Engineering NiMoP nanosheets on hollow Cu3P nanotube arrays as efficient electrocatalyst for hydrogen evolution reaction†
Received
8th July 2022
, Accepted 21st September 2022
First published on 17th November 2022
Abstract
Designing nonprecious but efficient electrocatalysts for the hydrogen evolution reaction (HER) in alkaline solution still remains a significant challenge. Herein, we report the NiMoP nanosheets on hollow Cu3P nanotube arrays on the Cu foam (NiMoP@Cu3P/CF) via solution immersion, hydrothermal reactions and phosphorization. By virtue of its unique core–shell open tubular structure, NiMoP@Cu3P/CF shows large specific surface area and mesoporous, short diffusion paths, which facilitates mass transport and easy accessibility of water, resulting in a superior HER activity in 1.0 M KOH. NiMoP@Cu3P/CF only requires 81 mV and 226 mV of overpotential when the current density is 10 mA cm−2 and 100 mA cm−2 for HER in 1.0 M KOH, respectively, with a low Tafel slope (133.1 mV dec−1) and as well as superior durability. Our work provides a new idea to design a powerful electrocatalyst for HER, which will be highly expected for various practical applications.
Introduction
In the era of the depletion of fossil fuels and increase in energy demands, carbon-based coal, gas fuel, and petroleum commonly used in various industrial technologies can cause serious air pollution. There is an urgent need to discover and utilize alternative energy and sufficient energy on the earth, and design effective energy storage equipment. Sustainable and renewable energy sources (wind, hydro, solar, etc.) can be used as alternative energy sources to solve energy problems.1,2 Hydrogen (H2) is a green energy with high energy density, which has attracted much attention.3–5 Electrolysis of water is a simple and promising method for H2 production. In general, a highly efficient electrocatalyst for the hydrogen evolution reaction (HER) is needed to achieve high current density at low overpotential. At present, Pt and Ru/Ir-based precious metal materials are considered as the most effective catalysts for reducing the overpotential for HER and OER, but their high cost limits their widespread use.6,7 Therefore, the development of high-efficiency, stable, inexpensive electrocatalysts is essential for realizing cheap and efficient H2 production.
In the past few years, a lot of research has being conducted to develop dual-functional electrocatalysts to enhance the catalytic performance of HER,8,9 such as transition metal chalcogenides,10,11 oxides,12,13 phosphides,14,15 nitrides,16–18etc. Transition metal phosphide (TMP) is another potential non-noble metal catalyst similar to transition metal sulfide for HER. Experiments confirmed that the charge density is transferred from the transition metal to P in transition metal phosphides, resulting in the formation of two active sites of positively charged metal and negatively charged P, thereby enhancing the catalytic activity of the phosphide.19,20 Currently, Ni2P, CoP, FeP, WP, MoP and other TMPs have been continuously reported in the field of catalysis.21–24 Compared with oxides, hydroxides, and chalcogenide compounds, TMPs have low cost, nontoxicity, good chemical stability, and catalytic activity toward HER in electrolytes with a wide range of pH values, high electrical conductivity, and therefore often exhibit excellent catalytic activity.25,26 Compared with single-metal phosphides, bimetallic phosphides show excellent catalytic activities due to the coordination of two metals can provide bi-directional rich active centers and improved electronic conductivity. Tian et al.27 used carbon quantum dots to prepare NiMoP hollow nanoparticles and exhibited excellent electrocatalytic hydrogen evolution performance, in terms of a low overpotential of 183 mV at 10 mA cm−2, high conductivity, and remarkable long-term stability in acidic media for HER. However, the improvement of the conductivity in bimetallic phosphides is still limited, which often leads to instability of strong alkaline electrolytes and high overpotential under long-time operation. To solve this problem, the catalysts can be deposited on the conductive substrates to obtain accessibility of HER and OER relevant reactants with catalytic centers. It is also a good strategy by constructing hybrid electrocatalysts with hollow micro/nanostructures with a large number of exposed active sites and reduced diffusion length for both mass and charge transport.26,28
The electrochemical performance of electrocatalysts is strongly dependent on their morphologies and electrochemically active surface areas. Particularly, tubular Cu3P materials with both hollow and one-dimensional open structures, which provide high specific surface areas, low densities and fast diffusion of electrolyte/ionic species, have been used as effective electrocatalysts because they can better contact the electrolyte and have lower resistance along their longitudinal direction.29 Here, we have prepared a Cu3P hollow structure electrocatalyst on the copper foam through in situ reaction and Kirkendall effect,15 which has a higher specific surface area, more active sites and better chemical stability. This inspired us to explore a new type of electrocatalyst with core–shell structure by assembling NiMoP nanosheets on the surface of Cu3P. High performance electrocatalysts will be prepared by using the synergistic effect of the two components. The composite electrocatalyst is expected to have strong electron coupling and enhance the interface electron transport ability, prevent electrolyte erosion, reduce the dissolution of phosphorus, and improve the catalytic stability and durability.
In this work, Cu(OH)2 nanorod arrays were in situ grown on the surface of CF by chemical oxidation, then NiMo LDH nanosheets were directly grown on the surface of Cu(OH)2 nanotubes by hydrothermal method, and finally NiMoP@Cu3P nanotubes with hollow layered structure were prepared by reaction with sodium hypophosphite in a tube furnace. The nano-array of the core–shell structure is conducive to the full contact between the active material and the electrolyte. The nano-sheet structure of NiMoP provides a large specific surface area and is beneficial to expose more active sites and reduce the resistance. These unique structures make NiMoP@Cu3P nanoarrays have excellent HER performance in alkaline conditions.
Experimental section
Chemicals and reagents
Sodium molybdate dihydrate (Na2MoO4·2H2O), nickel(II) nitrate hexahydrate (Ni(NO3)2·6H2O), ammonium persulfate ((NH4)2S2O8), urea (CH4N2O), sodium hypophosphite hydrate (NaH2PO2·H2O) ethanol, HCl (37 wt%) and potassium hydroxide (KOH) were purchased from Sinopharm Chemical Reagent (Beijing Co., Ltd). Pt/C (20 wt%) was obtained from Shanghai Macklin Biochemical Co., Ltd. Commercial RuO2 were purchased from Aladdin reagent Co. Ltd. Nafion (5 wt%) was purchased from Dupont China Holding Co., Ltd. All reagents were of analytical grade and were utilized without further purification. Deionized water was utilized throughout the experiments. The Cu foam was received from Kunshan Jiayisheng Dianziwang Technology Co. Ltd. Before using, copper foam is pre cleaned for 10 minutes in acetone ultrasonic bath to remove oil. Subsequently, it was immersed in concentrated HCl (3 mol L−1) solution for 15 minutes to remove the surface oxide layer, then rinsed with deionized water and ethanol several times.
Characterization methods
Field emission scanning electron microscopy (FESEM, Nova NanoSEM 450) measurements with an energy dispersive spectroscopic (EDX) detector was conducted at an accelerating voltage of 15 kV. Transmission electron microscopy (TEM, JEM 2100) was operated at 200 kV. X-ray diffractometer (XRD, Philips) patterns were examined by using CuKα radiation (λ = 0.15406 nm) at a scanning rate of 5° min−1. X-ray photoelectron spectroscopy (XPS, AXIS-ULTRA DLD) spectra were obtained with an AlKα X-ray source. The synthesized nanorod array materials were scratched off from Cu foam and used for XPS. Inductively coupled plasma mass spectrometer (ICP-MS, Agilent 7700X) the inductively coupled plasma mass spectrometer dissociates the solution sample into ionized gas under the action of the plasma light source, and then measures the element content or isotope ratio by the quadrupole mass spectrometer.
Preparation of Cu(OH)2/CF electrode.
The Cu(OH)2 nanorods on Cu foams (denoted as Cu(OH)2/CF) were prepared via a simple oxidation precipitation method at the room temperature according to the previous literature.30 The cleaned Cu foam (1 × 3 cm2) was then immersed into 15 mL of an aqueous solution containing 40 mmol KOH and 2 mmol (NH4)2S2O8 for 30 min silently. Finally, the Cu(OH)2/CF was taken out from the solution, rinsed with deionized water and dried in freeze-drying for overnight, showing a light blue surface.
Preparation of NiMo LDH@Cu(OH)2/CF electrode.
Typically, 0.242 g of Na2MoO4·2H2O, 0.291 g of Ni(NO3)2 6H2O and 0.3 g CH4N2O were dissolved in 30 mL of deionized water in a 50 mL conical flask under magnetic stirring at room temperature for 15 min. Subsequently, the mixed solution was transferred into a 50 mL Teflon-lined stainless-steel autoclave, and then one piece of pre-prepared Cu(OH)2/CF was immersed into the autoclave. After that, the autoclave was standing for 2 h in 160 °C. The obtained product was washed thoroughly with methanol several times and finally freeze-dried for overnight to obtain the NiMo LDH@Cu(OH)2/CF.
By contrast, the preparation of NiMo LDH/CF electrode was similar to the above-mentioned method except for the use of bare CF instead of Cu(OH)2/CF.
Preparation of NiMoP@Cu3P/CF electrode.
NiMoP@Cu3P/CF was synthesized via a low-temperature phosphating process. The as-prepared NiMo LDH@Cu(OH)2/CF was put into a crucible and 1 g of NaH2PO2 H2O was placed frontally. Then, the boat was put into a tube furnace, which was further annealed with NaH2PO2 at 300 °C for 2 h with the heating rate of 2 °C min−1 under the inert N2. After cooling to room temperature, the NiMoP@Cu3P/CF was successfully synthesized. For comparison, The NiMoP/CF and Cu3P/CF were also fabricated using the same procedure as the NiMoP@Cu3P/CF by replacing NiMo LDH@Cu(OH)2/CF with Cu(OH)2/CF or NiMo LDH/CF.
Preparation of RuO2 and Pt/C electrode.
RuO2 and electrode was prepared by drop coating method. 4 mg of commercial RuO2 or Pt/C and 40 μL Nafion solution (5 wt%) were dispersed in 960 μL solvent (720 μL ultrapure water and 240 μL ethanol). After ultrasonic for 1 h, the dispersion solution was dried on pure Cu foam, and the loading of RuO2 or Pt/C is about 4 mg cm−2.
Electrochemical measurements.
All the electrochemical experiments were conducted in a CHI 760E electrochemical workstation (CHI instrument, China). In 1.0 M KOH aqueous solution (pH = 13.7), the as-prepared NiMoP@Cu3P/CF with a geometric area of 0.5 × 0.5 cm2 was used as the working electrode, and a graphite rod and a saturated calomel electrode (SCE) were used as the counter and reference electrodes, respectively. All samples were activated by cyclic voltammetry (CV) at 0.268–0.368 V versus RHE before electrochemical measurements. All the potentials were measured versus SCE electrode and converted to RHE according to the following equation:
ERHE = ESCE + 0.241 + 0.059 × pH |
Linear sweep voltammetry (LSV) plots were recorded in the potential range of −1.668 to −0.868 V vs. RHE for HER at a scan rate of 2 mV s−1 with 85% iR compensation.
The Tafel plots were directly derived from the corresponding LSV curves according to the Tafel equation:
η = a + b log j |
where
η was the overpotential,
j was current density, and
b refers Tafel slope.
To measure double-layer capacitance (Cdl) of the resulting electrocatalysts, the cyclic voltammetry measurement (CV) at different scan rates (20 to 100 mV s−1) was carried out in the potential range without faradaic reactions. There is a positive correlation between electrochemically active surface (ECSA) and Cdl. The higher the Cdl, the larger the electrochemically active specific surface area.31,32
Electrochemical impedance spectroscopy (EIS) analyses were researched at a potential of −0.2 V vs. RHE for HER in the frequency range of 10 Hz to 100
000 Hz with AC amplitude of 5 mV. The durability test was measured by both CV and chronopotentiometric response.
Result and and discussion
In this paper, the NiMoP@Cu3P/CF samples were prepared by solution immersion, hydrothermal and low-temperature phosphating methods. The specific preparation process is shown in Fig. 1. First, Cu(OH)2 nanorod arrays were in situ grown on the surface of CF by chemical oxidation. Then ultrathin NiMo LDH nanosheets were directly grown on the surface of Cu(OH)2 nanorods by hydrothermal method. Finally, tubular NiMoP@Cu3P materials with both hollow and one-dimensional open structures were prepared by reaction with sodium hypophosphite in a tube furnace. The color changes of the samples from orange to light blue, green, and finally to brownish black were clearly observed, as shown in Fig. S1.† The specific reaction mechanism is shown in the ESI†.
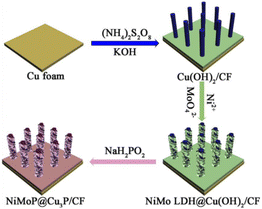 |
| Fig. 1 Schematic illustration of the synthesis process of hollow hierarchical structure of NiMoP@Cu3P nanorod arrays on copper foam substrate. | |
Crystalline structure and phase purity of the NiMoP@Cu3P/CF was analyzed using X-ray diffraction (XRD) studies (Fig. 2). The intensity of the overall XRD peak of NiMoP@Cu3P/CF is shown in Fig. S2.† XRD results show that three strong signal peaks of NiMoP@Cu3P/CF are located at 43.4° (111), 50.5° (200) and 74.2° (220), which are attributed to the 3D Cu foam (JCPDS No.04-0836).33 In addition, the sample has obvious diffraction peaks at 36.1°, 39.3°, 41.7°, 45.1°, 46.2°, 47.3°, 53.4°, 66.6° and 77.3°, corresponding to (112), (202), (211), (300), (113), (212), (104), (214) and (412) crystal planes of Cu3P (JCPDS No. 71-2261),34 indicating the exposed copper framework and Cu(OH)2 nanotubes are converted to Cu3P during the phosphating process. The diffraction peaks of the sample at 40.8°, 44.6°, 47.3° and 74.7°, correspond to the (111), (201), (210) and (400) crystal planes of Ni2P (JCPDS NO.03-0953).34 The diffraction peaks at 27.6°,31.9° and 43.2°, correspond to the (001), (100) and (101) crystal plane of MoP (JCPDS NO.24-0771).35 These results indicate that Cu3P, Ni2P and MoP were successfully prepared on the CF substrate. The diffraction peaks of Ni2P and MoP shifted slightly, indicating that there is an interaction between Ni2P and MoP and Cu3P, which affects the positions of the diffraction peaks of Ni2P and MoP.
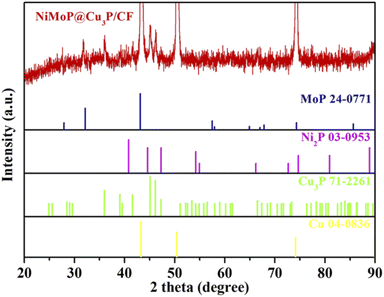 |
| Fig. 2 XRD patterns of NiMoP@Cu3P/CF. | |
The morphology and structure of the synthesized sample were characterized by SEM, and the results are shown in Fig. 3 and S3.† The SEM image of Cu(OH)2/CF shows that the Cu(OH)2 nanorod array is densely and uniformly covered on the surface of CF (Fig. S1†). The diameter of Cu(OH)2 nanorods is about 300 nm. Cu(OH)2/CF has a highly smooth surface, which can be used as a hard template for subsequent synthesis. As shown in Fig. 3a, the SEM of NiMo LDH@Cu(OH)2/CF shows that hierarchical nanotubes are uniformly arranged on the CF substrate, and the size of the nanotubes is about 1 μm. From left to right, the surface of the nanotubes grows in a porous structure, formed by staggered stacked nanosheets. This structure can increase the specific surface area of the material, thereby improving its catalytic performance. The SEM image of NiMoP@Cu3P/CF is shown in Fig. 3b. It can be seen that the tube-like structure of the NiMo LDH@Cu(OH)2/CF still exists after phosphating. The diameter of the nanorods is about 800–900 nm, but the surface shrinks due to the high temperature annealing of the nanosheet structure. The smooth surface may be attributed to the volume shrinkage of NiMo LDH nanosheets during the phosphating process. Moreover, the STEM Energy dispersive spectrum demonstrate that the NiMoP@Cu3P/CF includes Cu, Ni, Mo and P (Fig. 3c). Through ICP-MS test, the result shows that the atomic ratio of Cu, Ni and Mo is 7.14
:
2.32
:
1. The energy-dispersive spectroscopy (EDS) mapping results of the NiMoP@Cu3P/CF in Fig. 3d clearly suggest the homogeneous distribution of the elements Cu, Ni, Mo, P, and O across the entire nanosheet. The presence of oxygen is mainly due to the oxidation of the sample when exposed to air. These results prove that the core–shell structure of NiMoP@Cu3P/CF was synthesized successfully.
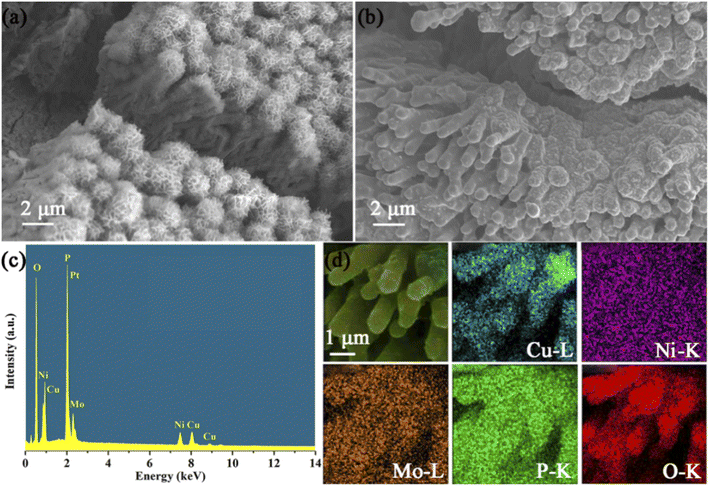 |
| Fig. 3 SEM images of NiMo LDH@Cu(OH)2/CF (a) and NiMoP@Cu3P/CF (b). Energy dispersive spectrum of NiMoP@Cu3P/CF (c). SEM and corresponding EDX elemental mapping images of NiMoP@Cu3P/CF (d). | |
The TEM images of NiMo LDH@Cu(OH)2 and NiMoP@Cu3P are shown in Fig. 4a–d and S8.† It can be seen from Fig. 4a that during the growth of NiMo LDH ultra-thin nanosheets on the surface of Cu(OH)2 nanorods, the Kirkendall effect will cause the Cu(OH)2 nanorods to form a hollow structure.36 It can be seen from the inset in Fig. 4a that the width of the NiMo LDH ultra-thin nanosheet is about 260 nm, and the diameter of the hollow Cu(OH)2 nanotube cavity is about 230 nm. Fig. 4b is a TEM image of NiMoP@Cu3P. It can be seen that the nanosheets and hollow nanotubes still exist after phosphating, but the width of the nanosheets on the nanotubes decreases to 180 nm, which is mainly due to the shrinkage of the nanosheets during phosphating. The hollow layered structure has a large specific surface area, which accelerates the transfer of substances and enhances its activity. Fig. 4c and d is a HRTEM image of NiMoP@Cu3P. The lattice fringe with a lattice spacing of 0.502 nm and 0.228 nm can be clearly observed, which corresponds to the (010) and (111) crystal plane of Ni2P, which is consistent with the XRD results.
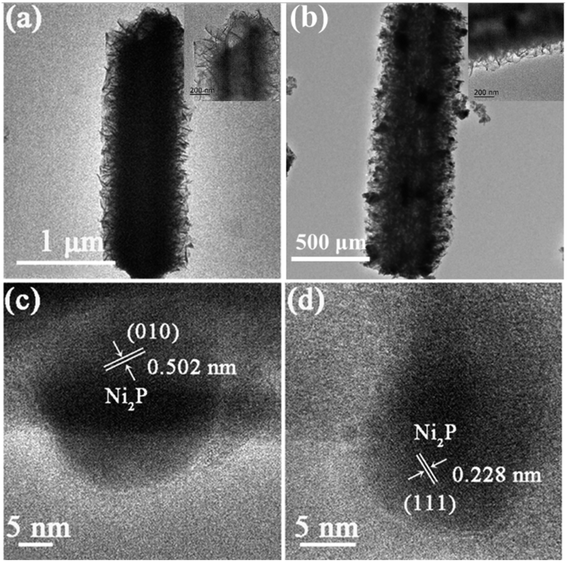 |
| Fig. 4 Low-resolution (a) TEM image of NiMo LDH@Cu(OH)2. Low-resolution (b) and high-resolution (c and d) TEM images of NiMoP@Cu3P. | |
X-ray photoelectron spectroscopy (XPS) was used to test and analyze the elemental composition and valence of NiMoP@Cu3P/CF, thereby revealing the electronic interaction between Cu3P and NiMoP (Fig. 5). The scanning spectrum of NiMoP@Cu3P/CF (Fig. 5a) confirms the presence of Cu, Ni, Mo, P, C and O elements. The presence of the O element may be caused by exposure of the sample to the air. In the Cu 2p spectrum (Fig. 5b), the binding energy peaks at 932.26 and 934.81 eV are attributed to Cu 2p2/3 of Cu+ and Cu2+, respectively, and the peaks at 952.01 and 954.95 eV are also attributed to Cu 2p1/2 of Cu+ and Cu2+. The peaks of 942.32, 944.02, 961.63 and 962.71 eV are characteristic satellite peaks of Cu2+.37,38Fig. 5c is the XPS spectrum of NiMoP@Cu3P/CF material Ni 2p. The peaks at 854.79 and 872.33 eV correspond to the Ni 2p3/2 and Ni 2p1/2 spin orbits of Ni2+, respectively, and the peaks at 856.57 and 874.18 eV correspond to the Ni 2p3/2 and Ni 2p1/2 spin orbitals of Ni3+ valence, respectively.39 In the spin orbit, the peak at 851.75 eV may be attributed to Ni+ or metallic nickel. The peaks at 860.48 and 878.54 eV are satellite peaks of Ni 2p.40Fig. 5d is the XPS spectrum of Mo 3d. It can be clearly seen that Mo 3d has four high-intensity peaks. The peaks at 230.4 and 233.5 eV are attributed to Mo4+ 3d5/2 and Mo4+ 3d3/2 of spin orbits of Mo4+, respectively. The peaks at 232.1 and 235.25 eV are assigned to the Mo6+ 3d5/2 and Mo6+ 3d3/2 of spin orbits of Mo6+, respectively. The positive shift of the Mo element may be due to the existence of the O element.39,41Fig. 5e is the XPS spectrum of P 2p. The strong peak at 132.05 eV can be attributed to phosphorus oxide (P–O), while the features at 127.45 eV and 128.26 eV can be attributed to P 2p3/2 and P 2p1/2 of metal phosphide (Mo–P).42,43 These results indicate that the electron density is transferred from Ni and Mo to Cu and P. This electron density transfer is conducive to exposing more active sites and enhancing the electron transfer between interfaces, thereby increasing the HER activity of NiMoP@Cu3P/CF.
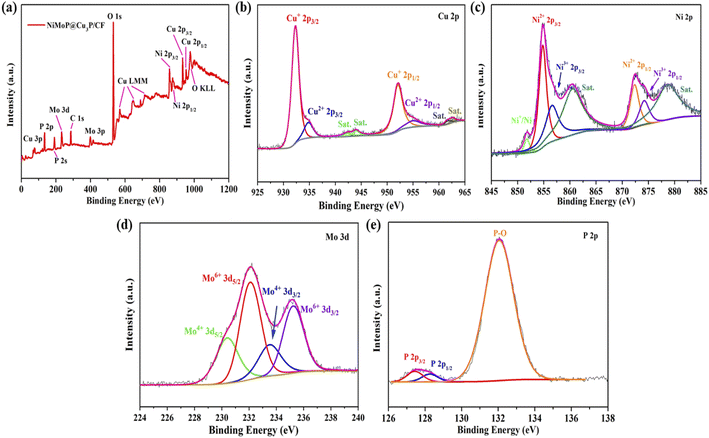 |
| Fig. 5 XPS survey spectrum of NiMoP@Cu3P/CF (a), high-resolution scans of Cu 2p (b), Ni 2p (c), Mo 3d (d), and P 2p electrons for NiMoP@Cu3P/CF (e). | |
Electrochemical activity and stability of NiMoP/CF toward HER
Linear sweep voltammetry (LSV) is a commonly used electrochemical technique to evaluate different electrocatalysts. The overpotential (ηj) that reaches a certain current density is used to evaluate the electrocatalytic activity in the standard three electrode electrochemical cell using 1.0 M KOH solution. Besides, the HER activity of the contrast materials such as Cu(OH)2/CF, Cu3P/CF, CF, NiMo LDH/CF, NiMoP/CF, NiMo LDH@Cu(OH)2/CF and commercial Pt/C (20 wt%) was also tested under the same condition, as shown in Fig. 6a. It can be observed that NiMoP@Cu3P/CF requires 81 mV and 226 mV when the current density is 10 mA cm−2 and 100 mA cm−2, which is lower than Cu3P/CF (η10 = 176 mV) and NiMoP/CF (η10 = 156 mV). This result shows that the unique structure is beneficial to the contact of the active site with the electrolyte and the escape of gas. At the same time, the synergistic effect of Cu3P and NiMoP is also the reason for enhancing the HER activity of the composite material. The overpotential of NiMoP@Cu3P/CF at 10 mA cm−2 is also lower than that of NiMo LDH@Cu(OH)2/CF (η10 = 111 mV), indicating that the phosphide has better hydrogen evolution reaction activity than the precursor hydroxide. Some recent studies similar to NiMoP@Cu3P/CF are listed in Table S1.† By comparing the performance of the catalysts, such as NiCu–P (η10 = 175 mV), MoP@NCF (η10 = 129.5 mV), Cu3P/N–C (η10 = 211.1 mV),44 Cu3P@NPC-CF (η10 = 135.5 mV),45 C@NiCoP (η10 = 46.3 mV),46 MoS2-MoP/FPC (η10 = 144 mV),47 JLNU-COFs (η10 = 136 mV),48etc. it was found that NiMoP@Cu3P/CF showed excellent HER activity in alkaline electrolyte.
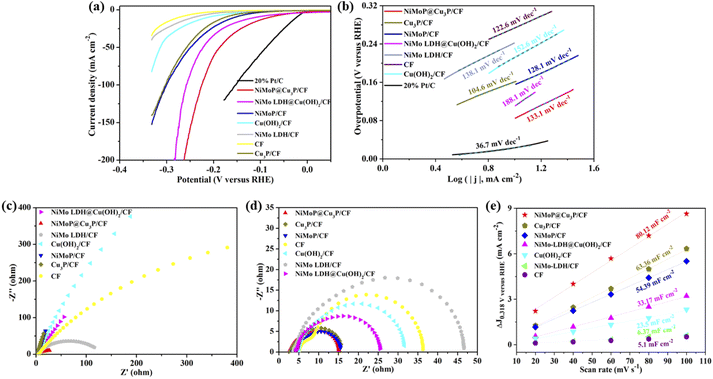 |
| Fig. 6 (a) Polarization curves of the samples for HER. Tafel plots derived from the polarization curves (b), EIS Nyquist plots of different electrodes at open-circuit potentials (c) and overpotential at −200 mV versus RHE (d) in 1.0 M KOH. Different current densities plotted against the scan rate for the determination of the double-layer capacitance (Cdl) (e). | |
In order to further understand the electrocatalytic HER kinetics of the prepared materials, the Tafel slope was studied, as shown in Fig. 6b It can be seen that the Tafel slope value of NiMoP@Cu3P/CF is 133.1 mV dec−1, which is lower than the Tafel slope of NiMo LDH@Cu(OH)2/CF (188.1 mV dec−1). The lower Tafel slope value of NiMoP@Cu3P/CF catalyst indicates that the Volmer–Heyrovsky reaction pathway is operative with the superior HER kinetics of desorption of hydrogen (Volmer step) and rapid increase of catalytic current towards the HER catalytic activity.
Electrochemical impedance spectroscopy (EIS) is utilized to probe the resistance and kinetics of electrocatalytic reaction that occurs at the interface between electrode and electrolyte (Fig. 6c and d). In the Nyquist plots measured under open-circuit potential (Fig. 6c), the solution resistance (Rs) of all synthetic materials is very small (<5 Ω), indicating that the rod-shaped structure is in good contact with the CF substrate, thereby reducing electrical resistance. NiMoP@Cu3P/CF electrode exhibits the outstanding HER performance with small charge transfer resistance (Rct) (12.1 Ω) at the overpotential at −200 mV versus RHE (Fig. 6d), which is lower than other samples. The results show that the electron transfer between nanosheet structure NiMoP and hollow structure Cu3P reduces the Rct and improves the conductivity of the composite.
To further estimate the electrochemical activity surface area (ECSA) of CF, NiMo LDH/CF, Cu(OH)2/CF, NiMo LDH@Cu(OH)2/CF, NiMoP/CF, Cu3P/CF and NiMoP@Cu3P/CF, electrochemical double-layer capacitance (Cdl) was measured via cyclic voltammetry (CV) at various scan rates in the non-Faraday region (Fig. 6e). Fig. S4a–h† shows the CV curves of CF, NiMo LDH/CF, Cu(OH)2/CF, NiMo LDH@Cu(OH)2/CF, NiMoP/CF, Cu3P/CF and NiMoP@Cu3P/CF in the voltage range of 0.268–0.368 V versus RHE at different scanning speeds. It can be observed that NiMoP@Cu3P/CF has the largest closed curve at each scanning speed, indicating that it has the largest electric double layer capacitance. Fig. 6e depicts the CV curves of various electrodes at scan rates of 20, 40, 60, 80, and 100 mV s−1. After calculation, the Cdl value of NiMoP@Cu3P/CF is 40.06 mF cm−2, which is significantly higher than CF (2.05 mF cm−2), NiMo LDH/CF (3.19 mF cm−2), Cu(OH)2/CF (11.75 mF cm−2), NiMo LDH@Cu(OH)2/CF (16.59 mF cm−2), NiMoP/CF (27.2 mF cm−2) and Cu3P/CF (31.68 mF cm−2). The electrochemically active specific surface area of the material is positively correlated with the electric double layer capacitance. The higher the electric double layer capacitance corresponds to the larger the electrochemically active specific surface area. The large electrochemically active specific surface area of NiMoP@Cu3P/CF indicates that it has more efficient active sites, which can be attributed to its unique hollow composite structure and electronic effects. Therefore, these parameters such as hollow composite structure, low charge transfer resistance, high active surface area and synergistic effects are all conducive to the progress of HER.
From the perspective of practical application, stability or durability is one of the important properties of the catalyst, and it is the ability of the catalyst to maintain the original activity for a long period of time. The stability of the catalyst can be evaluated by recording the change in cathode current density under the applied overpotential, or by measuring the continuous CV curve. We have also tested the HER stability of NiMoP@Cu3P/CF by continuous cyclic voltammetry scan between −0.2 and 0.2 V vs. RHE at a 100 mV s−1 scan rate. Fig. 7a is the polarization curve after the first and 1000 continuous CV scanning experiments. It can be seen that the hydrogen evolution performance of NiMoP@Cu3P/CF has basically not changed after 1000 continuous CV scanning. Besides, the electrocatalytic durability of NiMoP@Cu3P/CF during HER was further examined in 1.0 M KOH by the chronopotentiometry method at the current density of 10 mA cm−2 for 12 h under alkaline conditions. As shown in Fig. 7b, the overpotential still demonstrates a surprisingly stable HER behavior with a nearly unchanged overpotential for 12 h of continuous electrolysis, confirming the excellent durability of NiMoP@Cu3P/CF. To further investigate the possible morphological or composition changes after long-term HER, we performed XRD (Fig. S5†), SEM (Fig. 7c), and XPS (Fig. S6†) analyses on the post-HER NiMoP@Cu3P/CF. The XRD patterns in Fig. S5† show that the bulk phase of NiMoP@Cu3P/CF remains unchanged after electrolysis. As observed by SEM, the morphology of NiMoP@Cu3P/CF is also well-maintained. We noted that the Cu 2p, Ni 2p, Mo 3d, and P 2p spectra of NiMoP@Cu3P after electrolysis are consistent with the fresh ones, and there is no shift in binding energy. All of the studies shown above confirm that our NiMoP@Cu3P heterostructure is a highly efficient and stable electrocatalyst toward the HER.
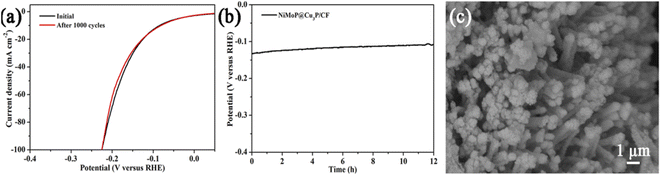 |
| Fig. 7 The HER polarization curves (a) of NiMoP@Cu3P/CF before and after 1000 cycles of voltammetry between −0.2 and 0.2 V versus RHE. Chronopotentiometry curves (b) and SEM image (c) after testing of NiMoP@Cu3P/CF in 1.0 M KOH solution at a constant current density of 10 mA cm−2. | |
Conclusion
In conclusion, the tuber and core–shell structure of NiMoP@Cu3P/CF was successfully prepared by the three-step method of solution immersion, hydrothermal synthesis and low-temperature phosphating. The experimental results confirmed that Cu3P and NiMoP were successfully supported on the copper foam substrate. XPS spectroscopy strongly reveals the electron transfer effect between Cu3P and NiMoP, which reduces the charge transfer resistance and enhances the catalytic activity. When the current density is 10 mA cm−2, the overpotential of the NiMoP@Cu3P/CF electrode is only 81 mV, and the Tafel slope is 133.1 mV dec−1. The orderly outward arrangement of the NiMoP@Cu3P/CF promotes the escape of the generated hydrogen bubbles. The present work not only constructs a solid non-noble metal electrocatalyst for HER, but also provides a new selection of HER electrocatalyst, which is of significance in both theory and practical application.
Conflicts of interest
There are no conflicts to declare.
Acknowledgements
This research was supported by the National Natural Science Foundation of China (22078251; 51808414), the research project of Hubei Provincial Department of Education (D20191504), as well as the Graduate Innovative Fund of Wuhan Institute of Technology (CX2021012; CX2020006).
References
- N. Cai, J. Fu and V. Chan,
et al., MnCo2O4@ nitrogen-doped carbon nanofiber composites with meso-microporous structure for high-performance symmetric supercapacitors, J. Alloys Compd., 2019, 782, 251–262 CrossRef CAS.
- M. Wang, P. Li and F. Yu, Hierarchical porous carbon foam-based phase change composite with enhanced loading capacity and thermal conductivity for efficient thermal energy storage, Renewable Energy, 2021, 172, 599–605 CrossRef CAS.
- X. F. Lu, S. L. Zhang, W. L. Sim, S. Gao and X. W. Lou (David), Phosphorized CoNi2S4 Yolk-Shell Spheres for Highly Efficient Hydrogen Production via Water and Urea Electrolysis, Angew. Chem., Int. Ed., 2021, 60(42), 22885–22891 CrossRef CAS PubMed.
- J. Wang, S. Qi and X. Song,
et al., First-principles design of highly-efficient earth-abundant electrocatalysts for hydrogen evolution reaction: TiF3 and its analogs, Appl. Surf. Sci., 2019, 495, 143623 CrossRef CAS.
- D. Kong, J. Hu and Z. Chen,
et al., Ti-Gradient doping to stabilize layered surface structure for high performance high-Ni oxide cathode of Li-ion battery, Adv. Energy Mater., 2019, 9(41), 1901756 CrossRef CAS.
- P. Zhou, G. Zhai and X. Lv,
et al., Boosting the electrocatalytic HER performance of Ni3N-V2O3via the interface coupling effect, Appl. Catal., B, 2021, 283, 119590 CrossRef CAS.
- S. L. Zhang, X. F. Lu, Z.-P. Wu, D. Luan and X. W. Lou (David), Engineering Platinum–Cobalt Nano-Alloys in Porous Nitrogen-Doped Carbon Nanotubes for Highly Efficient Electrocatalytic Hydrogen Evolution, Angew. Chem., Int. Ed., 2021, 60(35), 19068–19073 CrossRef CAS PubMed.
- Z. X. Cai, Z. L. Wang and Y. J. Xia,
et al., Tailored Catalytic Nanoframes from Metal–Organic Frameworks by Anisotropic Surface Modification and Etching for the Hydrogen Evolution Reaction, Angew. Chem., Int. Ed., 2021, 60(9), 4747–4755 CrossRef CAS PubMed.
- Y. Men, P. Li and F. Yang,
et al., Nitrogen-doped CoP as robust electrocatalyst for high-efficiency pH-universal hydrogen evolution reaction, Appl. Catal., B, 2019, 253, 21–27 CrossRef CAS.
- W. K. Jo, S. Moru and S. Tonda, Cobalt-coordinated sulfur-doped graphitic carbon nitride on reduced graphene oxide: an efficient metal-(N, S)-C-class bifunctional electrocatalyst for overall water splitting in alkaline media, ACS Sustainable Chem. Eng., 2019, 7(18), 15373–15384 CrossRef CAS.
- H. Sun, Y. Zhang and S. Li,
et al., Multifunctional core–shell zwitterionic nanoparticles to build robust, stable antifouling membranes via magnetic-controlled surface segregation, ACS Appl. Mater. Interfaces, 2019, 11(38), 35501–35508 CrossRef CAS.
- J. Du, C. Li and Q. Tang, Oxygen vacancies enriched Co3O4 nanoflowers with single layer porous structures for water splitting, Electrochim. Acta, 2020, 331, 135456 CrossRef CAS.
- L. Li, T. Zhang and J. Yan,
et al., P doped MoO3−x nanosheets as efficient and stable electrocatalysts for hydrogen evolution, Small, 2017, 13(25), 1700441 CrossRef.
- J. Chang, Q. Lv and G. Li,
et al., Core–shell structured Ni12P5/Ni3 (PO4)2 hollow spheres as difunctional and efficient electrocatalysts for overall water electrolysis, Appl. Catal., B, 2017, 204, 486–496 CrossRef CAS.
- K. He, T. Tadesse Tsega and X. Liu,
et al., Utilizing the Space-Charge Region of the FeNi-LDH/CoP p–n Junction to Promote Performance in Oxygen Evolution Electrocatalysis, Angew. Chem., Int. Ed., 2019, 58(34), 11903–11909 CrossRef CAS PubMed.
- X. Gao, Y. Zhou and S. Liu,
et al., Single cobalt atom anchored on N-doped graphyne for boosting the overall water splitting, Appl. Surf. Sci., 2020, 502, 144155 CrossRef CAS.
- Y. Ha, L. Shi and X. Yan,
et al., Multifunctional electrocatalysis on a porous N-doped NiCo2O4@ C nanonetwork, ACS Appl. Mater. Interfaces, 2019, 11(49), 45546–45553 CrossRef CAS.
- D. Chen, J. Zhu and X. Mu,
et al., Nitrogen-Doped carbon coupled FeNi3 intermetallic compound as advanced bifunctional electrocatalyst for OER, ORR and zn-air batteries, Appl. Catal., B, 2020, 268, 118729 CrossRef CAS.
- B. Chang, J. Yang and Y. Shao,
et al., Bimetallic NiMoN nanowires with a preferential reactive facet: an ultraefficient bifunctional electrocatalyst for overall water splitting, ChemSusChem, 2018, 11(18), 3198–3207 CrossRef CAS PubMed.
- C. Wan, Y. N. Regmi and B. M. Leonard, Multiple phases of molybdenum carbide as electrocatalysts for the hydrogen evolution reaction, Angew. Chem., 2014, 126(25), 6525–6528 CrossRef.
- C. Zhang, Z. Pu and I. S. Amiinu,
et al., Co2P quantum dot embedded N, P dual-doped carbon self-supported electrodes with flexible and binder-free properties for efficient hydrogen evolution reactions, Nanoscale, 2018, 10(6), 2902–2907 RSC.
- Y. Zeng, Y. Wang and G. Huang,
et al., Porous CoP nanosheets converted from layered double hydroxides with superior electrochemical activity for hydrogen evolution reactions at wide pH ranges, Chem. Commun., 2018, 54(12), 1465–1468 RSC.
- C. Lin, Z. Gao and J. Yang,
et al., Porous superstructures constructed from ultrafine FeP nanoparticles for highly active and exceptionally stable hydrogen evolution reaction, J. Mater. Chem. A, 2018, 6(15), 6387–6392 RSC.
- H. Lei, M. Chen and Z. Liang,
et al., Ni2P hollow microspheres for electrocatalytic oxygen evolution and reduction reactions, Catal. Sci. Technol., 2018, 8(9), 2289–2293 RSC.
- J. Yu, G. Cheng and W. Luo, Hierarchical NiFeP microflowers directly grown on Ni foam for efficient electrocatalytic oxygen evolution, J. Mater. Chem. A, 2017, 5(22), 11229–11235 RSC.
- X. F. Lu, L. Yu and X. W. Lou (David), Highly Crystalline Ni-Doped FeP/Carbon Hollow Nanorods as All-PH Efficient and Durable Hydrogen Evolving Electrocatalysts, Sci. Adv., 2019, 5(2), eaav6009 CrossRef CAS PubMed.
- L. Tian, G. Qiu and Y. Shen,
et al., Carbon quantum dots modulated NiMoP hollow nanopetals as efficient electrocatalysts for hydrogen evolution, Ind. Eng. Chem. Res., 2019, 58(31), 14098–14105 CrossRef CAS.
- H. Liu, Z.-S. Wu, L. Huang, B.-W. Zhang, L.-C. Yin, C.-Y. Xu and L. Zhen, Mechanistic Insights into Interfaces and Nitrogen Vacancies in Cobalt Hydroxide/Tungsten Nitride Catalysts to Enhance Alkaline Hydrogen Evolution, J. Mater. Chem. A, 2021, 9(18), 11323–11330 RSC.
- C. C. Hou, Q. Q. Chen and C. J. Wang,
et al., Self-supported cedarlike semimetallic Cu3P nanoarrays as a 3D high-performance Janus electrode for both oxygen and hydrogen evolution under basic conditions, ACS Appl. Mater. Interfaces, 2016, 8(35), 23037–23048 CrossRef CAS.
- J. Tian, Q. Liu and N. Cheng,
et al., Self-supported Cu3P nanowire arrays as an integrated high-performance three-dimensional cathode for generating hydrogen from water, Angew. Chem., 2014, 126(36), 9731–9735 CrossRef.
- W. Zhang, X. Chen and J. Zhang,
et al., Exposure of active edge structure for electrochemical H2 evolution from VS2/MWCNTs hybrid catalysts, Int. J. Hydrogen Energy, 2018, 43(51), 22949–22954 CrossRef CAS.
- H. Fan, W. Chen and G. Chen,
et al., Plasma-heteroatom-doped Ni–V–Fe trimetallic phospho-nitride as high-performance bifunctional electrocatalyst, Appl. Catal., B, 2020, 268, 118440 CrossRef CAS.
- J. Huang, H. Li and Y. Zhu,
et al., Sculpturing metal foams toward bifunctional 3D copper oxide nanowire arrays for pseudo-capacitance and enzyme-free hydrogen peroxide detection, J. Mater. Chem. A, 2015, 3(16), 8734–8741 RSC.
- X. Ma, Y. Chang and Z. Zhang,
et al., Forest-like NiCoP@ Cu3P supported on copper foam as a bifunctional catalyst for efficient water splitting, J. Mater. Chem. A, 2018, 6(5), 2100–2106 RSC.
- K. Liang, S. Pakhira and Z. Yang,
et al., S-doped MoP nanoporous layer toward high-efficiency hydrogen evolution in pH-universal electrolyte, ACS Catal., 2018, 9(1), 651–659 CrossRef.
- N. Yu, W. Cao and M. Huttula,
et al., Fabrication of FeNi hydroxides double-shell nanotube arrays with enhanced performance for oxygen evolution reaction, Appl. Catal., B, 2020, 261, 118193 CrossRef CAS.
- C. Zhou, H. Li and J. Lin,
et al., Matchstick-like Cu2S@ CuxO nanowire film: transition of superhydrophilicity to superhydrophobicity, J. Phys. Chem. C, 2017, 121(36), 19716–19726 CrossRef CAS.
- R. Wang, X. Y. Dong and J. Du,
et al., MOF-derived bifunctional Cu3P nanoparticles coated by a N, P-codoped carbon shell for hydrogen evolution and oxygen reduction, Adv. Mater., 2018, 30(6), 1703711 CrossRef.
- J. Balamurugan, C. Li and V. Aravindan,
et al., Hierarchical Ni-Mo-S and Ni-Fe-S Nanosheets with Ultrahigh Energy Density for Flexible All Solid-State Supercapacitors, Adv. Funct. Mater., 2018, 28(35), 1803287 CrossRef.
- J. Wang, C. Chen and N. Cai,
et al., High topological tri-metal phosphide of CoP@ FeNiP toward enhanced activities in oxygen evolution reaction, Nanoscale, 2021, 13(2), 1354–1363 RSC.
- B. Zhang, Y. Xue and A. Jiang,
et al., Ionic liquid as reaction medium for synthesis of hierarchically structured one-dimensional MoO2 for efficient hydrogen evolution, ACS Appl. Mater. Interfaces, 2017, 9(8), 7217–7223 CrossRef CAS.
- H. Gu, W. Fan and T. Liu, Phosphorus-doped NiCo2S4 nanocrystals grown on electrospun carbon nanofibers as ultra-efficient electrocatalysts for the hydrogen evolution reaction, Nanoscale Horiz., 2017, 2(5), 277–283 RSC.
- X. Xie, R. Yu and N. Xue,
et al., P doped molybdenum dioxide on Mo foil with high electrocatalytic activity for the hydrogen evolution reaction, J. Mater. Chem. A, 2016, 4(5), 1647–1652 RSC.
- H. Kim, Y. Lee, D. Song, Y. Kwon, E.-J. Kim and E. Cho, Cu3P/PAN Derived N-Doped Carbon Catalyst with Non-Toxic Synthesis for Alkaline Hydrogen Evolution Reaction, Sustainable Energy Fuels, 2020, 4(10), 5247–5253 RSC.
- E. Jiang, J. Jiang, G. Huang, Z. Pan, X. Chen, G. Wang, S. Ma, J. Zhu and P. K. Shen, Porous Nanosheets of Cu3P@N,P Co-Doped Carbon Hosted on Copper Foam as an Efficient and Ultrastable PH-Universal Hydrogen Evolution Electrocatalyst, Sustainable Energy Fuels, 2021, 5(9), 2451–2457 RSC.
- J. Zhou, Y. Dou, T. He, X.-J. Kong, L.-H. Xie and J.-R. Li, Encapsulation of Bimetallic Phosphides into Graphitized Carbon for PH-Universal Hydrogen Evolution Reaction, J. Energy Chem., 2021, 63, 253–261 CrossRef CAS.
- Q. Zhou, J. Feng, X. Peng, L. Zhong and R. Sun, Porous Carbon Coupled with an Interlaced MoP–MoS2 Heterojunction Hybrid for Efficient Hydrogen Evolution Reaction, J. Energy Chem., 2020, 45, 45–51 CrossRef.
- Y. Ma, Y. Fu, W. Jiang, Y. Wu, C. Liu, G. Che and Q. Fang, Excellent Electrocatalytic Performance of Metal-Free Thiophene–Sulfur Covalent Organic Frameworks for Hydrogen Evolution in Alkaline Medium, J. Mater. Chem. A, 2022, 10(18), 10092–10097 RSC.
Footnotes |
† Electronic supplementary information (ESI) available. See https://doi.org/10.1039/d2se00942k |
‡ These authors contributed equally to this work and should be considered co-first authors. |
|
This journal is © The Royal Society of Chemistry 2023 |
Click here to see how this site uses Cookies. View our privacy policy here.