DOI:
10.1039/D3SD00214D
(Critical Review)
Sens. Diagn., 2023,
2, 1360-1375
Advancing healthcare applications: wearable sensors utilizing metal–organic frameworks
Received
11th August 2023
, Accepted 8th September 2023
First published on 21st September 2023
Abstract
Recent advancements in wearable sensor technologies have sparked a revolution in healthcare, enabling real-time monitoring and diagnostics. Metal–organic frameworks (MOFs) have emerged as a promising class of materials in developing wearable sensors due to their unique properties, including high surface area, tunable porosity, and exceptional adsorption capacity. This review overviews the state-of-the-art MOF-based wearable sensors for healthcare applications. It explores their fabrication methods, sensing mechanisms, and applications in various medical fields, such as disease detection, physiological monitoring, and drug delivery. Additionally, we discuss the challenges and prospects of integrating MOFs into wearable devices to enhance their performance and utility in the healthcare domain.
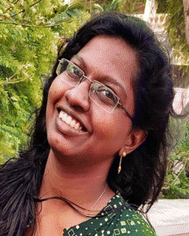 P. N. Blessy Rebecca | P. N. Blessy Rebecca is pursuing her PhD at the Department of Physics and Nanotechnology, SRM Institute of Science and Technology, located in Kattankulathur, Chennai. Prior to this, she earned her Master's degree in Nanoscience and Nanotechnology from the National Centre for Nanoscience and Nanotechnology, University of Madras. Her research is primarily on advancing graphene-based nanocomposites, with a specific focus on their potential applications in the energy and healthcare fields. |
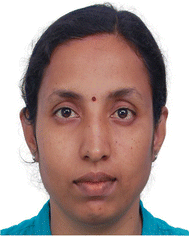 D. Durgalakshmi | D. Durgalakshmi obtained her PhD in Nanoscience and Nanotechnology from the National Centre for Nanoscience and Nanotechnology, University of Madras, Chennai. She is an Assistant Professor at the Department of Medical Physics, Anna University, Chennai. Her research interests focus on biomass-derived graphene and its applications in biosensors and bone and dental regeneration. |
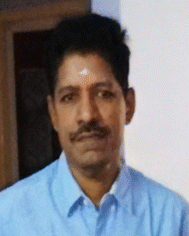 S. Balakumar | S. Balakumar is a professor and the director of the National Centre for Nanoscience and Nanotechnology at the University of Madras, Chennai, India. He obtained his PhD from Anna University and gained post-doctoral experience at the Chinese University of Hong Kong and the National University of Singapore. His primary research focuses on multifunctional nano-materials, exploring their applications in the energy, environmental, and healthcare sectors. |
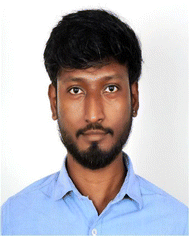 R. Ajay Rakkesh | R. Ajay Rakkesh obtained his PhD in Nanoscience and Nanotechnology from the National Centre for Nanosciences and Nanotechnology, University of Madras, Chennai. Presently, he serves as an Assistant Professor at the Department of Physics and Nanotechnology, SRM Institute of Science and Technology, Kattankulathur, Chennai. His research focuses on graphene-based nanoarchitectonics for applications in the energy and healthcare domains. |
Introduction
Wearable sensors have witnessed tremendous growth and popularity in healthcare due to their potential to revolutionize patient monitoring and personalized medicine.1–3 Metal–organic frameworks (MOFs) are hybrid materials with intriguing properties that make them suitable for sensor applications.4–8 This review focuses on recent developments in MOF-based wearable sensors for healthcare, aiming to explore their potential to address critical medical challenges.
Metal–organic frameworks (MOFs) have gained significant attention in wearable sensor fabrication due to their exceptional properties and versatile applications.9–12 MOFs are hybrid materials of metal ions coordinated with organic linkers, resulting in a three-dimensional porous structure. This unique architecture gives MOFs an incredibly high surface area, which can be precisely tailored by varying the metal ions and organic linkers used in their synthesis.13–16 By tuning the parameters, two-dimensional metal–organic frameworks have recently attracted interest in various fields including wearable devices. The favorable properties of these two-dimensional materials such as large surface area and tunable properties have intrigued the researchers in the field of wearable sensors.17–20
One of the primary reasons why MOFs appeal to wearable sensors is their tunable porosity, allowing them to adsorb and desorb target molecules selectively. This property makes MOFs well-suited for sensing various analytes, including gases, liquids, and biomolecules.21–24 MOFs offer high sensitivity and selectivity, making them excellent candidates for detecting even trace amounts of specific substances.
In the context of wearable sensors, MOFs provide several advantages. First and foremost, their integration into wearable substrates is relatively straightforward. MOFs can be synthesized on flexible materials or coated onto wearable devices, allowing lightweight and unobtrusive sensors to be developed.25–28 This feature is crucial in healthcare applications, where patients prefer non-invasive and comfortable monitoring devices.
The fabrication methods for MOF-based wearable sensors vary depending on the specific application and desired properties. Standard synthesis techniques include solvothermal, hydrothermal, and microwave-assisted methods.29–31 These methods enable the controlled growth of MOFs, ensuring uniformity and stability in the final sensor device.
Furthermore, researchers have explored different approaches to functionalize MOFs for specific sensing applications. By modifying the surface chemistry of MOFs or introducing functional groups, sensor selectivity can be fine-tuned to target particular analytes or biomarkers. For instance, MOFs can be engineered to selectively capture glucose molecules in diabetes monitoring or detect volatile organic compounds indicative of certain diseases.32–36 The exceptional porosity of MOFs also allows for efficient drug loading and release, making them promising candidates for wearable drug delivery systems.37–39 MOFs can serve as drug carriers, delivering therapeutics in a controlled manner to the wearer, thus improving treatment efficacy and reducing side effects.
Despite the numerous advantages, there are some challenges associated with MOF-based wearable sensor fabrication. One primary concern is the stability of MOFs under real-world conditions. MOFs can be sensitive to moisture, temperature fluctuations, and chemical environments, affecting long-term performance. Researchers are actively working on strategies to improve MOF stability and develop protective coatings to enhance durability. Another challenge lies in ensuring the biocompatibility of MOFs when used for medical applications. While many MOFs are safe, their potential toxicity or immune response must be thoroughly investigated before deploying them in wearable healthcare devices.40–42
Moreover, MOFs have emerged as a promising class of materials for wearable sensor fabrication, offering unique properties such as tunable porosity, high surface area, and exceptional sensing capabilities. Their integration into wearable substrates allows for the development of unobtrusive and sensitive sensors for various healthcare applications, including disease detection, physiological monitoring, and drug delivery.43–47 Despite some challenges, ongoing research in MOFs holds great promise for revolutionizing healthcare by enabling personalized, real-time monitoring and diagnostics with the help of wearable sensors.
MOFs in wearable sensor fabrication
Metal–organic frameworks (MOFs) have gained significant attention recently due to their unique structural properties and vast applications, including wearable sensors. MOFs are a class of highly ordered, porous materials constructed from metal ions (or clusters) and organic ligands. Their tunable structures and surface functionalities make them ideal candidates for sensing various analytes in wearable devices.48–52 This article delves into the synthesis and fabrication methods of MOFs for wearable sensors, focusing on solvothermal, hydrothermal, and microwave-assisted techniques and strategies to integrate MOFs onto flexible and wearable substrates.
Synthesis methods of MOFs for wearable sensors
Solvothermal method
The solvothermal method involves the reaction of metal salts and organic ligands in a solvent under elevated temperature and pressure conditions. This process allows for precise MOF size, morphology, and crystallinity control. A schematic illustration of the solvothermal method is shown in Fig. 1a. Solvothermal synthesis provides a high yield of MOFs with well-defined structures, making it a popular choice for wearable sensor applications. The reaction parameters such as temperature, pressure, reaction time, and choice of solvent can influence the final properties of the MOF.53–59
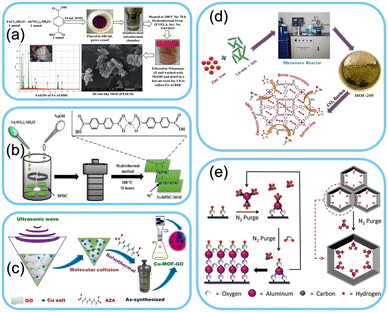 |
| Fig. 1 Common methods involved in the synthesis of MOFs: a) Solvothermal method. Reproduced from ref. 57 with permission from The Royal Society of Chemistry, copyright 2022. b) Hydrothermal method. Reproduced from ref. 65 with permission from MDPI, copyright 2022. c) Sonochemical method. Reproduced from ref. 72 with permission from American Chemical Society, copyright 2022. d) Microwave-assisted method. Reproduced from ref. 67 with permission from American Chemical Society, copyright 2016. e) Vapor phase synthesis method. Reproduced from ref. 78 with permission from American Chemical Society, copyright 2013. | |
Hydrothermal method
Hydrothermal synthesis is similar to the solvothermal method but operates at lower temperatures and pressures. The process occurs in an aqueous environment, where the metal salts and ligands react under controlled hydrothermal conditions. This technique is advantageous for producing MOFs on a larger scale and can be easily scaled up for the industrial production of wearable sensors. An illustration of the hydrothermal method is shown in Fig. 1b. Hydrothermal synthesis also allows for incorporating various functional groups into the MOF structure, enhancing the sensor's selectivity and sensitivity.60–65
Microwave-assisted method
Microwave-assisted synthesis is a relatively newer technique that offers rapid and efficient MOF formation. It involves exposing the reaction mixture to microwave irradiation, significantly accelerating the chemical reaction and reducing the synthesis time. This method provides better nucleation and crystal growth control, resulting in highly crystalline MOFs with improved sensor performance. A schematic representation of microwave-assisted synthesis is shown in Fig. 1d. Microwave-assisted synthesis is particularly suitable for the on-demand fabrication of wearable sensors, as it reduces production time and energy consumption.66–70
Sonochemical method
The sonochemical method also called ultrasound-assisted synthesis has advantages over conventional methods. The synthesis relies on the ultrasound-induced cavitation to induce chemical reactions. The process involves quick dispersion of the solutes and increases the reaction speed improving the efficiency and shortening the synthesis time. The method produces uniform crystals with size comparatively smaller than the conventional methods like solvothermal and hydrothermal. An illustration representing the process of sonochemical-based synthesis of MOFs is shown in Fig. 1c.71–77
Vapor phase synthesis method
The vapor phase synthesis method involves the formation of metal–organic frameworks through the use of less solvents or solventless synthesis. It is more suitable for developing thin films and more favored in industrial device fabrication. The process involves the deposition of MOFs through vaporized metal precursors and linkers on the substrate. The growth of the films depends on the substrate orientation. An illustration of MOF synthesis through the vapor phase method is shown in Fig. 1e. The process is favourable for preparing thin films for wearable devices at an industrial level. Some types of the vapor phase synthesis include chemical vapor deposition, atomic layer deposition and pulsed laser ablation (Table 1).78–83
Table 1 Advantages and disadvantages of synthesis methods of MOFs
Synthesis method |
Advantages |
Disadvantages |
Solvothermal method |
Controlled nucleation growth |
Long reaction time |
High crystallinity |
Large amounts of solvent are required |
Wide temperature range |
Hydrothermal method |
Low cost |
Long reaction time |
Large-scale preparation |
High energy consumption |
Controlled nucleation and growth |
Microwave-assisted method |
Short reaction time |
Reaction solvent requirements are limited |
Simple and energy efficient |
High purity |
Isolation of single crystals is difficult |
Small and uniform particles |
Sonochemical method |
Short reaction process |
Ultrasound waves hinder formation of large crystals |
Homogeneous particle size and morphology |
Vapor phase synthesis method |
Use less solvents |
Substrate dependent nucleation and growth |
Large-scale synthesis |
Suitable for thin films |
Integration of MOFs onto flexible and wearable substrates
Inkjet printing
Inkjet printing is a popular technique for integrating MOFs onto flexible substrates.84–86 The MOF ink, containing dispersed MOF particles in a solvent, is ejected through a nozzle and deposited onto the substrate in a controlled pattern. The inkjet printing process is shown in Fig. 2b. This method allows for precise deposition, creating customized sensor arrays with different MOFs for selective sensing of multiple analytes. Inkjet printing is cost-effective, scalable, and compatible with many substrates, making it ideal for wearable sensor fabrication.87–91
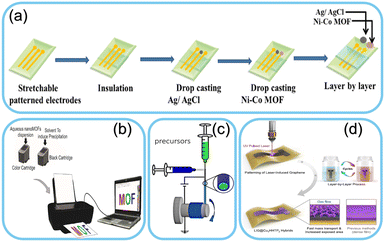 |
| Fig. 2 Schematics of different fabrication technologies involved in wearable sensors. a) Drop casting method. Reproduced from ref. 97 with permission from The Royal Society of Chemistry, copyright, 2022. b) Inkjet printing. Reproduced from ref. 89 with permission from American Chemical Society, copyright 2015. c) Electrospinning. Reproduced from ref. 103 with permission from American Chemical Society, copyright 2021. d) Layer by layer assembly. Reproduced from ref. 110 with permission from Springer Nature, copyright 2023. | |
Drop-casting
Drop-casting is a straightforward method for MOF integration on wearable substrates. In this approach, a solution containing MOF particles is directly dropped or cast onto the substrate and allowed to dry. The deposited MOF layer adheres to the substrate surface, forming a thin film that can be used as a sensing element. An illustration of the drop-casting process is shown in Fig. 2a. Drop-casting is suitable for proof-of-concept experiments and rapid prototyping of wearable sensors due to its ease of use and minimal equipment requirements.92–97
Electrospinning
Electrospinning is a versatile technique for integrating MOFs into wearable sensor platforms. It involves the electrostatic deposition of MOF nanofibers onto flexible substrates. The resulting fibrous structure enhances the active surface area of the sensor, leading to improved sensitivity and response times. Electrospinning also allows for combining MOFs with other nano-materials, such as carbon nanotubes or graphene, further enhancing sensor performance. The process of electrospinning is shown in Fig. 2c.98–103
Layer-by-layer assembly
Layer-by-layer assembly involves the sequential deposition of MOF layers and other functional materials onto the substrate. This approach enables precise control over the MOF thickness and the incorporation of additional functionalities, such as polymers or enzymes, for targeted sensing applications. An illustration of layer-by-layer assembly is shown in Fig. 2d. Layer-by-layer assembly can be performed using various techniques, including dip-coating, spin-coating, or spray-coating, offering flexibility in sensor design (Table 2).104–110
Table 2 Comparison of different fabrication techniques involved in wearable sensors
Fabrication method |
Advantages |
Disadvantages |
Inkjet printing |
Simple and fast fabrication process |
Customized ink jet printers are required |
Allows mass production |
Nozzle clogging |
Drop-casting |
Simple and low cost |
Limitations in large area coverage |
No wastage of materials |
Poor uniformity |
Film thickness is difficult to control |
Electrospinning |
Simple, scalable and cost-effective |
Toxic solvents |
Fabricates fiber diameters from a few nm to several microns |
Jet instability |
Layer-by-layer assembly |
Good uniformity |
Wastage of materials |
Control of layer thickness |
Limits in adhesion to the substrate |
Large area coverage |
Sensing mechanisms
The evolution of wearable technology has revolutionized our lives, making it possible to monitor our health, environment, and surroundings with unprecedented ease and precision. One of the key advancements in this domain is the utilization of metal–organic frameworks (MOFs) as sensing materials in wearable devices. MOFs are highly porous materials composed of metal ions or clusters coordinated with organic ligands. Their unique structural properties make them ideal candidates for diverse sensing applications, including chemical, physical, and biological sensing.111–115 In this article, we delve into the sensing mechanisms employed by MOF-based wearable sensors, highlighting specific examples of their utilization as gas sensors, biosensors, and pH sensors.
Chemical sensing mechanisms
Gas sensors
MOFs possess a high surface area and tunable pore sizes, providing an exceptional gas adsorption and detection platform.116–119 For instance, a MOF composed of zinc ions and 2-methylimidazole ligands has been employed to detect volatile organic compounds (VOCs) like benzene, toluene, and xylene. These VOCs are prevalent indoor air pollutants that can cause health issues upon prolonged exposure.120–124 Ali et al. reported the fabrication of a MOF–polymer mixed flexible membrane for detecting hydrogen sulfide, as shown in Fig. 3a. The prepared MOF-5 has a higher surface area of 621 m2 g−1 and 643 m2 g−1 and a larger pore size with the number density of MOF-5 being 2.46 × 1028 atoms per m3 which enhanced the energy transport. The presence of MOF-5 in the membrane matrix improves the transportation of H2S acidic protons across the membrane and throughout the open porosity of the MOF structure. The developed gas sensor showed high sensitivity, low power consumption and flexibility at room temperature.125 Metal–organic frameworks have been reported as sensor materials for gas sensors under varying humidity. In another study, five types of MOF-based sensors were tested for nitrogen gas, and the fabricated sensor is shown in Fig. 3b.126 The adsorption of specific gas molecules onto the MOF surface alters its electrical conductivity, leading to a measurable change in resistance. Another study reported developing dual-function metal–organic framework-based wearable fibres for NO2 sensors. The developed sensor showed an ultralow detection limit due to the presence of a gas sensitive MOF and high specific capacitance demonstrating its potential application for wearable devices.127 By integrating this MOF into a wearable sensor, individuals can now monitor their indoor air quality in real time, allowing timely intervention and improved health outcomes.
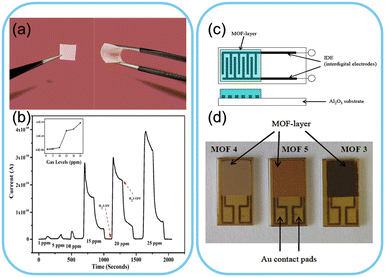 |
| Fig. 3 Gas sensors based on MOFs. a) MOF-5 based nanocomposite membrane. b) Electrical current response of the MOF-5 nanocomposite as a function of time and H2S concentration measured at room temperature. Inset: Response for the corresponding gas concentration. Reproduced from ref. 125 with permission from American Chemical Society, copyright 2021. c) Systematic view and d) schematic view of the developed MOF-based gas sensor. Reproduced from ref. 126 with permission from MDPI, copyright 2009. | |
pH sensors
MOFs can be engineered to exhibit pH-responsive behaviour due to protonatable functional groups in their organic linkers.128–130 A prime example is the utilization of UiO-66, a zirconium-based MOF, which undergoes structural changes in response to varying pH levels. These structural changes significantly alter the MOF's optical properties, making it an effective pH sensor.131–133 When incorporated into wearable devices, such pH-sensitive MOFs can continuously monitor physiological parameters like sweat pH, offering valuable insights into an individual's health status and hydration levels.
Physical sensing mechanisms
Strain and pressure sensors
The mechanical flexibility of MOFs makes them suitable candidates for strain and pressure-sensing applications. When subjected to external mechanical forces, MOFs can undergo reversible deformations, influencing their electrical conductivity or optical properties.134–138 A MOF-based strain sensor can be incorporated into wearable textiles to monitor body movements or detect potential injuries during physical activities. An ultrasensitive, anti-jamming and durable sensor was developed by Pan et al. They developed a metal–organic framework-based strain sensor with accurate signal detection and noise-screening capability. The developed sensor easily differentiated between muscle hyperplasia from subtle swaying and other vigorous activities. The fabricated sensor is shown in Fig. 4.139 In another study, 3D-printed colourimetric and mechanical sensors based on MOFs were developed. The developed device produced a colour change in the presence of acidic components and showed high sensitivity to mechanical deformation and various body movements.140 Another study fabricated a wearable self-powered pressure sensor based on ZIF-8, a zinc-based metal–organic framework. The prepared triboelectric nanogenerator is a wearable biomotion sensor that detects body movements with high sensitivity.141 This real-time feedback can help individuals optimize their exercise routines and avoid overexertion.
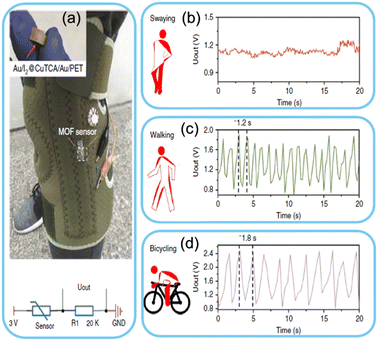 |
| Fig. 4 Strain sensor based on MOFs. a) Schematic of a developed Cu-based MOF integrated with an intelligent kneecap. The sensor's output signal under b) leg swaying, c) walking and d) bicycling. Reproduced from ref. 139 with permission from Springer Nature, copyright 2018. | |
Temperature sensors
The thermal responsiveness of certain MOFs allows them to serve as temperature sensors. When exposed to varying temperatures, the MOF lattice undergoes structural changes that lead to detectable electrical conductivity or thermal emissivity alterations.142–146 A thermometer based on a lanthanide metal–organic framework is reported with good sensitivity in the wide temperature range of 4 to 290 K.147 Another study reported the development of a multimode temperature sensor with high resolution demonstrated by temperature mapping.148 Integrating these MOFs into wearable devices enables precise temperature monitoring, which is crucial for healthcare, sports, and environmental monitoring applications.
Biological sensing mechanisms
Biosensors
The versatility of MOFs enables their functionalization with biomolecules, such as enzymes, antibodies, or nucleic acids, enabling the development of highly sensitive and specific biosensors.149–154 For example, a MOF-based biosensor functionalized with glucose oxidase can detect glucose levels in sweat or interstitial fluids, providing non-invasive glucose monitoring for individuals with diabetes.155 Creatine kinase, a cardiac biomarker widely used to diagnose myocardial infarction accurately, can be detected using MOFs with high sensitivity and selectivity (Fig. 5b).156 The immobilized biomolecules interact selectively with target analytes, triggering detectable changes in the MOF's properties, such as its optical absorbance or electrical impedance. By incorporating these MOF-based biosensors into wearable devices, real-time health monitoring and disease management become feasible for users.
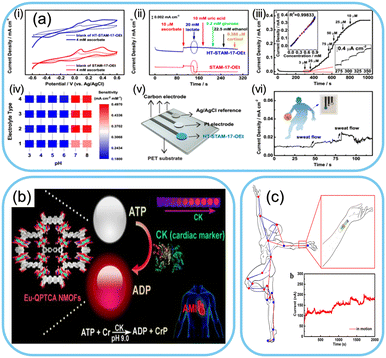 |
| Fig. 5 Biosensors based on MOFs. a) Ascorbate sensor based on a MOF: (i)–(iii) current response for ascorbate, Inset of (iii) shows corresponding calibration curve of amperometric response (iv) sensitivity in different electrolytes, (v) and (vi) fabricated sensor and data collection. Reproduced from ref. 161 with permission from Springer Nature, copyright 2023. b) Schematic representation of cardiac biomarker sensing by a lanthanide MOF. Reproduced from ref. 156 with permission from American Chemical Society, copyright 2019. c) Schematic diagram of a dancer dancing with a MOF-based glucose monitor and the corresponding response signal. Reproduced from ref. 162 with permission from Springer Nature, copyright 2023. | |
Furthermore, MOF-based wearable sensors represent a remarkable leap in sensing technology, offering a wide range of chemical, physical, and biological applications. Their unique structural properties, high surface area, and tunability enable precise detection and monitoring of various analytes, making them invaluable tools for healthcare, environmental monitoring, and beyond.157–160 Wang et al. reported a copper metal–organic framework sensor for sensing ascorbate in sweat. The developed sensor showed high selectivity for ascorbate. The sensing performance of the developed sensor is shown in Fig. 5a.161 A bimetallic metal–organic framework-based sensor was designed for detecting glucose from sweat during dancing. As shown in Fig. 5c, the wearable glucose sensor showed high sensitivity, repeatability, reproducibility, long-term stability and a low detection limit while monitoring sweat glucose levels during dancing.162 As researchers continue to explore novel MOF materials and functionalization techniques, the potential of wearable sensors to enhance our lives and well-being is bound to grow further, paving the way for a more thoughtful and healthier future.
Healthcare applications
Disease detection
MOF-based sensors have demonstrated tremendous potential in identifying diseases such as diabetes, cancer, and infectious diseases at their nascent stages, enabling timely interventions and improving patient outcomes.163–167 This review delves into the fascinating world of MOF-based sensors. It analyses the specific MOFs utilized, the target biomarkers they detect, and their exceptional sensitivity and selectivity in revolutionizing disease diagnosis.
Understanding MOFs and their sensing mechanism.
Metal–organic frameworks (MOFs) are a class of crystalline materials composed of metal ions or clusters linked by organic ligands. Their unique structural properties include a high surface area and tunable porosity, making them ideal for various applications, including gas storage, separation, and catalysis.168–172 More recently, scientists have harnessed the exceptional characteristics of MOFs to develop ultrasensitive sensors for disease detection.
MOF-based sensors operate on the principle of host–guest interactions. When the target biomarkers specific to a particular disease interact with the MOF's porous framework, they induce a change in the sensor's physical properties, such as electrical conductivity, fluorescence, or mass, allowing for precise and real-time detection.173–177
Early detection of diabetes.
Diabetes, a metabolic disorder affecting millions worldwide, requires early detection for effective management. MOF-based sensors have emerged as a promising tool for monitoring blood glucose levels non-invasively. By functionalizing the MOF surface with glucose-binding molecules, these sensors can detect even minute changes in glucose concentrations.178–180 A non-invasive flexible electrode material based on bimetallic metal–organic frameworks was fabricated by Zha et al. for glucose sensing and micro supercapacitors. The developed sensor exhibits high glucose sensitivity and high energy density. The intelligent sensing system with an integrated micro supercapacitor could accurately measure sweat glucose in real time.181 Another group prepared a non-invasive electrochemical glucose sensor that accurately detects glucose from sweat. The bimetallic-based glucose sensor showed excellent sensitivity and high stretchability and stability. The fabricated sensor is illustrated in Fig. 6a.182 In another study, a bimetallic MOF-based electrochemical sweat sensor was reported to monitor glucose levels from sweat continuously. The flexible sensor showed high sensitivity and stability and can be attached to the skin for continuous glucose monitoring for one day. The high-performance wearable sensor is shown in Fig. 6b.97 This advance in technology offers diabetic patients a convenient and pain-free alternative to traditional blood glucose monitoring methods.
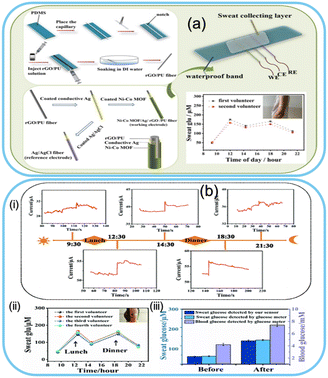 |
| Fig. 6 Wearable glucose sensor based on MOFs. a) Highly stretchable wearable Ni–Co MOF-based nanocomposite fibre for sweat glucose detection. Reproduced from ref. 182 with permission from American Chemical Society, copyright 2021. b) NCAP film-based wearable sweat sensor: (i) and (ii) real-time monitoring of glucose, (iii) comparison of the developed sensor with a commercial glucose meter. Reproduced from ref. 97 with permission from The Royal Society of Chemistry, copyright 2022. | |
Unveiling cancer biomarkers.
Cancer, a complex and heterogeneous disease, demands early diagnosis for successful treatment outcomes. MOF-based sensors have revolutionized cancer diagnostics by detecting specific biomarkers associated with different types of cancer. Functionalizing MOFs with antibodies or aptamers enables them to recognize and bind to cancer-specific biomolecules. This leads to early and accurate detection of cancerous cells or circulating tumour markers in bodily fluids.183–187 A CuBTC metal–organic framework-based composite was developed to detect ovarian cancer biomarkers. Due to the synergistic effect between the composite materials, the developed sensor showed excellent electrochemical performance and high practicability.188 Another study reported a Ni–hermin MOF, which mimics an enzyme for colourimetric cancer cell detection. The developed nanocomposite with folic acid as a recognition element was used for the colourimetric assay for direct cancer cell detection.189 Consequently, this technology holds tremendous promise in enhancing cancer screening and patient survival rates.
Combatting infectious diseases.
Infectious diseases remain a significant global health challenge, necessitating rapid and accurate diagnostics. MOF-based sensors offer an innovative approach to detect infectious agents such as bacteria, viruses, and parasites.190–192 A fluorescence molecularly imprinted sensor based on a metal–organic framework was developed to detect the virus accurately. The developed sensor showed high selectivity and specificity.193 Another luminescence-based biosensor based on an Fe-MOF was developed for common pathogens. The biosensor was able to detect P. aeruginosa and E. coli.194 Another study reported the optical detection of E. coli using a terbium-based MOF with high sensitivity and specificity.195 The high selectivity of MOFs allows them to be tailored to target distinct biomarkers associated with specific pathogens. As a result, MOF-based sensors provide a reliable and efficient means of early diagnosis, crucial for timely treatment and containment of infectious disease outbreaks.
Sensitivity and selectivity advantages.
One of the most remarkable attributes of MOF-based sensors is their exceptional sensitivity and selectivity. The high surface area and tunable porosity of MOFs enable efficient capture and concentration of target biomolecules, ensuring that even trace amounts of disease-related markers can be detected. Moreover, MOFs can be engineered to be highly selective, accurately distinguishing between similar biomolecules and reducing the likelihood of false-positive results.196–200
Physiological monitoring
These innovative sensors offer a revolutionary approach to physiological monitoring, enabling real-time tracking of vital signs such as heart rate, body temperature, and blood pressure.201–206 This article explores the significance of MOF-based wearable sensors and their potential to transform how we monitor and manage our health.
Understanding MOF-based wearable sensors
Metal–organic frameworks (MOFs) are porous materials of metal ions connected by organic linkers. Their unique structure gives them an exceptionally high surface area and tunable properties, making them ideal candidates for various applications, including gas storage, catalysis, and sensing.207–209
In the context of wearable sensors, MOFs have gained attention due to their ability to adsorb and interact with specific molecules, including gases and biomolecules.210–212 This property opens up new possibilities for non-invasive physiological monitoring. High-performance electronic devices for monitoring physiological changes are developed. A study reported the development of a wearable sensor based on zirconium-based metal–organic frameworks for arterial pulse monitoring. The developed device is based on the piezoelectric performance of the nanocomposites, and the results revealed high sensitivity for pulse monitoring as shown in Fig. 7a.213 Another group developed a breath sensor based on HKUST-1 MOF and MoS2 for monitoring respiratory disorders. The developed sensor was efficient in various breaths and showed fast response time and excellent stability. The prepared device is shown in Fig. 7b.214 Researchers have harnessed the versatility of MOFs to develop wearable sensors that can capture and detect biomarkers indicative of an individual's health status. An illustration representing the advantages and applications is shown in Fig. 8.
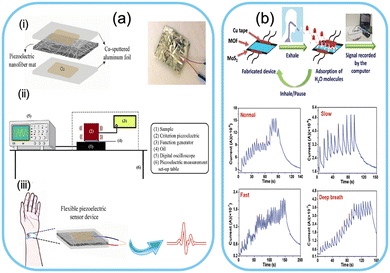 |
| Fig. 7 Physiological monitoring systems based on MOFs. a) Artery pulse monitoring. Schematic of (i) sensor assembly, (ii) set-up, (iii) recording of the artery pulse signal. Reproduced from ref. 213 with permission from American Chemical Society, copyright 2020. b) Fabricated breath sensor and current response showing different types of breath. Reproduced from ref. 214 with permission from The Royal Society of Chemistry, copyright 2020. | |
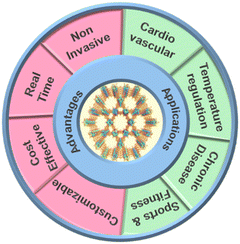 |
| Fig. 8 Schematic illustration of advantages and applications of MOF-based wearable sensors. | |
Advantages of MOF-based wearable sensors
Non-invasiveness
MOF-based wearable sensors offer a non-invasive alternative to traditional methods of vital sign monitoring. Unlike invasive techniques requiring needles or catheters, MOF-based sensors can be easily integrated into everyday accessories like wristbands or clothing, minimizing discomfort and promoting continuous monitoring.215–217
Real-time monitoring
MOFs' high sensitivity and selectivity enable real-time monitoring of various biomarkers. This capability is crucial for promptly detecting fluctuations in vital signs, allowing for timely intervention and better management of health conditions.218–220
Customizability
Researchers can design MOFs with tailored properties to target specific biomarkers. This customizability allows the development of wearable sensors optimized for monitoring various health parameters, making them versatile tools for personalized healthcare.221,222
Cost-effectiveness
Compared to some conventional medical monitoring techniques, MOF-based wearable sensors can be produced relatively cheaply. This cost-effectiveness makes them more accessible to a broader population, potentially improving healthcare outcomes across different socio-economic backgrounds.223
Applications of MOF-based wearable sensors
Cardiovascular health monitoring
MOF-based wearable sensors can detect and track heart rate and blood pressure, providing valuable insights into an individual's cardiovascular health. Continuous monitoring of these vital signs can aid in identifying irregularities, helping to prevent serious complications such as heart attacks or strokes.224,225
Temperature regulation
Monitoring body temperature is vital for detecting fever or hypothermia, which can indicate various infections or illnesses. MOF-based sensors can offer accurate and continuous body temperature tracking, making them essential for fever surveillance and patient care.226,227
Chronic disease management
Patients with chronic conditions like diabetes or hypertension can benefit significantly from MOF-based wearable sensors. These devices can help individuals manage their conditions more effectively by providing real-time data on blood glucose levels or blood pressure fluctuations.228,229
Sports and fitness
Athletes and fitness enthusiasts can utilize MOF-based wearable sensors to monitor their performance and physical exertion levels during workouts. These sensors can track heart rate, providing valuable information to optimize training routines and avoid overexertion.230–233
Drug delivery
MOFs are highly versatile and tunable structures of metal ions or clusters coordinated with organic linkers. Their unique properties, including high porosity, large surface area, and customizable chemical structures, have garnered immense interest for applications in various fields, including drug delivery.234–237 In this article, we will explore how MOFs are revolutionizing drug delivery systems, particularly in the context of wearable devices, offering unprecedented opportunities for personalized and precise medication administration.
The versatility of MOFs in drug delivery
MOFs are renowned for their extraordinary flexibility in design, allowing researchers to tailor their properties to suit specific drug delivery needs. The most critical property of drug carriers is the biocompatibility of the material. The tunable properties of MOFs favors producing a system with high biocompatibility and biodegradability.238–241 By selecting appropriate metal ions and organic linkers, MOFs can be engineered to possess desired pore sizes and surface functionalities. These characteristics enable MOFs to encapsulate a wide range of drug molecules, including small molecules, proteins, peptides, and nucleic acids. Moreover, MOFs can protect drugs from degradation and undesirable environmental interactions, ensuring their stability and bioavailability during delivery.242–246 However, continued research is needed to determine the long-term safety of MOF-based wearable devices.
Controlled drug release mechanisms
One of the most significant advantages of utilizing MOFs as drug carriers is their ability to control drug release rates. MOFs can be engineered to have stimuli-responsive behaviours triggered by environmental cues such as temperature, pH, light, or specific chemical signals. This responsiveness allows for precise on-demand drug release, maximizing therapeutic efficacy while minimizing potential side effects.247–250 Yang et al. developed an insulin delivery system depending on the blood glucose level. The enzyme-loaded drug delivery system was designed for pain-free insulin delivery through stimuli-responsive microneedles. The schematic illustration of drug release is shown in Fig. 9a.251 Another study reported the development of MOFs as a controllable drug delivery system for nitric oxide. The developed drug delivery system releases NO for accelerating diabetic wound healing as shown in Fig. 9b.252 Wearable devices equipped with MOF-based drug delivery systems can be programmed to release medications at specific times or in response to physiological indicators, making them ideal for treating chronic conditions or managing acute symptoms efficiently.
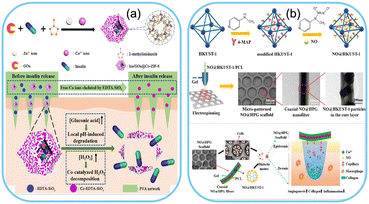 |
| Fig. 9 MOF-based drug delivery systems. Schematic representation of a) MOF-based microneedles for glucose-mediated transdermal insulin delivery. Reproduced from ref. 251 with permission from American Chemical Society, copyright 2020. b) Cu-based MOF for NO release for enhanced diabetic wound healing. Reproduced from ref. 252 with permission from American Chemical Society, copyright 2020. | |
Integration with wearable devices
Integrating MOFs into wearable devices marks a significant advancement in drug delivery technology. Wearable devices, such as smartwatches, patches, or implants, offer continuous monitoring and personalized care, enabling real-time feedback and adjustment of drug dosages.253–257 MOFs, as drug carriers in these devices, ensure that the right amount of medication is delivered at the right time, enhancing treatment outcomes and patient compliance. Additionally, the portability and unobtrusiveness of wearable devices make them an attractive option for patients, facilitating seamless drug administration and management of chronic conditions.
Advantages of MOF-based smart drug delivery systems
a. Improved patient adherence
MOF-based wearable drug delivery systems enable a more consistent and automated drug administration process, reducing the chances of missed doses and promoting patient adherence to treatment regimens.258
b. Enhanced therapeutic efficacy
The controlled and targeted drug release offered by MOFs ensures that medications reach their intended site of action in optimal concentrations, enhancing therapeutic efficacy while reducing the risk of systemic side effects.259,260
c. Reduced healthcare burden
With precise drug dosing and improved patient compliance, MOF-based intelligent drug delivery systems can potentially reduce hospital admissions and emergency room visits, alleviating the burden on healthcare systems.261
d. Personalized medicine
MOFs' tunable nature allows for personalized drug delivery strategies, tailoring treatments to individual patient needs to be based on their unique physiological profiles and disease characteristics.262
Challenges and future prospects
a. Stability in harsh environments
MOFs may experience degradation due to moisture, chemicals, or other harsh environmental conditions. Researchers need to develop strategies to enhance the stability of MOFs under real-world operating conditions.
b. Scalability and cost-effectiveness
Large-scale synthesis of MOFs at an affordable cost remains challenging. Researchers are exploring novel synthesis methods and scalable production techniques to make MOFs more commercially viable.
c. Selectivity and cross-sensitivity
Achieving high selectivity and avoiding cross-sensitivity to other compounds are critical for accurate and reliable sensing. Continued research is necessary to improve the selectivity of MOF-based sensors (Fig. 10).
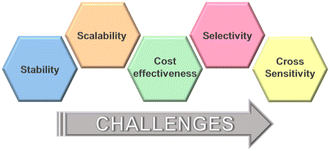 |
| Fig. 10 Schematic illustration of challenges in MOF-based wearable systems. | |
Conclusion
Metal–organic frameworks have emerged as a promising class of materials in developing wearable sensors for healthcare applications. The unique properties of MOFs offer opportunities for highly sensitive and selective sensing and intelligent drug delivery. While challenges remain, ongoing research and innovation hold tremendous potential for advancing MOF-based wearable sensors and revolutionizing healthcare monitoring and diagnostics. As technology evolves, these sensors may become integral to personalized medicine, leading to improved patient outcomes and enhanced quality of life.
Data availability
All required data can be found within the main manuscript file.
Code availability
Not applicable.
Author contributions
P. N. Blessy Rebecca: conceptualization, investigation, methodology, writing – original draft; D. Durgalakshmi: conceptualization, investigation, methodology; S. Balakumar: conceptualization, validation, review; R. Ajay Rakkesh: investigation, supervision, review & editing, conceptualization.
Conflicts of interest
The authors declare that they have no conflict of interest.
Acknowledgements
The authors acknowledge the SRM Institute of Science and Technology for providing SRM fellowship to conduct this research.
References
- A. A. Smith, R. Li and Z. T. H. Tse, Sci. Rep., 2023, 13, 4998 CrossRef CAS PubMed
.
- A. Sharma, M. Badea, S. Tiwari and J. L. Marty, Molecules, 2021, 26, 748 CrossRef CAS PubMed
.
- J. Kim, A. S. Campbell, B. E. F. de Avila and J. Wang, Nat. Biotechnol., 2019, 37, 389–406 CrossRef CAS PubMed
.
- H. C. Zhou, J. R. Long and O. M. Yaghi, Chem. Rev., 2012, 112, 673–674 CrossRef CAS PubMed
.
- P. Kumar, A. Deep and K. H. Kim, TrAC, Trends Anal. Chem., 2015, 73, 39–53 CrossRef CAS
.
- X. Fang, B. Zong and S. Mao, Nano-Micro Lett., 2018, 10, 64 CrossRef PubMed
.
- P. H. F. Fasna and S. Sasi, ChemistrySelect, 2021, 6, 6365–6379 CrossRef
.
- R. J. Kuppler, D. J. Timmons, Q. R. Fang, J.-R. Li, T. A. Makal, M. D. Young, D. Yuan, D. Zhao, W. Zhuang and H. C. Zhou, Coord. Chem. Rev., 2009, 253, 3042–3066 CrossRef CAS
.
- V. Russo, M. Hmoudah, F. Broccoli, M. R. Iesce, O. S. Jung and M. D. Serio, Front. Chem. Eng, 2020, 2, 581487 CrossRef
.
- A. R. M. Silva, J. Y. N. H. Alexandre, J. E. S. Souza, J. G. L. Neto, P. G. S. Junior, M. V. P. Rocha and J. C. S. dos Santos, Molecules, 2022, 27, 4529 CrossRef CAS PubMed
.
- L. Chen, X. Zhang, X. Cheng, Z. Xie, Q. Kuang and L. Zheng, Nanoscale Adv., 2020, 2, 2628–2647 RSC
.
- V. F. Yusuf, N. I. Malek and S. K. Kailasa, ACS Omega, 2022, 7, 44507–44531 CrossRef CAS PubMed
.
- Y. Li, G. Wen, J. Li, Q. Li, H. Zhang, B. Tao and J. Zhang, Chem. Commun., 2022, 58, 11488–11506 RSC
.
- M. Ma, J. Chen, H. Liu, Z. Huang, F. Huang, Q. Li and Y. Xu, Nanaoscale, 2022, 14, 13405–13427 RSC
.
- L. Jiao, J. Y. R. Seow, W. S. Skinner, Z. U. Wang and H.-L. Jiang, Mater. Today, 2019, 27, 43–68 CrossRef CAS
.
- H. Furukawa, K. E. Cordova, M. Okeeffe and O. M. Yaghi, Science, 2013, 341, 6149 CrossRef PubMed
.
- Y. Lin, Y. Li, Y. Cao and X. Wang, Chem. – Asian J., 2021, 16, 3281–3298 CrossRef CAS PubMed
.
- W. Wang, Y. Yu, Y. Jin, X. Liu, M. Shang, X. Zheng, T. Liu and Z. Xie, J. Nanobiotechnol., 2022, 20, 207 CrossRef CAS PubMed
.
- G. Chakraborty, I. H. Park, R. Medishetty and J. V. Vittal, Chem. Rev., 2021, 121, 3751–3891 CrossRef CAS PubMed
.
- M. T. Ulhakim, M. Rezki, K. K. Dewi, S. A. Abrori, S. Harimurti, N. L. W. Septiani, K. A. Kurnia, W. Setyaningsih, N. Darmawan and B. Yuliarto, J. Electrochem. Soc., 2020, 167, 136509 CrossRef CAS
.
- G. Cai, P. Yan, L. Zhang, H. C. Zhou and H. L. Jiang, Chem. Rev., 2021, 121, 12278–12326 CrossRef CAS PubMed
.
- X. Zhang, Z. Chen, X. Liu, S. L. Hanna, X. Wang, R. T. Ledari, A. Maleki, P. Li and O. K. Farha, Chem. Soc. Rev., 2020, 49, 7406–7427 RSC
.
- H. Furukawa, N. Ko, Y. B. Go, N. Aratani, S. B. Choi, E. Choi, A. O. Yazaydin, R. Q. Snurr, M. Okeeffee, J. Kim and O. M. Yaghi, Science, 2010, 329, 424–428 CrossRef CAS PubMed
.
- S. Yuan, L. Zou, J. S. Qin, J. Li, L. Huang, L. Feng, X. Wang, M. Bosch, A. Alsalme, T. Cagin and H. C. Zhou, Nat. Commun., 2017, 8, 15356 CrossRef CAS PubMed
.
- A. Sharma, A. Singh, V. Gupta, A. K. Sundramoorthy and S. Arya, Trends Environ. Anal. Chem., 2023, 38, e00200 CrossRef CAS
.
- S. Balasubramanian, A. J. Kulandaisamy, K. J. Babu, A. Das and J. B. B. Rayappan, Ind. Eng. Chem. Res., 2021, 60, 4218–4239 CrossRef CAS
.
- V. Stavila, A. A. Talin and M. D. Allendorf, Chem. Soc. Rev., 2014, 43, 5994–6010 RSC
.
- X. Wang, Y. Wang and Y. Ying, TrAC, Trends Anal. Chem., 2021, 143, 116395 CrossRef CAS
.
- M. Safaei, M. M. Foroughi, N. Ebrahimpoor, S. Jahani, A. Omidi and M. Khatami, TrAC, Trends Anal. Chem., 2019, 118, 401–425 CrossRef CAS
.
- N. Stock and S. Biswas, Chem. Rev., 2012, 112, 933–969 CrossRef CAS PubMed
.
- Y. R. Lee, J. Kim and W. S. Ahn, Korean J. Chem. Eng., 2013, 30, 1667–1680 CrossRef CAS
.
- X. Fu, B. Ding and D. D'Alessandro, Coord. Chem. Rev., 2023, 475, 214814 CrossRef CAS
.
-
D. Durgalakshmi, R. Ajay Rakkesh and J. Mohanraj, Graphene-Metal-Organic Framework-Modified Electrochemical Sensors, ed. A. Pandikumar and P. Rameshkumar, Elsevier, 2019, ch. 11, pp. 275–296 Search PubMed
.
- A. Sharma, J. Lim and M. S. Lah, Coord. Chem. Rev., 2021, 479, 214995 CrossRef
.
- B. Jie, H. Lin, Y. Zhai, J. Ye, D. Zhang, Y. Xie, X. Zhang and Y. Yang, Chem. Eng. J., 2023, 454, 139931 CrossRef CAS
.
- R. Sakamoto, N. Fukui, H. Maeda, R. Toyoda, S. Takaishi, T. Tanabe, J. Komeda, P. Amo-Ochoa, F. Zamora and H. Nishihara, Coord. Chem. Rev., 2022, 472, 214787 CrossRef CAS
.
- J. Munawar, M. S. Khan, S. E. Z. Syeda, S. Nawaz, F. A. Janjhi, H. U. Haq, E. U. Rashid, T. Jesionowski and M. Bilal, Inorg. Chem. Commun., 2023, 147, 110145 CrossRef CAS
.
- B. Xu, Z. Huang, Y. Liu, S. Li and H. Liu, Nanotoday, 2023, 48, 101690 CrossRef CAS
.
- B. Liu, M. Jiang, D. Zhu, J. Zhang and G. Wei, Chem. Eng. J., 2022, 428, 131118 CrossRef CAS
.
- J. Heikenfeld, A. Jajack, J. Rogers, P. Gutruf, L. Tian, T. Pan, R. Li, M. Khine, J. Kim, J. Wang and J. Kim, Lab Chip, 2018, 18, 217–248 RSC
.
- S. O. Kelley, ACS Sens., 2022, 7, 345–346 CrossRef CAS PubMed
.
- S. Nasiri and M. R. Khosravani, Sens. Actuators, A, 2020, 312, 112105 CrossRef CAS
.
- M. A. Sharabati, R. Sabouni and G. A. Husseini, Nanomaterials, 2022, 12, 277 CrossRef PubMed
.
- X. Ma, M. Lepoitevin and C. Serre, Mater. Chem. Front., 2021, 5, 5573–5594 RSC
.
- H. S. Wang, Y. H. Wang and Y. Ding, Nanoscale Adv., 2020, 2, 3788–3797 RSC
.
- T. Rezaee, R. F. Zarandi, A. Karimi and A. A. Ensafi, J. Pharm. Biomed. Anal., 2022, 221, 115026 CrossRef CAS PubMed
.
- J. Haider, A. Shahzadi, M. U. Akbar, I. Hafeez, I. Shahzadi, A. Khalid, A. Ashfaq, A. O. A. Ahmad, S. Dilpazir, M. Imran, M. Ikram, G. Ali, M. Khan, Q. Khan and M. Maqbool, Biomater. Adv., 2022, 140, 213049 CrossRef CAS
.
- H. Liu, L. Wang, G. Lin and Y. Feng, Biomater. Sci., 2022, 10, 614–632 RSC
.
- H. C. Zhou and S. Kitagawa, Chem. Soc. Rev., 2014, 43, 5415–5418 RSC
.
- J. Lei, R. Qian, P. Ling, L. Cui and H. Ju, TrAC, Trends Anal. Chem., 2014, 58, 71–78 CrossRef CAS
.
- M. G. Campbell and M. Dinca, Sensors, 2017, 17, 1108 CrossRef
.
- B. Liu, J. Mater. Chem., 2012, 22, 10094–10101 RSC
.
- K. Kamal, M. A. Bustam, M. Ismail, D. Grekov, A. M. Shariff and P. Pre, Materials, 2020, 13, 2741 CrossRef CAS PubMed
.
- P. Pachfule, R. Das, P. Poddar and R. Banerjee, Cryst. Growth Des., 2011, 11, 1215–1222 CrossRef CAS
.
- C. McKinstry, R. J. Cathcart, E. J. Cussen, A. J. Fletcher, S. V. Patwardhan and J. Sefcik, Chem. Eng. J., 2016, 285, 718–725 CrossRef CAS
.
- B. Zhang, Y. Luo, K. Kanyuck, N. Saenz, K. Reed, P. Zavalij, J. Mowery and G. Bauchan, RSC Adv., 2018, 8, 33059–33064 RSC
.
- A. Mukherjee, P. Dhak and D. Dhak, Environ. Sci.: Adv., 2022, 1, 121–137 Search PubMed
.
- K. Yu, G. Zhang, H. Chai, L. Qu, D. Shan and X. Zhang, Sens. Actuators, B, 2022, 362, 131808 CrossRef CAS
.
- M. Y. Zorainy, M. Sheashea, S. Kaliaguine, M. Gobara and D. C. Boffito, RSC Adv., 2022, 12, 9008–9022 RSC
.
- Z. Hu, Y. Wang and D. Zhao, Acc. Mater. Res., 2022, 3, 1106–1114 CrossRef CAS
.
- Y. Liang, W. G. Yuan, S. F. Zhang, Z. He, J. Xue, X. Zhang, L. H. Jing and D. B. Qin, Dalton Trans., 2016, 45, 1382–1390 RSC
.
- M. S. Samuel, K. V. Savunthari and S. Ethiraj, Environ. Sci. Pollut. Res., 2021, 28, 40835–40843 CrossRef CAS PubMed
.
- M. Ranjbar, M. A. Taher and A. Sam, J. Porous Mater., 2016, 23, 375–380 CrossRef CAS
.
- J. L. Crane, K. E. Anderson and S. G. Conway, J. Chem. Educ., 2015, 92, 373–377 CrossRef CAS
.
- W. Zhang, H. Yin, Z. Yu, X. Jia, J. Liang, G. Li, Y. Li and K. Wang, Nanomaterials, 2022, 12, 2062 CrossRef CAS PubMed
.
- Z. Ni and R. I. Masel, J. Am. Chem. Soc., 2006, 128, 12394–12395 CrossRef CAS PubMed
.
- R. Babu, R. Roshan, A. C. Kathalikkattil, D. W. Kim and D. W. Park, ACS Appl. Mater. Interfaces, 2016, 8, 33723–33731 CrossRef CAS PubMed
.
- J. Kliowski, F. A. A. Paz, P. Silva and J. Rocha, Dalton Trans., 2011, 40, 321–330 RSC
.
- H. Liu, Y. Zhao, C. Zhou, B. Mu and L. Chen, Chem. Phys. Lett., 2021, 7780, 138906 CrossRef
.
- L. H. T. Nguyen, T. T. T. Nguyen, Y. T. Dang, P. H. Tran and T. L. H. Doan, Asian J. Org. Chem., 2019, 8, 2276–2281 CrossRef CAS
.
- S. Glowniak, B. Szczesniak, J. Choma and M. Jaroniec, Molecules, 2023, 28, 2639 CrossRef CAS PubMed
.
- P. Arul, N. S. K. Gowthaman, S. A. John and H. N. Lim, ACS Omega, 2020, 5, 14242–14253 CrossRef CAS PubMed
.
- A. Taghipour, A. Rahimpour, M. Rastgar and M. Sadrzadeh, Ultrason. Sonochem., 2022, 90, 106202 CrossRef CAS PubMed
.
- O. Abuzalat, D. Wong, M. Elsayed, S. Park and S. Kim, Ultrason. Sonochem., 2018, 45, 180–188 CrossRef CAS PubMed
.
- Z. Q. Li, L. G. Qiu, T. Xu, Y. Wu, W. Wang, Z. Y. Wu and X. Jiang, Mater. Lett., 2009, 63, 78–80 CrossRef CAS
.
- F. Israr, D. K. Kim, Y. Kim, S. J. Oh, K. C. Ng and W. Chun, Ultrason. Sonochem., 2016, 29, 186–193 CrossRef CAS PubMed
.
- N. A. Khan and S. H. Jhung, Coord. Chem. Rev., 2015, 285, 11–23 CrossRef CAS
.
- J. E. Mondloch, W. Bury, D. F. Jimenez, S. Kwon, E. J. DeMarco, M. H. Weston, A. A. Sarjeant, S. T. Nguyen, P. C. Stair, R. Q. Snurr, O. K. Farha and J. T. Hupp, J. Am. Chem. Soc., 2013, 135, 10294–10297 CrossRef CAS PubMed
.
- B. Gikonyo, F. Liu, S. De, C. Journet, C. Marichy and A. Fateeva, Dalton Trans., 2023, 52, 211–217 RSC
.
- I. Stassen, D. D. Vos and R. Ameloot, Chem. – Eur. J., 2016, 22, 14452–14460 CrossRef CAS PubMed
.
- I. S. Kim, S. Ahn, N. A. Vermeulen, T. E. Webber, L. C. Gallington, K. W. Chapman, R. L. Penn, J. T. Hupp, O. K. Farha, J. M. Notestein and A. B. F. Martinson, J. Am. Chem. Soc., 2020, 142, 242–250 CrossRef CAS PubMed
.
- P. Su, M. Tu, R. Ameloot and W. Li, Acc. Chem. Res., 2022, 55, 186–196 CrossRef CAS PubMed
.
- W. Wu, J. Su, M. Jia, Z. Li, G. Liu and W. Li, Sci. Adv., 2020, 6, 18 Search PubMed
.
- D. A. Gregory, J. Nicks, J. A. Arnaudas, M. S. Harris, J. A. Foster and P. J. Smith, Adv. Mater. Interfaces, 2023, 10, 2300027 CrossRef CAS
.
- M. Gao, L. Li and Y. Song, J. Mater. Chem. C, 2017, 5, 2971–2993 RSC
.
- X. Du, S. P. Wankhede, S. Prasad, A. Shehri, J. Morse and N. Lakal, J. Mater. Chem. C, 2022, 10, 14091–14115 RSC
.
- P. Goel, S. Singh, H. Kaur, S. Mishra and A. Deep, Sens. Actuators, B, 2021, 329, 129157 CrossRef CAS
.
- C. H. Su, C. W. Kung, T. H. Chang, H. C. Lu, K. C. Ho and Y. C. Liao, J. Mater. Chem. A, 2016, 4, 11094–11102 RSC
.
- L. L. Da Luz, R. Milani, J. F. Felix, I. R. B. Ribeiro, M. Talhavini, B. A. D. Neto, J. Chojnacki, M. O. Rodrigues and S. A. Junior, ACS Appl. Mater. Interfaces, 2015, 7, 27115–27123 CrossRef CAS PubMed
.
- J. L. Zhuang, S. Ar, X. J. Yu, J. X. Liu and A. Terfort, Adv. Mater., 2013, 25, 4631–4635 CrossRef CAS PubMed
.
- M. Huo, H. Zhao, Y. Feng and J. Ge, Bioresour. Bioprocess., 2017, 4, 40 CrossRef
.
- N. K. Shrestha, S. A. Patil, S. Cho, Y. Jo, H. Kim and H. Im, J. Mater. Chem. A, 2020, 8, 24408–24418 RSC
.
- S. A. Patil, N. K. Shrestha, A. I. Inamdar, C. Bathula, J. Jung, S. Hussain, G. Nazir, M. Kaseem, H. Im and H. Kim, Nanomaterials, 2022, 12, 1916 CrossRef CAS PubMed
.
- Y. Zhang and C. H. Chang, Processes, 2020, 8, 377 CrossRef CAS
.
- P. Falcaro, A. J. Hill, K. M. Nairn, J. Jasieniak, J. I. Mardel, T. J. Bestow, S. C. Mayo, M. Gimona, D. Gomez, H. J. Whitfield, R. Ricco, A. Patellli, B. Marmiroli, H. Amenitsh, T. Colson, L. Villanova and D. Buso, Nat. Commun., 2011, 2, 237 CrossRef PubMed
.
- Y. He, Y. Wang, J. Shi, X. Lu, Q. Liu, Y. Liu, T. Zhu, D. Wang and Q. Yang, Chem. Eng. J., 2022, 446, 136866 CrossRef CAS
.
- Y. Shu, Z. Shang, T. Su, S. Zhang, Q. Lu, Q. Xu and X. Hu, Analyst, 2022, 147, 1440–1448 RSC
.
- X. Li, R. Zhou, Z. Wang, M. Zhang and T. He, J. Mater. Chem. A, 2022, 10, 1642–1681 RSC
.
- Q. Guo, Y. Li, X. Y. Wei, L. W. Zheng, Z. Q. Li, K. G. Zhang and C. G. Yuan, Ecotoxicol. Environ. Saf., 2021, 228, 112990 CrossRef CAS PubMed
.
- M. H. Hashem, M. Wahbe, P. Damacet, R. K. E. Habbal, N. Ghaddar, K. Ghali, M. N. Ahmed, P. Karam and M. Hmadeh, Langmuir, 2023, 39, 9503–9513 CrossRef CAS PubMed
.
- C. Chen, W. Zhanf, H. Zhu, B. G. Li, Y. Lu and S. Zhu, Nano Res., 2021, 14, 1465–1470 CrossRef CAS
.
- Y. Dou, W. Zhang and A. Kaiser, Adv. Sci., 2019, 7, 1902590 CrossRef PubMed
.
- M. Molco, F. Laye, E. Samperio, S. Z. Sharabani, V. Fourman, D. Sherman, M. Tsotsalas, C. Woll, J. Lahann and A. Sitt, ACS Appl. Mater. Interfaces, 2021, 13, 12491–12500 CrossRef CAS PubMed
.
- D. Jiang, A. D. Burrows, Y. Xiong and K. J. Edler, J. Mater. Chem. A, 2013, 1, 5497–5500 RSC
.
- L. Sarango, L. Paseta, M. Navarro, B. Zornoza and J. Coronas, J. Ind. Eng. Chem., 2018, 59, 8–16 CrossRef CAS
.
- B. Yi, Y. L. Wong, K. Li, C. Hou, T. Ma, Z. Xua and X. Yao, Nano Res., 2023, 16, 7716–7723 CrossRef CAS
.
- X. Wang, Y. Zheng, T. Wang, X. Xiong, X. Fang and Z. Zhang, Anal. Methods, 2016, 8, 8004–8014 RSC
.
- Y. S. Chae, S. Park, D. W. Kang, D. W. Kim, M. Kang, D. S. Choi, J. H. Choe and C. S. Hong, Chem. Eng. J., 2022, 433, 133856 CrossRef
.
- V. Chernikova, O. Shekhah and M. Eddaoudi, ACS Appl. Mater. Interfaces, 2016, 8, 20459–20464 CrossRef CAS
.
- H. Lim, H. Kwon, H. Kang, J. E. Jang and H. J. Kwon, Nat. Commun., 2021, 14, 3114 CrossRef PubMed
.
- J. E. Ellis, S. E. Crawford and K. J. Kim, Mater. Adv., 2021, 2, 6169–6196 RSC
.
- A. Zuliani, N. Khiar and C. C. Carrion, Anal. Bioanal. Chem., 2023, 415, 2005–2023 CrossRef CAS PubMed
.
- N. A. I. M. Mokhtar, R. M. Zawawi, W. M. Khairul and N. A. Yusof, Environ. Chem. Lett., 2022, 20, 3099–3131 CrossRef CAS
.
- S. N. Nangare, S. R. Patel, A. G. Patil, Z. G. Khan, P. K. Deshmukh, R. S. Tade, M. R. Mahajan, S. B. Bari and P. O. Patil, J. Nanostruct. Chem., 2022, 12, 729–764 CrossRef CAS
.
- S. N. Nangare, A. G. Patil, S. M. Chandankar and P. O. Patil, J. Nanostruct. Chem., 2023, 13, 197–242 CrossRef CAS
.
- B. Huang, Y. Li and W. Zeng, Chemosensors, 2021, 9, 226 CrossRef CAS
.
- C. Z. Wang, J. Chen, Q. H. Li, G. E. Wang, X. L. Ye, J. Lv and G. Xu, Angew. Chem., Int. Ed., 2023, 62, e202302996 CrossRef CAS PubMed
.
- R. Zhang, L. Lu, Y. Chang and M. Liu, J. Hazard. Mater., 2022, 429, 128321 CrossRef CAS
.
- O. Yassine, O. Shekhah, A. H. Assen, Y. Belmabkhout, K. N. Salama and M. Eddaoudi, Angew. Chem., Int. Ed., 2016, 55, 15879–15883 CrossRef CAS PubMed
.
- H. Yuan, N. L. W. Fan, H. Cai and D. Zhao, Adv. Sci., 2021, 9, 2104374 CrossRef PubMed
.
- H. Sohrabi, S. Ghasemzadeh, Z. Ghoreishi, M. R. Majidi, Y. Yoon, N. Dizge and A. Khataee, Mater. Chem. Phys., 2023, 299, 127512 CrossRef CAS
.
- H. Y. Li, S. N. Zhao, S. Q. Zang and J. Li, Chem. Soc. Rev., 2020, 49, 6364–6401 RSC
.
- K. Yu, M. Li, H. Chai, Q. Liu, X. Hai, M. Tian, L. Qu, T. Xu, G. Zhang and X. Zhang, Chem. Eng. J., 2023, 451, 138321 CrossRef CAS
.
- X. Peng, X. Wu, M. Zhang and H. Yuan, ACS Sens., 2023, 8, 2471–2492 CrossRef CAS PubMed
.
- A. Ali, A. Alzamly, Y. E. Greish, M. Bakiro, H. L. Nguyen and S. T. Mahmoud, ACS Omega, 2021, 6, 17690–17697 CrossRef CAS PubMed
.
- S. Achmann, G. Hagen, J. Kita, I. M. Malkowsky, C. Kiener and R. Moos, Sensors, 2009, 9, 1574–1589 CrossRef CAS PubMed
.
- K. Rui, X. Wang, M. Du, Y. Zhang, Q. Wang, Z. Ma, Q. Zhang, D. Li, X. Huang, G. Sun, J. Zhu and W. Huang, ACS Appl. Mater. Interfaces, 2018, 10, 2837–2842 CrossRef CAS PubMed
.
- B. V. Harbuzaru, A. Corma, F. Rey, J. L. Jorda, D. Ananias, L. D. Carlos and J. Rocha, Angew. Chem., Int. Ed., 2009, 48, 6476–6479 CrossRef CAS PubMed
.
- C. He, K. Lu and W. Lin, J. Am. Chem. Soc., 2014, 136, 12253–12256 CrossRef CAS PubMed
.
- H. Chen, J. Wang, D. Shan, J. Chen, S. Zhang and X. Lu, Anal. Chem., 2018, 90, 7056–7063 CrossRef CAS PubMed
.
- H. L. Jiang, D. Feng, K. Wang, Z. Y. Gu, Z. Wei, Y. P. Chen and H. C. Zhou, J. Am. Chem. Soc., 2013, 135, 13934–13938 CrossRef CAS PubMed
.
- Y. S. Sung, L. Y. Lin and H. Y. Lin, J. Taiwan Inst. Chem. Eng., 2020, 116, 197–204 CrossRef CAS
.
- J. A. Sigalat and D. Bradshaw, Chem. Commun., 2014, 50, 4711–4713 RSC
.
- L. Liu, Y. Gao, Y. Liu, M. Xu, S. Yang, K. Li, S. Zhao, D. Cao and J. H. Ahn, Appl. Surf. Sci., 2022, 593, 153417 CrossRef CAS
.
- M. Andrzejewski and A. Katrusiak, J. Phys. Chem. Lett., 2017, 8, 279–284 CrossRef CAS PubMed
.
- Y. Zhao, X. Li, N. Hou, T. Yuan, S. Huang, L. Li, X. Li and W. Zhang, Nano Energy, 2022, 104, 107966 CrossRef CAS
.
- N. Hou, Y. Zhao, R. Jiang, L. Nie, J. Yang, Y. Wang, L. Li, X. Li and W. Zhang, Colloids Surf., A, 2022, 650, 129638 CrossRef CAS
.
- H. H. M. Yeung, G. Yoshikawa, K. Minami and K. Shiba, J. Mater. Chem. A, 2020, 8, 18007–18014 RSC
.
- L. Pan, G. Liu, W. Shi, J. Shang, W. R. Leow, Y. Liu, Y. Jiang, S. Li, X. Chen and R. W. Li, Nat. Commun., 2018, 9, 3813 CrossRef PubMed
.
- S. Pal, Y. Z. Su, Y. W. Chen, C. H. Yu, C. W. Kung and S. S. Yu, ACS Appl. Mater. Interfaces, 2022, 14, 28247–28257 CrossRef CAS PubMed
.
- M. T. Rahman, M. S. Rahman, H. Kumar, K. Kim and S. Kim, Adv. Funct. Mater., 2023, 2303471 CrossRef
.
- X. Lian, D. Zhao, Y. Cui, Y. Yang and G. Qian, Chem. Commun., 2015, 51, 17676–17679 RSC
.
- S. Wang, J. Jiang, Y. Lu, J. Liu, X. Han, D. Zhao and C. Li, J. Lumin., 2020, 226, 117418 CrossRef CAS
.
- X. Liu, X. Han, Y. Lu, S. Wang, D. Zhao and C. Li, Inorg. Chem., 2021, 60, 4133–4143 CrossRef PubMed
.
- N. Hou, Y. Zhao, T. Yuan, L. Li, X. Li and W. Zhang, Composites, Part A, 2022, 163, 107177 CrossRef CAS
.
- Y. Wan, Y. Cui, Y. Yang and G. Qian, Chin. Chem. Lett., 2021, 32, 1511–1514 CrossRef CAS
.
- X. Liu, S. Akerboom, M. de Jong, I. Multikainen, S. Tanase, A. Meijerink and E. Bouwman, Inorg. Chem., 2015, 54, 11323–11329 CrossRef CAS PubMed
.
- W. Cao, Y. Cui, Y. Yang and G. Qian, ACS Mater. Lett., 2021, 3, 1426–1432 CrossRef CAS
.
- M. Li, G. Zhang, A. Boakye, H. Chai, L. Qu and X. Zhang, Front. Bioeng. Biotechnol, 2021, 9, 797067 CrossRef PubMed
.
- V. N. Palakollu, D. Chen, J. N. Tang, L. Wang and C. Liu, Microchim. Acta, 2022, 189, 161 CrossRef CAS
.
- J. Mohanraj, D. Durgalakshmi, R. Ajay Rakkesh, S. Balakumar, S. Rajendran and H. Karimi-Maleh, J. Colloid Interface Sci., 2020, 566, 463–472 CrossRef CAS PubMed
.
- J. Mohanraj, D. Durgalakshmi and R. Ajay Rakkesh, J. Electrochem. Soc., 2020, 167, 067523 CrossRef CAS
.
- P. N. Blessy, D. Durgalakshmi, S. Balakumar and R. Ajay Rakkesh, ChemistrySelect, 2022, 7, e202104013 CrossRef
.
- T. Rasheed and K. Rizwan, Biosens. Bioelectron., 2022, 199, 113867 CrossRef CAS PubMed
.
- M. Adeel, K. Asif, A. A. Rahman, S. Daniele, V. Canzonieri and F. Rizzolio, Adv. Funct. Mater., 2021, 31, 2106023 CrossRef CAS
.
- X. Li, S. Zhou, S. Lu, D. Tu, W. Zheng, Y. Liu, R. Li and X. Chen, ACS Appl. Mater. Interfaces, 2019, 11, 43989–43995 CrossRef CAS PubMed
.
- H. Sohrabi, S. Ghasemzadeh, S. Shakib, M. R. Majidi, A. Razmjou, Y. Yoon and A. Khataee, Ind. Eng. Chem. Res., 2023, 62, 4611–4627 CrossRef CAS
.
- T. Yan, G. Zhang, K. Yu, H. Chai, M. Tian, L. Qu, H. Dong and X. Zhang, Chem. Eng. J., 2023, 455, 140779 CrossRef CAS
.
- R. Ajay Rakkesh, D. Durgalakshmi and S. Balakumar, Nanotechnology, 2022, 33, 495703 CrossRef PubMed
.
- L. Du, W. Chen, P. Zhu, Y. Tian, Y. Chen and C. Wu, Biotechnol. J., 2020, 16, 1900424 CrossRef PubMed
.
- Z. Wang, Y. Huang, K. Xu, Y. Zhong, C. He, L. Jiang, J. Sun, Z. Rao, J. Zhu, J. Huang, F. Xiao, H. Liu and B. Y. Xia, Nat. Commun., 2023, 14, 69 CrossRef CAS PubMed
.
- Y. Mao, T. Chen, Y. Hu and K. Son, Discover Nano, 2023, 18, 62 CrossRef CAS PubMed
.
- Y. Wang, Y. Hu, Q. He, J. Yan, H. Xiong, N. Wen, S. Cai, D. Peng, Y. Liu and Z. Liu, Biosens. Bioelectron., 2020, 169, 112604 CrossRef CAS PubMed
.
- J. P. Leite, F. Figueria, R. F. Mendes, F. A. A. Paz and L. Gales, ACS Sens., 2023, 8, 1033–1053 CrossRef CAS PubMed
.
- S. Prabha, D. Durgalakshmi and R. Ajay Rakkesh, Surf. Interfaces, 2023, 41, 103207 CrossRef
.
- P. N. Blessy Rebecca, A. Krishna, D. Durgalakshmi, S. Balakumar and R. Ajay Rakkesh, Surf. Interfaces, 2023, 42, 103275 CrossRef
.
- S. Tajahmadi, H. Molavi, F. Ahmadijokani, A. Shamloo, A. Shojei, M. Sharifzadeh, M. Rezakazemi, A. Fatehizadeh, T. M. Aminabhavi and M. Arjmand, J. Controlled Release, 2023, 353, 1–29 CrossRef CAS
.
- A. U. Czaja, N. Trukhan and U. Muller, Chem. Soc. Rev., 2009, 38, 1284–1293 RSC
.
- X. Li, X. Yang, H. Xue, H. Pang and Q. Xu, EnergyChem, 2020, 2, 100027 CrossRef
.
- H. Li, L. Li, R. B. Lin, W. Zhou, Z. Zhang, S. Xiang and B. Chen, EnergyChem, 2019, 1, 100006 CrossRef
.
- L. Dandan, X. H. Qun, J. Long and J. H. Long, EnergyChem, 2019, 1, 100005 CrossRef
.
- A. Bavykina, N. Kolobov, II, S. Khan, J. A. Bau, A. Ramirez and J. Gascon, Chem. Rev., 2020, 120, 8468–8535 CrossRef CAS PubMed
.
- A. C. McKinlay, R. E. Morris, P. Horcajada, G. Ferey, R. Gref, P. Couvreur and C. Serre, Angew. Chem., Int. Ed., 2010, 49, 6260–6266 CrossRef CAS PubMed
.
- X. Chen, J. Dong, K. Chi, L. Wang, F. Xiao, S. Wang, Y. Zhao and Y. Liu, Adv. Funct. Mater., 2021, 31, 2102855 CrossRef CAS
.
- D. I. Osman, S. M. El-Sheikh, S. M. Sheta, O. I. Ali, A. M. Salem, W. G. Shousha, S. F. El-Khamisy and S. M. Shawky, Biosens. Bioelectron., 2019, 141, 111451 CrossRef CAS PubMed
.
- X. Guo, L. Zhou, X. Liu, G. Tan, F. Yuan, A. N. Ejhieh, N. Qi, J. Liu and Y. Peng, Colloids Surf., B, 2023, 229, 113455 CrossRef CAS PubMed
.
- P. P. Panahi, S. Belali, H. Sohrabi, F. Oroojalian, M. Hashemzaei, A. Mokhtarzadeh and M. de la Guardia, TrAC, Trends Anal. Chem., 2021, 141, 116285 CrossRef
.
- F. Hekmat, M. A. Kachouei, S. T. Foshtomi, S. Shahrokhian and Z. Zhu, Talanta, 2023, 257, 124375 CrossRef CAS PubMed
.
- X. Zhu, S. Yuan, Y. Ju, J. Yang, C. Zhao and H. Liu, Anal. Chem., 2019, 91, 10764–10771 CrossRef CAS
.
- S. Song, X. Ma, W. Li, B. Zhang, B. Shao, X. Chang and X. Liu, J. Alloys Compd., 2023, 931, 167413 CrossRef CAS
.
- X. Zha, W. Yang, L. Shi, Q. Zeng, J. Xu and Y. Yang, Dalton Trans., 2023, 52, 2631–2640 RSC
.
- Y. Shu, T. Su, Q. Lu, Z. Shang, Q. Xu and X. Hu, Anal. Chem., 2021, 93, 16222–16230 CrossRef CAS
.
- S. Zhang, F. Rong, C. Guo, F. Duan, L. He, M. Wang, Z. Zhang, M. Kang and M. Du, Coord. Chem. Rev., 2021, 439, 213948 CrossRef CAS
.
- B. Mohan, S. Kumar, H. Xi, S. Ma, Z. Tao, T. Xing, H. You, Y. Zhang and P. Ren, Biosens. Bioelectron., 2022, 197, 113738 CrossRef CAS
.
- S. Afreen, Z. He, Y. Xiao and J. J. Zhu, J. Mater. Chem. B, 2020, 8, 1338–1349 RSC
.
- B. Mohan, S. Kumar, V. Kumar, T. Jiao, H. K. Sharma and Q. Chen, TrAC, Trends Anal. Chem., 2022, 157, 116735 CrossRef CAS
.
- B. Mohan, D. Dhiman, B. Virender, G. Mehak, Priyanka, Q. Sun, M. Jan, G. Singh and N. Raghav, Microchem. J., 2023, 193, 108956 CrossRef CAS
.
- S. Li, C. Hu, C. Chen, J. Zhang, Y. Bai, C. S. Tan, G. Ni, F. He, W. Li and D. Ming, ACS Appl. Bio Mater., 2021, 4, 5494–5502 CrossRef CAS PubMed
.
- N. Alizadeh, A. Salimi, R. Hallaj, F. Fathi and F. Soleimani, J. Nanobiotechnol., 2018, 16, 93 CrossRef CAS PubMed
.
- F. Figueira, J. S. Barbosa, R. F. Mendes, S. S. Braga and F. A. A. Paz, Mater. Today, 2021, 43, 84–98 CrossRef CAS
.
- G. A. Udoutioh, M. M. Solomona and E. I. Epelle, Cell. Mol. Bioeng., 2021, 14, 535–553 CrossRef PubMed
.
- X. Guo, L. Wang, L. Wang, Q. Huang, L. Bu and Q. Wang, Front. Chem., 2023, 11, 1116524 CrossRef CAS PubMed
.
- J. Yang, W. Feng, K. Liang, C. Chen and C. Cai, Talanta, 2020, 212, 120744 CrossRef CAS PubMed
.
- D. Bhatt, S. Singh, N. Singhal, N. Bhardwaj and A. Deep, Anal. Bioanal. Chem., 2023, 415, 659–667 CrossRef CAS PubMed
.
- A. Gupta, M. Garg, S. Singh, A. Deep and A. L. Sharma, ACS Appl. Mater. Interfaces, 2020, 12, 48198–48205 CrossRef CAS PubMed
.
- S. Carrasco, Biosensors, 2018, 8, 92 CrossRef CAS PubMed
.
- S. E. Miller, M. H. Teplensky, P. Z. Moghadam and D. F. Jimenez, Interface Focus, 2016, 6, 20160027 CrossRef PubMed
.
- Z. Dourandish, S. Tajik, H. Beitollahi, P. M. Jahani, F. G. Nejad, I. Sheikhshoaie and A. D. Bartolomeo, Sensors, 2022, 22, 2238 CrossRef CAS PubMed
.
- A. Aggarwal, S. Solanki and B. D. Malhotra, Chem. Sci., 2022, 13, 8727–8743 RSC
.
- J. E. Souza, G. P. Oliveira, J. Y. N. H. Alexandre, J. G. L. Neto, M. B. Sales, P. G. S. Junior, A. L. B. Oliveira, M. C. M. Souza and J. C. S. Santos, Electrochemistry, 2022, 3, 89–113 CAS
.
- X. Wu, J. Yuan, X. Guo, L. Ma, G. Wu, G. J. Cheng, Y. Liu and F. Liu, ACS Appl. Electron. Mater., 2022, 4, 1723–1731 CrossRef CAS
.
- Q. Zhou, Z. Geng, L. Yang, B. Shen, Z. Kan, Y. Qi, S. Hu, B. Dong, X. Bai, L. Xu, H. Song and L. Ren, Adv. Sci., 2023, 10, 2207663 CrossRef CAS PubMed
.
- S. Wang, M. Liu, Y. Shi, X. Yang, L. Li, Q. Lu, H. Zheng, S. Feng, Y. Bai and T. Zhang, Sens. Actuators, B, 2022, 369, 132290 CrossRef CAS
.
- Y. Zhao, N. Hou, Y. Wang, C. Fu, X. Li, L. Li and W. Zhang, J. Mater. Chem. A, 2022, 10, 1248–1256 RSC
.
- S. Tharani, D. Durgalakshmi, S. Balakumar and R. Ajay Rakkesh, ChemistrySelect, 2022, 7, e202203603 CrossRef CAS
.
- C. Viravaux, O. Oms, A. Dolbecq, E. Nassar, L. Busson, C. M. Draznieks, R. Dessapt, H. S. Brault and P. Mialane, J. Mater. Chem. C, 2021, 9, 8323–8328 RSC
.
- C. Wang, D. Liu and W. Lin, J. Am. Chem. Soc., 2013, 135, 13222–13234 CrossRef CAS PubMed
.
- Y. Feng, Y. Wang and Y. Ying, Coord. Chem. Rev., 2021, 446, 214102 CrossRef CAS
.
- S. Yuan, J. Peng, B. Cai, Z. Huang, A. T. Garcia-Esparza, D. Sokaras, Y. Zhang, L. Giordano, K. Akkiraju, Y. G. Zhu, R. Hubner, X. Zou, Y. R. Leshkov and Y. S. Horn, Nat. Mater., 2022, 21, 673–680 CrossRef CAS PubMed
.
- C. Jiang, X. Wang, Y. Ouyang, K. Lu, W. Jiang, H. Xu, X. Wei, Z. Wang, F. Dai and D. Sun, Nanoscale Adv., 2022, 4, 2077–2089 RSC
.
- J. Zhuang, A. P. Young and C. K. Tsung, Small, 2017, 13, 1700880 CrossRef PubMed
.
- Q. Xing, Y. Pan, Y. Hu and L. Wang, Front. Chem., 2020, 8, 642 CrossRef CAS PubMed
.
- B. H. Moghadam, M. Hasanzadeh and A. Simchi, ACS Appl. Nano Mater., 2020, 3, 8742–8752 CrossRef CAS
.
- T. Leelasree, V. Selamneni, T. Akshaya, P. Sahatiya and H. Aggarwal, J. Mater. Chem. B, 2020, 8, 10182–10189 RSC
.
- J. Xiao, C. Fan, T. Xu, L. Su and X. Zhang, Sens. Actuators, B, 2022, 359, 121586 Search PubMed
.
- X. Yang, J. Yi, T. Wang, Y. Feng, J. Wang, J. Yu, F. Zhang, Z. Jiang, Z. Lv, H. Li, T. Huang, D. Si, X. Wang, R. Cao and X. Chen, Adv. Mater., 2022, 34, 2201768 CrossRef CAS PubMed
.
- Q. Qiu, H. Chen, Z. You, Y. Feng, X. Wang, Y. Wang and Y. Ying, ACS Appl. Mater. Interfaces, 2020, 12, 5429–5436 CrossRef CAS PubMed
.
- F. Cao, E. Ju, C. Liu, W. Li, Y. Zhang, K. Dong, Z. Liu, J. Ren and X. Qu, Nanoscale, 2017, 9, 4128–4134 RSC
.
- J. Yang, Z. Wang, Y. Li, Q. Zhuang and J. Gu, Chem. Mater., 2016, 28, 2652–2658 CrossRef CAS
.
- J. Li, J. Yu, Z. Sun, H. Liu and X. Wang, ACS Appl. Mater. Interfaces, 2021, 13, 41753–41772 CrossRef CAS PubMed
.
- A. Libanori, G. Chen, X. Zhao, Y. Zhou and J. Chen, Nat. Electron., 2022, 5, 142–156 CrossRef CAS
.
- I. Stassen, N. Burtch, A. Talin, P. Falcaro, M. Allendorf and R. Ameloot, Chem. Soc. Rev., 2017, 46, 3185–3241 RSC
.
- Y. Wang, M. Chao, P. Wan and L. Zhang, Nano Energy, 2020, 70, 104560 CrossRef CAS
.
- C. Rejeeth, A. Sharma, R. S. Kumar, A. I. Almansour, N. Arumugam, N. B. Varukattu and S. Afewerki, ACS Appl. Nano Mater., 2023, 6, 8071–8081 CrossRef CAS
.
- Y. Li, R. Wang, G. Wang, S. Feng, W. Shi, Y. Cheng, L. Shi, K. Fu and J. Sun, ACS Nano, 2022, 16, 473–484 CrossRef CAS PubMed
.
- Y. Zhao, X. Li, T. Yuan, S. Huang, R. Jiang, X. Duan, L. Li, X. Li and W. Zhang, Lab Chip, 2022, 22, 4593–4602 RSC
.
- Q. Gao, Q. Bai, C. Zheng, N. Sun, J. Liu, W. Chen, F. Hu and T. Lu, Biomolecules, 2022, 12, 1240 CrossRef CAS PubMed
.
- S. Fakharzadeh, H. Argani, P. M. Torbati, S. Dadashzadeh, S. Kalanaky, M. H. Nazaran and A. Basiri, Biology, 2020, 61, 126547 CAS
.
- Y. Xiong, Q. Feng, L. Lu, X. Qiu, S. Knoedler, A. C. Panayi, D. Jiang, Y. Rinkevich, Z. Lin, B. Mi, G. Liu and Y. Zhao, Adv. Mater., 2023, 2302587 CrossRef PubMed
.
- N. Wei, Y. Tang, Y. Li, Q. Wang, W. Zhang, D. Liang and R. Wu, Appl. Surf. Sci., 2022, 599, 153822 CrossRef CAS
.
- N. Hou, H. Wang, A. Zhang, L. Li, X. Li and W. Zhang, Lab Chip, 2023, 23, 2294–2303 RSC
.
- Z. Wang, T. Liu, L. Jiang, M. Asif, X. Qiu, Y. Yu, F. Xiao and H. Liu, ACS Appl. Mater. Interfaces, 2019, 1, 32310–32319 CrossRef PubMed
.
- S. M. S. Rana, M. A. Zahed, M. R. Islam, O. Faruk, H. S. Song, S. H. Jeong and J. Y. Park, Chem. Eng. J., 2023, 473, 144989 CrossRef
.
- H. D. Lawson, S. P. Walton and C. Chan, ACS Appl. Mater. Interfaces, 2021, 13, 7004–7020 CrossRef CAS PubMed
.
- J. D. Rocco, D. Liu and W. Lin, Acc. Chem. Res., 2011, 44, 957–968 CrossRef PubMed
.
- S. He, L. Wu, X. Li, H. Sun, T. Xiong, J. Liu, C. Huang, H. Xu, H. Sun, W. Chen, R. Gref and J. Zhang, Acta Pharm. Sin. B, 2021, 11, 2362–2395 CrossRef CAS PubMed
.
- Y. Sun, L. Zheng, Y. Yang, X. Qian, T. Fu, X. Li, Z. Yang, H. Yan, C. Cui and W. Tan, Nano-Micro Lett., 2020, 13, 103 CrossRef PubMed
.
- N. Singh, S. Qutub and N. M. Khashab, J. Mater. Chem. B, 2021, 9, 5925–5934 RSC
.
- M. Hoop, C. F. Walde, R. Ricco, F. Mushtaq, A. Terzopoulou, X. Z. Chen, A. J. DeMello, C. J. Doonan, P. Falcaro, B. J. Nelson, J. P. Luis and S. Pane, Appl. Mater. Today, 2018, 11, 13–21 CrossRef
.
- M. Hanke, H. K. Arslan, S. Bauer, O. Zybaylo, C. Christophis, H. Gliemann, A. Rosenhahn and C. Woll, Langmuir, 2012, 28, 6877–6884 CrossRef CAS PubMed
.
- G. Luo, Y. Jiang, C. Xie and X. Lu, Biosurf. Biotribol., 2021, 7, 99–112 CrossRef
.
- W. Cai, J. Wang, C. Chu, W. Chen, C. Wu and G. Liu, Adv. Sci., 2018, 6, 1801526 CrossRef PubMed
.
- Q. Sun, H. Bi, Z. Wang, C. Li, X. Wang, J. Xu, H. Zhu, R. Zhao, F. He, S. Gai and P. Yang, Biomaterials, 2019, 223, 119473 CrossRef CAS PubMed
.
- B. M. Jarai, Z. Stillman, L. Attia, G. E. Decker, E. D. Bloch and C. A. Fromen, ACS Appl. Mater. Interfaces, 2020, 12, 38989–39004 CrossRef CAS PubMed
.
- A. A. E. Bindary, E. A. Toson, K. R. Shoueir, H. A. Aljohani and M. M. Abo-Ser, Appl. Organomet. Chem., 2020, 34, e5905 CrossRef
.
- Y. Liu, C. S. Gong, Y. Dai, Z. Yang, G. Yu, Y. Liu, M. Zhang, L. Lin, W. Tang, Z. Zhou, G. Zhu, J. Chen, O. Jacobson, D. O. Kiesewetter, Z. Wang and X. Chen, Biomaterials, 2019, 218, 119365 CrossRef CAS
.
- Z. Zhou, M. V. Gonzalez and I. Willner, Chem. Soc. Rev., 2021, 50, 4541–4563 RSC
.
- S. Karimzadeh, S. Javanbakht, B. Baradaran, M. A. Shahbazi, M. Hashemzaei, A. Mokhtarzadeh and H. A. Santos, Chem. Eng. J., 2021, 408, 127233 CrossRef CAS
.
- X. Feng, Z. Xu, P. Dong, W. Yu, F. Liu, Q. Jiang, F. Wang and X. Liu, J. Mater. Chem. B, 2019, 7, 994–1004 RSC
.
- C. Li, K. Wang, J. Li and Q. Zhang, ACS Mater. Lett., 2020, 2, 779–797 CrossRef CAS
.
- X. X. Yang, P. Feng, J. Cao, W. Liu and Y. Tang, ACS Appl. Mater. Interfaces, 2020, 12, 13613–13621 CrossRef CAS PubMed
.
- P. Zhang, Y. Li, Y. Tang, H. Shen, J. Li, Z. Yi, Q. Ke and H. Xu, ACS Appl. Mater. Interfaces, 2020, 12, 18319–18331 CrossRef CAS PubMed
.
- Y. Zhang, T. T. Li, L. Sun, B. C. Shiu, L. Zhang, J. H. Lin and C. W. Lou, Colloids Surf., B, 2023, 229, 113442 CrossRef CAS PubMed
.
- C. C. Carrion, Anal. Bioanal. Chem., 2020, 412, 37–54 CrossRef
.
- G. W. Peterson, D. T. Lee, H. F. Barton, T. H. Epps II and G. N. Parsons, Nat. Rev. Mater., 2021, 6, 605–621 CrossRef CAS
.
- B. Zhang, H. Chen, Q. Hu, L. Jiang, Y. Shen, D. Zhao and Z. Zhou, Adv. Funct. Mater., 2021, 31, 2105395 CrossRef CAS
.
- X. G. Wang, L. Xu, M. J. Li and X. Z. Zhang, Angew. Chem., Int. Ed., 2020, 59, 18078–18086 CrossRef CAS PubMed
.
- H. Zhang, Y. Shang, Y. H. Lim, S. K. Sun and X. B. Yin, ACS Appl. Mater. Interfaces, 2019, 11, 1886–1895 CrossRef CAS PubMed
.
- Y. Feng, X. Cao, L. Zhang, J. Li, S. Cui, Y. Bai, K. Chen and J. Ge, Chem. Eng. J., 2022, 439, 135736 CrossRef CAS
.
- M. Falsafi, A. S. Saljooghi, K. Abnous, S. M. Taghdisi, M. Ranezani and M. Alibolandi, Biomater. Sci., 2021, 9, 1503–1529 RSC
.
- T. Wen, G. Quan, B. Niu, Y. Zhou, Y. Zhao, C. Lu, X. Pan and C. Wu, Small, 2021, 17, 2005064 CrossRef CAS
.
- Z. Chen, M. C. Wasson, R. J. Drout, L. Robison, K. B. Idrees, J. G. Knapp, F. A. Son, X. Zhang, W. Hierse, C. Kuhn, S. Marx, B. Hernandez and O. K. Farha, Faraday Discuss., 2021, 225, 9–69 RSC
.
|
This journal is © The Royal Society of Chemistry 2023 |