DOI:
10.1039/D2SD00233G
(Critical Review)
Sens. Diagn., 2023,
2, 307-319
Recent progress in nanozyme-based sensors for ion detection: strategies, trends, and challenges
Received
28th December 2022
, Accepted 6th February 2023
First published on 15th February 2023
Abstract
In situ real-time monitoring of pollution sources is one of the most effective means to control and prevent toxic ions from harming the environment and human health. To this end, it is critical to develop rapid, cost-effective, and user-friendly methods for ion detection. Nanozymes, like natural enzymes, can efficiently catalyze substrate reactions with the advantages of simple synthesis, low cost, long storage time, and excellent environmental stability. Importantly, certain ions can regulate the properties of nanozymes or affect their catalytic systems. Therefore, nanozymes have found increasing use in ion sensing. To highlight the latest advances made in this interesting area, here we focused on summarizing the progress in nanozyme-based ion assays developed mainly in the past three years. Emphatically, current trends and future challenges as well as potential solutions were discussed in detail. Our review will provide some guides to rationally design and develop nanozymes for biochemical sensing, especially for toxic ion detection and control. Also, it is expected to attract more interest in this promising field.
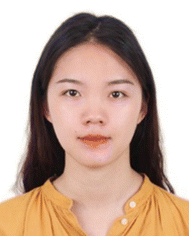 Xin Li | Xin Li is currently a special research assistant at Lanzhou Institute of Chemical Physics, Chinese Academy of Sciences. She received her Ph.D. degree from Jiangsu University in 2022 and spent two years as a visiting scholar at Washington State University during her doctoral studies. She has continually worked on novel nanozyme applications in environmental sensing and is currently working on the design and preparation of novel MOF nanozymes for use in natural drug screening. |
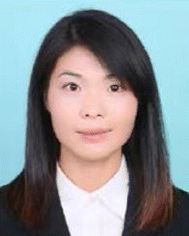 Jinjin Liu | Jinjin Liu is an assistant engineer working in Prof. Niu's group in the University of South China. She received her master's degree from East China University of Science and Technology in 2013. She is now studying the design and preparation of new nanozymes and their biochemical analytical applications. |
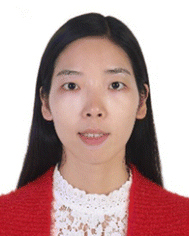 Jia Chen | Jia Chen received her master's degree at Guangxi Normal University and Ph.D. degree at the University of Chinese Academy of Science. Currently she is an associate professor in Lanzhou Institute of Chemical Physics, Chinese Academy of Sciences. Her main research interest focuses on the development of new functional nanomaterials and their applications in chemical and biological sensing. She has published more than 100 papers and 26 patents. |
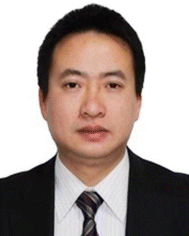 Hongdeng Qiu | Hongdeng Qiu is a full professor in Lanzhou Institute of Chemical Physics, Chinese Academy of Sciences. He received his Ph.D. degree from Lanzhou Institute of Chemical Physics, Chinese Academy of Sciences, and had a postdoctoral position in Kumamoto University from 2009 to 2012 (JSPS Fellow). His current research interests are the applications of new materials in chromatography and biosensors, especially ionic liquids and porous nanomaterials for separation and detection of complex analytes. He is the Editor of Chinese Chemical Letters and an Editorial Board Member of Chromatographia, Separation Science Plus, and Chinese Journal of Chromatography. He has published more than 190 papers and 30 patents. |
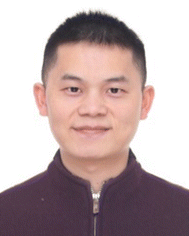 Xiangheng Niu | Xiangheng Niu is a professor now working in the University of South China. He received his Ph.D. degree from East China University of Science and Technology in 2015. After that, he worked in Jiangsu University several years. He focuses his interest on the development of nanozymes and their applications in medical diagnosis, biochemical analysis, environmental monitoring, and food safety. He has published over 130 peer-reviewed papers with an H-index of 42. |
1. Introduction
Because of large-scale mining, industrial development and unregulated waste discharge, inorganic ions, particularly heavy metal ions, have brought severe pollution and damage to the environment and health impacts worldwide.1 As one of the most effective means to control and prevent toxic ion pollution, developing efficient methods to monitor them becomes especially important. Although a few instrumental techniques (atomic emission spectrometry, atomic absorption spectrometry, atomic fluorescence spectrometry, ion chromatography, mass spectrometry, et al.) have been established well for ion detection in the laboratory, the requirements of sophisticated instruments and professional operators hinder their wider use in practical in-field analysis. Developing rapid, cost-effective, and user-friendly methods for ion sensing is always desired.2
Since magnetic Fe3O4 particles were discovered to exhibit intrinsic peroxidase-mimetic catalytic activity by Yan's group in 2007,3 nanomaterials with enzyme-like characteristics, defined as ‘nanozymes’ by Wei and Wang,4 have gradually become the most popular artificial enzymes,5 with the superiorities of simple synthesis, low cost, long storage time, and excellent environmental stability over natural enzymes. A lot of materials, including carbon materials, noble metals, and transition metal oxides/sulfides/hydroxides, have been explored as enzyme mimics to be used in biomedicine, environmental remediation, and sensing.6–10 In particular, in the biochemical sensing field, nanozymes can trigger chromogenic or fluorigenic reactions stably to give easy-to-read and sensitive results with catalytic signal amplification.11,12 This attractive feature makes them intensively employed as alternatives to natural enzymes or catalytic labels in various biochemical analyses.13–17
As a typical example of analytical applications, various nanozymes have been explored to establish methods for toxic ion detection. Currently, there are mainly four principles developed to monitor ions based on nanozyme catalysis (Fig. 1). Ions like Ce4+, Cu2+ and Fe2+ show certain catalytic activity, enabling the detection of these ions when used as nanozyme catalysts. Instead, some ions participate in nanozyme-catalyzed reactions as co-reactants, providing another important principle to sense ions. More commonly, many ions have the capacity to specifically adjust the catalytic performance of nanozymes, offering a general strategy to realize the determination of target ions via measuring the latter's activity change. Besides, some target ions are involved in reaction systems catalyzed by nanozymes, also enabling one to develop sensors for ion monitoring. By employing these principles, increasing experimental studies have been performed to detect toxic ions based on nanozyme catalysis.
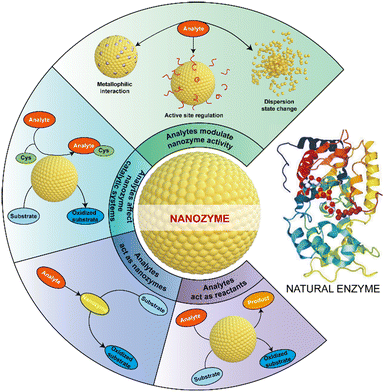 |
| Fig. 1 Principles of ion detection based on nanozyme catalysis. | |
To date, many experimental studies have been carried out to sense toxic ions with nanozymes. Several reviews have summarized this hot topic. For example, we previously presented a comprehensive overview on the applications of nanozymes as an emerging tool in environmental pollutant detection, where we systematically sorted out nanozyme-based analytical approaches for various pollutants.18 Yan et al. addressed the mechanisms of the interactions between nanozyme-based colorimetric sensors and heavy metal ions from the perspective of nanozyme materials, such as metal nanoparticles, metal oxides, metal sulfides, graphene-based nanomaterials, and G-quadruplexes.19 Recently, Huang et al. also summarized the strategies and mechanisms of toxic ion detection by nanozymes.20 Although these reviews were published, no one summarized the latest nanozyme sensing trends for toxic ions. To highlight the latest advances made in this flourishing field, here we tried to make a minireview of the progress of nanozyme-based sensors for ion monitoring. Different from the previous reviews focusing on the mechanisms of nanozyme-based ion assays,19,20 in the present work we summarized the latest advances of nanozyme-based sensors achieved mainly in the past three years for ion monitoring, with our focus on analyzing the development trends and current challenges of this area. Common sensing strategies were introduced first, followed by emphatically discussing current trends and future challenges as well as potential solutions in detail. Our work uncovered the mainstream of this field, revealed some doubts or controversies about detection mechanisms, and contributed to the rational development of nanozymes for ion detection. It is believed that this minireview of the latest advances can help one better understand the popular area.
2. Nanozyme strategies to detect ions
2.1. Analytes act as reactants
One of the most crucial aspects of environmental evaluation is to detect target ions selectively and sensitively. Given that the biological toxicity of multivalent ions varies with their forms, detecting these unstable species becomes more important and difficult. The rapid conversion, hydrolysis, and coordination between different forms render them unsuitable for conventional measurement methods that require long-term storage or long-distance transfer of samples. Visualization methods based on nanozyme catalysis have recently been discovered to allow rapid sensing of multivalent ions in situ. Taking advantage of the redox property of multivalent ions, metal species like Cr6+ and Fe2+ can act as co-reactants to trigger nanozyme-catalyzed reactions,21–24 thus supplying the basis to identify these multivalent ions. Typically, Lu's group explored Au@Hg as an oxidoreductase mimic and employed it to catalyze the redox reaction between Cr6+ and 3,3′,5,5′-tetramethylbenzidine (TMB), thus establishing a colorimetric method for Cr6+ analysis.23 In the presence of a small quantity of oxidoreductase-like Au@Hg, Cr6+ replaced H2O2 and directly oxidized the colorless reactant TMB to blue oxTMB (Fig. 2). The color of the solution changed dramatically from colorless to blue as the Cr6+ concentration increased. This finding provided a new foundation to detect Cr6+ with high sensitivity, rapid response, and excellent selectivity against coexisting ions.
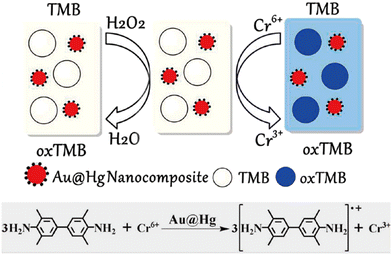 |
| Fig. 2 Cr6+ acted as a co-reactant to induce the oxidation of TMB with Au@Hg as an oxidoreductase mimic (reproduced from ref. 23 with permission from the American Chemical Society, copyright 2018). | |
2.2. Analytes act as nanozymes
Certain ions like Ce4+,25 Cu2+ (ref. 26) and UO22+ (ref. 27) exhibit unexpected enzyme-like activity through hydrolysis, oxidation or the formation of intermediates, which can catalyze some reaction systems to allow the establishment of analytical methods for target ions. For example, considering that Ce4+ was easily hydrolyzed and difficult to detect, Liu's group proposed an ingenious detection idea by using the hydrolyzate of Ce4+ as a nanozyme, whose catalytic activity could further be enhanced with the help of F−.25 Similarly, the same group found that the enzyme-like activity of Cu2+ was effectively improved after the addition of nucleotides or bases to generate coordination polymers, on the basis of which a colorimetric detection strategy for Cu2+ was implemented.26 Jiang et al. found that UO22+ exhibited efficient artificial peroxidase activity to catalyze the oxidation of 2,2′-azino-bis-(3-ethylbenzthiazoline-6-sulphonate) (ABTS) by H2O2, whereas other common substrates were not oxidized.27 Based on this finding, they developed a simple, cost-effective, and stable colorimetric method to detect UO22+.
2.3. Analytes modulate nanozyme activity
Since the catalytic sites of nanozymes are often exposed on the external surface, their activity is susceptible to the influence of outside substances. The modulation of nanozyme catalytic activity by toxic ions leads to a main principle to detect these targets. In detail, certain metal ions can be reduced in situ and deposited onto the surface of nanozymes through metallophilic interactions; also, target ions can strengthen or decrease the catalytic activity of nanozymes via interacting with the modifiers on the latter's surface; besides, some ions can change the dispersion state of nanozymes, which also affects the catalytic activity of the latter. As a result, these interactions result in a few strategies for detecting target ions based on nanozyme activity change.
(1) Metallophilic interactions between analytes and nanozymes.
Many studies have demonstrated that some ions can be rapidly adsorbed, reduced, and deposited onto the surface of noble metal nanozymes (Pt, Au, Ag, etc.) via metallophilic interactions to form alloys, thereby modulating the catalytic activity of the nanozymes.28,32–42 Typically, Hg2+ can be reduced by nanosized noble metal atoms or by interaction with modifiers on the surface of the nanozyme. As a result, the catalytic activity of these noble metals is stimulated or inhibited by the presence of Hg2+. For instance, Wu et al. created platinum nanoparticles (Pt NPs) using sodium borohydride and trisodium citrate as reducing and stabilizing agents, respectively, where the citrate species covering the surface of the Pt NPs could chemically reduce Hg2+ into Hg0, and the formation of the PtHg amalgam inhibited the peroxidase-like activity of Pt NPs significantly.32 Based on such a principle, a colorimetric sensor with high specificity and good sensitivity for Hg2+ was developed. In this experiment, a suitable reducing agent is usually desired to transform Hg2+ totally to Hg0. The type and content of the reducing agent used can greatly affect the assay's accuracy. Instead of using any reducing agent, Hg2+ can also be directly reduced on the Pt surface due to the difference in the standard potentials of Pt4+/Pt and Hg2+/Hg.34,43 The findings demonstrate that Pt0 stimulates Hg2+ reduction in Pt NPs and produces PtHg alloy on its surface, which drastically suppresses the nanozyme's catalytic activity. Apart from Pt nanozymes, Au-based nanomaterials can also interact with Hg2+ to form amalgams.28,39 Typically, Ma et al. synthesized a MoS2–Au composite with peroxidase activity via a microwave-assisted solvothermal method, where MoS2 nanosheets provided a large surface area and synergized with Au to endow the composite with excellent catalytic activity.28 The addition of Hg2+ could further stimulate its peroxidase-like activity, which was used to determine Hg2+ through employing the chromogenic system of H2O2 and TMB (Fig. 3A).
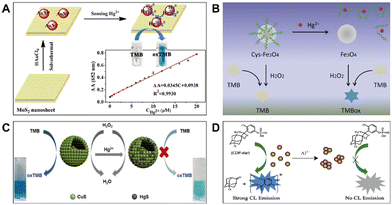 |
| Fig. 3 (A) Schematic diagram for the colorimetric determination of Hg2+ based on the excitation of MoS2–Au peroxidase activity (reproduced from ref. 28 with permission from Elsevier, copyright 2019). (B) Principle of Hg2+ detection based on the target-triggered peroxidase activity of Cys–Fe3O4 (reproduced from ref. 29 with permission from Elsevier, copyright 2019). (C) Addition of Hg2+ led to the production of HgS on the CuS surface and decreased the enzymatic activity of the latter (reproduced from ref. 30 with permission from the American Chemical Society, copyright 2020). (D) Al3+-induced aggregation effect of ceria nanoparticles led to a decrease in their phosphatase mimetic activity (reproduced from ref. 31 with permission from Elsevier, copyright 2020). | |
(2) Analyte-induced active site regulation of nanozymes.
Because the catalytic reaction takes place primarily at the catalyst's interface, the exposure or masking of active sites becomes one of the most important factors influencing nanozyme catalytic activity. With this consideration, our group suggested a new method based on the peroxidase-mimicking activity of cysteine-modified ferromagnetic particles (Cys–Fe3O4) for the ultrasensitive and selective measurement of Hg2+.29 Due to the coordination interaction between Cys and Fe3O4, Cys wrapped around the outer surface of Fe3O4 and blocked its active sites. Therefore, the prepared Cys–Fe3O4 showed almost no peroxidase activity (Fig. 3B). When Hg2+ was present, the stronger coordination between Hg2+ and Cys (Cys–Hg2+–Cys) caused Cys to slip off the surface of Fe3O4, resulting in the re-exposure of the Fe3O4 active site and the restoration of the catalytic substrate color development ability. Because of the strong interaction between Cys and Hg2+, the developed sensor has improved selectivity and can detect Hg2+ without external interference. A similar strategy was also applied to the sensing of Ag+ when using histidine-modified palladium nanoparticles as a nanozyme.44
Another strategy for analyte-induced modulation of enzyme activity is based on the high affinity of Hg2+ toward S-containing nanozymes. Hg2+ can generate stable HgS via strong chelation with the S2− component in nanozymes, and the formed HgS inhibits the nanozymes' activity via replacing their active components and blocking reaction sites.30,45–48 Thus, Hg2+ can effectively modulate the catalytic activity of nanozymes. For example, Fang et al. found that CuS nanoparticles with peroxidase-like activity exhibited excellent selectivity and adsorption capacity for Hg2+ (Fig. 3C).30 By utilizing the analyte-triggered peroxidase activity inhibition via forming an inert HgS layer onto the CuS surface, an extremely sensitive Hg2+ nanosensor was verified, demonstrating its great promise in environmental and food analyses.
(3) Analyte-induced dispersion state change of nanozymes.
Recent research has indicated that the target-induced dispersion state change can be utilized to modulate nanozyme activity.31,49,50 The aggregation state of nanozymes has a significant impact on their catalytic activity; thus, the resulting target-induced changes in the dispersion state of nanozymes will have a significant impact on the catalytic system. Tian et al. described a highly sensitive chemiluminescence strategy for Al3+ sensing based on ceria's phosphatase mimetic activity.31 As a result of the coordination between Al3+ and ceria, the nanozyme aggregated and lost its catalytic activity for substrate hydrolysis (Fig. 3D). Similarly, our group applied the nanozyme dispersion state change strategy to detect arsenite. Arsenite could cause the aggregation of citrate-stabilized gold nanoparticles to exhibit enhanced oxidase-like activity, providing a new colorimetric pathway for arsenite determination.51
2.4. Analytes affect nanozyme catalytic systems
Some nanozyme catalytic systems can be modulated indirectly by the stronger interactions between target ions and foreign reagents like cysteine (Cys),52,53 glutathione (GSH),54,55 mercaptopropionic acid56 and 8-hydroxyquinoline.57 Typically, Christus et al. discovered that the Fe3O4@ZIF-67 hybrid showed peroxidase activity to catalyze the conversion of colorless TMB to blue TMBox in the presence of H2O2.54 After the addition of GSH to the solution, the blue color disappeared due to its strong antioxidant ability against reactive oxygen species (Fig. 4A). When Hg2+ was further introduced into the Fe3O4@ZIF-67/TMB/GSH system, the solution color returned to blue because of the generation of mercury–thiol complexes. Using this mechanism, the authors created a high-selectivity Hg2+ colorimetric sensor. The strategy assisted by foreign molecules can also be extended to detect other toxic cations and anions, including Cr6+,57 Cu2+ (ref. 56) and phosphate ions.58
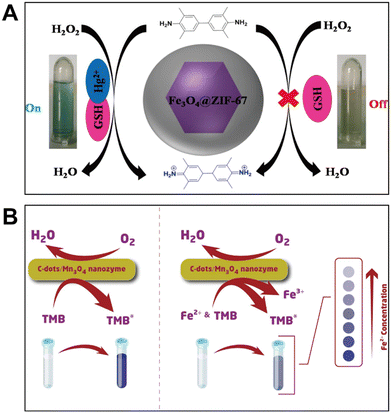 |
| Fig. 4 (A) Colorimetric detection of Hg2+ using Fe3O4@ZIF-67 to catalyze the oxidation of TMB affected by GSH (reproduced from ref. 54 with permission from Elsevier, copyright 2018). (B) Colorimetric sensing of Fe2+ based on its competitive effect on the TMB + O2 system catalyzed by C-dots/Mn3O4 (reproduced from ref. 59 with permission from Springer Nature, copyright 2020). | |
Another sensing strategy affecting nanozyme catalytic systems is based on the chromogenic substrates' competitive interaction with target ions, which alters the color of the solution. For example, C-dots/Mn3O4 acting as an oxidase mimic facilitates the transformation of Fe2+ into Fe3+ (Fig. 4B). Meanwhile, this oxidation reaction had a significant impact on the nanozyme-catalyzed TMB oxidation.59 Due to the competitive interaction between TMB and Fe2+, a colorimetric method for Fe2+ detection could be proposed. Furthermore, S2− can be detected in a similar manner, but the potential mechanism involves not only the competitive oxidation of S2− and TMB but also the re-reduction of TMBox to colorless TMB by S2−.60
3. Recent progress in detecting ions using nanozymes
With the rapid development of nanozymes for toxic ion detection, a large number of studies have emerged in the last few years. To summarize the cutting-edge advances in this flourishing area, the mainstream ideas and research hotspots would be analyzed and discussed in this part, mainly including improvement of nanozyme catalytic activity, expansion of enzyme-like activity types, design of multifunctional nanozymes, and diversification of detection modes (Fig. 5).
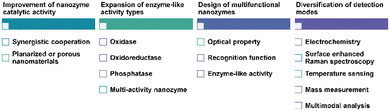 |
| Fig. 5 Recent progress in detecting ions using nanozymes. | |
3.1. Improvement of nanozyme catalytic activity
Improvement of nanozyme catalytic activity is critical to ion sensing because the activity of the nanozyme used directly decides detection sensitivity. For most of the nanomaterials with enzyme mimetic properties, their catalytic activities still have a certain gap compared with natural enzymes. As a result, one of the most important topics in this field is how to improve their catalytic activity. To date, several strategies have been proposed to efficiently improve nanozyme catalytic activity, such as size control, morphology screening, element doping, and crystal plane regulation. Here we would like to introduce the material compositing strategy and the active area increasing method to boost the activity of nanozymes, both of which have been verified to be effective in improving the analytical performance of toxic ions.
In recent years, the synergistic cooperation of different property compounds that can confer new or enhanced properties to nanozymes has attracted a great deal of research interest. Typically, Guo and coworkers successfully prepared 5,10,15,20-tetrakis(4-carboxyphenyl)porphyrin (H2TCPP)-modified bimetallic sulfide ZnS/CoS core–shell nanospheres with significantly enhanced peroxidase activity.61 It was demonstrated that the increased catalytic activity of H2TCPP/ZnS/CoS is due to the high number of active sites produced by metal cations on the surface of the nanozyme and the synergistic interaction between the various components. The synergistic interaction between porphyrin molecules and ZnS/CoS not only improves the catalytic activity of the nanozyme but also unexpectedly contributes to the selectivity of the nanozyme analysis. For example, Dai et al. achieved ultrasensitive colorimetric detection of Ag+ by synergistic interaction between different components as well as active site modulation (Fig. 6A).62 In this work, natural peat moss (PM) leaves were selected as a carbon source, enabling the in situ reduction and stabilization of Pt NPs on the formed support (carbonized peat moss, CPM). The porous structure and large surface area of CPM allowed more Pt NPs to be uniformly loaded, and the abundant active sites give the material good peroxidase activity and boost the detection performance. The analyte Ag+ could interact with Pt NPs via metallophilic interactions as well as the component synergistic effect to generate Ag atoms, thus modulating the enzyme-like activity of the hybrid and enabling the rapid detection of ultratrace Ag+ with satisfactory selectivity.
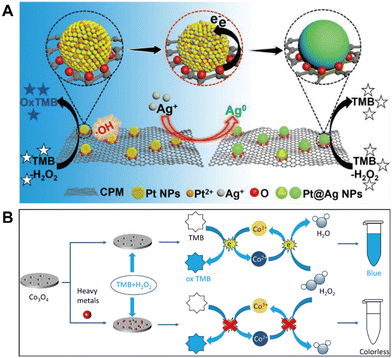 |
| Fig. 6 (A) Ag+ colorimetric assay based on the enhanced peroxidase activity of CPM-Pt NPs (reproduced from ref. 62 with permission from the American Chemical Society, copyright 2022). (B) Multi-heavy metal colorimetric detection based on target suppressed peroxidase activity of porous Co3O4 nanodisks (reproduced from ref. 63 with permission from Elsevier, copyright 2021). | |
Since the catalytic ability of nanozymes is closely related to their surface properties, designing planarized or porous nanomaterials is one of the simplest and most effective ways to increase the specific surface area, expose the active site, and thus improve the catalytic activity of nanozymes. Recently, Zou and coworkers obtained porous Co3O4 nanodisks with significantly enhanced peroxidase mimetic activity.63 With a soft template method, the obtained porous Co3O4 could show significantly improved peroxidase-mimicking activity compared to the counterpart without pores. This was because the porous Co3O4 nanodisks offered a larger area, thus exposing more active sites for catalyzing reactions. The peroxidase-like activity of porous Co3O4 nanodisks is severely inhibited by many heavy metals, including Cd(II), Hg(II), Pb(II), and arsenic. As a result, the authors constructed an ultrasensitive colorimetric sensing platform for the detection of these four heavy metals (Fig. 6B). Thanks to the nanozyme's enhanced enzyme-like activity, the colorimetric sensor could detect these metal ions with high sensitivity and reliability.
3.2. Expansion of enzyme-like activity types
The nanozyme-based sensing approach now relies mostly on peroxidase-like activity. In fact, the peroxidase-like activity has some deficiencies. Typically, high-concentration H2O2 is often required to fabricate reaction systems, while the H2O2 species itself is a potentially harmful chemical to the environment and human health. On the other hand, H2O2 is a redox agent, and the use of high-concentration H2O2 may change the form of analytes in original samples. Instead, a few studies have explored the use of oxidases,64,65 oxidoreductases,21,22 phosphatase mimics31,66 or multi-activity nanozymes24,67,68 for toxic ion detection. For instance, using ascorbic acid-coated platinum nanoparticles (AA-PtNPs) with catalase properties to catalyze the decomposition of H2O2 to produce hydrogen gas, the produced gas can expel water from the device, leading to a reduction in the weight of the device,69 and because Ag+ can inhibit the AA-PtNPs' catalytic activity and reduce gas production, it is possible to measure the Ag+ content by observing changes in the device's weight (Fig. 7A). The device can accurately measure Ag+ and AgNPs without relying on advanced instruments with minimum detection limits of 2.0 nM and 3.8 pM, respectively.
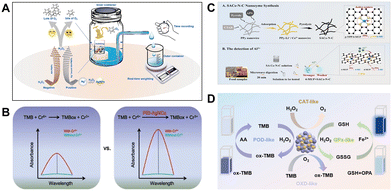 |
| Fig. 7 (A) Schematic diagram of a catalase-based weight method for the detection of Ag+ and AgNPs (reproduced from ref. 69 with permission from Elsevier, copyright 2021). (B) Colorimetric determination of Cr6+ using PEI-AgNCs as oxidoreductase mimics (reproduced from ref. 21 with permission from Springer Nature, copyright 2020). (C) Synthesis of the phosphatase-like SA Ce–N–C enzymes and detection of Al3+ (reproduced from ref. 70 with permission from Elsevier, copyright 2022). (D) Multi-target assay based on multiple enzyme activities (AA and Fe2+) (reproduced from ref. 67 with permission from the American Chemical Society, copyright 2021). | |
As mentioned above, some materials have been discovered to exhibit oxidoreductase-like activity capable of catalyzing the redox reaction between Cr6+ and TMB.23 In this regard, our group proposed an environmentally friendly and inexpensive colorimetric method for Cr6+ detection using polyethyleneimine-stabilized silver nanoclusters (PEI-AgNCs) (Fig. 7B). It was demonstrated that the PEI-AgNCs acted as neither an oxidant nor an oxidase mimic. Instead, they function as an oxidoreductase mimic, catalyzing the oxidation process between TMB and Cr6+, where electrons tended to flow from TMB to the PEI-AgNCs and then are delivered to Cr6+. As a result, the PEI-AgNCs could be regarded as an electron mediator in accelerating the redox process. Compared to the use of Au@Hg as an oxidoreductase,23 the PEI-AgNCs seem to be more attractive in terms of reduced material cost and secondary pollution risk to the environment. Instead of using any noble metal, Alula and co-workers found that magnetic Fe3O4 nanoparticles also possessed the oxidoreductase-like activity to realize the determination of Cr6+.22
Recently, some nanomaterial-based phosphatase mimics to facilitate the hydrolysis of phosphate esters have emerged for ion analysis.31,66,70 For instance, Song et al. demonstrated that single-atom Ce–N–C has good phosphatase activity,70 which could catalyze the dephosphorylation of organophosphates. Simultaneously, they discovered that Al3+ could specifically attach to the O atom in the nanozyme, inhibiting its phosphatase-like activity. Based on this principle, they constructed a facile, portable, and highly efficient fluorescent sensor to detect Al3+ in food matrices (Fig. 7C).
In natural living systems, two or more enzymes are often used to form cascade reactions that are involved in catalyzing metabolism. Researchers are developing nanozymes that can display numerous activities simultaneously under similar conditions in an effort to more closely replicate these systems. Consequently, several materials with multiple enzyme-like activities have been developed for ion determination.24,67,68 For example, Xu et al. proposed polymetallic oxide (POM)-decorated copper oxide nanoparticles (CuO NPs) with four-in-one enzyme-like activities for multi-target detection (Fig. 7D).67 Based on the fact that ascorbic acid (AA) could hinder the peroxidase-like activity of CuO NP–POM and Fe2+ could boost its glutathione peroxidase-like activity, highly sensitive colorimetric and fluorescence platforms were constructed for AA and Fe2+ sensing, respectively. In a different study, a self-cascade catalytic system was created using the nanozyme's dual-mimetic activity.68 The CuO NPs are capable of catalyzing the oxidation of glutathione to generate glutathiol (GSSG) and H2O2, exhibiting glutathione oxidase and peroxidase activities. Also, p-phenylene dicarboxylic acid (TA) can be further oxidized by the released H2O2 to provide the intensely fluorescent product TAOH. On the basis of multi-enzymatic activity, a self-cascade reaction system was developed. When Ag+ was added to the catalytic system, Ag+ ions could quench the fluorescence of the catalytic system, based on which the authors established a fluorescence method for the detection of GSH and Ag+. The use of nanozymes with multiple activities has greatly benefited the sensing field. Despite the success of developing multi-activity nanozymes in the field of analytical sensing, there are still many issues to be addressed. Because different classes of enzyme activities can be expressed simultaneously under similar conditions, it is difficult to select the appropriate enzyme activity for the test, potentially causing the assay results to be influenced. For one aspect, in multi-activity catalytic reactions, the same substrates may be involved and consumed, as well as the same intermediates produced, which can result in potentially competing reactions that will inevitably affect the test's accuracy. For example, both peroxidase- and oxidase-like mimics generate signal output by oxidizing chromogenic substrates, and oxidases can directly catalyze chromogenic reactions under oxygen conditions, which will unavoidably affect peroxidase nanozyme-based detection assays. As for another aspect, multi-active nanozymes may be more susceptible to environmental factors than single-active materials. In particular, in cascade catalytically involved sensing systems, an external element that influences the activity of the nanozyme might have a significant effect on the entire process. As a result, more variables that could have an impact on the sensing system need to be investigated, and a more stable and dependable nanozyme sensing system has to be developed.14
3.3. Design of multifunctional nanozymes
Thanks to the designability of nanomaterials, multifunctional nanozymes for analytical applications have gradually become a research hotspot in recent years. One can integrate some optical properties with enzyme-like activity into the same material.71–73 Also, both the target recognition function and the loading function can be introduced to nanozymes.74,75 As a result, the integrated multifunctional nanozymes enable not only the enhancement of detection performance but also the expansion of application scenarios.
As a typical nanozyme material widely used in biochemical analysis, metal–organic frameworks (MOFs) can play multiple roles in fabricating sensors.16 Typically, MOFs are preferred materials for encapsulating natural enzymes due to their ordered channels, stable structures and abundant functional groups. The integration of nanozymes or natural enzymes into MOFs can simplify the assay process, improve the catalytic efficiency of the loaded enzymes or nanozymes, and protect the loaded enzymes from being exposed to external interference. In this regard, our group developed Zn-based metal–organic framework nanosheets (ACP/hemin@Zn-MOF) loaded with acid phosphatase and hemin as a multifunctional sensing element for high-performance arsenate detection.78 Additionally, the plentiful and adaptable metal nodes and tailored organic ligands in MOF materials can offer different multi-signal output sources for chemical detection directly or indirectly. With reference to this advantage, our group proposed a trifunctional Fe/Zr bimetal–organic framework (UiO-66(Fe/Zr)–NH2) and constructed a ratiometric fluorescence sensing platform for phosphate ion (Pi) detection. The fluorescent 2-aminoterephthalic acid (1,4-BDC-NH2) used as an organic ligand will give the MOF material noticeable intrinsic fluorescence at 435 nm. The Fe3+/Fe2+ nodes provide good peroxidase ability for the rapid conversion of o-phenylenediamine (OPD) into an oxidation product that emits fluorescence at 555 nm (Fig. 8A), but the intrinsic fluorescence of UiO-66(Fe/Zr)–NH2 at 435 nm will be quenched by the generated OPDox through the internal filtration effect (IFE). Zr4+ nodes are not only involved in coordination to form stable MOFs structures but will also contribute to the selective detection of Pi. These tri-functional MOFs can be used to detect Pi, and when Pi is present, UiO-66(Fe/Zr)–NH2 peroxidase mimetic activity is inhibited and fluorescent OPDox yield is reduced while its intrinsic fluorescence is restored. Two fluorescence peaks can be obtained at the same excitation wavelength, independent of each other and with opposite trends, and thus can be used to construct a ratiometric fluorescence method for Pi detection, resulting in reliable detection performance.
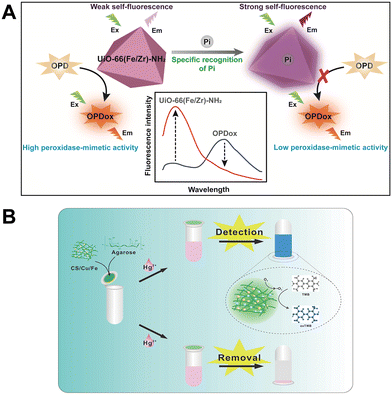 |
| Fig. 8 (A) Ratiometric fluorescent Pi sensing based on trifunctional UiO-66(Fe/Zr)–NH2 nanozyme (reproduced from ref. 76 with permission from the Royal Society of Chemistry, copyright 2020). (B) Hg2+ detection and removal process based on CS/Cu/Fe composite (reproduced from ref. 77 with permission from Elsevier, copyright 2022). | |
Apart from sensing target ions, some nanomaterials can also play a specific adsorption function to remove these toxic species simultaneously. Recently, an economical and environmentally friendly nanozyme, chitosan/copper/iron (CS/Cu/Fe), was prepared by Zou et al.77 A portable analytical tube was built by combining CS/Cu/Fe composites with good thermal stability, compatibility and oxidase-mimetic enzyme properties with agarose hydrogels (Fig. 8B). Based on the inhibition of TMB-catalyzed oxidation by cysteine and the specific interaction between cysteine and Hg2+, Hg2+ can eventually restore the CS/Cu/Fe oxidase activity, thus allowing a highly sensitive and selective colorimetric detection of Hg2+ in the analytical tube. In addition, CS/Cu/Fe composites and agarose hydrogels in analytical tubes were found to achieve efficient Hg2+ adsorption in a short period of time, making the platform a promising tool for simultaneous detection and removal of Hg2+.
3.4. Diversification of detection modes
In most of the currently developed nanozyme-based ion assays, colorimetric measurement is the dominant form. Although it is relatively simple to obtain results with such an optical method, the shortcoming of poor sensitivity hinders its use for trace analysis. To obtain highly sensitive determination or more convenient monitoring, in recent years researchers have attempted to combine nanozyme catalysis with some other detection modes for toxic ion analysis, including electrochemistry,79 surface-enhanced Raman spectroscopy (SERS),80,81 temperature sensing,31 mass measurement69 and multimodal analysis.82
Conventional electrochemical methods have high sensitivity and fast response, allowing it to detect trace heavy metal ions effectively. However, most previous methods required complicated operating procedures, relatively expensive electrode materials, and interference from coexisting metal cations. Recently, Qiu's team reported a dual-mode colorimetric/electrochemical determination of arsenate using CoOOH nanoflakes with peroxidase-like activity.79 In the presence of H2O2, colorless ABTS can be successfully converted to green oxidation products (ABTSox) thanks to the peroxidase-like activity of CoOOH nanoflakes. As(V) could specifically interact with CoOOH nanoflakes via electrostatic attraction and covalent-type interactions (Fig. 9A), significantly reducing the CoOOH peroxidase-like activity and decreasing catalytic efficiency. As a result, a colorimetric sensing method for As(V) detection based on the peroxidase-like activity of CoOOH nanoflakes can be developed. Meanwhile, ABTS can also be used as a potential electrochemical probe. By modifying CoOOH nanoflakes on the electrode surface, the redox signals of ABTS and ABTSox on the electrode surface can be output, and thus an electrochemical method for the detection of trace As(V) can be developed with significantly improved sensitivity and detection limits.
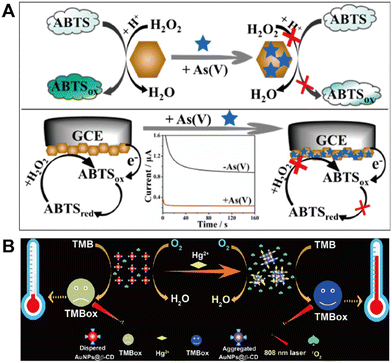 |
| Fig. 9 (A) Colorimetric/electrochemical dual-mode arsenate determination based on the peroxidase activity of CoOOH nanoflakes (reproduced from ref. 79 with permission from the American Chemical Society, copyright 2019). (B) A platform for Hg2+ temperature analysis based on the altered aggregation state of AuNPs@β-CD (reproduced from ref. 50 with permission from the Royal Society of Chemistry, copyright 2020). | |
Interestingly, Xue's team has recently developed a photothermometric system for home use, utilizing the photothermal effect of the TMBox to convert mercury detection into temperature monitoring in an innovative manner.50 As illustrated in Fig. 9B, under 808 nm laser irradiation, cyclodextrin-capped Au nanoparticles (AuNPs@β-CD) hardly oxidized the substrate TMB to TMBox with no significant temperature change. When a small amount of Hg2+ was added, AuNPs@β-CD agglomerated, resulting in a significant increase in the material's catalytic activity and the rapid formation of a blue TMBox. The material also displayed a photothermal effect, significantly raising the temperature in response to laser irradiation. Therefore, changes in the temperature of the reaction system can be recorded with an ordinary thermometer, and the quantitative determination of trace amounts of Hg2+ can be achieved.
4. Challenges and potential solutions
It is undeniable that nanozymes have become one of the most attractive materials for applications in many areas. In particular, for ion detection, nanozymes offer several potential principles for the development of sensing methods and devices. Driven by these principles, great success has been achieved in nanozyme-based ion assays. Even so, there is still plenty of room to promote the application area, including understanding the sensing mechanisms better, achieving high-selectivity detection, expanding target ions, exploring low-cost and environmentally friendly nanozymes for sensing, and developing portable detection technologies and devices.
(1) Better understanding of sensing mechanisms
Although effective detection of many toxic ions has been achieved using nanozyme catalysis, some of the detection mechanisms are ambiguous due to the complexity of nanozyme-involved processes. For example, in the redox process of substrates catalyzed by nanozymes, one often employs the electron paramagnetic resonance (EPR) technique or chemical reagents to identify the reactive radicals generated during reactions, and thus proposes that the oxidation of substrates is caused by the radicals produced from nanozyme-catalyzed H2O2 or O2, while in some cases nanozymes catalyze reactions through electron transfer. Even in the same system, the two mechanisms may coexist. In terms of regulating nanozyme activity via foreign substances, our group found that phosphate ions could promote the oxidase-like activity of oxidized UiO-66(Ce/Zr) when TMB was used as the substrate, while an inhibiting effect was found when ABTS was employed.83 The zeta potential characterization revealed that this phenomenon was closely related to the change of the electrical charge on the nanozyme surface. As a result, the substrates with different charges responded to it differently. Additionally, many studies demonstrated that Hg2+ could be reduced in situ and deposited on the surface of noble metals (Au, Ag, Pt, etc.) to form amalgams, but the generated AuHg alloy typically promoted the catalytic activity of Au-based nanozymes,84,85 while the PtHg alloy often exerted an inhibiting effect oppositely.86 The detailed reason for the different impacts has not been revealed well. Even in the same category of materials, e.g., Au-based peroxidase mimics, different activity regulation effects made by Hg2+ were observed.38,87 To better understand the above phenomena and results, advanced characterization techniques and theoretical calculations may help one uncover the underlying mechanisms.
(2) How to achieve high-selectivity detection
Despite the rapid development of nanozymes in ion sensing, their biggest weakness is the far less selectivity compared to natural enzymes, particularly when multivalent or interconvertible ions are to be measured. In many cases, the nanozyme sensing technology cannot detect target ions specifically. Therefore, there is an urgent need to explore effective methods to improve detection selectivity.10 One of the most effective strategies at present is to introduce recognition elements into nanozyme catalytic systems to possess special interactions with target ions.64,65,88–90 For instance, doping S2− or Se2− into nanozymes enables the highly selective detection of Hg2+ by taking advantage of the strong precipitation between Hg2+ and S2− or Se2−. Another appealing strategy is the use of the molecular imprinting technique, where analytes are imprinted onto the surface of nanozymes as template molecules or ions.91,92 After the template molecules or ions are eluted off, recognition sites perfectly matching the size and shape of the analytes are obtained for measurement. Finally, some efforts have been made on the differentiation and simultaneous detection of analogues by constructing nanozyme sensor arrays.93–95 Such a strategy can effectively solve the interference problem of coexisting substances.
(3) Expansion of target ions
Although many sensor systems based on nanozyme catalysis have been successfully manufactured to detect certain analytes, the type of target ions that nanozymes can selectively detect is quite limited (mainly limited to Hg2+, Pb2+, Cr6+, Ag+ and F−). This is because the type of analytes interacting with nanozymes directly or indirectly is still very limited. It is quite necessary to systematically study the influences of different analytes on the catalytic behavior of nanozymes. Encouragingly, several recent studies have expanded the detectable targets to rare earth elements.27,96 In addition to inorganic ions, some organic forms are more harmful to living organisms. Recently, detection of methylmercury has been achieved by combining nanozyme catalysis with SERS sensing.80 Future exploration should be encouraged to develop potential principles and methods for the detection of more targets.
(4) Developing low-cost and environmentally friendly nanozymes for sensing
Compared to natural enzymes, one of the most significant advantages of nanozymes is their low cost. To further reduce the cost, non-precious metal or metal-free materials are attracting increasing attention to replace noble metal-based nanozymes. Furthermore, when nanozymes are used in environmental analysis, their own toxicity cannot be overlooked. Because most of the nanozymes used in currently available sensing systems cannot be recycled and reused and some materials may have adverse effects on the environment and human health, the toxicity of nanozymes is of great concern to the academia and the public. Exploring low-cost, environmentally friendly nanozymes for toxic ion detection should be a continuing goal for the field.77,89,97
(5) Development of portable detection technologies and devices
In order to obtain mobile devices suitable for in situ analysis, integrating sensors with portable tools, such as smartphones, is an effective and easy-to-prepare strategy. Similarly, there is growing interest in combining nanozyme-based assays with low-cost test strips to create inexpensive, portable assays.64,98,99 The development of intelligent devices and test strips can help detect analytes quickly in remote areas. Currently, smartphone-based optical sensors with catalytic signal amplification are very popular in quick analysis, but this paradigm highly depends on the color change of test strips with a complicated matrix background. In some of these sensors, the detection sensitivity obtained is still low even with the help of color extraction software. It is necessary to develop sensitive and stable portable devices with the assistance of other high-sensitivity paradigms like electrochemical measurement.
5. Conclusions
To sum up, the fascinating advantages of low cost, excellent stability and easy-to-adjust performance have endowed nanozymes with extensive applications in the past few years. In particular, in the ion sensing field, several detection strategies have been explored and verified to monitor toxic heavy metals. More excitingly, increasing efforts are being paid to push forward this area by developing high-activity and multifunctional nanozymes as well as establishing new detection methods and modes. In this review, we have briefly introduced common principles for the determination of toxic ions based on nanozyme catalysis, summarized the latest developments in this area, and discussed current problems and potential solutions for toxic ion monitoring. It is believed that the work will provide guides to rationally design and develop nanozymes for biochemical sensing, especially for toxic ion analysis and control. Also, we hope that the review can attract more attention to this promising field.
List of abbreviations
1,4-BDC-NH2 | 2-Aminoterephthalic acid |
AA | Ascorbic acid |
AA-PtNPs | Ascorbic acid-coated platinum nanoparticles |
ABTS | 2,2′-Azino-bis-(3-ethylbenzthiazoline-6-sulphonate) |
ACP/hemin@Zn-MOF | Zn-based metal–organic framework nanosheets loaded with acid phosphatase and hemin |
AuNPs@β-CD | Cyclodextrin-capped Au nanoparticles |
CPM | Carbonized peat moss |
CS | Chitosan |
CuO NPs | Copper oxide nanoparticles |
Cys | Cysteine |
Cys–Fe3O4 | Cysteine-modified ferromagnetic particles |
EPR | Electron paramagnetic resonance |
GSH | Glutathione |
GSSG | Glutathiol |
H2TCPP | 5,10,15,20-Tetrakis(4-carboxyphenyl)porphyrin |
IFE | Internal filtration effect |
MOFs | Metal–organic frameworks |
OPD |
o-Phenylenediamine |
PEI-AgNCs | Polyethyleneimine-stabilized silver nanoclusters |
PM | Peat moss |
POM | Polymetallic oxide |
Pt NPs | Platinum nanoparticles |
SERS | Surface-enhanced Raman spectroscopy |
TA |
p-Phenylene dicarboxylic acid |
TMB | 3,3′,5,5′-Tetramethylbenzidine |
UiO-66(Fe/Zr)–NH2 | Fe/Zr bimetal–organic frameworks |
Author contributions
Supervision: Xiangheng Niu; writing – original draft: Xin Li and Jinjin Liu; writing – review & editing: Jia Chen, Hongdeng Qiu and Xiangheng Niu.
Conflicts of interest
There are no conflicts to declare.
Acknowledgements
The authors appreciate the support from the Start-Up Research Fund of University of South China (221RGC011) and the Fujian Key Laboratory of Functional Marine Sensing Materials, Minjiang University (MJUKF-FMSM2022010).
References
-
A. S. Mohammed, A. Kapri and R. Goel, in Biomanagement of Metal-Contaminated Soils, ed. M. Khan, A. Zaidi, R. Goel and J. Musarrat, Springer, 2011 Search PubMed.
- G. Aragay, J. Pons and A. Merkoçi, Chem. Rev., 2011, 111, 3433–3458 CrossRef CAS PubMed.
- L. Gao, J. Zhuang, L. Nie, J. Zhang, Y. Zhang, N. Gu, T. Wang, J. Feng, D. Yang, S. Perrett and X. Yan, Nat. Nanotechnol., 2007, 2, 577–583 CrossRef CAS PubMed.
- H. Wei and E. Wang, Chem. Soc. Rev., 2013, 42, 6060–6093 RSC.
- J. Wu, X. Wang, Q. Wang, Z. Lou, S. Li, Y. Zhu, L. Qin and H. Wei, Chem. Soc. Rev., 2019, 48, 1004–1076 RSC.
- Q. Wang, H. Wei, Z. Zhang, E. Wang and S. Dong, TrAC Trends Anal. Chem., 2018, 105, 218–224 CrossRef CAS.
- Y. Huang, J. Ren and X. Qu, Chem. Rev., 2019, 119, 4357–4412 CrossRef CAS PubMed.
- M. Liang and X. Yan, Acc. Chem. Res., 2019, 52, 2190–2200 CrossRef CAS PubMed.
- X. Li, L. Wang, D. Du, L. Ni, J. Pan and X. Niu, TrAC Trends Anal. Chem., 2019, 120, 115653 CrossRef CAS.
- X. Li, H. Zhu, P. Liu, M. Wang, J. Pan, F. Qiu, L. Ni and X. Niu, TrAC Trends Anal. Chem., 2021, 143, 116379 CrossRef CAS.
- C. Gao, H. Zhu, J. Chen and H. Qiu, Chin. Chem. Lett., 2017, 28, 1006–1012 CrossRef CAS.
- Y. Liu, X. Wei, J. Chen, Y. L. Yu, J. H. Wang and H. Qiu, Anal. Chem., 2022, 94, 5970–5979 CrossRef CAS PubMed.
- X. Niu, N. Cheng, X. Ruan, D. Du and Y. Lin, J. Electrochem. Soc., 2020, 167, 037508 CrossRef CAS.
- X. Niu, B. Liu, P. Hu, H. Zhu and M. Wang, Biosensors, 2022, 12, 251 CrossRef CAS PubMed.
- J. Liu and X. Niu, Chemosensors, 2022, 10, 386 CrossRef CAS.
- X. Niu, X. Li, Z. Lyu, J. Pan, S. Ding, X. Ruan, W. Zhu, D. Du and Y. Lin, Chem. Commun., 2020, 56, 11338–11353 RSC.
- H. Zhang, J. Chen, Y. Yang, L. Wang, Z. Li and H. Qiu, Anal. Chem., 2019, 91, 5004–5010 CrossRef CAS PubMed.
-
X. Li, P. Liu, M. Wang and X. Niu, in Nanozymes: Advances and Applications, ed. S. Gunasekaran, CRC Press, 2022 Search PubMed.
- Z. Yan, H. Yuan, Q. Zhao, L. Xing, X. Zheng, W. Wang, Y. Zhao, Y. Yu, L. Hu and W. Yao, Analyst, 2020, 145, 3173–3187 RSC.
- B. Unnikrishnan, C. W. Lien, H. W. Chu and C. C. Huang, J. Hazard. Mater., 2021, 401, 123397 CrossRef CAS PubMed.
- Q. Xue, X. Li, Y. Peng, P. Liu, H. Peng and X. Niu, Microchim. Acta, 2020, 187, 263 CrossRef CAS PubMed.
- M. T. Alula and M. L. Madingwane, Sens. Actuators, B, 2020, 324, 128726 CrossRef CAS.
- X. Zhang, W. Liu, X. Li, Z. Zhang, D. Shan, H. Xia, S. Zhang and X. Lu, Anal. Chem., 2018, 90, 14309–14315 CrossRef CAS PubMed.
- C. Liu, Y. Yan, X. Zhang, Y. Mao, X. Ren, C. Hu, W. He and J. J. Yin, Nanoscale, 2020, 12, 3068–3075 RSC.
- Y. Wang, J. Yang, Y. Zhao and J. Liu, Chem. Commun., 2019, 55, 13434–13437 RSC.
- D. Peng, R. P. Liang, J. D. Qiu and J. Liu, J. Anal. Test., 2019, 3, 260–268 CrossRef.
- Z. Jiang, H. Li, R. Ai, Y. Deng and Y. He, ACS Sustainable Chem. Eng., 2020, 8, 11630–11637 CrossRef CAS.
- C. Ma, Y. Ma, Y. Sun, Y. Lu, E. Tian, J. Lan, J. Li, W. Ye and H. Zhang, J. Colloid Interface Sci., 2019, 537, 554–561 CrossRef CAS PubMed.
- X. Niu, Y. He, X. Li, H. Zhao, J. Pan, F. Qiu and M. Lan, Sens. Actuators, B, 2019, 281, 445–452 CrossRef CAS.
- Y. Fang, Y. Zhang, L. Cao, J. Yang, M. Hu, Z. Pang and J. He, ACS Appl. Mater. Interfaces, 2020, 12, 11761–11768 CrossRef CAS PubMed.
- X. Tian, H. Liao, M. Wang, L. Feng, W. Fu and L. Hu, Biosens. Bioelectron., 2020, 152, 112027 CrossRef CAS PubMed.
- G. W. Wu, S. B. He, H. P. Peng, H. H. Deng, A. L. Liu, X. H. Lin, X. H. Xia and W. Chen, Anal. Chem., 2014, 86, 10955–10960 CrossRef CAS PubMed.
- Y. Zhou and Z. Ma, Sens. Actuators, B, 2017, 249, 53–58 CrossRef CAS.
- A. J. Kora and L. Rastogi, Sens. Actuators, B, 2018, 254, 690–700 CrossRef CAS.
- W. Chen, X. Fang, H. Li, H. Cao and J. Kong, Sci. Rep., 2016, 6, 31948 CrossRef CAS PubMed.
- C. Song, J. Li, Y. Sun, X. Jiang, J. Zhang, C. Dong and L. Wang, Sens. Actuators, B, 2020, 310, 127849 CrossRef CAS.
- H. Li, H. Liu, J. Zhang, Y. Cheng, C. Zhang, X. Fei and Y. Xian, ACS Appl. Mater. Interfaces, 2017, 9, 40716–40725 CrossRef CAS PubMed.
- R. Zhu, Y. Zhou, X. L. Wang, L. P. Liang, Y. J. Long, Q. L. Wang, H. J. Zhang, X. X. Huang and H. Z. Zheng, Talanta, 2013, 117, 127–132 CrossRef CAS PubMed.
- W. Liu, L. Tian, J. Du, J. Wu, Y. Liu, G. Wu and X. Lu, Analyst, 2020, 145, 5500–5507 RSC.
- M. Yin, Y. Wan, S. Li, X. Zhao, W. Zhang, Y. Zhang and H. Wang, J. Hazard. Mater., 2021, 408, 124978 CrossRef CAS PubMed.
- P. Ju, Z. Wang, Y. Zhang, X. Zhai, F. Jiang, C. Sun and X. Han, Colloids Surf., A, 2020, 603, 125203 CrossRef CAS.
- L. Kong, C. Wang, W. Yang, L. Zhou and S. Wei, J. Hazard. Mater., 2022, 427, 128135 CrossRef CAS PubMed.
- Q. Lian, H. Liu, X. Zheng, X. Li, F. Zhang and J. Gao, Appl. Surf. Sci., 2019, 483, 551–561 CrossRef CAS.
- W. Zhang, X. Niu, S. Meng, X. Li, Y. He, J. Pan, F. Qiu, H. Zhao and M. Lan, Sens. Actuators, B, 2018, 273, 400–407 CrossRef CAS.
- N. Li, Y. He, J. Lian, Q. Y. Liu, Y. X. Zhang and X. Zhang, Inorg. Chem., 2020, 59, 18384–18395 CrossRef CAS PubMed.
- Q. Zhao, W. Gou, X. Zhang, M. Zhang, Y. Bu, L. Wang, L. Hu, W. Yao and Z. Yan, Appl. Surf. Sci., 2021, 545, 148973 CrossRef CAS.
- P. Borthakur, G. Darabdhara, M. R. Das, R. Boukherroub and S. Szunerits, Sens. Actuators, B, 2017, 244, 684–692 CrossRef CAS.
- P. Borthakur, P. K. Boruah and M. R. Das, ACS Sustainable Chem. Eng., 2021, 9, 13245–13255 CrossRef CAS.
- H. Rao, X. Xue, M. Luo, H. Liu and Z. Xue, Chin. Chem. Lett., 2021, 32, 25–32 CrossRef CAS.
- P. An, H. Rao, M. Gao, X. Xue, X. Liu, X. Lu and Z. Xue, Chem. Commun., 2020, 56, 9799–9802 RSC.
- Q. Xue, X. Niu, P. Liu, M. Wang, Y. Peng, H. Peng and X. Li, Sens. Actuators, B, 2021, 334, 129650 CrossRef CAS.
- A. A. B. Christus, A. Ravikumar, P. Panneerselvam and K. Radhakrishnan, Appl. Surf. Sci., 2018, 449, 669–676 CrossRef CAS.
- J. Mu, J. Li, X. Zhao, E. C. Yang and X. J. Zhao, Sens. Actuators, B, 2018, 258, 32–41 CrossRef CAS.
- A. A. B. Christus, P. Panneerselvam, A. Ravikumar, N. Morad and S. Sivanesan, J. Photochem. Photobiol., A, 2018, 364, 715–724 CrossRef CAS.
- R. Li, X. He, R. Javed, J. Cai, H. Cao, X. Liu, Q. Chen, D. Ye and H. Zhao, Sci. Total Environ., 2022, 834, 155428 CrossRef CAS PubMed.
- L. L. Wu, Z. J. Qian, Z. J. Xie, Y. Y. Zhang and C. F. Peng, Chin. J. Anal. Chem., 2017, 45, 471–476 CAS.
- Y. Mao, S. Gao, L. Yao, L. Wang, H. Qu, Y. Wu, Y. Chen and L. Zheng, J. Hazard. Mater., 2021, 408, 124898 CrossRef CAS PubMed.
- X. Li, B. Liu, K. Ye, L. Ni, X. Xu, F. Qiu, J. Pan and X. Niu, Sens. Actuators, B, 2019, 297, 126822 CrossRef CAS.
- F. Honarasa, F. Peyravi and H. Amirian, J. Iran. Chem. Soc., 2020, 17, 507–512 CrossRef CAS.
- X. Liu, L. Huang, Y. Wang, J. Sun, T. Yue, W. Zhang and J. Wang, Sens. Actuators, B, 2020, 306, 127565 CrossRef CAS.
- X. Guo, F. Yang, L. Jing, J. Li, Y. Li, R. Ding, B. Duan and X. Zhang, J. Hazard. Mater., 2022, 431, 128621 CrossRef CAS PubMed.
- F. Dai, M. Xie, Y. Wang, L. Zhang, Z. Zhang and X. Lu, Anal. Chem., 2022, 94, 10462–10469 CrossRef CAS PubMed.
- W. Zou, Y. Tang, H. Zeng, C. Wang and Y. Wu, J. Hazard. Mater., 2021, 417, 125994 CrossRef CAS PubMed.
- L. Luo, C. Xi, J. Zhuo, G. Liu, S. Yang, Y. Nian, J. Sun, M. Q. Zhu and J. Wang, Biosens. Bioelectron., 2022, 215, 114519 CrossRef CAS PubMed.
- Y. Chang, M. Liu and J. Liu, Anal. Chem., 2020, 92, 3118–3124 CrossRef CAS PubMed.
- Y. Xiong, L. Su, F. Ye and S. Zhao, ACS Appl. Bio Mater., 2021, 4, 6962–6973 CrossRef CAS PubMed.
- Y. Xu, P. Li, X. Hu, H. Chen, Y. Tang, Y. Zhu, X. Zhu, Y. Zhang, M. Liu and S. Yao, ACS Appl. Nano Mater., 2021, 4, 8302–8313 CrossRef CAS.
- L. He, Y. Lu, X. Gao, P. Song, Z. Huang, S. Liu and Y. Liu, ACS Sustainable Chem. Eng., 2018, 6, 12132–12139 CrossRef CAS.
- X. Ma, Z. Wang, X. Hu, J. Chen, H. Zhang, X. Li, F. Xie and J. Xu, J. Hazard. Mater., 2021, 415, 125689 CrossRef CAS PubMed.
- G. Song, J. C. Li, Z. Majid, W. Xu, X. He, Z. Yao, Y. Luo, K. Huang and N. Cheng, Food Chem., 2022, 390, 133127 CrossRef CAS PubMed.
- L. Wang and Y. Chen, ACS Appl. Mater. Interfaces, 2020, 12, 8351–8358 CrossRef CAS PubMed.
- T. Lin, Y. Qin, Y. Huang, R. Yang, L. Hou, F. Ye and S. Zhao, Chem. Commun., 2018, 54, 1762–1765 RSC.
- J. Wu, K. Qin, D. Yuan, J. Tan, L. Qin, X. Zhang and H. Wei, ACS Appl. Mater. Interfaces, 2018, 10, 12954–12959 CrossRef CAS PubMed.
- Z. Zhao, Y. Huang, W. Liu, F. Ye and S. Zhao, ACS Sustainable Chem. Eng., 2020, 8, 4481–4488 CrossRef CAS.
- H. Shen, Z. Zhou, W. He, H. Chao, P. Su, J. Song and Y. Yang, ACS Appl. Mater. Interfaces, 2021, 13, 14995–15007 CrossRef CAS PubMed.
- X. Li, P. Liu, X. Niu, K. Ye, L. Ni, D. Du, J. Pan and Y. Lin, Nanoscale, 2020, 12, 19383–19389 RSC.
- Y. Zou, Q. Chai, T. Zhu, X. Yu, G. Mao, N. Li, J. Chen and G. Lai, Spectrochim. Acta, Part A, 2022, 266, 120410 CrossRef CAS PubMed.
- X. Xu, Z. Luo, K. Ye, X. Zou, X. Niu and J. Pan, J. Hazard. Mater., 2021, 412, 124407 CrossRef CAS PubMed.
- S. H. Wen, X. L. Zhong, Y. D. Wu, R. P. Liang, L. Zhang and J. D. Qiu, Anal. Chem., 2019, 91, 6487–6497 CrossRef CAS PubMed.
- H. Liu, Y. Guo, Y. Wang, H. Zhang, X. Ma, S. Wen, J. Jin, W. Song, B. Zhao and Y. Ozaki, J. Hazard. Mater., 2021, 405, 124642 CrossRef CAS PubMed.
- S. Wen, Z. Zhang, Y. Zhang, H. Liu, X. Ma, L. Li, W. Song and B. Zhao, ACS Sustainable Chem. Eng., 2020, 8, 11906–11913 CrossRef CAS.
- X. Li, S. Ding, Z. Lyu, P. Tieu, M. Wang, Z. Feng, X. Pan, Y. Zhou, X. Niu, D. Du, W. Zhu and Y. Lin, Small, 2022, 18, 2203001 CrossRef CAS PubMed.
- X. Li, X. Niu, P. Liu, X. Xu, D. Du and Y. Lin, Sens. Actuators, B, 2020, 321, 128546 CrossRef CAS.
- Y. J. Long, Y. F. Li, Y. Liu, J. J. Zheng, J. Tang and C. Z. Huang, Chem. Commun., 2011, 47, 11939–11941 RSC.
- L. Zhi, W. Zuo, F. Chen and B. Wang, ACS Sustainable Chem. Eng., 2016, 4, 3398–3408 CrossRef CAS.
- W. Li, B. Chen, H. Zhang, Y. Sun, J. Wang, J. Zhang and Y. Fu, Biosens. Bioelectron., 2015, 66, 251–258 CrossRef CAS PubMed.
- C. Jiang, Z. Li, Y. Wu, W. Guo, J. Wang and Q. Jiang, Bull. Korean Chem. Soc., 2018, 39, 625–630 CrossRef CAS.
- J. Lian, P. Liu and Q. Liu, J. Hazard. Mater., 2022, 433, 128766 CrossRef CAS PubMed.
- L. Huang, Q. Zhu, J. Zhu, L. Luo, S. Pu, W. Zhang, W. Zhu, J. Sun and J. Wang, Inorg. Chem., 2019, 58, 1638–1646 CrossRef CAS PubMed.
- Y. Liu, D. Ding, Y. Zhen and R. Guo, Biosens. Bioelectron., 2017, 92, 140–146 CrossRef CAS PubMed.
- Y. Zhang, Y. S. Feng, X. H. Ren, X. W. He, W. Y. Li and Y. K. Zhang, Biosens. Bioelectron., 2022, 196, 113718 CrossRef CAS PubMed.
- B. Liu, H. Zhu, R. Feng, M. Wang, P. Hu, J. Pan and X. Niu, Sens. Actuators, B, 2022, 370, 132451 CrossRef CAS.
- L. Wang, X. Xu, P. Liu, M. Wang, X. Niu and J. Pan, Anal. Chim. Acta, 2021, 1160, 338451 CrossRef CAS PubMed.
- L. Qin, X. Wang, Y. Liu and H. Wei, Anal. Chem., 2018, 90, 9983–9989 CrossRef CAS PubMed.
- Y. Guan, Y. Lu, J. Sun, J. Zhao, W. Huang, X. Zhang and Y. Liu, ACS Sustainable Chem. Eng., 2021, 9, 9802–9812 CrossRef CAS.
- H. H. Deng, B. Y. Luo, S. B. He, R. T. Chen, Z. Lin, H. P. Peng, X. H. Xia and W. Chen, Anal. Chem., 2019, 91, 4039–4046 CrossRef CAS PubMed.
- H. Tian, J. Liu, J. Guo, L. Cao and J. He, Talanta, 2022, 242, 123320 CrossRef CAS PubMed.
- P. Hu, C. Xia, B. Liu, R. Feng, M. Wang, H. Zhu and X. Niu, Talanta, 2023, 253, 124055 CrossRef CAS.
- X. Li, B. Liu, Z. Hu, P. Liu, K. Ye, J. Pan and X. Niu, Environ. Res., 2020, 189, 109921 CrossRef CAS PubMed.
|
This journal is © The Royal Society of Chemistry 2023 |
Click here to see how this site uses Cookies. View our privacy policy here.