingly, PIC formation using water-soluble polysaccharides can provide structural materials with the desired properties.
Biocompatible polymer films are two-dimensional structural materials used in biomaterials such as cell scaffolds, wound dressings, and drug carriers.8,10,25 Solution casting and layer-by-layer (LbL) assembly have been used to fabricate these films.26–31 The use of water-soluble polysaccharides as raw materials has been reported.32–36 However, controlling the film thickness and density using the conventional casting method is difficult. Additionally, LbL films are only several tens of nanometers thick; therefore, they are not free-standing films. Furthermore, increasing the loading amount into LbL films is difficult because of their small thickness. Thus, the application of biocompatible polymer films to medical materials presents several challenges.
Hence, we developed polysaccharide composite films using PICs comprising CS and CHI by hot press techniques.37–42 The resulting films (CS/CHI films) are insoluble in aqueous solutions but are swollen. The swelling properties are determined by the polysaccharide species and solution pH. Furthermore, the CS/CHI films exhibit loading and release abilities for water-soluble molecules such as methylene blue (MB).39 Because the films are formed by non-covalent assembly of ionic polysaccharides, the loaded molecules are surrounded by polysaccharide chains depending on the molecular species, indicating that various species of molecules can be loaded into the films. The loaded molecules should interact with CS and CHI not only through van der Waals interactions and hydrogen bonding, but also by electrostatic interactions. Furthermore, since CS and CHI contain carboxylic acid and amino groups, respectively, and the ionization ratio of these groups changes under a certain pH environment, the CS/CHI films can be utilized as pH-responsive drug carriers. Actually, the loading and release properties are correlated with the swelling behaviour of the film, which depends on the solution pH. Here, although the loading and release behaviours were evaluated in detail, the interactions between loaded molecules and polysaccharides have not been well investigated. Understanding this point will provide useful insights into the potential of polysaccharide PIC films as a carrier for various molecules, including drugs.
In this study, to evaluate the interaction between loaded molecules and the polysaccharides of CS/CHI films, fluorescein (FL)-loaded polysaccharide composite films (CS/CHI-FL films) were fabricated, and the ionization and release behaviour of the FL in the CS/CHI-FL films were investigated. The ionization of FL, in other words, the type and number of cationic and anionic groups in FL, depends on the pH of the solution. Therefore, the absorption spectrum, fluorescence spectrum, and solubility of FL change depending on the pH of the solution. Thus, electrostatic interactions with polysaccharides can be confirmed using FL as a probe molecule and by observing the colour and fluorescence of the film. Specifically, the FL release behaviour in solutions with different pH values was evaluated, and spectroscopic properties of FL molecules remained in the film after release experiments were examined. Furthermore, gas and droplets of HCl and NH3 were reacted with the FL in the films to elucidate the difference in reactivity of FL in the solution state.
2. Experimental
2.1. Materials
Chitosan (CHI, from crab shell, molecular weight (MW) ≥ 100
000), chondroitin sulfate C (CS, sodium salt, from shark cartilage, MWca. 20
000) (Fig. 1a), acetic acid, HCl (35–37 wt% aqueous solution), NH3 (28 wt% aqueous solution), NaOH, NaH2PO4, Na2HPO4, and fluorescein (FL) were purchased from Nacalai Tesque Inc. All the reagents were used without further purification. Distilled water and ultrapure water (18.2 MΩ cm) prepared by Advantec RFD210TA and Advantec RFD414BA, respectively, were used in the experiments.
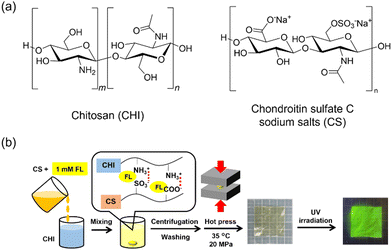 |
| Fig. 1 (a) Chemical structures and abbreviations of the polysaccharides; (b) schematic illustration of fabrication of CS/CHI-FL films. | |
2.2. Preparation of FL-loaded polysaccharide composite films
The CS/CHI-FL film was prepared by hot press techniques used in our previous study, with slight modifications (Fig. 1b).39 First, a mixture comprising aqueous solutions CS (2.0 wt% as sodium salts) and FL (1.0 mM) were added dropwise to an aqueous acetic acid (1.0 wt%) solution of CHI (2.0 wt%) until the formation of FL-loaded gel-like polyion complexes (PICs) was completed. The PIC gels obtained were washed with distilled water and separated by centrifugation (6000 rpm, 10 min).
The obtained PIC gel was sandwiched between polytetrafluoroethylene sheets and polyethylene terephthalate sheets (thickness: 100 μm) and placed on hot-press apparatus (H300-15, AS ONE Corp.) preheated at 35 °C. The gel was hot pressed at 20 MPa for 5 min. The pressed PIC gel was folded into pieces measuring 1 × 1 cm after each pressing process. The film thicknesses were controlled to 100 μm using a polyethylene terephthalate sheet spacer with square holes (5 cm × 5 cm). The resulting films were cooled to room temperature and cut into the desired sizes using a cutter knife. The obtained FL-loaded films were denoted as CS/CHI-FL films. In some cases, CS/CHI films without FL were prepared and used as controls.
2.3. Characterization of FL-loaded films
Fourier transform infrared (FT-IR) measurements were performed using a Nicolet 380 (Thermo Fisher Scientific Inc.). FT-IR spectra were recorded using a single-reflection attenuation total-reflection (ATR) method with a Quest ATR accessory (GS-10800, Specac Ltd). The absorbance and fluorescence spectra of the FL solutions and FL-loaded films were recorded using a spectrophotometer (V-660, JASCO Corp.) and a fluorescence spectrophotometer (FP-8500, JASCO Corp.), respectively. An ultraviolet (UV) lamp with a wavelength of 365 nm (UV handy lamp, AS ONE) was used for the macroscopic observation of fluorescence from FL-loaded films. The morphology of the films was evaluated using scanning electron microscopy (SEM, JSM-7001F; JEOL Ltd) with an acceleration voltage of 10 kV. In some cases, the specimens were coated with Pt–Pd using an ion-sputtering device (MC1000; Hitachi Ltd) to prevent charge-ups.
2.4. Film swelling behaviour
The swelling behaviour of the films in buffers was investigated using previously reported procedures.38,42 The films (1 cm × 1 cm) were immersed in phosphate buffer (PB) solutions (pH 2.0, 3.0, 5.8, 7.4, and 8.0) at 25 °C. At specified time intervals, the film was removed from the solutions, and the net weight of the swollen film sample was recorded after excess surface water was blotted. The degree of swelling (%) and weight loss (%) were calculated using eqn (1) and (2), respectively. | Degree of swelling (%) = (WS − WF)/W1 × 100 | (1) |
| Weight loss (%) = (W1 − WF)/W1 × 100, | (2) |
where W1 is the initial weight of the film (1 cm × 1 cm) before immersion, WS is the weight of the swollen film after immersion (180 min), and WF is the final weight of the film after the immersion experiments followed by air drying.
2.5. Tensile strength measurements
The mechanical properties of the films were evaluated quantitatively as the tensile strength using a universal tester (Autograph AGS-500NJ; Shimadzu Corp.). First, the film thickness was measured using a micrometre (MDE-25MJ, Mitutoyo Corp.). Subsequently, the films were cut into strips (1 cm × 3 cm) and placed in the apparatus while maintaining an initial gauge length of 2 cm. The stretching speed was set at 1 mm min−1. The obtained stress–strain curves were analysed using the Trapezium X software (Shimadzu Corp.). The maximum tensile strength was expressed as the mean ± standard deviation.
2.6. Evaluation of FL loading and release abilities of the films
The FL loading of the CS/CHI-FL films was evaluated using an ultra violet-visible (UV-vis) spectrophotometer. The FL solutions before treatment and the supernatants of the mixtures after PIC formation were examined. Aliquots of the sample solutions were diluted 10 or 100 times, and then the spectra were recorded. The absorbance at the maximum absorption of FL (491 nm) was used to calculate the FL amounts. The differences in the absorbance of the solutions were used in the calculations. The details of the calculation method is provided in the ESI.†
The release behaviour of FL from the films was examined by UV-vis spectroscopy. CS/CHI-FL films (2 cm × 2 cm) were immersed in 20 mL buffer solutions: 0.1 M phosphate buffer (PB) (pH 5.8, 7.4, and 8.0) and buffer prepared using 0.2 M Na2HPO4, 5 M HCl, and 1 M NaOH (pH 2.0, 3.0). Subsequently, the samples were incubated at 36.5 °C using a water bath under gentle stirring. At predetermined time intervals, 1 mL of the release medium was withdrawn and replaced with an equivalent volume of fresh buffer to maintain a constant volume. The UV absorption of the released molecules was measured in sampled solutions of pH 2.0, 3.0, 5.8, 7.4, and 8.0 using UV-vis spectroscopy with absorption maxima at 438, 479, and 491 nm, respectively. The amount of molecules in the solution was determined using a calibration curve of FL in the buffer solution. The accumulative release of FL was obtained using eqn (3).
|  | (3) |
where
VE and
V0 (mL) are the sampling volume and initial volume of the release media, respectively;
Ci and
Cn are the FL concentrations (mol L
−1);
i and
n are the sampling times; and
nA ascertains the amount of FL incorporated in the film samples (mol).
In addition, the UV-vis and fluorescence spectra of the films after these examinations were recorded to evaluate the ionic state of FL remaining in the films.
2.8. Evaluation of pH responsiveness of FL-loaded films in the dried state
The CS/CHI-FL films (2 cm × 2 cm) were placed in a desiccator with 20 mL of 36 wt% HCl or 28 wt% NH3 aqueous solution to fill the desiccator with each gas. The films were exposed to each environment for 60 min. The UV-vis spectra and fluorescence intensity of the films were recorded at various time intervals. Photographs were obtained under natural and UV light.
3. Results and discussion
3.1. Preparation and physical characterization of FL-loaded CS/CHI films
To investigate the pH responsiveness of fluorescein (FL) in an aqueous solution, measurements of UV-vis and fluorescence spectra of FL in phosphate buffer (PB) of pH 2.0, 3.0, 5.8, 7.4, and 8.0 were performed. When the pH was 2.0 or 3.0, an absorption peak appeared at 430 nm, and the absorption decreased as the pH increased (Fig. S1a, ESI†). At pH values of 5.8, 7.4, and 8.0, absorption peaks at 450 and 485 nm were observed. Furthermore, an isosbestic point did not appear in these spectra, indicating that FL underwent at least two structural changes between pH 2.0 and 8.0. The pKa values of FL are known to be 2.24, 4.20, and 6.39, and FL can form cationic, neutral, monoanionic, and dianionic structures (Fig. 2a).43 The molar ratio of FL can be calculated using the Henderson–Hasselbalch equation (Fig. S2a, ESI†). The calculated ratio of each structure in aqueous solution suggests that the structures of FL in aqueous solution are at least two species between pH 0 and 8.0. The fluorescence spectra of FL excited at 487 nm showed that the intensity increased with the solution pH (Fig. S2b and S4, ESI†). This indicates that the fluorescence intensity of FL can be used to detect the ionization of FL in polysaccharide composite films.
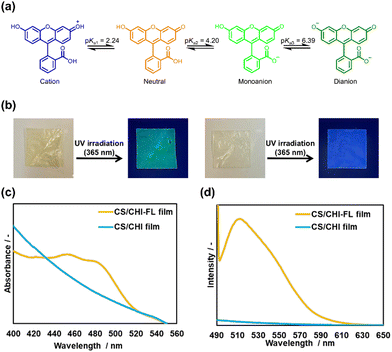 |
| Fig. 2 (a) Structural changes of FL and their pKa values. (b) Macroscopic images of the CS/CHI-FL film (left) and CS/CHI film (right) under natural and UV light; (c) UV-vis spectra of CS/CHI-FL and CS/CHI films; (d) fluorescence spectra of CS/CHI-FL and CS/CHI films. | |
Subsequently, the FL-loaded chondroitin sulfate C (CS)/chitosan (CHI) composite films (CS/CHI-FL films) were prepared using hot press techniques. The techniques give uniform and dense polysaccharide composite films.37,38 To obtain the CS/CHI-FL films, FL-containing PIC gels were prepared. The solubility of FL in water was higher in the basic solution than in the acidic solution. Therefore, FL was dissolved in a small amount of NaOH, and the solution was mixed with the CS aqueous solution before it was mixed with the acetic acid solution of CHI. Subsequently, it was mixed with CHI solution to obtain a yellow gel. Next, the films were prepared at a hot press temperature of 35 °C, which is different from the standard temperature of 120 °C, to prevent thermal deactivation in FL. The control CS/CHI films were colourless and transparent compared with those prepared at 120 °C (Fig. S4, ESI†). The thicknesses of the CS/CHI-FL film and CS/CHI film prepared at 35 °C were 0.062 ± 0.001 mm and 0.059 ± 0.001 mm, respectively. Meanwhile, the film thicknesses of CS/CHI films provided at 120 °C was 0.106 ± 0.002, which was smaller than those films prepared at 35 °C and approximately equal to the thickness of a polyethylene terephthalate sheet spacer. The reason why the thickness of the film was reduced by hot pressing at 35 °C could be swelling during film production. When the film was prepared at 120 °C, almost all the water in the gel evaporated, resulting in a dried film. Therefore, the obtained films had almost the same thickness as the spacer. In the case of the film prepared at 35 °C, a certain amount of water remains in the gel, and the swollen films having the thickness of the spacer were obtained. When the films were dried, the films lost moisture and shrank, resulting in a smaller film thickness. Notably, these films were obtained with a uniform and dense structure. The CS/CHI-FL films appeared pale yellow under natural light and emitted green fluorescence upon UV irradiation (Fig. 2b, left), whereas no green fluorescence was observed on the CS/CHI films (Fig. 2b, right). In the fluorescence and UV-vis spectra of the FL-loaded films (Fig. 2c and d), the presence of FL-derived absorption peaks (455 and 483 nm) and a luminescence peak (515 nm) was confirmed, indicating that FL was loaded into the film. The peaks at 455 and 483 nm were attributed to neutral and monoanionic FL, respectively. Furthermore, the pH value of the supernatant during the PIC gel formation was 4.2, indicating that 50% of FL formed neutral charges and the other 50% formed monoanions based on the ionization degree of FL (Fig. S2a, ESI†). Therefore, the loaded FL was composed of neutral charges and monoanions. The loading amount of FL was determined by subtracting the amount of FL in the supernatant during gel formation from that in the initial FL solution. The loading ratio was calculated by dividing the moles of FL in the film by the moles of initially added FL. The loading amount of FL was 9.8 × 10−7 mol (18%), which was lower than the 49% loading ratio of MB.39 Since the structure of the loaded FL was mainly neutral and monoanionic, which resulted in fewer electrostatic interaction points compared with MB, it was less likely to be incorporated into the PIC gel.
FT-IR measurements and tensile tests were conducted to clarify the effects of FL loading on the composite films. Fig. S5 (ESI†) shows the FT-IR spectra of the FL powder, CS/CHI film, and CS/CHI-FL film. The peak around 1530 cm−1 supports the formation of PICs from NH3+. A peak assignable to SO3− (∼1220 cm−1) was observed for CS. Meanwhile, a shift in these peaks was not observed in the CS/CHI-FL film, indicating that FL did not affect the interaction between CS and CHI. Results of tensile tests showed that the maximum stress of the CS/CHI and CS/CHI-FL films were 79.4 ± 8.3 and 74.9 ± 15.3 MPa, respectively (Fig. S6, ESI†). This indicates that the mechanical properties of the films were not altered significantly by the incorporation of FL. Accordingly, the properties of the FL-loaded films did not differ significantly from those of the CS/CHI films, and the films maintained sufficient mechanical strength with the incorporation of FL.
3.2. FL release behaviours of FL-loaded CS/CHI films under different pH conditions
Although we focused on FL in the CS/CHI-FL films, the parts of FL molecules were actually released from the film when incubating in aqueous solutions. Therefore, we first evaluated the FL release behaviours, and then evaluated the properties of FL that remained in the films. Fig. 3a and b show the time course of FL release and the swelling ratio of buffer solutions with different pH values. The order of the release ratio at 3 h was as follows: pH 7.4 > pH 8.0 > pH 2.0 > pH 5.8 > pH 3.0 (Fig. 3c). These tendencies were attributed to the swelling ratio of the film and the solubility of the FL molecules. Similarly, the swelling ratios of the CS/CHI films at 3 h were higher at pH 2.0, 7.4, and 8.0, than at pH 3.0 and 5.8 (Fig. 3b). The pKa of the carboxy groups of CS was 3.8,44 and the pKa of the ammonium groups of CHI was 6.5,45 which suggests that approximately 97% of the carboxy groups in CS were deionized at pH 2.0, and 89% of the amino groups in CHI were deionized at pH 7.4 (Fig. S2b, ESI†). Furthermore, almost all amino groups in CHI were deionized at pH 8.0. Therefore, electrostatic interactions between CS and CHI decreased at pH 2.0, 7.4, and 8.0, compared with those at pH 5.8. Consequently, the repulsion of the same charges relaxed the electrostatic interactions, which resulted in a faster release of the FL molecules. In particular, the most prominent swelling behaviour was indicated at pH 2.0 under these conditions. However, the FL release ratio at pH 2.0 was lower than that at pH 7.4 and 8.0. This can be explained by the solubility of FL molecules. A comparison of the solubility of FL at pH 2.0, 7.4, and 8.0 showed that PBS at pH 7.4 and 8.0 indicated much higher solubility than PBS at pH 2.0 (Fig. S7, ESI†). At pH 7.4 and 8.0, almost all FL molecules exhibited a dianionic structure. By contrast, the FL structure at pH 2.0 contained 50% cations and 50% neutral charges. The solubility of FL was the minimum at pH 3.4, i.e., 1.50 × 10−4 M, but was higher at pH below and above 3.4.46 This is consistent with the pH at the maximum abundance ratio of FL in the neutral state shown in Fig. S3a (ESI†). Therefore, deionized FL (neutral) is less soluble in aqueous solutions compared with ionized FL (cationic, monoanionic, and dianionic structures). At pH 2.0, the FL release ratio was low and increased gradually. The swelling ratios were comparable between pH 3.0 and pH 5.8. However, the release ratio at pH 3.0 was lower than that at pH 5.8. These results can be similarly explained by the solubility of FL. When the pH of the aqueous solution was 5.8, almost all the FL formed a monoanionic structure. When the pH was 3.0, 80% of FL was neutral, which implies low solubility. Accordingly, when the pH of the solution was 2.0, 7.4, and 8.0, where the degree of swelling was high, the release ratio of FL was higher than at pH 3.0 and 5.8, where the degree of swelling was low. Furthermore, at pH 2.0, the solubility of FL was low, and therefore the release ratio of FL decreased, while the solubility of FL was high and thus the release ratio of FL increased at pH 5.8.
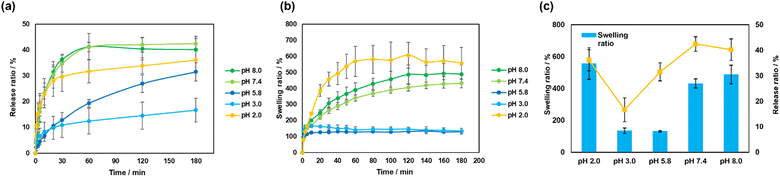 |
| Fig. 3 (a) Release behaviours of FL in different pH buffers; (b) swelling behaviours of CS/CHI films in different pH buffers; (c) correlation between release ratio of FL and swelling ratio of CS/CHI films in different pH buffers. | |
Furthermore, the effect of ionic concentrations of PB on the pH responsiveness of CS/CHI films was also considered. In general, the pH responsiveness of film-like materials composed of polyions depended on the ionic strength of the buffer solution.41,47–49 The CS/CHI films in the high ionic strength buffer such as phosphate buffer saline (PBS, pH 7.4, I = 0.16) reduced electrostatic interactions between polysaccharides.41 To clarify the effect of the ionic strength of PB, the calculation of the ionic strength of PB was performed and the order was as follows; pH 8.0 (I = 0.027) > 7.4 (I = 0.022) > 5.8 (I = 0.011) > 3.0 (I = 0.010) > 2.0 (I = 0.007). The tendency was different from release and swelling behaviour, suggesting that the values of ionic strength were too small to affect the pH responsiveness of the films. Thus, it was confirmed that the release and swelling behaviours were not correlated with the ionic strength of PB. Accordingly, the release ratio of FL depended not only on the interaction among polysaccharides and the swelling behaviour, but also on solubility changes due to FL ionization.
The morphologies of the FL-released films were observed by SEM. Fig. 4 shows the cross-section of the film before and after FL was released under each pH condition. The initial state of the film was dense and smooth. At pH 5.8, the film structure was mostly dense, with few pores. The smooth structure of the cross-section at pH 5.8 also indicated that the electrostatic interactions between polysaccharides were mostly maintained. At pH 2.0 and pH 8.0, many cracks and pores were observed, suggesting that electrostatic interactions between CS and CHI decreased. These results correspond to the swelling behaviour under different pH conditions.
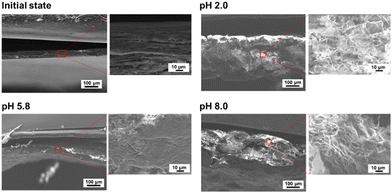 |
| Fig. 4 SEM images of cross-sections of FL-released films. | |
3.3. pH responsiveness of FL remaining in CS/CHI films after release experiments
After releasing FL for 180 min in buffers of different pH, the FL-released films emitted different colours, as shown in Fig. 5. The proportions of FL molecules remaining in the films at pH 2.0, 3.0, 5.8, 7.4, and 8.0 were approximately 64 ± 4%, 83± 5%, 69 ± 4%, 58 ± 3%, and 60 ± 4%, respectively. Considering the pH dependency of the UV-vis and fluorescence spectra of FL solutions, the fluorescence of the FL-released films was expected to be blue at pH 2.0, weakly green at pH 3.0, 5.8, and strongly green at pH 7.4 and 8.0. However, the actual films emitted blue fluorescence at pH 2.0, 3.0, 5.8, and 7.4, and green fluorescence at pH 8.0. Thus, the interactions of FL with polysaccharides, such as those via hydrogen bonding and electrostatic interactions, might have caused the pKa of FL to fluctuate.
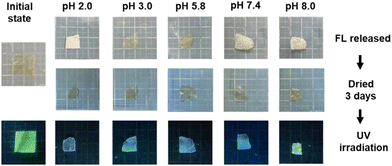 |
| Fig. 5 Macroscopic images of FL-released films after release experiments and then dried for 3 days. | |
The fluorescence spectra of the FL-released films were recorded to evaluate the structure of the remaining FL. At pH 2.0, a much weaker peak at 512 nm appeared in the fluorescence spectrum at an excitation wavelength of 487 nm (Fig. S8a, ESI†). The fluorescence spectrum of FL at an excitation wavelength of 445 nm showed a peak at 497 nm (Fig. S8b, ESI†). The absorption and peaks in each spectrum of the cationic structure with a water/ethanol solution were reported to be 436 and 480 nm, respectively.46 Thus, the main structure of the remaining FL after the FL release experiments at pH 2.0 was the cationic structure. When the pH was 3.0, a weak peak at 503 nm appeared in the fluorescence spectrum at an excitation wavelength of 445 nm (Fig. S8, ESI†). The zwitter ion-type neutral structure indicated peaks at 480 nm with a water/ethanol solution,46 indicating that the FL-released FL films at pH 3.0 contained neutral FL as zwitter ions (Fig. S8c, ESI†). Therefore, the release at pH 3.0 resulted in the formation of a zwitter ion-type neutral structure of FL in the films. At pH 5.8 and 7.4, a peak at 512 nm originating from monoanions appeared in the spectra (Fig. S8, ESI†). Therefore, monoanionic FL remained in the films after the FL-release experiments at pH 5.8 and pH 7.4. At pH 8.0, a peak was observed at 512 nm (Fig. S8, ESI†), and the intensity at pH 8.0 was higher than that at pH 5.8 and pH 7.4. The peak intensity of the dianionic structure has been reported to be higher than that of monoanionic FL.46 Thus, the FL-released films at pH 8.0 contained dianionic FL. Based on the discussion above, presumable FL structures in the CS/CHI-FL films before and after the release experiments are shown in Fig. 6(a)–(e). The ionized FL interacted with the polysaccharides in the films via electrostatic interactions and hydrogen bonding. A comparison of these results with the ratio of FL ions in an aqueous solution obtained from the Henderson–Hasselbalch equation (Fig. S2a, ESI†) indicates that the ionization of FL at pH 2.0, 3.0, 5.8, and 8.0 present the same trend. Meanwhile, the Henderson–Hasselbalch equation shows that the ratio of the monoanionic structure to the dianionic structure is 1
:
4 in a pH 7.4 aqueous solution. This is different from the result where most of the FL structures in the film are monoanionic. Deprotonation of the hydroxy group in FL is necessary to form a dianionic structure from a monoanionic structure. The hydrogen bonding between the hydroxyl groups of polysaccharides and FL might inhibit the deprotonation of FL (Fig. 6d). Accordingly, the loaded molecules in the polysaccharide composite films are ionized in the same manner as in a solution, and ionization by changing the solution pH may be difficult when the molecule interacts with polysaccharides, such as through hydrogen bonding.
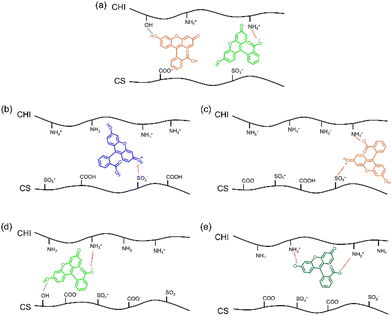 |
| Fig. 6 Schematic illustration of (a) CS/CHI-FL film and FL-released films in PB at pH (b) 2.0, (c) 3.0, (d) 5.8 and 7.4, and (e) pH 8.0. | |
3.4. Responsiveness of FL in CS/CHI-FL films to HCl and NH3 gases and droplets
The aforementioned results can be regarded as representative of the pH responsiveness of wet-state FL in the film. For comparison, the pH responsiveness of dry-state FL in the film should be evaluated. The dried films show a dense structure, and therefore the diffusion of molecules within the film is restricted. It means that the FL of the film inner is unlikely to undergo structural changes by acids or bases. In this study, the FL-loaded films were exposed to HCl and NH3 gases for 1 hour. Fig. S9 (ESI†) shows the changes in the UV-vis spectrum and colour of the film under natural light. When the film was exposed to HCl gas, the absorption signal at 487 nm in the UV-vis spectrum disappeared, whereas a new signal appeared at approximately 445 nm. The colour of the film transformed from yellow to pale green due to fluorescence. When the film was exposed to NH3 gas, the absorption signal at 445 nm in the UV-vis spectrum disappeared, whereas a new signal appeared at approximately 500 nm. The colour of the film changed from pale green to dark yellow. Accordingly, the FL in the films can react with the gas phase of the acid and base.
Subsequently, changes in the fluorescence intensity of the dried films upon exposure to HCl and NH3 were observed. Fig. 7a shows the fluorescence intensity and the colour change of the film under UV light at approximately 20% relative humidity (RH). When the film was exposed to HCl gas, the fluorescence intensity at 512 nm in the fluorescence spectrum decreased after 10 min. The fluorescence of the film under UV irradiation changed from green to blue. When the film was exposed to NH3 gas, the fluorescence intensity at 523 nm in the fluorescence spectrum increased gradually, reached a maximum value in 10 min, and then gradually decreased. The fluorescence of the film changed from blue to green. These colour changes were due to the structural change of FL in the films (Fig. S1b, ESI†). When the film was exposed to HCl gas, the FL exhibited a cationic structure. Meanwhile, the FL exhibited a dianionic structure when the film was exposed to NH3 gas. The gradual colour changes suggest that the entanglement of polysaccharides decelerated the diffusion of gases into the film. Furthermore, the change in fluorescence intensity in a high humidity environment (RH 70%), which corresponded to the swelling of the films, was confirmed (Fig. 7b). The FL in the films ionized completely in approximately 1 min, and the ionization was faster than that at RH 20%. This indicates that the polysaccharide in the film loosened in the high-humidity environment, and the gases were dispersed more rapidly. Accordingly, the CS/CHI-FL films swelled with moisture in the air, and the diffusion of gas molecules within the films changed.
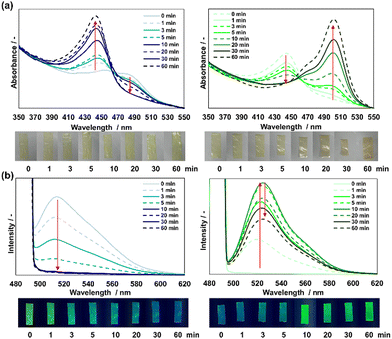 |
| Fig. 7 Fluorescence spectra and colour change of the CS/CHI-FL films under UV light at low (left) and high (right) relative humidities (RHs). (a) Exposed to HCl gas; (b) exposed to NH3 gas. | |
A comparison of the ionization of FL in the CS/CHI-FL films after exposure to gases and immersion in PB was conducted. The wavelength originating from FL in the CS/CHI-FL films after gas exposure was the same as that of FL in the FL-released films (Fig. 7 and Fig. S9, ESI†). Wang et al. reported the ionization of FL in agarose-based films via HCl and NH3 gases and reported no peak shift between the films and in solutions.50 Thus, the interaction between FL and polysaccharides was the same in the solutions and gas phases. By contrast, the polysaccharide network in the CS/CHI-FL films was loosened in PB. Consequently, the internal molecules diffused easily, thus facilitating the ionization of FL in the film. Owing to gas exposure at low humidity, molecular diffusion was low and FL ionization was slower than that in PB. Furthermore, high humidity caused the CS/CHI films to swell sufficiently for molecular diffusion; therefore, the ionization of FL was much faster than that in low-humidity conditions. This suggests that the weakened electrostatic interaction between CS and CHI by HCl and NH3 was due to the diffusion of molecules in the films. Accordingly, the intermolecular interactions between FL and the polysaccharides in the composite films did not change, regardless of whether swelling occurred in the film. By contrast, film swelling proceeded via the diffusion of molecules and facile FL ionization. The fast ionization might be due to the weakened electrostatic interaction between CS and CHI by HCl and NH3, which is advantageous to the development of pH-responsive materials.
Finally, the FL-loaded films were characterized after they were exposed to HCl and NH3 gases. The FT-IR spectra of the films exposed to each gas are shown in Fig. S10 (ESI†). When the film was exposed to HCl gas, the peak corresponding to COO− (ca. 1580 cm−1) disappeared, whereas the peak corresponding to COOH (ca. 1710 cm−1) appeared, indicating that the carboxy groups of CS in the films were protonated. In the case of exposure to NH3 gas, the peak corresponding to NH3+ (ca. 1530 cm−1) descended slightly, which suggests the deprotonation of amino groups of CHI in the film. The tensile strength of the films exposed to HCl and NH3 gas decreased from 74.9 ± 15.3 MPa to 58.48 ± 17.4 and 62.56 ± 6.63 MPa, respectively (Fig. S11, ESI†). Thus, exposure to each gas suggests relaxation in the electrostatic interactions between the polysaccharides.
4. Conclusions
CS/CHI-FL films were successfully prepared using FL-containing PIC gels composed of oppositely charged polysaccharides. The loading of FL did not significantly affect the mechanical and morphological properties of the films. The release ratio of FL was lower than 50% for all the pH values investigated, and FL remained in the films. The release behaviour of FL was determined by the solution pH, interaction among polysaccharides, swelling behaviour, and solubility changes due to FL ionization. The loaded FL molecules in the polysaccharide composite films were ionized similarly as in solution, and the ionization of the remaining FL was difficult when the molecules interacted with polysaccharides, such as through hydrogen bonding. As for the pH-responsiveness of FL in the film in the dried state, the CS/CHI-FL films were swollen with moisture in the air, which facilitated the diffusion of molecules and FL ionization. As mentioned above, the electrostatic interaction between polysaccharides in the CS/CHI films changed depending on the solution pH, swelling behaviour, which affected the release ratio of the loaded molecule. Furthermore, the CS/CHI films were swollen by air moisture, and the diffusion of molecules inside the film accelerated. These properties indicate that polysaccharide-derived PIC films, such as CS/CHI films, can be used as pH-responsive materials not only in the biomedical field but also in other applications. The results of this study provide a design criterion for loading ionizable molecules with the desired ionized structures in PIC-based films under specific pH conditions.
Author contributions
Konatsu Takagi: investigation, methodology, visualization, and writing – original draft. Takuya Sagawa: conceptualization, supervision, data curation, and writing – review & editing. Mineo Hashizume: project administration, conceptualization, supervision, and writing – review & editing.
Conflicts of interest
There are no conflicts to declare.
Acknowledgements
This work was partially supported by a Grant-in-Aid for Scientific Research (C) (no. 19K05588). The authors also thank Editage (www.editage.com) for English language editing.
References
- M. A. C. Stuart, W. T. S. Huck, J. Genzer, M. Müller, C. Ober, M. Stamm, G. B. Sukhorukov, I. Szleifer, V. V. Tsukruk, M. Urban, F. Winnik, S. Zauscher, I. Luzinov and S. Minko, Emerging applications of stimuli-responsive polymer materials, Nat. Mater., 2010, 9, 101–113 CrossRef PubMed.
- M. Bustamante-Torres, D. Romero-Fierro, B. Arcentales-Vera, K. Palomino, H. Magaña and E. Bucio, Hydrogels classification according to the physical or chemical interactions and as stimuli-sensitive materials, Gels, 2021, 7, 182 CrossRef CAS PubMed.
- F. Diehl, S. Hageneder, S. Fossati, S. K. Auer, J. Dostalek and U. Jonas, Plasmonic nanomaterials with responsive polymer hydrogels for sensing and actuation, Chem. Soc. Rev., 2022, 51, 3926–3963 RSC.
- A. Bordbar-Khiabani and M. Gasik, Smart Hydrogels for Advanced Drug Delivery Systems, Int. J. Mol. Sci., 2022, 23, 3665 CrossRef CAS PubMed.
- K. Matthias, P. Andrij, H. Thomas, H. Todd, L. A. Lyon, J. J. Crassous, D. Suzuki, A. G. Rustam, S. Stefanie, I. P. Igor and R. Walter, Nanogels and microgels: from model colloids to applications, recent developments, and future trends, Langmuir, 2019, 35, 6231–6255 CrossRef PubMed.
- X. Xu, Y. Liu, W. Fu, M. Yao, Z. Ding, J. Xuan, D. Li, S. Wang, Y. Xia and M. Cao, Poly(N-isopropylacrylamide)-based thermoresponsive composite hydrogels for biomedical applications, Polymers, 2020, 12, 580 CrossRef CAS PubMed.
- M. Rizwan, R. Yahya, A. Hassan, M. Yar, A. D. Azzahari, V. Selvanathan, F. Sonsudin and C. N. Abouloula, pH sensitive hydrogels in drug delivery: brief history, properties, swelling, and release mechanism, material selection and applications, Polymers, 2017, 9, 137 CrossRef PubMed.
- M. U. A. Khan, S. I. A. Razak, W. S. A. Arjan, S. Nazir, T. J. S. Anand, H. Mehboob and R. Amin, Recent advances in biopolymeric composite materials for tissue engineering and regenerative medicines: a review, Molecules, 2021, 26, 619 CrossRef CAS.
- L. I. Atanase, Micellar drug delivery systems based on natural biopolymers, Polymers, 2021, 13, 477 CrossRef CAS PubMed.
- M. Fwu-Long, S. Shin-Shing, W. Yu-Bey, L. Sung-Tao, S. Jen-Yeu and H. Rong-Nan, Fabrication and characterization of a sponge-like asymmetric chitosan membrane as a wound dressing, Biomaterials, 2001, 22, 165–173 CrossRef PubMed.
- A. E. Stoica, C. Chircov and A. M. Grumezescu, Nanomaterials for wound dressings: an up-to-date overview, Molecules, 2020, 25, 2699 CrossRef CAS PubMed.
- M. N. V. Ravi Kumar, R. A. A. Muzzarelli, C. Muzzarelli, H. Sashiwa and A. J. Domb, Chitosan chemistry and pharmaceutical perspectives, Chem. Rev., 2004, 104, 6017–6084 CrossRef PubMed.
- N. M. Salwowska, K. A. Bebenek, D. A. Zazdło and D. L. Wcisło-Dziadecka, Physiochemical properties and application of hyaluronic acid: a systematic review, J. Cosmet. Dermatol., 2016, 15, 520–526 CrossRef PubMed.
- R. A. A. Muzzarellia, F. Grecoa, A. Busilacchia, V. Sollazzob and A. Gigante, Chitosan, hyaluronan and chondroitin sulfate in tissue engineering for cartilage regeneration: A review, Carbohydr. Polym., 2012, 89, 723–739 CrossRef PubMed.
- V. Vijayalekshmi and D. Khastgir, Eco-friendly methanesulfonic acid and sodium salt of dodecylbenzene sulfonic acid doped cross-linked chitosan based green polymer electrolyte membranes for fuel cell applications, J. Membr. Sci., 2017, 523, 45–59 CrossRef CAS.
- J. J. Water, M.
M. Schack, A. Velazquez-Campoy, M. J. Maltesen, M. van de Weert and L. Jorgensen, Complex coacervates of hyaluronic acid and lysozyme: Effect on protein structure and physical stability, Eur. J. Pharm. Sci., 2014, 88, 325–331 CAS.
- J. Wu, X. Wang, J. K. Keum, H. Zhou, M. Gelfer, C. Avila-Orta, H. Pan, W. Chen, S. Chiao, B. S. Hsiao and B. Chu, Water soluble complexes of chitosan-g-MPEG and hyaluronic acid, J. Biomed. Mater. Res., Part B, 2007, 80, 800–812 CrossRef.
- K. Ohkawa, M. Yamada, A. Nishida, N. Nishi and H. Yamamoto, Biodegradation of chitosan-gellan and poly(l-lysine)-gellan polyion complex fibers by pure cultures of soil filamentous fungi, J. Polym. Environ., 2000, 8, 59–66 CrossRef CAS.
- K. Iijima, A. Suzuki and M. Hashizume, Preparation of swellable and highly operable composite films made of polysaccharide and supporting substrates, Kobunshi Ronbunshu, 2018, 75, 195–202 CrossRef CAS.
- Y. Yataka, A. Suzuki, K. Iijima and M. Hashizume, Enhancement of the mechanical properties of polysaccharide composite films utilizing cellulose nanofibers, Polym. J., 2020, 52, 645–653 CrossRef CAS.
- P. Coimbra, P. Alves, T. A. M. Valente, R. Santos, I. J. Correia and P. Ferreira, Sodium hyaluronate/chitosan polyelectrolyte complex scaffolds for dental pulp regeneration: Synthesis and characterization, J. Biol. Macromol., 2011, 49, 573–579 CrossRef CAS PubMed.
- T. Delair, Colloidal polyelectrolyte complexes of chitosan and dextran sulfate towards versatile nanocarriers of bioactive molecules, Eur. J. Pharm. Sci., 2011, 78, 10–18 CAS.
- Z. Li, H. R. Ramay, K. D. Hauch, D. Xiao and M. Zhang, Chitosan–alginate hybrid scaffolds for bone tissue engineering, Biomaterials, 2005, 26, 3919–3928 CrossRef CAS.
- A. Sintov, N. Di-Capua and A. Rubinstein, Cross-linked chondroitin sulphate: characterization for drug delivery purposes, Biomaterials, 1995, 16, 473–478 CrossRef CAS PubMed.
- N. Z. Alexander, Drug releasing polymer thin films: new era of surface-mediated drug delivery, ACS Nano, 2010, 4, 2494–2509 CrossRef.
- K. Ariga, Y. Yamauchi, G. Rydzek, Q. Ji, Y. Yonamine, K. C.-W. Wu and J. P. Hill, Layer-by-layer Nanoarchitectonics: Invention, Innovation, and Evolution, Chem. Lett., 2014, 43, 36–38 CrossRef CAS.
- K. Ariga, E. Ahn, M. Park and B.-S. Kim, Layer-by-Layer Assembly: Recent Progress from Layered Assemblies to Layered Nanoarchitectonics, Molecules, 2019, 14, 2553 CAS.
- G. Decher, Fuzzy Nanoassemblies: Toward Layered Polymeric Multicomposites, Science, 1997, 277, 1232–1237 CrossRef CAS.
- P. T. Hammond, Form and function in multilayer assembly: new applications at the nanoscale, Adv. Mater., 2004, 16, 1271–1293 CrossRef CAS.
- T. Fujie, Y. Okamura and S. Takeoka, Ubiquitous transference of a free-standing polysaccharide nanosheet with the development of a nano-adhesive plaster, Adv. Mater., 2007, 19, 3549–3553 CrossRef CAS.
- V. Gribova, R. Auzely–Velty and C. Picart, Polyelectrolyte multilayer assemblies on materials surfaces: from cell adhesion to tissue engineering, Chem. Mater., 2011, 24, 854–869 CrossRef PubMed.
- S. G. Caridade, C. Monge, F. Gilde, T. Boudou, J. F. Mano and C. Picart, Free–standing polyelectrolyte membranes made of chitosan and alginate, Biomacromolecules, 2013, 14, 1653–1660 CrossRef CAS PubMed.
- X. Qi, W. Pan, X. Tong, T. Gao, Y. Xiang, S. You, R. Mao, J. Chi, R. Hu, W. Zhang, H. Deng and J. Shen, ε-Polylysine-stabilized agarose/polydopamine hydrogel dressings with robust photothermal property for
wound healing, Carbohydr. Polym., 2021, 264, 118046 CrossRef CAS.
- G. Lalevée, G. Sudre, A. Montembault, J. Meadows, S. Malaise, A. Crépet, L. David and T. Delair, Polyelectrolyte complexes via desalting mixtures of hyaluronic acid and chitosan—Physicochemical study and structural analysis, Carbohydr. Polym., 2016, 154, 86–95 CrossRef PubMed.
- H. Chen and M. Fan, Chitosan/carboxymethyl cellulose polyelectrolyte complex scaffolds for pulp cells regeneration, J. Bioact. Compat. Polym., 2007, 22, 475–491 CrossRef CAS.
- A. Drogoz, L. David, C. Rochas, A. Domard and T. Delair, Polyelectrolyte complexes from polysaccharides: formation and stoichiometry monitoring, Langmuir, 2007, 23, 10950–10958 CrossRef CAS PubMed.
- M. Hashizume, H. Kobayashi and M. Ohashi, Preparation of free–standing films of natural polysaccharides using hot press technique and their surface functionalization with biomimetic apatite, Colloids Surf., B, 2011, 88, 534–538 CrossRef CAS PubMed.
- M. Hashizume, M. Ohashi, H. Kobayashi, Y. Tsuji and K. Iijima, Free-standing polysaccharide composite films: Improved preparation and physical properties, Colloids Surf., A, 2015, 483, 18–24 CrossRef CAS.
- M. Hashizume, Y. Murata, K. Iijima and T. Shibata, Drug loading and release behaviors of freestanding polysaccharide composite films, Polym. J., 2016, 48, 545–554 CrossRef CAS.
- K. Iijima, Y. Tsuji, I. Kuriki, A. Kakimoto, Y. Nikaido, R. Ninomiya, T. Iyoda, F. Fukai and M. Hashizume, Control of cell adhesion and proliferation utilizing polysaccharide composite film scaffolds, Colloids Surf., B, 2017, 160, 228–237 CrossRef CAS.
- K. Iijima, T. Kimura, R. Sato, T. Takahashi and M. Hashizume, Kinetic Analysis of molecular permeabilities of free-standing polysaccharide composite films, Macromol. Chem. Phys., 2017, 218, 1600391 CrossRef.
- T. Sagawa, M. Sakakibara, K. Iijima, Y. Yataka and M. Hashizume, Preparation and physical properties of free-standing films made of polyion complexes of carboxymethylated hyaluronic acid and chitosan, Polymer, 2022, 253, 125033 CrossRef CAS.
- Z. Tamura, T. Morioka, M. Maeda and A. Tsuji, Spectrophotometric estimation of the pKa values, absorption spectra and structural formulas of molecular species in aqueous solutions of fluorescein and sulfonefluorescein, Bunseki Kagaku, 1993, 43, 339–346 CrossRef.
- J. W. Park and B. Chakrabarti, Optical characteristics of carboxyl group in relation to the circular dichroic properties and dissociation constants of glycosaminoglycans, Biochim. Biophys. Acta, 1978, 554, 667–675 CrossRef PubMed.
- A. Domard, pH and c.d. measurements on a fully deacetylated chitosan: application to CuII—polymer interactions, Int. J. Biol. Macromol., 1987, 9, 98–104 CrossRef CAS.
- D. T. D. Z. Atvars, Thermal transitions of poly(vinyl alcohol) hydrogel sensed by a fluorescent probe, J. Appl. Polym., 2000, 75, 815–824 CrossRef.
- H. Frederi and T. Esben, Influence of Ionic Strength and Specific Ion Effects on Polyelectrolyte Multilayer Films with pH-Responsive Behavior, Langmuir, 2023, 39, 5012–5020 CrossRef PubMed.
- P. Gong, T. Wu and I. Sxleifer, Behavior of Surface-Anchored Poly(acrylic acid) Brushes with Grafting Density Gradients on Solid Substrates:
2. Theory, Macromolecules, 2007, 40, 8765–8773 CrossRef CAS.
- K. Ehtiati, S. Z. Moghaddam, A. E. Daugaard and E. Thormann, How Dissociation of Carboxylic Acid Groups in a Weak Polyelectrolyte Brush Depend on Their Distance from the Substrate, Langmuir, 2020, 36, 2339–2348 CrossRef CAS PubMed.
- B. Wang, W. Gao, Y. Ma, D. Li, L. Wua and L. Bi, Enhanced sensitivity of color/emission switching of fluorescein film by incorporation of polyoxometalate using HCl and NH3 gases as in situ stimuli, RSC Adv., 2015, 5, 41814–41819 RSC.
|
This journal is © The Royal Society of Chemistry 2023 |