High-performance FeOx@CoOx/NC electrocatalysts for the oxygen reduction reaction in alkaline media†
Received
27th September 2022
, Accepted 10th November 2022
First published on 16th November 2022
Abstract
Herein, cobalt oxide encapsulated nitrogen-doped tubular carbon (CoOx/NC) is derived via one-step carbonization of ZIF-12. Iron oxide nanoparticles are then generated over the CoOx/NC support by decomposition of the iron phenanthroline complex to obtain FeOx@CoOx/NCs. The synthesized electrocatalysts are tested for the oxygen reduction reaction (ORR). The optimized electrocatalyst FeOx@CoOx/NC2 exhibits superior activity to 20 wt% Pt/C in 0.1 M KOH with the E1/2 = 0.89 VRHE, Eonset=1.03 VRHE and j = 6.32 mA cm−2 values. The enhanced activity is attributed to the cumulative effect of FeOx, CoOx and presence of four types of nitrogen atoms. For comparison, FeOx@MWCNTs demonstrates lower activity than the synthesized catalysts (E1/2 = 0.76 VRHE and Eonset = 0.94 VRHE) which proves the synergistic role of CoOx towards the ORR. The oxygen reduction reaction ability of the synthesized catalyst (FeOx@CoOx/NC2) is also investigated in the presence of 0.5 M methanol. The catalyst exhibits enhanced tolerance to methanol addition. The accelerated durability test (ADT) performed in 0.1 M KOH over 10
000 cycles shows a decay of only 17 mV.
Introduction
The cumulative global energy crisis encourages the development of electrochemical energy conversion technologies such as fuel cells and metal–air batteries due to their high-power density, low emission of gases, high efficiency and renewable source of reactants.1 The upcoming energy crisis can be alleviated by the pivotal process of the cathodic oxygen reduction reaction (ORR). Porous structure catalysts are considered important for efficient electrocatalysis.2 The sluggish reaction kinetics of the ORR always necessitates the high loading of Pt and Pd. However, the precious and scarce nature, dissolution and durability of these metals challenge the commercialization of fuel cell technology.3–7 Motivated by this challenge, lots of efforts have been devoted to exploit high-performance electrocatalysts for oxygen reduction with accelerated kinetics, low overpotential and low cost to completely replace the Pt-based catalysts.8 Zhaojun et al. synthesized hollow PtFe alloy nanoparticles from Pt–Fe3O4 dimers through a silica-protection reduction strategy for effective electrochemical reduction of oxygen. The incorporation of iron into Pt reduces the cost of noble metal-based electrocatalysts.9 Xiaoying et al. synthesized low cost NiFe alloy nanoparticles decorated over nitrogen-doped carbon nanotubes as a low-cost bifunctional electrocatalyst towards the oxygen evolution reaction (OER) and oxygen reduction reaction (ORR).10 Similarly, FeCu-DA/NC, a low cost ORR electrocatalyst was reported by Cheng et al. which exhibited 30 mV more positive half-wave potential than the state of the art catalyst (Pt/C).11 Metal-free heteroatom-doped carbon-based materials have been reported by researchers as efficient catalysts for ORR.12 The doping of nitrogen into the carbon matrix formulates a different electronic environment around the carbon centres, creates active sites, and modulates chemisorption energy of O2 and the reaction mechanism (2e−/4e−) leading to enhanced electrocatalytic activity.12,13 Liang Chen et al. recently studied the effect of dopant concentration and carbon precursor on the electrocatalytic activity of the synthesized materials towards the ORR.14 However, the dissolution and irremediable deterioration of carbon-based materials under harsh environmental conditions of fuel cells limits the conversion efficiency of fuel cells.15–17 In contrast, a nanostructured transition metal oxide support could be a good candidate as an electrocatalyst for the ORR. Transition metal oxides change the electronic properties and Fermi level of the loaded nanoparticles through strong metal support interaction (SMSI).18 The SMSI minimizes the surface energy by modulating the properties of the supported particles leading to enhancement in electrocatalytic ability and durability.19 Their low conductivity, dissolution, sintering, agglomeration and poor electrochemical properties can be effectively buffered by the intrinsic conductivity of carbon-based support materials.20,21 For instance, Weifeng et al. reported quantum dot-stabilized transition-metal nanoparticles for the hydrogen evolution reaction (HER).22 Xiangqian et al. synthesized α-Fe2O3/GO and investigated its ORR activity which was superior to that of α-FeOOH and α/γ-FeOOH supported over GO (graphene oxide).23 Dena et al. synthesized a mixed transition metal oxide (MTMO) supported over Vulcan XC-72 (M = Co, Cu, Mn) to efficiently catalyse the ORR.24 Similarly transition metal nanoparticle decorated carbon-based materials with co-doping of sulphur and nitrogen have also been investigated for the ORR.25 Recently, M–N–C–based catalysts with varied metal centres have been explored as the oxygen reduction electrocatalysts with comparable activity to Pt, thus setting up a new generation of electrocatalysts for the ORR. Among the non-noble metals, Fe–N–C and Co–N–C have proven to be highly active for the ORR because of the active sites created on the surface by metal–nitrogen coordination (M–Nx) during the pyrolysis step.8,26 The coordination of different types of nitrogen to the metal centre and in particular to iron imparts excellent stability to the electrocatalyst. The outstanding electrocatalytic activity in alkaline media and impressive activity in acidic media for the ORR using Fe–Nx/C catalysts have been witnessed among the newly developed M–N–C catalysts.27 For example, Zhengkun et al. reported Fe SAs/N–C which exhibited superior ORR activity in alkaline and acidic environments with excellent cycling stability compared to Pt/C.28 Similarly, Lianli et al. synthesized Fe–N/CNRib and studied the metal active sites immobilization during electrochemical performance.29 Han et al. investigated ORR catalytic activity of the UF Co–N–C electrocatalyst.30 A promising way to increase the catalytic activity of M–N–C type materials is to introduce adjacent isolated metal centres which optimize the electronic structure of metal active sites and accelerate the ORR kinetics.31 Recently, Ali et al. studied the modulation effect of the adjacent Pt–N4 moiety on the isolated Fe–N4 sites, where 3d electronic orbitals of Fe–N4 sites were tailored by Pt–N4 sites with optimized ORR activity.32 However, the traditional method of pyrolysis at elevated temperature leading to compositional and highly structural heterogeneity hinders the correlation between the electrocatalytic activity and atomic structure of single metal atom sites.31 In addition, expensive and complicated characterization techniques also obstruct the synthesis of single-atom–coordinated nitrogen centres (M–N–C) as electrocatalysts for the ORR. Therefore, an efficient, simple, and scalable approach is needed for the synthesis of a highly efficient and stable electrocatalytic material that eliminates the formation of single atoms with superior activity to the benchmark catalyst (20 wt% Pt/C). In view of the above discussion, herein we report a novel iron oxide decorated CoOx encapsulated nitrogen-doped tubular carbon (NC) which is found to be a highly efficient and extremely stable electrocatalytic material. The synthesis of bimetallic transition metal oxide incorporated nitrogen-doped carbon as a catalyst for electrochemical applications involves a complex preparation process. The method presented here is facile and consists of two steps with the encapsulation of CoOx nanoparticles in the ligand-derived matrix via pyrolysis by using ZIF-12 as the precursor in the first step. The second step involves the deposition of FeOx nanoparticles over this support material. The synthesized electrocatalyst displayed superior activity to 20 wt% Pt/C for the ORR. The concentration effect was examined by synthesizing different materials by varying the concentration of the precursor metal salt (iron sulphate heptahydrate). The enhanced electrocatalytic ability is attributed to outstanding properties of one-dimensional nitrogen-doped tubular carbon (NC) encapsulating CoOx and having FeOx nanoparticles deposited over its surface. The four types of nitrogen (graphitic, pyridinic, pyrrolic and oxidized) in the carbon matrix tailor the electronic properties of the FeOx sites leading to enhanced activity. The d-band centre of FeOx was tailored by CoOx and four types of nitrogen, unveiling enhanced activity of the synthesized catalyst towards the ORR.
Experimental
Synthesis of ZIF-12
Cobalt nitrate hexahydrate (1.318 mmol) and benzimidazole (6.09 mmol) were dissolved separately in 7 mL of N,N-dimethylformamide, mixed and stirred. The solution was then transferred to a Teflon-lined stainless steel autoclave. The autoclave was heated to 150 °C in an oven for two days. After cooling to room temperature, a purple coloured crystalline product was obtained. The product was washed with distilled water and DMF, dried and used for further processing.
Synthesis of the CoOx/NC support material
The synthesized ZIF-12 was calcined in a tube furnace at elevated temperature under an inert atmosphere. For this purpose, ZIF-12 (200 mg) was transferred to a quartz boat and placed in a tube furnace. The tube furnace was degassed by flowing Ar for 2 h to eliminate oxygen gas. The system was then heated to 850 °C for 8 h at a heating rate of 5 °C min−1. After natural cooling to room temperature, the product was collected and further treated with analytical grade HCl to remove excess cobalt species adhering to the surface and to functionalize the surface so that the adsorption capacity and dispensability can be increased. After acid leaching, the material still retained ca. 2.2 wt% of cobalt as indicated by XPS analysis and AAS analysis.
Synthesis of catalysts
Three types of catalysts were synthesized by dispersing CoOx/NC (150 mg) in deionized water (20 mL) in a sonicator for 120 min. Afterward, 50 μL of each solution of aqueous iron sulphate heptahydrate (FeSO4·7H2O) with different concentrations, i.e., 0.1 mM, 0.167 mM and 0.35 mM and 50 μL of each solution of aqueous 1,10-phenanthroline having concentrations of 0.3 mM, 0.5 mM and 1.1 mM were added to synthesize FeOx@CoOx/NC1, FeOx@CoOx/NC2, and FeOx@CoOx/NC3, respectively. The materials were air dried in an oven at 70 °C. The dried materials were then transferred to an alumina boat and heated to 100 °C at a heating rate of 5 °C min−1 and maintained at that temperature for 60 min. The whole process was carried out under an inert atmosphere. After natural cooling to room temperature, the product was collected, characterized, and used for electrochemical studies. To investigate the concentration effect, catalysts with different concentrations were obtained and their electrochemical activities were measured subsequently. For comparison, FeOx supported over commercial multiwalled carbon nanotubes (FeOx@MWCNTs) was synthesized to observe the effect of cobalt oxide together with iron oxide towards the oxygen reduction reaction. The catalyst (FeOx@MWCNTs) was synthesized following the same synthetic protocol as adopted for FeOx@CoOx/NC2.
Electrochemical measurements
An electrochemical workstation was used for assessing the catalytic performance. The electrochemical setup was composed of a single compartment cell in which a glassy carbon (GC) rotating ring disk (RDE) electrode was used as the substrate for the fabrication of the working electrode. Hg/HgO and a graphite rod were used as the reference and counter electrodes, respectively. The working electrodes of all the synthesized electrocatalysts (FeOx@CoOx/NC1, FeOx@CoOx/NC2 and FeOx@CoOx/NC3) were fabricated by dispersing 2 mg of synthesized catalysts in 80 μL isopropanol and 20 μL of 5% Nafion mixture. The mixture was then sonicated for 3 h to fully disperse the synthesized electrocatalyst and to prepare a homogenous slurry. The prepared ink (5 μL) was then deposited on the GC RDE by the drop casting technique. Cyclic voltammetry (CV) and linear sweep voltammetry (LSV) were performed in 0.1 M KOH in an O2/Ar saturated environment to determine the cathodic oxygen reduction ability of the synthesized catalysts. All the potentials were transformed to the RHE scale for comparison with the reported literature. The current density was obtained by normalizing the current with respect to the geometric area of the electrode (0.19625 cm2). The obtained electrochemical results were compared to those of Pt/C (20 wt%), a reference and benchmark catalyst. To ensure the stability of the synthesized catalyst, the accelerated durability test (ADT) was performed over 10
000 cycles in an O2 saturated environment. The catalyst stability was also determined by chronoamperometric measurements. All the measurements were carried out at room temperature. Scheme 1 represents the synthetic protocol of FeOx@CoOx/NCs.
 |
| Scheme 1 Synthetic protocol for FeOx@CoOx/NCs. | |
Results and discussion
The powder X-ray diffraction characterization technique is used to determine the crystal structure, phase purity and chemical composition of the synthesized sample. Fig. 1a shows the X-ray diffractogram of calcined ZIF-12, i.e., CoOx-embedded NC and synthesized electrocatalysts. The strong diffraction peak at 2θ = 25.8° corresponds to the (002) facet of pyrolyzed graphitic carbon in all the synthesized electrocatalysts. It is clear from Fig. 1a that after the incorporation of FeOx, the graphitic carbon maintained its integrity in all the synthesized samples. The other two strong diffraction peaks detected at 2θ values of ∼44.4° and ∼51.8° (Fig. 1a) arising from the (004) and (100) facets could also be indexed to the graphitic carbon material, respectively, according to the standard pattern (JCPDS card no. 00-013-0148). The diffraction peak positions corresponding to graphitic carbon are identical in all the synthesized electrocatalysts. The diffraction peaks for cobalt oxide (CoOx) nanostructures are not marked in the powder X-ray diffractogram due to very low quantity of cobalt (2 wt%) as determined from the XPS analysis. However, these nanoparticles are clearly visible in HRTEM, XPS and SAED analysis.33 These nanoparticles are completely encapsulated by nitrogen-doped tubular carbon (NC) as confirmed from HRTEM analysis in our previous report.33 It is believed that polycrystalline cobalt oxides are embedded in the nitrogen-doped tubular carbon. As the concentration of cobalt is very low (2 wt%), the type of cobalt oxide cannot be determined from the conventional powder XRD pattern and more likely the mixed cobalt oxides (CoOx). The peaks for iron oxides (FeOx) are also not visible in the powder XRD pattern. The concentration of FeOx is around 1.1 wt% in the synthesized final material and hence cannot be determined from the powder XRD pattern. The intensity of diffraction peaks corresponding to graphitic carbon is decreased after the introduction of FeOx nanoparticles. The decrease in intensity is attributed to the formation of FeOx nanoparticles on the surface of tubular carbon. The XRD pattern of MWCNTs and FeOx@MWCNTs has been presented in Fig. S1.†
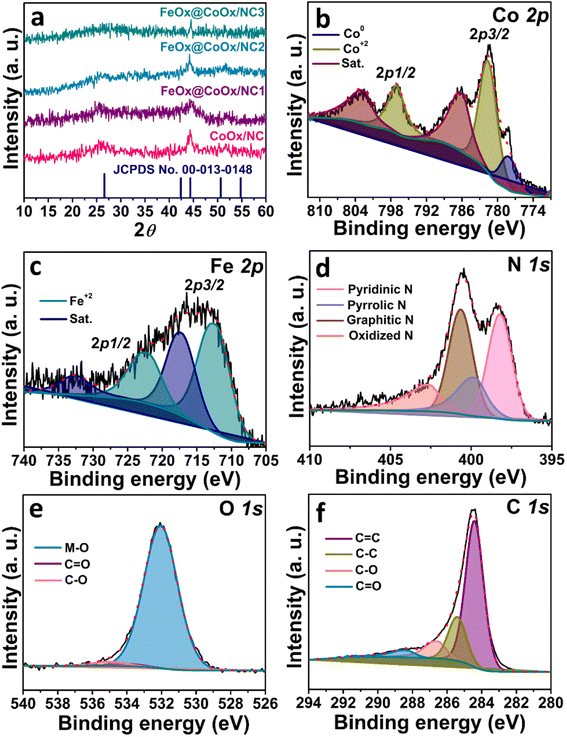 |
| Fig. 1 (a) PXRD pattern of the synthesized catalysts. (b) XPS spectrum of cobalt, (c) iron, (d) nitrogen, (e) oxygen, and (f) carbon in FeOx@CoOx/NC2. | |
To probe the oxidation states of metals, surface elemental composition and electronic properties of the synthesized electrocatalyst, X-ray photoelectron spectroscopy was performed (Fig. 1). The surface analysis of the synthesized electrocatalyst (FeOx@CoOx/NC2) confirms the presence of iron, cobalt, oxygen, nitrogen, and carbon. The high-resolution Co 2p spectrum can be deconvoluted into two major regions accompanying the satellite peaks. These two regions correspond to Co 2p3/2 and Co 2p1/2. The peak at 778.10 eV can be ascribed to metallic cobalt. The peaks at 781.5 eV and 797.02 eV corresponding to Co 2p3/2 and Co 2p1/2 regions respectively, are shifted positively from the standard binding energy of metallic cobalt (Fig. 1b). These shifts toward the higher binding energy side indicate that cobalt is present in the +2 oxidation state.33 The peaks at 786.01 eV and 802.59 eV represent the associated satellite peaks (Fig. 1b). The core-level XPS spectrum of iron is deconvoluted into two major peaks corresponding to Fe 2p3/2 (lower energy band) and Fe 2p1/2 (higher energy band). The peaks at 712.11 eV and 722.71 eV correspond to Fe2+ in Fe 2p3/2 and Fe 2p1/2 core lines, respectively. The peaks located at ∼717.43 eV and ∼732.61 eV are the associated satellite peaks (Fig. 1c).34 The nitrogen 1s core-level XPS spectrum is deconvoluted into four peaks. These four peaks located at ∼398.21 eV ∼399.7 eV ∼400.64 eV and ∼402.61 eV are indexed to the pyridinic, pyrrolic, graphitic and oxidized nitrogen respectively (Fig. 1d). The four types of nitrogen are supposed to provide stability to the synthesized electrocatalyst.1,28,32 The shifting of the d-band centre of FeOx downward to compensate for the distortion caused as a result of electronic interaction with cobalt oxides (CoOx) and nitrogens is in agreement with the d-band centre model. Enhanced electrocatalytic activity of the FeOx@CoOx/NC2 electrocatalyst is attributed to the cumulative electronic interaction of cobalt, iron and nitrogen sites, thereby modulating the chemisorption energy of O2 and the reaction mechanism.35 Three peaks located at ∼532.04 eV ∼534.04 eV and ∼535.04 eV are observed in the high-resolution profile of the O 1s XPS spectrum (Fig. 1e). These signals correspond to oxygen bonded to the metal surface and surface-bound oxygen, respectively.33 The deconvolution of the C 1s XPS spectrum reveals four peaks at binding energy values ∼284.48 eV, ∼285.42 eV, ∼286.7 eV and ∼288.6 eV (Fig. 1f). These peaks arise due to the presence of sp2(C
C) and sp3(C–C) hybridized carbon and carbon bonded to oxygen (C–O and C
O, respectively) in the synthesized electrocatalyst.36 The XPS spectrum of CoOx/NC has been presented in Fig. S6.† The percentage of cobalt and iron in the synthesized electrocatalyst (FeOx@CoOx/NC2) was also determined from the AAS technique and found to be 2.3% and 1.3%, respectively, which is in close agreement with the XPS results.
The morphology of bimetallic metal oxides and nitrogen-doped tubular carbon (NC) was further investigated by high-resolution transmission electron microscopy (HRTEM) measurements (Fig. 2). The formation of well-defined cylindrical tubes has been observed in the HRTEM analysis. The ends of nitrogen-doped tubular carbon are occupied by CoOx nanoparticles which are of ca. 50 nm in size. Some nanoparticles are less than 50 nm (around 22 nm) anchored on the surface of nitrogen-doped tubular carbon (Fig. S2†). These CoOx nanoparticles are encapsulated within the multilayers of nitrogen-doped tubular carbon. Eccentric, curved growth lines are formed during the formation of multiwalled tubular carbon. These curved lines are formed from the surface of nanoparticles in semi-circular layers (Fig. S2†). An intertwined mat of cylindrical nitrogen-doped tubular carbon with the interlinked nodes and internodes was developed by the condensation of carbon at elevated temperature. It is believed that adsorption capacity for the loaded nanoparticles is increased as these interlinked nodes provide anchoring sites to the metal for adsorption (Fig. S2†). Elemental mapping results further confirm the presence of cobalt, iron, nitrogen, carbon, and oxygen. Sphere-shaped iron oxide (FeOx) nanoparticles are significantly distributed over CoOx encapsulated nitrogen-doped tubular carbon. HRTEM elemental mapping analysis confirms the effective deposition of FeOx nanoparticles over the cobalt oxide embedded nitrogen-doped tubular carbon skeleton (CoOx/NC). These results are indication of successful loading of FeOx nanoparticles over the CoOx/NC skeleton. The fringes of FeOx nanoparticles are clearly visible in the HRTEM results at a resolution of 10 nm (Fig. 2b). These fringes of d-spacing of ∼0.271 nm and ∼0.187 nm can be ascribed to the (104) and (024) facets of FeOx nanoparticles, respectively. Fig. S3 and S7† show the EDX pattern and HAADF-TEM image of FeOx@CoOx/NC2.
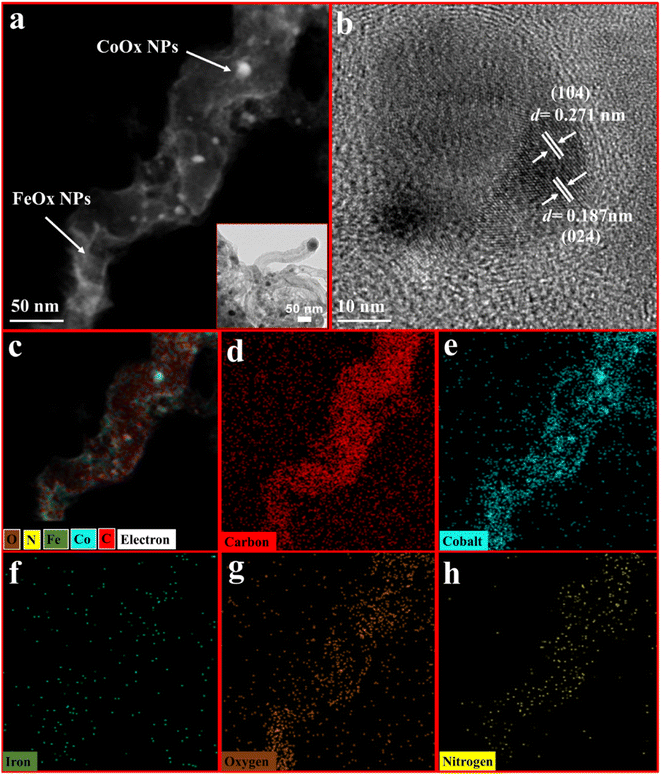 |
| Fig. 2 (a) HRTEM analysis of FeOx@CoOx/NC2 at 50 nm; the inset in (a) represents the HRTEM analysis of CoOx/NC at 50 nm. (b) HRTEM image of FeOx@CoOx/NC2 at 10 nm. (c) Elemental mapping of FeOx@CoOx/NC2;(d) carbon, (e) cobalt, (f) iron, (g) oxygen, and (h) nitrogen. | |
Electrochemical studies
Cyclic voltammetry (CV) and linear sweep voltammetry (LSV) curves were obtained in O2/Ar saturated 0.1 M KOH to assess the ORR catalytic ability of the synthesized materials. The aim was to investigate the synergistic effect of CoOx and FeOx leading to enhancement of electrochemical conversion of O2 to H2O through a direct route. By careful analysis of the electrochemical results, we concluded that the synergistic effect is maximum in FeOx@CoOx/NC2. XPS analysis of our present work reveals the percentage of cobalt to be 2.2 wt% and iron to be 1.1 wt% in our synthesized material (FeOx@CoOx/NC2). The percentage of cobalt and iron was also determined by AAS and found to be 2.3% and 1.3%, respectively. This means that the optimum ratio at which the interaction of cobalt oxide and iron oxide is maximum is 2
:
1. The strong chemical interaction between FeOx, CoOx and nitrogen-doped tubular carbon enhances the electrochemical behaviour of the synthesized material towards the ORR. The detailed mechanism of transition metal oxide (TMO)-based catalysts for the ORR is still under investigation. However, theoretical and experimental studies have shown that Fe3+ can easily oxidize cobalt in bimetallic catalysts, facilitating the formation of surface-adsorbed intermediates with optimum binding energies.37 The charge transfer between the loaded nanoparticles and substrate is due to strong metal support interaction (SMSI) resulting in noteworthy variation in the electronic distribution. This re-distribution of electronic densities modulates the chemisorption energies of adsorbed intermediates, facilitating the ORR kinetics.35 The superior catalytic activity and stability of FeOx@CoOx/NC2 can be attributed to the following key points. (1) Strong synergism between FeOx, CoOx and four types of nitrogen in nitrogen-doped tubular carbon; (2) the introduction of four types of nitrogen as a result of decomposition of 1,10-phenanthroline induces uneven charge distribution in the carbon matrix, greatly facilitating the charge transfer; (3) greater number of active sites and exposed surface area of the synthesized electrocatalyst.35 To assess the efficiency of the catalyst, all the electrochemical results were compared to those of commercial Pt/C under the same experimental conditions.
The ORR is a sluggish reaction and generally follows two routes in alkaline media, i.e., a direct pathway which proceeds through four electrons leading to the final product and an indirect pathway which proceeds through two electrons. The low overpotential of the direct pathway favours it over two-electron pathways. Besides low overpotential required for an efficient catalyst, chemisorption strength of adsorbed O2 on the surface of the catalyst is also a major factor which determines the effectiveness of the catalyst.36,38 From the reported literature, iron-based materials have been found to be highly efficient for catalysing the oxygen reduction reaction (ORR), particularly iron single-atom catalysts.26,28,39,40 However, the effect of cobalt oxide on the d-band centre of iron oxide for the ORR has not been reported yet to the best of our knowledge. Hence, the design of hybrid CoOx and FeOx nanoparticle sites supported over nitrogen-doped tubular carbon in this work is noteworthy. To measure the ORR activity of all synthesized electrocatalysts, cyclic voltammetry (CV) and linear sweep voltammetry (LSV) curves were recorded by employing the rotating disk electrode technique (RDE). The LSV curves were obtained in a wide range of rotation rates in an O2-saturated environment in 0.1 M KOH at a scan rate of 10 mV s−1. The linear sweep voltammetry curves were recorded under hydrodynamic conditions viz., RDE measurements over a similar potential window to study the kinetics of the ORR. The representative LSV curves of FeOx@CoOx/NCs have been presented in Fig. 3. The LSV curves of FeOx@CoOx/NC2 demonstrated a direct relation of current density with the rotation rate, signifying that the synthesized electrocatalyst is active towards the ORR in 0.1 M KOH (Fig. S4†). A superior onset potential (1.03 VRHE) and comparable current density (6.32 mA cm−2) with commercial Pt/C (20 wt%) were exhibited by the synthesized final electrocatalyst (FeOx@CoOx/NC2). The half wave potential (E1/2), which is the main determining parameter for assessing the electrocatalytic performance of a material towards the ORR is much higher for FeOx@CoOx/NC2 than Pt/C (Fig. 3a and f). The activities of FeOx@CoOx/NC1 and FeOx@CoOx/NC3 are inferior to those of Pt/C, a reference and benchmark catalyst (Fig. 3c and f). A possible reason for this is that the synergistic effect is maximum between CoOx and FeOx in FeOx@CoOx/NC2 compared to the other two catalysts. From this we can conclude that the optimum ratio of cobalt and iron for enhanced electrocatalytic activity towards the ORR is 2
:
1, respectively. For comparative studies, FeOx nanoparticles were deposited over commercial multiwalled carbon nanotubes (MWCNTs) to determine the contribution of cobalt towards lowering the d-band centre of iron. The catalyst (FeOx@MWCNTs) displayed greatly a negative shift from FeOx@CoOx/NC2 and Pt/C (Eonset: 0.94 VRHE, E1/2: 0.77 VRHE, and j: 3.83 mA cm−2) in 0.1 M KOH which validates the effect of cobalt on the d-band centre of iron (Fig. 3b and f). The LSV polarization curve of commercial multiwalled carbon nanotubes (MWCNTs) in 0.1 M KOH has been given in Fig. S9.† The ORR activity of MWCNTs is inferior to that of FeOx@MWCNTs with an Eonset of 0.85 VRHE and an E1/2 of 0.73 VRHE, which supports the effect of transition metal oxides (TMOs) on the ORR activity of MWCNTs. To determine the reaction kinetics, experimental data were plotted between 1/j and the inverse of rotation rates in a wide potential window and from that Koutecky–Levich plots were drawn (Fig. 4a). The electron transfer number based on the K–L plot was calculated to be 3.8 ensuring the direct pathway on the surface of the synthesized electrocatalyst (FeOx@CoOx/NC2). To further unfold the reaction kinetics, Tafel plots were drawn from the analogous polarization curves for all the synthesized electrocatalysts. Our material (FeOx@CoOx/NC2) exhibited a smaller Tafel slope than the commercial Pt/C, which validates better ORR kinetics on the surface of the synthesized material (Fig. 3e). The stability of the synthesized electrocatalyst was determined by performing the accelerated durability test (ADT) over 10
000 cycles. The catalyst decayed only by 17 mV indicating excellent cycling stability of the synthesized electrocatalyst (Fig. 3d). The catalyst stability was also determined by recording the current vs. time response at 1600 rpm for a period of 12 h in an O2 saturated environment. The catalyst (FeOx@CoOx/NC2) retained 97.8% of its initial current density after chronoamperometric measurements (Fig. S8†). The electrocatalytic activity of Pt/C (20 wt%) towards the ORR was also recorded for comparison. The electrocatalytic ability of all the synthesized electrocatalysts is in the order: FeOx@CoOx/NC2 > Pt/C > FeOx@CoOx/NC3 > FeOx@CoOx/NC1 > FeOx@MWCNTs > CoOx/NC > MWCNTs. The comparison of the ORR evaluating parameters of all the synthesized electrocatalysts with those of Pt/C has been presented in a bar graph (Fig. 3f and S5†). The enhanced electrocatalytic ability of FeO@CoOx/NC2 can be attributed to a greater number of exposed active sites which is directly related to the electrochemical active surface area (ECSA) of the working electrode. The electrochemical active surface area of all the synthesized electrocatalysts was estimated by recording cyclic voltammetry curves in 0.1 M KOH in an Ar saturated environment. The curves were documented in the non-faradaic potential region (Fig. S10†). By plotting average current densities (ja − jc/2) versus scan rates at 0.96 VRHE, double layer capacitance (Cdl) was calculated. The ECSA of the as-synthesized electrocatalysts was calculated by dividing the slope (Cdl) obtained from the linear fit to experimental data by the specific capacitance (Cs) using the following relation (Fig. 4b).
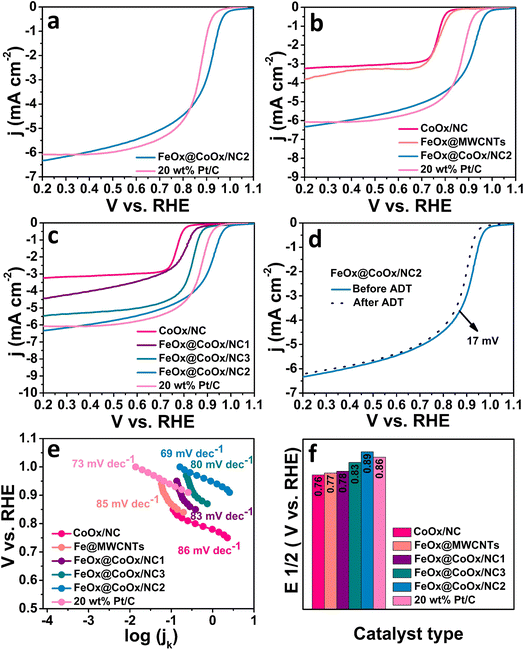 |
| Fig. 3 Electrocatalytic ability of the synthesized materials towards the ORR at 1600 rpm at a scan rate of 10 mV s−1. (a) Comparison of electrocatalytic activity of FeOx@CoOx/NC2 and 20 wt% Pt/C, (b) controlled experiment and comparison of ORR performance with the synthesized catalysts and 20 wt% Pt/C, (c) effect of concentration on the synthesized materials, (d) accelerated durability test of FeOx@CoOx/NC2, (e) Tafel slope of the as-synthesized catalysts, and (f) comparison of E1/2 of the synthesized catalysts and 20 wt% Pt/C. | |
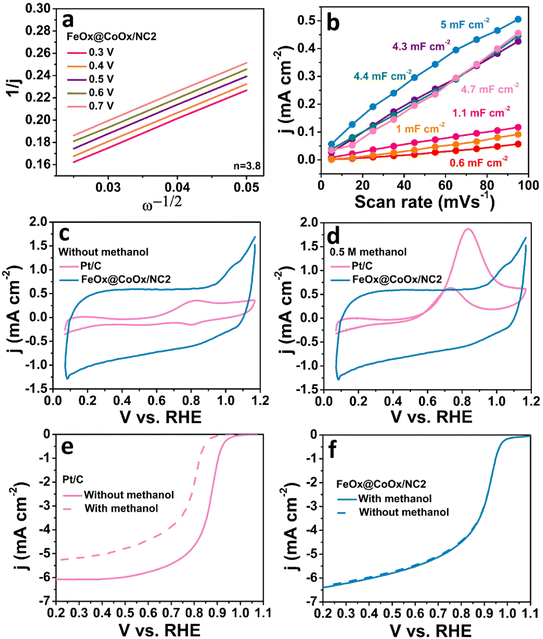 |
| Fig. 4 (a) Electron number of FeOx@CoOx/NC2. (b) Linear fit to experimental data obtained from CV curves of all the synthesized electrocatalysts at a fixed potential (0.96 VRHE). (c) CV curves of FeOx@CoOx/NC2 and Pt/C in the absence of methanol. (d) CV curves of FeOx@CoOx/NC2 and Pt/C in the presence of 0.5 M methanol. (e) Effect of methanol on the ORR polarization curve of Pt/C. (f) Effect of methanol on the ORR polarization curve of FeOx@CoOx/NC2. | |
The standard value of 40 μF cm−2 has been chosen for specific capacitance.41,42 The estimated value of ECSA ∼125 cm2 was calculated for FeOx@CoOx/NC2 by using the above relation. Table S1† gives the values of ECSA for all the synthesized electrocatalysts.
The ORR activity of the synthesized electrocatalyst (FeOx@CoOx/NC2) was also investigated in the presence of 0.5 M methanol. The synthesized material exhibited enhanced tolerance to methanol in comparison to 20 wt% Pt/C. The LSV polarization curve of FeOx@CoOx/NC2 remained unaffected after the addition of 0.5 M methanol (Fig. 4f). However, the ORR polarization curve of Pt/C decayed by 75 mV, signifying the occupation of active sites by methanol molecules (Fig. 4e). The absence of methanol oxidation peaks in the cyclic voltammetry curves of FeOx@CoOx/NC2 in the presence of 0.5 M methanol further confirms enhanced tolerance to methanol addition in comparison to 20 wt% Pt/C in which strong methanol oxidation peaks were observed (Fig. 4d). Table 1 compares the electrocatalytic activity of the synthesized material (FeOx@CoOx/NC2) with the reported work.
Table 1 Comparison of the synthesized electrocatalysts with the reported literature
Catalysts |
E
onset (VRHE) KOH |
E
1/2 (VRHE) |
Reference |
FeOx@CoOx/NC |
1.03 |
0.89 |
This work |
FeO1.4/N–C |
0.91 |
0.78 |
34
|
Fe–N/C |
0.94 |
0.81 |
26
|
Fe/N-G-SAC |
0.98 |
0.89 |
8
|
Fe-PA/ML0.5-900 |
1.00 |
0.86 |
1
|
Ca2FeRuO6 |
0.90 |
0.78 |
43
|
C/TP-Fe700 |
0.97 |
0.86 |
44
|
K-FePc2Ph |
0.95 |
0.88 |
45
|
FeS/Fe3C@NS-C-900 |
1.03 |
0.78 |
46
|
Fe/Meso-NC-1000 |
0.97 |
0.88 |
31
|
The ORR electrocatalytic activity of FeOx@CoOx/NC2 was compared with that of composites of iron, iron selenides and single iron atoms deposited over nitrogen-doped carbon reported in the literature. The as-synthesized material (FeOx@CoOx/NC2) exhibited comparable activity in terms of half wave potential with the iron single metal atom catalyst (Fe/N-G-SAC).8 The onset potential of FeOx@CoOx/NC2 is far more superior to that of Fe/N-G-SAC. The enhanced electrocatalytic activity of FeOx@CoOx/NC2 is the result of synergistic interaction of CoOx and FeOx supported over nitrogen-doped tubular carbon (NC).
Conclusions
To summarize, we have analysed the optimal regulation of the electronic structure of FeOx and confirmed that CoOx and four types of nitrogen can promote the ORR activity of the synthesized electrocatalyst (FeOx@CoOx/NC2). A series of catalysts with different iron concentrations have been designed. FeOx@CoOx/NC2, being the most active towards the ORR has optimum concentration of cobalt oxides and iron oxides with an E1/2 of 0.89 V which is superior to that of 20 wt% Pt/C. The as-synthesized catalyst exhibited superior durability in 0.1 M KOH over 10
000 cycles. The current versus time response of FeOx@CoOx/NC2 remained constant for a period of 12 h. The catalytic activity of FeOx@CoOx/NC2 was also investigated in the presence of 0.5 M methanol. The synthesized material demonstrated enhanced tolerance to methanol addition. The superior activity and enhanced tolerance to methanol addition could be attributed to the formation of different electronic environments around FeOx centres because of the interaction of CoOx nanoparticles and nitrogen sites. This work provides a new avenue for the development of cost effective and durable catalysts by using non-noble metals for the cathodic oxygen reduction reaction in fuel cells.
Conflicts of interest
There are no conflicts to declare.
Acknowledgements
This work was financially supported by the Pakistan Academy of Sciences (PAS) and Higher Education Commission (HEC) of Pakistan (No. 8400/Federal/NRPU/R&D/HEC/2017).
References
- A. Mahmood, N. Xie, B. Zhao, L. Zhong, Y. Zhang and L. Niu, Optimizing Surface N-Doping of Fe-N-C Catalysts Derived from Fe/Melamine-Decorated Polyaniline for Oxygen Reduction Electrocatalysis, Adv. Mater. Interfaces, 2021, 8(13), 2100197 CrossRef CAS
.
- C. Du, P. Li, Z. Zhuang, Z. Fang, S. He, L. Feng and W. Chen, Highly porous nanostructures: rational fabrication and promising application in energy electrocatalysis, Coord. Chem. Rev., 2022, 466, 214604 CrossRef CAS
.
- S. K. Singh, K. Takeyasu and J. Nakamura, Active sites and mechanism of oxygen reduction reaction electrocatalysis on nitrogen-doped carbon materials, Adv. Mater., 2019, 31(13), 1804297 CrossRef PubMed
.
- X. Liu and L. Dai, Carbon-based metal-free catalysts, Nat. Rev. Mater., 2016, 1(11), 1–12 Search PubMed
.
- S. Cherevko, G. P. Keeley, S. Geiger, A. R. Zeradjanin, N. Hodnik, N. Kulyk and K. J. Mayrhofer, Dissolution of platinum in the operational range of fuel cells, ChemElectroChem, 2015, 2(10), 1471–1478 CrossRef CAS PubMed
.
- C. Kim, F. Dionigi, V. Beermann, X. Wang, T. Möller and P. Strasser, Alloy nanocatalysts for the electrochemical oxygen reduction (ORR) and the direct electrochemical carbon dioxide reduction reaction (CO2RR), Adv. Mater., 2019, 31(31), 1805617 CrossRef PubMed
.
- M. Shao, Q. Chang, J.-P. Dodelet and R. Chenitz, Recent advances in electrocatalysts for oxygen reduction reaction, Chem. Rev., 2016, 116(6), 3594–3657 CrossRef CAS PubMed
.
- M. Xiao, Z. Xing, Z. Jin, C. Liu, J. Ge, J. Zhu, Y. Wang, X. Zhao and Z. Chen, Preferentially engineering FeN4 edge sites onto graphitic nanosheets for highly active and durable oxygen electrocatalysis in rechargeable Zn–air batteries, Adv. Mater., 2020, 32(49), 2004900 CrossRef CAS PubMed
.
- Z. Yang, L. Shang, X. Xiong, R. Shi, G. I. Waterhouse and T. Zhang, Hollow PtFe Alloy Nanoparticles Derived from Pt-Fe3O4 Dimers through a Silica-Protection Reduction Strategy as Efficient Oxygen Reduction Electrocatalysts, Eur. J. Chem., 2020, 26(18), 4090–4096 CrossRef CAS PubMed
.
- X. Xie, L. Shang, R. Shi, G. I. Waterhouse, J. Zhao and T. Zhang, Tubular assemblies of N-doped carbon nanotubes loaded with NiFe alloy nanoparticles as efficient bifunctional catalysts for rechargeable zinc-air batteries, Nanoscale, 2020, 12(24), 13129–13136 RSC
.
- C. Du, Y. Gao, H. Chen, P. Li, S. Zhu, J. Wang, Q. He and W. Chen, A Cu and Fe dual-atom nanozyme mimicking cytochrome c oxidase to boost the oxygen reduction reaction, J. Mater. Chem. A, 2020, 8(33), 16994–17001 RSC
.
- Z. Wu, Z. Iqbal and X. Wang, Metal-free, carbon-based catalysts for oxygen reduction reactions, Front. Chem. Sci. Eng., 2015, 9(3), 280–294 CrossRef CAS
.
- Q. Wei, X. Tong, G. Zhang, J. Qiao, Q. Gong and S. Sun, Nitrogen-doped carbon nanotube and graphene materials for oxygen reduction reactions, Catalysts, 2015, 5(3), 1574–1602 CrossRef CAS
.
- L. Chen, Z. Chen, Z. Huang, Z. Huang, Y. Wang, H. Li, H. Zhou and Y. Kuang, Influence of carbon precursors on the structure, composition, and oxygen reduction reaction performance of nitrogen-doped carbon materials, J. Phys. Chem. C, 2015, 119(52), 28757–28765 CrossRef CAS
.
- J. Yin, Y. Li, F. Lv, Q. Fan, Y.-Q. Zhao, Q. Zhang, W. Wang, F. Cheng, P. Xi and S. Guo, NiO/CoN porous nanowires as efficient bifunctional catalysts for Zn–air batteries, ACS Nano, 2017, 11(2), 2275–2283 CrossRef CAS
.
- S. Liu, X. Zhang, G. Wang, Y. Zhang and H. Zhang, High-Efficiency Co/CoxSy@S,N-Codoped Porous Carbon Electrocatalysts Fabricated from Controllable Grown Sulfur-and Nitrogen-Including Cobalt-Based MOFs for Rechargeable Zinc–Air Batteries, ACS Appl. Mater. Interfaces, 2017, 9(39), 34269–34278 CrossRef CAS PubMed
.
- J. Lyu, X. Wang, L. Liu, Y. Kim, E. K. Tanyi, H. Chi, W. Feng, L. Xu, T. Li and M. A. Noginov, High strength conductive composites with plasmonic nanoparticles aligned on aramid nanofibers, Adv. Funct. Mater., 2016, 26(46), 8435–8445 CrossRef CAS
.
- J. Li, H. Zhou, H. Zhuo, Z. Wei, G. Zhuang, X. Zhong, S. Deng, X. Li and J. Wang, Oxygen vacancies on TiO2 promoted the activity and stability of supported Pd nanoparticles for the oxygen reduction reaction, J. Mater. Chem. A, 2018, 6(5), 2264–2272 RSC
.
- J. Zhang, J. Ma, T. S. Choksi, D. Zhou, S. Han, Y.-F. Liao, H. B. Yang, D. Liu, Z. Zeng, W. Liu, X. Sun, T. Zhang and B. Liu, Strong Metal–Support Interaction Boosts Activity, Selectivity, and Stability in Electrosynthesis of H2O2, J. Am. Chem. Soc., 2022, 144(5), 2255–2263 CrossRef CAS PubMed
.
- M. Sun, H. Liu, Y. Liu, J. Qu and J. Li, Graphene-based transition metal oxide nanocomposites for the oxygen reduction reaction, Nanoscale, 2015, 7(4), 1250–1269 RSC
.
- C. Goswami, K. K. Hazarika and P. Bharali, Transition metal oxide nanocatalysts for oxygen reduction reaction, Mater. Sci. Technol., 2018, 1(2), 117–128 Search PubMed
.
- W. Chen, J. Shen, Y. Huang, X. Liu and D. Astruc, Catalyzed hydrolysis of tetrahydroxydiboron by graphene quantum dot-stabilized transition-metal nanoparticles for hydrogen evolution, ACS Sustainable Chem. Eng., 2020, 8(19), 7513–7522 CrossRef CAS
.
- X. Liu and W. Hu, Iron oxide/oxyhydroxide decorated graphene oxides for oxygen reduction reaction catalysis: a comparison study, RSC Adv., 2016, 6(35), 29848–29854 RSC
.
- D. Z. Khater, R. Amin, M. Mahmoud and K. El-Khatib, Evaluation of mixed transition metal (Co, Mn, and Cu) oxide electrocatalysts anchored on different carbon supports for robust oxygen reduction reaction in neutral media, RSC Adv., 2022, 12(4), 2207–2218 RSC
.
- K. Huang, W. Zhang, R. Devasenathipathy, Z. Yang, X. Zhang, X. Wang, D.-H. Chen, Y. Fan and W. Chen, Co nanoparticles and ZnS decorated N,S co-doped carbon nanotubes as an efficient oxygen reduction catalyst in zinc-air batteries, Int. J. Hydrogen Energy, 2021, 46(58), 30090–30100 CrossRef CAS
.
- Y. Zhang, N. Wang, N. Jia, J. Wang, J. Sun, F. Shi, Z. H. Liu and R. Jiang, A Low-Cost and Facile Method for the Preparation of Fe-N/C-Based Hybrids with Superior Catalytic Performance toward Oxygen Reduction Reaction, Adv. Mater. Interfaces, 2019, 6(8), 1900273 CrossRef
.
- L. Yang, X. Zeng, W. Wang and D. Cao, Recent progress in MOF-derived, heteroatom-doped porous carbons as highly efficient electrocatalysts for oxygen reduction reaction in fuel cells, Adv. Funct. Mater., 2018, 28(7), 1704537 CrossRef
.
- Z. Yang, Y. Wang, M. Zhu, Z. Li, W. Chen, W. Wei, T. Yuan, Y. Qu, Q. Xu and C. Zhao, Boosting oxygen reduction catalysis with Fe–N4 sites decorated porous carbons toward fuel cells, ACS Catal., 2019, 9(3), 2158–2163 CrossRef CAS
.
- L. Zou, Y.-S. Wei, C.-C. Hou, M. Wang, Y. Wang, H.-F. Wang, Z. Liu and Q. Xu, One-Step Synthesis of Ultrathin Carbon Nanoribbons from Metal–Organic Framework Nanorods for Oxygen Reduction and Zinc–Air Batteries, CCS Chem., 2022, 4(1), 194–204 CrossRef CAS
.
- H. Ye, L. Li, D. Liu, Q. Fu, F. Zhang, P. Dai, X. Gu and X. Zhao, Sustained-Release Method for the Directed Synthesis of ZIF-Derived Ultrafine Co-N-C ORR Catalysts with Embedded Co Quantum Dots, ACS Appl. Mater. Interfaces, 2020, 12(52), 57847–57858 CrossRef CAS PubMed
.
- S. N. Zhao, J. K. Li, R. Wang, J. Cai and S. Q. Zang, Electronically and Geometrically Modified Single-Atom Fe Sites by Adjacent Fe Nanoparticles for Enhanced Oxygen Reduction, Adv. Mater., 2022, 34(5), 2107291 CrossRef CAS
.
- A. Han, X. Wang, K. Tang, Z. Zhang, C. Ye, K. Kong, H. Hu, L. Zheng, P. Jiang and C. Zhao, An adjacent atomic platinum site enables single-atom iron with high oxygen reduction reaction performance, Angew. Chem., Int. Ed., 2021, 60(35), 19262–19271 CrossRef CAS PubMed
.
- I. Khan, F. Nasim, M. Choucair, S. Ullah, A. Badshah and M. Nadeem, Cobalt oxide nanoparticle embedded N-CNTs: lithium ion battery applications, RSC Adv., 2016, 6(2), 1129–1135 RSC
.
- Y. Ma, H. Wang, J. Key, V. Linkov, S. Ji, X. Mao, Q. Wang and R. Wang, Ultrafine iron oxide nanoparticles supported on N-doped carbon black as an oxygen reduction reaction catalyst, Int. J. Hydrogen Energy, 2014, 39(27), 14777–14782 CrossRef CAS
.
- K. Maiti, N. H. Kim and J. H. Lee, Strongly stabilized integrated bimetallic oxide of Fe2O3-MoO3 Nano-crystal entrapped N-doped graphene as a superior oxygen reduction reaction electrocatalyst, Chem. Eng. J., 2021, 410, 128358 CrossRef CAS
.
- C. Goswami, H. Saikia, K. Tada, S. Tanaka, P. Sudarsanam, S. K. Bhargava and P. Bharali, Bimetallic palladium–nickel nanoparticles anchored on carbon as high-performance electrocatalysts for oxygen reduction and formic acid oxidation reactions, ACS Appl. Energy Mater., 2020, 3(9), 9285–9295 CrossRef CAS
.
- L. Wei, H. E. Karahan, S. Zhai, H. Liu, X. Chen, Z. Zhou, Y. Lei, Z. Liu and Y. Chen, Amorphous bimetallic oxide–graphene hybrids as bifunctional oxygen electrocatalysts for rechargeable Zn–air batteries, Adv. Mater., 2017, 29(38), 1701410 CrossRef PubMed
.
- I. A. Khan, Y. Qian, A. Badshah, M. A. Nadeem and D. Zhao, Highly porous carbon derived from MOF-5 as a support of ORR electrocatalysts for fuel cells, ACS Appl. Mater. Interfaces, 2016, 8(27), 17268–17275 CrossRef CAS PubMed
.
- U. Martinez, S. Komini Babu, E. F. Holby, H. T. Chung, X. Yin and P. Zelenay, Progress in the development of Fe-based PGM-free electrocatalysts for the oxygen reduction reaction, Adv. Mater., 2019, 31(31), 1806545 CrossRef PubMed
.
- S. Zhao, L. Zhang, B. Johannessen, M. Saunders, C. Liu, S. Z. Yang and S. P. Jiang, Designed iron single atom catalysts for highly efficient oxygen reduction reaction in alkaline and acid media, Adv. Mater. Interfaces, 2021, 8(8), 2001788 CrossRef CAS
.
- P. Connor, J. Schuch, B. Kaiser and W. Jaegermann, The determination of electrochemical active surface area and specific capacity revisited for the system MnOx as an oxygen evolution catalyst, Z. Physiol. Chem., 2020, 234(5), 979–994 CrossRef CAS
.
- M. E. Kreider, M. B. Stevens, Y. Liu, A. M. Patel, M. J. Statt, B. M. Gibbons, A. Gallo, M. Ben-Naim, A. Mehta and R. C. Davis, Nitride or oxynitride? Elucidating the composition–activity relationships in molybdenum nitride electrocatalysts for the oxygen reduction reaction, Chem. Mater., 2020, 32(7), 2946–2960 CrossRef CAS
.
- N. Kumar, K. Naveen, M. Kumar, T. C. Nagaiah, R. Sakla, A. Ghosh, V. Siruguri, S. Sadhukhan, S. Kanungo and A. K. Paul, Multifunctionality Exploration of Ca2FeRuO6: An Efficient Trifunctional Electrocatalyst toward OER/ORR/HER and Photocatalyst for Water Splitting, ACS Appl. Energy Mater., 2021, 4(2), 1323–1334 CrossRef CAS
.
- H. Xue, T. He, J. M. Chabu, J. Liu, H. Wu, J. Zheng, M. Tan, J. Ma, R. Shen and L. Deng, Iron Single Clusters Anchored on N-Doped Porous Carbon as Superior Trace-Metal Catalysts toward Oxygen Reduction, Adv. Mater. Interfaces, 2018, 5(7), 1701345 CrossRef
.
- A. Valverde-González, L. Z. Guan, M. L. Ferrer, M. Iglesias and E. M. Maya, Iron phthalocyanine-knitted polymers as electrocatalysts for the oxygen reduction reaction, ACS Appl. Mater. Interfaces, 2020, 12(29), 32681–32688 CrossRef PubMed
.
- Y.-W. Li, W.-J. Zhang, J. Li, H.-Y. Ma, H.-M. Du, D.-C. Li, S.-N. Wang, J.-S. Zhao, J.-M. Dou and L. Xu, Fe-MOF-derived efficient ORR/OER bifunctional electrocatalyst for rechargeable zinc–air batteries, ACS Appl. Mater. Interfaces, 2020, 12(40), 44710–44719 CrossRef CAS PubMed
.
|
This journal is © The Royal Society of Chemistry 2023 |
Click here to see how this site uses Cookies. View our privacy policy here.