DOI:
10.1039/D3SC03052K
(Edge Article)
Chem. Sci., 2023,
14, 9533-9542
From H12C4N2CdI4 to H11C4N2CdI3: a highly polarizable CdNI3 tetrahedron induced a sharp enhancement of second harmonic generation response and birefringence†
Received
15th June 2023
, Accepted 17th August 2023
First published on 17th August 2023
Abstract
In this study, we identify a novel class of second-order nonlinear optical (NLO) crystals, non-π-conjugated piperazine (H10C4N2, PIP) metal halides, represented by two centimeter-sized, noncentrosymmetric organic–inorganic metal halides (OIMHs), namely H12C4N2CdI4 (P212121) and H11C4N2CdI3 (Cc). H12C4N2CdI4 is the first to be prepared, and its structure contains a CdI4 tetrahedron, which led to a poor NLO performance, including a weak and non-phase-matchable second harmonic generation (SHG) response of 0.5 × KH2PO4 (KDP), a small birefringence of 0.047 @1064 nm and a narrow bandgap of 3.86 eV. Moreover, H12C4N2CdI4 is regarded as the model compound, and we further obtain H11C4N2CdI3via the replacement of CdI4 with a highly polarizable CdNI3 tetrahedron, which results in a sharp enhancement of SHG response and birefringence. H11C4N2CdI3 exhibits a promising NLO performance including 6 × KDP, 4.10 eV, Δn = 0.074 @1064 nm and phase matchability, indicating that it is the first OIMH to simultaneously exhibit strong SHG response (>5 × KDP) and a wide bandgap (>4.0 eV). Our work presents a novel direction for designing high-performance NLO crystals based on organic–inorganic halides and provides important insights into the role of the hybridized tetrahedron in enhancing the SHG response and birefringence.
Introduction
Nonlinear optical (NLO) materials, particularly second harmonic generation (SHG) crystals, offer effective ways for tuning the laser wavelength and expanding its spectral range.1–6 Traditionally, high-quality second-order NLO crystals must satisfy several fundamental conditions, such as exhibiting a noncentrosymmetric structure, possessing a strong SHG effect, having a wide bandgap and transmission window, featuring appropriate birefringence for phase matchability, and having a conducive crystal growth method.7–10 To date, several commercialized NLO crystals have been used, including KBe2BO3F2 (KBBF), β-BaB2O4 (BBO), KH2PO4 (KDP), KTiOPO4 (KTP), AgGaS2 (AGS), and α-LiIO3.
Over the past few years, interest in the development of semi-organic NLO crystals has been steadily increasing. These crystals feature many advantages such as the availability of a wide range of organic components, tunability of inorganic structures, and ease of single crystal growth.11–15 Notably, the compounds containing π-conjugated organic moieties have attracted the most attention, and many promising NLO materials in such classes have been reported, such as KLi(HC3N3O3)·2H2O (5.3 × KDP),11 Lu5(C3N3O3)(OH)12 (4.2 × KDP),12 C(NH2)3SO3F (5 × KDP),13 (C5H6ON)+(H2PO4)− (3 × KDP),14 and [o-C5H4NHOH]2[I7O18(OH)]·3H2O (8.5 × KDP).15 Alongside these compounds, several organic–inorganic metal halides (OIMHs) formed from the combination of a π-conjugated organic cation with a metal-halide polyhedron have also been discovered to show NLO performance, such as (H7C3N6)(H6C3N6)MCl3 (M = Hg and Zn; 5 × and 2.8 × KDP),16,17 [C18H21N4][AgX3]X (X = Cl, Br, and I, 6.2, 6.5 and 7.6 × KDP),18 α-(CN3H6)3Cu2I5 (1.8 × KDP),19 and (C6H11N2)PbBr3 (8 × KDP).20
It is also worth studying the NLO properties of non-π-conjugated OIMHs, which is similar to the approach of expanding the chemical system from π-conjugated borates to non-π-conjugated phosphates or sulfates.21–27 Traditionally, the introduction of metal cations with stereo-chemically active lone-pair electrons (SCALP cation, e.g. Bi3+, Pb2+, and Ge2+) or d10 transition metal cations (d10-TM cation, e.g. Zn2+ and Cd2+) can effectively design novel SHG-active non-π-conjugated OIMHs.28–34 However, on the one hand, the use of SCLAP cations is known to cause red shifts in absorption edges and reduce bandgaps of the resulting crystals, limiting their potential applications in the ultraviolet (UV) and deep-UV regions.35–40 For instance, Mao's group recently reported a series of Ge2+-containing OIMHs, in which (CH3NH3)GeBr3 shows a large SHG response (5.3 × KDP) but a small bandgap (2.91 eV). There are also similar cases to C4H9NOBiBr7 (2.2 × urea, 2.67 eV) and (C6H5(CH2)4NH3)4BiI7·H2O (1.3 × AGS, 2.29 eV).41 On the other hand, many non-π-conjugated OIMHs with d10-TM cations, such as (C4H10NO)2Cd2Cl6,42 (L/D-C10H20N2O4)2Cd5Cl12,43L/D-C6H10N3O2ZnBr3,44 [C5H14NO]CdCl3,45L/D-C12H20N6O4Cd2Cl5,44 and ((CH3)3NCH2Cl)CdCl3,46 usually possess wide bandgaps (>5.0 eV) but relatively weak SHG effects (<1.0 × KDP). Hence, it is challenging to develop NLO OIMHs that simultaneously exhibit both a strong SHG effect (>5 × KDP) and wide bandgap (>4.0 eV).
To overcome this challenge, our efforts are focused on the non-π-conjugated organic moiety-Cd2+/Zn2+-I− system. In the beginning, a noncentrosymmetric OIMH, H12C4N2CdI4, has been hydrothermally prepared. But our experimental results show that this compound has a small bandgap (3.86 eV) along with a weak and non-phase-matchable SHG response (0.5 × KDP). Such an undesired NLO performance could be attributed to the high symmetry and weak anisotropy of the CdI4 tetrahedron. To the best of our knowledge, this phenomenon is reminiscent of metal sulfates based on a tetrahedral SO42− unit. And in metal sulfates, one effective route to overcome this problem is to partially substitute O atoms in SO42−, as seen in LiSO3F,47 Na10Cd(NO3)4(SO3S)4,48 Ba(NH2SO3)2,49 Ba(SO3CH3)2,50 and so on. Hence, furthermore, H12C4N2CdI4 is regarded as the model compound, and we consider that the coordination of H10C4N2 with a Cd2+ cation could result in the partial substitution of I− anions in the CdI4 tetrahedron, forming a polarizable Cd–I–N unit, which could enhance the SHG response and birefringence in new OIMHs. Additionally, we also hope that new compound involves a smaller number of I− anions in order to exhibit a wider bandgap. Therefore, a novel OIMH, namely H11C4N2CdI3, is prepared via regulating the synthetic conditions of H12C4N2CdI4 (a lower concentration of I− anions in the reaction system). H11C4N2CdI3 contains a distorted CdNI3 tetrahedron and demonstrates superior NLO performance in comparison to H12C4N2CdI4. Specifically, H11C4N2CdI3 exhibits a strong and phase-matchable SHG effect (6 × KDP), large birefringence (Δn = 0.062 @1064 nm), and a wide bandgap (4.10 eV), indicating its potential as a promising ultraviolet NLO material.
Methods
Materials and synthesis
CdO (>99%), Y2O3 (>99%), and piperazine (>99%), HI (55–58% wt), were used as purchased from Adamas-beta. There are different synthesis conditions of H12C4N3CdI4 and H11C4N2CdI3 (Table S1†), and the best conditions (larger size and higher yields) are mentioned as follows. For the preparation of H12C4N2CdI4, the starting materials are CdO, (256.8 mg, 2 mmol), piperazine (86.14 mg, 1 mmol), HI (2 mL) and H2O (1 mL). A mixture of the starting materials was placed in Teflon pouches (23 mL) sealed in an autoclave which was heated at 90 °C for 72 hours, and cooled to 30 °C at 1.67 °C h−1. Yellow block-shaped crystals of H12C4N2CdI4 were obtained in high yields of ∼95% (based on Cd). For the preparation of H11C4N2CdI3, the starting materials are CdO, (128.4 mg, 1 mmol), Y2O3, (112.5 mg, 0.5 mmol), piperazine (172.28 mg, 2 mmol), HI (1 mL) and H2O (2 mL). A mixture of the starting materials was placed in Teflon pouches (23 mL) sealed in an autoclave which was heated at 110 °C for 72 hours, and cooled to 30 °C at 1.67 °C h−1. Yellow block-shaped crystals of H11C4N2CdI3 were obtained in high yields of ∼95% (based on Cd).
Single crystal structure determination
Single-crystal X-ray diffraction data for the title compounds were collected on an Xcalibur, Eos, Gemini diffractometer with Mo Kα radiation (λ = 0.71073 Å) at 293 K and a Rigaku XtaLAB Synergy-DW dual-wavelength CCD diffractometer with Cu Kα radiation (λ = 1.54184 Å) at 109 K. Data reduction was performed with CrysAlisPro, and absorption correction based on the multi-scan method was applied.51 The structures of H12C4N2CdI4 and H11C4N2CdI3 were determined by the direct methods and refined by full-matrix least-squares fitting on F2 using SHELXL-2014.52 All of the non-hydrogen atoms were refined with anisotropic thermal parameters. The structure was checked for missing symmetry elements using PLATON, and none were found.53 The Flack parameters refined for H12C4N2CdI4 and H11C4N2CdI3 are 0.45(12) and −0.01(2), respectively, indicating the correctness of their absolute structures. In addition, twinning was observed in H12C4N2CdI4 and H11C4N2CdI3, and the twin laws of these compounds were (−1.0, 0.0, 0.0, 0.0, −1.0, 0.0, 0.0, 0.0, −1.0) and (−1.0, 0.0, 0.0, 0.0, −1.0, 0.0, 0.0, 0.0, −1.0), respectively. Crystallographic data and structural refinements of the compounds are listed in Tables S2–S6.†
Powder X-ray diffraction
Powder X-ray diffraction (PXRD) patterns were recorded on a Rigaku Ultima IV diffractometer with graphite-monochromated Cu Kα radiation in the 2θ range of 10–70° with a step size of 0.02°.
Thermal analysis
Thermogravimetric analysis (TGA) was performed with a Rigaku TG-DTA 8121 unit under an Ar atmosphere, at a heating rate of 10 °C min−1 in the range from 30 to 800 °C.
Optical measurements
The infrared (IR) spectrum was recorded on a Thermo Fisher Nicolet 5700 FT-IR spectrometer in the form of KBr pellets in the range from 4000 to 400 cm−1.
The ultraviolet-visible-infrared (UV-vis-IR) spectrum in the range of 200–800 nm was recorded on a PerkinElmer Lambda 750 UV-vis-NIR spectrophotometer. The reflectance spectrum was converted into an absorption spectrum by using the Kubelka–Munk function.54
Second harmonic generation measurements
Powder SHG measurements were carried out with a Q switch Nd:YAG laser generating radiation at 1064 nm according to the method of Kurtz and Perry.55 Crystalline H12C4N2CdI4 and H11C4N2CdI3 samples were sieved into distinct particle-size ranges (50–70, 70–100, 100–140, 140–200, 200–250 and 250–325 μm). Sieved KH2PO4 (KDP) samples in the same particle-size ranges were used as references.
Elemental analysis
The elemental content was measured on a Vario EL Cube elemental analyzer from Elementar Analysensysteme GmbH, Germany. The combustion temperature was 800 °C (Table S7†).
Energy-dispersive X-ray spectroscopy
Microprobe elemental analyses were performed and the elemental distribution maps were obtained on a field-emission scanning electron microscope (Phenom LE) equipped with an energy-dispersive X-ray spectroscope (EDS, Phenom LE).
Computational method
The electronic structures and optical properties were obtained using a plane-wave pseudopotential method within density functional theory (DFT) implemented in the total energy code CASTEP.56,57 For the exchange and correlation functional, we chose Perdew–Burke–Ernzerhof (PBE) in the generalized gradient approximation (GGA).58 The interactions between the ionic cores and the electrons were described by the norm-conserving pseudopotential.59 The following orbital electrons were treated as valence electrons: I-5s25p5, Cd-4s24p65s2, H-1s1, C-2s22p2 and N-2s22p3. The numbers of plane waves included in the basis sets were determined at a cutoff energy of 700 eV. During the SCF and optical-property calculations of the two compounds, the k-point separation was set to 0.04 Å−1 to perform the numerical integration of the Brillouin zone, and the corresponding k-point samplings are 3 × 2 × 2 and 2 × 4 × 2 for H12C4N2CdI4 and H11C4N2CdI3, respectively. After the principal axis transformation, the k-point sampling of H11C4N2CdI3 changes to 2 × 3 × 2 when the k-point separation is 0.04 Å−1. The other parameters and convergent criteria were the default values of the CASTEP code.56,57
The calculations of second-order NLO susceptibilities were based on length-gauge formalism within the independent particle approximation.60,61 The second-order NLO susceptibility can be expressed as
χabcL(−2ω; ω, ω) = χabc inter (−2ω; ω, ω) + χabc inter(−2ω; ω, ω) + χabc mod(−2ω; ω, ω) |
where the subscript
L denotes the length gauge, and
χabc inter,
χabc intra and
χabc mod give the contributions to
χabcL from interband processes, intraband processes, and the modulation of interband terms by intraband terms, respectively.
The convergence test of the SHG coefficient upon k-point sampling and empty bands with H11C4N2CdI3 as an example was carried out (Table S9†), and we found that the SHG coefficient gradually converges when the k-point separation is not more than 0.04 Å−1 (2 × 3 × 2) and the quantity of the empty bands is not less than 2 times that of valence bands (280 bands). So the choice of k-point sampling and the empty band number during the optical property calculation is reasonable.
Results and discussion
In order to investigate the differences in the synthesis conditions of H12C4N3CdI4 and H11C4N2CdI3, we conducted multiple sets of control experiments as shown in Table S1.† Several observations were made: (1) the molar ratio of Cd to H10C4N2 had no impact on whether H12C4N3CdI4 or H11C4N2CdI3 was produced as the final product; (2) changes in temperature had minimal effects on the synthesis of H12C4N3CdI4; (3) the ratio of HI to H2O was the determining factor in the preparation of H12C4N3CdI4 or H11C4N2CdI3. We hypothesize that a lower HI
:
H2O ratio can reduce the concentration of iodide ions in the reaction system, thus facilitating direct coordination bonding between Cd2+ cations and H10C4N2, resulting in the formation of H11C4N2CdI3; (4) in the preparation process of H11C4N2CdI3, Y2O3 is essential and cannot be omitted. However, indeed, the specific role of Y2O3 in the synthesis process of H11C4N2CdI3 is currently unclear due to limitations in our current technological means. Their measured powder XRD patterns are consistent with those of simulated data, confirming the phase purity (Fig. S1†). The EDS results of the Cd
:
I ratios are 1.0
:
4.10 and 1.0
:
2.97 for H12C4N2CdI4 and H11C4N2CdI3, respectively (Fig. S2†). In addition, elemental analysis of C, N, and H atoms is provided in Table S6† with weight%: C 6.77, H 1.63, and N 4.02 for H12C4N2CdI4; C 8.30, H 1.85, and N 4.53 for H11C4N2CdI3 (Table S7†). Both of them are in good agreement with the crystal structure solution.
Crystal structure
H12C4N2CdI4 crystallizes in a noncentrosymmetric space group P212121 (No. 19) with one cationic H12C2N42+ (H2PIP2+) six-membered ring and one anionic CdI42− tetrahedron in each asymmetric unit (Fig. 1). In the H12C4N2-ring, each C and N atom exhibits sp3 hybridization, with C–N/C–C distances of 1.49(2)–1.50(2) Å and 1.474(17)–1.496(18) Å, respectively. The bond angles in the H12C4N2-ring range from 110.5(12)–111.2(11)°, which is consistent with those of previously reported piperazine compounds.62,63 As a result, the H12C2N42+ cation takes on a chair conformation, rather than a planar π-conjugated structure. Each Cd2+ cation bonds with four I− anions to form a CdI4 tetrahedron, with Cd–I distances ranging from 2.7548(14)–2.8089(14) Å and I–Cd–I angles of 104.50(4)–113.75(5)°. Neighboring H12C2N4 and CdI4 groups are isolated from each other and arranged in a quasi-two-dimensional (quasi-2D) [H12C4N2CdI4] layer (Fig. 1c), parallel to the ac plane. These pseudo-layers stack along the b direction to form the entire 3D network of H12C4N2CdI4 (Fig. 1d).
 |
| Fig. 1 Views of the structures of the CdI4 tetrahedron (a), the chair shaped H2PIP2+ cation (b), the quasi-2D H12C2N4CdI4 layer along the b-axis (c) and H12C2N4CdI4 along the a-axis (d). | |
H11C4N2CdI3 crystallizes in the polar space group Cc (No. 9), with an asymmetric unit including one protonated-piperazine group, one Cd, and three I atoms (Fig. 2a). In the chair-shaped H11C4N2-ring, C–N and C–C distances are 1.49(2)–1.532(17) Å and 1.462(17)–1.509(19) Å, respectively, and the bond angles are in the range of 108.7(11)–114.5(9)°, which are consistent with those of previously reported piperazine compounds.62,63 Each Cd2+ cation connects with one N atom and three I atoms to form a N-hybrid polyhedron, a CdNI3 tetrahedron. The length of Cd–N (2.300(11) Å) is smaller than those of Cd–I (2.7393(12)–2.7518(11) Å), and the bond angles of N–Cd–I and I–Cd–I are 100.8(3)–104.0(3)° and 112.80(3)–117.07(4)°, respectively. Furthermore, one H11C4N2-ring is connected with one CdNI3 tetrahedron via the corner-sharing of N atoms, forming a H11C4N2CdI3 molecule (Fig. 2a). Neighboring H11C4N2CdI3 molecules are interconnected in a quasi-1D zigzag chain along the c-direction, which is further arranged in quasi-2D layers parallel to the bc plane (Fig. 2b). These quasi-2D layers are stacked upward along the a-axis, resulting in the structure of H11C4N2CdI3 (Fig. 2c).
 |
| Fig. 2 Views of the structures of the H11C4N2CdI3 molecule (a), the quasi-2D H11C4N2CdI3 layer along the a-axis (b) and H11C4N2CdI3 along the b-axis. | |
Structural comparison
It is interesting to compare the structural differences of the title compounds to further understand why H11C4N2CdI3 is a polar compound while H12C4N2CdI4 is not. Firstly, we believe that the distinct coordination environments of Cd2+ ions play a key role. In H12C4N2CdI4, the molar ratio of Cd to I is 4, which results in each Cd2+ ion being surrounded by only four I atoms, forming a nearly undistorted CdI4 tetrahedron. In contrast, in H11C4N2CdI3, Cd: I is 3, which enables the N atom on the H10C4N2 unit to participate in Cd2+ coordination, forming a distorted CdNI3 tetrahedron. Secondly, the calculated dipole moments of the CdI4 and CdNI3 units are 3.27 and 5.94 D (Table S10†), respectively, indicating that CdNI3 exhibits more anisotropy, favoring the formation of a polar structure. Finally, the arrangement of Cd2+-centered tetrahedra can also affect the polarity. In H12C4N2CdI4, each H12C4N22+ cation is surrounded by four CdI4 tetrahedra, and their dipole moments cancel out each other along the a, b, and c axes (Table S10†). As a result, although H12C4N2CdI4 has a noncentrosymmetric structure, it is nonpolar, and its net dipole moment in a unit cell is zero. However, in H11C4N2CdI3, the dipole moments of CdNI3 tetrahedra cancel out each other only along the b axis, while they add up along the a and c axes, resulting in a large net dipole moment of about 17.09 D (Table S10†). Therefore, H11C4N2CdI3 is a noncentrosymmetric and polar compound.
Thermal and optical properties
Thermogravimetric analysis (TGA) shows the good thermal stabilities of H12C4N2CdI4 and H11C4N2CdI3, at around 300 °C (Fig. 3a). These values are higher than those of previously reported NLO organic–inorganic halides, including (H7C3N6)(H6C3N6)HgCl3 (225 °C),16 (CH3NH3)GeBr3 (500 K),30, [C18H21N4][AgI3]I (260 °C)18 and Rb2[PbI2(HCOO)2] (240 °C).64 The UV-vis spectra show the absorption edges of 291 and 289 nm for H12C4N2CdI4 and H11C4N2CdI3, respectively (Fig. S4†). Furthermore, compared to H12C4N2CdI4, H11C4N2CdI3 exhibits a slightly larger bandgap (4.10 eV vs. 3.86 eV) (Fig. 3b), which can primarily be attributed to a reduction in the number of I− ions. Besides, the bandgaps of H12C4N2CdI4 and H11C4N2CdI3 are wider than that of the NLO o-containing SCALP cation, such as (C4H10NO)PbCl3 (3.55 eV),65 (C7H15NCl)SbCl4 (3.05 eV),66 (C6H5(CH2)4NH3)4BiI7·H2O (2.29 eV),41 Rb2[PbI2(HCOO)2] (240 °C) (3.40 eV),64, (R/S-C16H22N2Cl)2SnCl6 (3.39 eV)67 and (CH3NH3)GeBr3 (2.91 eV).30
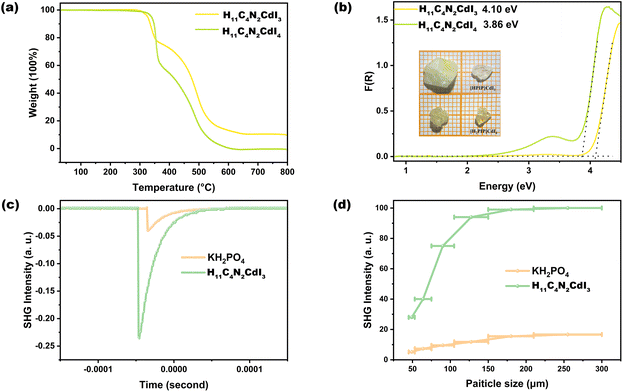 |
| Fig. 3 TGA curves (a), UV-vis diffuse reflectance spectra (inset picture is the crystal photo) (b), measured oscilloscope traces of the SHG signals (150–210 mm) (c) and SHG intensity vs. particle size of compounds under 1064 nm laser radiation (d). KDP served as the reference. | |
SHG properties
Since both H12C4N2CdI4 and H11C4N2CdI3 are noncentrosymmetric, their SHG effects are measured according to the method of Kurtz and Perry.55 For H12C4N2CdI4, as shown in Fig. S5,† the measurement result reveals unwanted non-phase matchable NLO properties, in which the SHG intensity of the crystalline samples exhibits an initial increase, followed by a subsequent decrease as the particle size increased. The maximum value, which is approximately 90 μm, is observed to be about 0.5 times that of KH2PO4 (KDP). This phenomenon is reminiscent of the behavior exhibited by traditional phosphate and sulfate compounds, where the CdI4 tetrahedron exhibits minimal distortion, and its optical anisotropy is mutually canceled within the P212121 space group. Conversely, as shown in Fig. 3c and d, the SHG intensity gradually increased and saturated with increasing particle size, indicating that H11C4N2CdI3 exhibits phase-matching NLO properties. The SHG effect of H11C4N2CdI3 is about six times that of KDP, measured at a particle size of 150–210 μm.
It is interesting to investigate the structure–property relationship among the title compounds and formerly reported Cd-centered NLO OIMHs, such as [(CH3)3NCH2Cl]CdCl3,46 (H10C4NO)2Cd2Cl6,42 (L/D-Hpro)2Cd5Cl12,43 (L/D-Hpro)(L-pro)CdCl3,43 and L/D-C12H20N6O4Cd2Cl5.44 The structure of [(CH3)3NCH2Cl]CdCl3 contains a 0D CdCl6 octahedron, whereas in (L/D-Hpro)2Cd5Cl12 and (L/D-Hpro)(L-pro)CdCl3, the CdCl6 octahedra are inter-connected into the 2D Cd5Cl12 layer via edge-sharing and the 1D CdCl3 chain via face-sharing, respectively. Notably, such CdCl6 octahedra only exhibit low optical anisotropy, with very small dipole moments (<0.1 D). Hence, their SHG response is relatively weak (<1.0 × KDP). Besides, the N/O atoms in non-π-conjugated organic groups are sp3 hybridized, which makes them more likely to form coordination bonds with Cd2+ cations in non-π-conjugated OIMHs. Therefore, many of them feature an O/N-hybridized polyhedron. In (H10C4NO)2Cd2Cl6, neighbouring CdCl6 and CdCl4O2 octahedra are bridged into a 1D chain via edge-sharing. L/D-C12H20N6O4Cd2Cl5 contains a CdOCl4 trigonal bipyramid, and each pair of them is formed into a Cd2O2Cl6 dimer via edge-sharing. Importantly, these Cd–O–Cl polyhedra feature larger dipole moments (1.14–5.03 D) that a CdCl6 octahedron. However, for example, the undesired arrangement of CdOCl4 groups leads to a small net dipole moment (1.19–1.20 D) and weak SHG response in L/D-C12H20N6O4Cd2Cl5 (∼0.2 × KDP). Most importantly, in this work, H12C4N2CdI4 and H11C4N2CdI3 involve CdI4 (3.27 D) and CdNI3 (5.94 D) tetrahedra, respectively, in which the polarization magnitudes of CdNI3 tetrahedra are mutually superimposed along the a- and c-axes resulting in a large net dipole moment of H11C4N2CdI3 (17.09 D) (Table S10†). Accordingly, compared with previously reported Cd-centered OIMHs and H12C4N2CdI4, H11C4N2CdI3 features a far large SHG response (6 × KDP).
Besides, importantly, compared with recently reported NLO OIMHs with SCALP or other d10-TM cations such as (C4H10NO)PbBr3 (0.81 × KDP),65 [C(NH2)3]SbF4 (2 × KDP),32 [N(CH3)4]HgBrI2 (4.5 × KDP),68 (H7C3N6)(H6C3N6)HgCl3 (5.8 × KDP),16 α-(CN3H6)3Cu2I5 (1.8 × KDP),19 (CH3NH3)GeBr3 (5.3 × KDP)30 and (C20H20P)CuCl2 (1.1 × KDP),69 H11C4N2CdI3 has a stronger SHG response (6 × KDP). Furthermore, the SHG responses and bandgaps of selected organic–inorganic halides are summarized in Fig. 4 and Table S11† (the relevant references are provided in the ESI†). Unlike other NLO crystals, H11C4N2CdI3 simultaneously exhibits both a strong SHG effect (>5 × KDP) and wide bandgap (>4.0 eV), which makes it promising as a high-performance ultraviolet NLO crystal.
 |
| Fig. 4 SHG response and energy bandgap of representative NLO organic–inorganic halides. Red ball: compound containing both a π-conjugated organic group and SCALP cation, red triangle: compound containing a π-conjugated organic group but without a SCALP cation, blue ball: compound without a π-conjugated organic group but with a SCALP cation, blue triangle: other compounds. | |
Structure–property relationship analysis
The calculated bandgaps of H12C4N2CdI4 and H11C4N2CdI3 are provided in Fig. S6,† indicating that they are direct bandgap compounds with values of 3.895 and 4.102 eV for H12C4N2CdI4 and H11C4N2CdI3, respectively, being comparable to the results from measurement (3.86 and 4.10 eV). Partial density of states (PDOS) of H12C4N2CdI4 and H11C4N2CdI3 is presented in Fig. 5a and b, respectively. The PDOS plots of both compounds are similar. The contribution to the valence band maximum (VBM) mainly comes from the I-p orbitals, as well as a small portion of the Cd-p orbitals. For the conduction band minimum (CBM), the contribution sizes rank in the order of Cd-s, I-p, and I-s orbitals. It is worth noting that in H11C4N2CdI3, a small amount of N-p orbitals also contributes to the VBM, which is consistent with the existence of the Cd–N bond. Therefore, we believe that the bandgaps of H12C4N2CdI4 and H11C4N2CdI3 are determined by the Cd-centered tetrahedra.
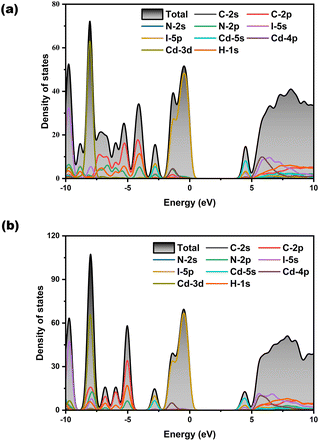 |
| Fig. 5 The density of states of H12C4N2CdI4 and H11C4N2CdI3 (b). | |
Herein, we use the calculated birefringence (Δn) and phase matching wavelength based on the relations between the wavelength and refractive index under both fundamental and harmonic conditions (Fig. 6). Both of them are biaxial crystals with three principal optical axes, i.e., X, Y, and Z. For the orthorhombic crystal H12C4N2CdI4, the calculated refractive index curves after structure optimization show the order of nc > na > nb in the low-frequency range, and to satisfy the biaxial condition nX > nY > nZ, the transformation of c → X, a → Y, and b → Z is performed and a correct order of nX > nY > nZ is shown in Fig. 6a. For the monoclinic crystal H11C4N2CdI3, the crystallographic axes and principal dielectric axes are not consistent with each other in the ac plane, so the principal dielectric axes in the ac plane must be determined after structure optimization and before the optical calculation. According to the principal axis transformation method in our previous work, the rotation angle θ between the original coordinate axes (i.e. y∥b, z∥c) and the principal dielectric axes is found to be 25.179°.70 After the principal axis transformation and based on the principal dielectric coordinate system, the calculated refractive index curves show an order of nX > nY > nZ and satisfy the biaxial condition. The shortest wavelengths for type-I phase matching are approximately 592 nm and 466 nm for H12C4N2CdI4 and H11C4N2CdI3, respectively. This implies that under 1064 nm laser radiation, H11C4N2CdI3 can achieve type-I phase matching, while H12C4N2CdI4 cannot. These findings are consistent with our SHG measurements. These calculated birefringences have been obtained from the refractive index difference (nX – nZ) (Fig. 6c), in which H11C4N2CdI3 possesses a larger birefringence of (0.090 @546 nm, 0.074 @1064 nm) than that of H12C4N2CdI4 (0.052 @546 nm, 0.047 @1064 nm). Since piperazine is a non-π conjugated group with negligible contribution of birefringence, the larger birefringence of H11C4N2CdI3 can be attributed to the microscopic asymmetry and well-arranged structure of the CdNI3 group, exemplified by the dipole moment calculation (Table S10†). Consequently, from H12C4N2CdI4 to H11C4N2CdI3, the polarizable CdNI3 tetrahedron induces an enlarged birefringence, ensuring phase-matching performance of H11C4N2CdI3.
 |
| Fig. 6 Refractive index dispersion curves for fundamental and second-harmonic light of H12C4N2CdI4 (a) and H11C4N2CdI3 (b), as well as the calculated birefringence (c). The type-I phase matching wavelengths in different planes were evaluated based on the calculated refractive index, in which we consider the type-I phase matching condition of n(ω) = n(2ω). | |
We further calculate the theoretical SHG coefficients of H12C4N2CdI4 and H11C4N2CdI3, as shown in Table S12.† For H12C4N2CdI4, it has three non-zero independent SHG tensors with the same value of 0.12 pm V−1, which is about 0.3 times that of KDP (0.39 pm V−1), being consistent with the experimental value (0.5 × KDP). More importantly, for H11C4N2CdI3, its largest independent SHG tensor is d11 = 2.74 pm V−1, about 7 times larger than that of KDP, which is agreement with the experimental result (6 × KDP). To explore the origin of the better SHG effect of H11C4N2CdI3, we further calculated the SHG density distribution of the d11 tensor (Fig. 7). In the VB, the main source of the SHG effect is the I-5p states. In addition, there are small contributions from the Cd 5s states and the N-2p states from the Cd–N bond. The maximum source in the CB is the Cd-5s orbitals, followed by the I-5s orbitals and the N-2p orbitals in the Cd–N bond. Furthermore, the contributions of each unit to the SHG response are calculated, and the CdNI3 tetrahedron has a contribution as high as 97.96%. Therefore, we believe that the distorted CdNI3 tetrahedron is the primary origin of the outstanding SHG effect of H11C4N2CdI3.
 |
| Fig. 7 SHG density plots for H11C4N2CdI3: VB (a) and CB (b). | |
Conclusions
In summary, we have discovered two new organic–inorganic metal halides (OIMHs), H12C4N2CdI4 and H11C4N2CdI3, by combining the protonated pyrazine cation with d10-TM-halide tetrahedra. The NLO properties of non-π-conjugated piperazine compounds are studied for the first time. Despite being a noncentrosymmetric compound, H12C4N2CdI4 exhibits a weak and non-phase-matchable SHG effect. In contrast, H11C4N2CdI3 is a polar compound with excellent NLO performance, including a strong SHG response (6 × KDP), a wide bandgap (4.10 eV), large birefringence (0.074 @1064 nm) for phase-matching requirement, good thermal stability (300 °C), and a suitable crystal growth habit. The structure–property relationship analysis indicates that the highly polarizable N-hybridized CdNI3 tetrahedron in the H11C4N2CdI3 structure is the primary cause of the birefringence and SHG effect. Our work provides new insights for exploring semi-organic NLO crystals that do not contain π-conjugated organic components. Further exploration of OIMHs containing N- or O-hybridized metal-halide polyhedra is currently underway.
Data availability
The experimental or computational data can be obtained via contacting the corresponding author.
Author contributions
Wu Huai-Yu: conceptualization, methodology, writing – original draft, data curation , visualization; Xu Miao-Bin, Chen Qian-Qian, and Ma Nan: data curation; Hu Chun-Li: formal analysis; Huang Xiao-Ying, Du Ke-Zhao and Chen Jin: writing – review & editing, supervision.
Conflicts of interest
There are no conflicts to declare.
Acknowledgements
Our work was supported by the National Natural Science Foundation of China (No. 22205037 and 22031009).
References
- M. Mutailipu, K. R. Poeppelmeier and S. Pan, Borates: A Rich Source for Optical Materials, Chem. Rev., 2021, 121, 1130–1202 CrossRef CAS PubMed.
- J. Chen, C. L. Hu, F. Kong and J. G. Mao, High-Performance Second-Harmonic-Generation (SHG) Materials: New Developments and New Strategies, Acc. Chem. Res., 2021, 54, 2775–2783 CrossRef CAS PubMed.
- L. Kang, F. Liang, X. Jiang, Z. Lin and C. Chen, First-Principles Design and Simulations Promote the Development of Nonlinear Optical Crystals, Acc. Chem. Res., 2020, 53, 209–217 CrossRef CAS PubMed.
- M. Mutailipu and S. Pan, Emergent Deep-Ultraviolet Nonlinear Optical Candidates, Angew. Chem., Int. Ed., 2019, 59, 20302–20317 CrossRef PubMed.
- X. Chen and K. M. Ok, Metal oxyhalides: an emerging family of nonlinear optical materials, Chem. Sci., 2022, 13, 3942–3956 RSC.
- L. Cao, H. Tian, D. Lin, C. Lin, F. Xu, Y. Han, T. Yan, J. Chen, B. Li, N. Ye and M. Luo, A flexible functional module to regulate ultraviolet optical nonlinearity for achieving a balance between a second-harmonic generation response and birefringence, Chem. Sci., 2022, 13, 6990–6997 RSC.
- K. M. Ok, Toward the Rational Design of Novel Noncentrosymmetric Materials: Factors Influencing the Framework Structures, Acc. Chem. Res., 2016, 49, 2774–2785 CrossRef CAS PubMed.
- Y. Pan, S.-P. Guo, B.-W. Liu, H.-G. Xue and G.-C. Guo, Second-order nonlinear optical crystals with mixed anions, Coord. Chem. Rev., 2018, 374, 464–496 CrossRef CAS.
- W. Wang, D. Mei, S. Wen, J. Wang and Y. Wu, Complex coordinated functional groups: a great genes for nonlinear optical materials, Chin. Chem. Lett., 2022, 33, 2301–2315 CrossRef CAS.
- C. Wu, T.-H. Wu, X.-X. Jiang, Z.-J. Wang, H.-Y. Sha, L. Lin, Z.-S. Lin, Z.-P. Huang, X.-F. Long, M. G. Humphrey and C. Zhang, Large Second-Harmonic Response and Giant Birefringence of CeF2(SO4) Induced by Highly Polarizable Polyhedra, J. Am. Chem. Soc., 2021, 143, 4138–4142 CrossRef CAS PubMed.
- D. Lin, M. Luo, C. Lin, F. Xu and N. Ye, KLi(HC3N3O3).2H2O: Solvent-drop Grinding Method toward the Hydro-isocyanurate Nonlinear Optical Crystal, J. Am. Chem. Soc., 2019, 141, 3390–3394 CrossRef CAS PubMed.
- X. Meng, X. Zhang, Q. Liu, Z. Zhou, X. Jiang, Y. Wang, Z. Lin and M. Xia, Perfectly Encoding pi-Conjugated Anions in the RE5(C3N3O3)(OH)12 (RE = Y, Yb, Lu) Family with Strong Second Harmonic Generation Response and Balanced Birefringence, Angew. Chem., Int. Ed., 2023, 62, e202214848 CrossRef CAS PubMed.
- M. Luo, C. Lin, D. Lin and N. Ye, Rational Design of the Metal-Free KBe2BO3F2 (KBBF) Family Member C(NH2)3SO3F with Ultraviolet Optical Nonlinearity, Angew. Chem., Int. Ed., 2020, 59, 15978–15981 CrossRef CAS PubMed.
- J. Lu, X. Liu, M. Zhao, X. B. Deng, K. X. Shi, Q. R. Wu, L. Chen and L. M. Wu, Discovery of NLO Semiorganic (C5H6ON)+(H2PO4)-: Dipole Moment Modulation and Superior Synergy in Solar-Blind UV Region, J. Am. Chem. Soc., 2021, 143, 3647–3654 CrossRef CAS PubMed.
- Q. Q. Chen, C. L. Hu, J. Chen, Y. L. Li, B. X. Li and J. G. Mao, [o-C5H4NHOH]2[I7O18(OH)] 3H2O: An Organic-Inorganic Hybrid SHG Material Featuring an [I7O18(OH)] infinity2- Branched Polyiodate Chain, Angew. Chem., Int. Ed., 2021, 60, 17426–17429 CrossRef CAS PubMed.
- Z. Bai, J. Lee, H. Kim, C. L. Hu and K. M. Ok, Unveiling
the Superior Optical Properties of Novel Melamine-Based Nonlinear Optical Material with Strong Second-Harmonic Generation and Giant Optical Anisotropy, Small, 2023, e2301756, DOI:10.1002/smll.202301756.
- L. Liu, Z. Bai, L. Hu, D. Wei, Z. Lin and L. Zhang, A melamine-based organic–inorganic hybrid material revealing excellent optical performance and moderate thermal stability, J. Mater. Chem. C, 2021, 9, 7452–7457 RSC.
- X. Y. Li, Q. Wei, C. L. Hu, J. Pan, B. X. Li, Z. Z. Xue, X. Y. Li, J. H. Li, J. G. Mao and G. M. Wang, Achieving Large Second Harmonic Generation Effects via Optimal Planar Alignment of Triangular Units, Adv. Funct. Mater., 2022, 33, 2210718 CrossRef.
- J. Wu, Y. Guo, J. L. Qi, W. D. Yao, S. X. Yu, W. Liu and S. P. Guo, Multi-Stimuli Responsive Luminescence and Domino Phase Transition of Hybrid Copper Halides with Nonlinear Optical Switching Behavior, Angew. Chem., Int. Ed., 2023, 62, e202301937 CrossRef CAS PubMed.
- Y. Deng, X. Dong, M. Yang, H. Zeng, G. Zou and Z. Lin, Two low-dimensional metal halides: ionothermal synthesis, photoluminescence, and nonlinear optical properties, Dalton Trans., 2019, 48, 17451–17455 RSC.
- Y. Li, J. Luo and S. Zhao, Local Polarity-Induced Assembly of Second-Order Nonlinear Optical Materials, Acc. Chem. Res., 2022, 55, 3460–3469 CrossRef CAS PubMed.
- M. Mutailipu, Z. Yang and S. Pan, Toward the Enhancement of Critical Performance for Deep-Ultraviolet Frequency-Doubling Crystals Utilizing Covalent Tetrahedra, Acc. Mater. Res., 2021, 2, 282–291 CrossRef CAS.
- X. Meng, W. Yin and M. Xia, Cyanurates consisting of intrinsic planar π-conjugated 6-membered rings: an emerging source of optical functional materials, Coord. Chem. Rev., 2021, 439, 213916 CrossRef CAS.
- S. Hongkang, Y. Ziting, H. Xueling and Z. Min, Fluorooxoborates: a precious treasure of deep-ultraviolet nonlinear optical materials, Chin. J. Struct. Chem., 2023, 42, 100027 CrossRef.
- Z. Boxuan and C. Zhaohui, Recent advances of inorganic phosphates with UV/DUV cutoff edge and large second harmonic response, Chin. J. Struct. Chem., 2023, 42, 100033 CrossRef.
- L. Qing-Fen, C. Wei-Feng, L. You-Zhao and C. Jian-Wen, Recent progress in ultraviolet and deep-ultraviolet nonlinear optical aluminoborates, Chin. J. Struct. Chem., 2023, 42, 100036 CrossRef.
- C. Xu, L. Yanqiang, L. Junhua and Z. Sangen, Recent advances in non-π-conjugated nonlinear optical sulfates with deep-UV absorption edge, Chin. J. Struct. Chem., 2023, 42, 100044 CrossRef.
- F. Wu, Q. Wei, X. Li, Y. Liu, W. Huang, Q. Chen, B. Li, J. Luo and X. Liu, Cooperative Enhancement of Second Harmonic Generation in an Organic–Inorganic Hybrid Antimony Halide, Cryst. Growth Des., 2022, 22, 3875–3881 CrossRef CAS.
- S. Qi, P. Cheng, X. Han, F. Ge, R. Shi, L. Xu, G. Li and J. Xu, Organic–Inorganic Hybrid Antimony(III) Halides for Second Harmonic Generation, Cryst. Growth Des., 2022, 22, 6545–6553 CrossRef CAS.
- Y. Liu, Y. P. Gong, S. Geng, M. L. Feng, D. Manidaki, Z. Deng, C. C. Stoumpos, P. Canepa, Z. Xiao, W. X. Zhang and L. Mao, Hybrid Germanium Bromide Perovskites with Tunable Second Harmonic Generation, Angew. Chem., Int. Ed., 2022, 61, e202208875 CrossRef CAS PubMed.
- X. Li, Y. Guan, X. Li and Y. Fu, Stereochemically Active Lone Pairs and Nonlinear Optical Properties of Two-Dimensional Multilayered Tin and Germanium Iodide Perovskites, J. Am. Chem. Soc., 2022, 144, 18030–18042 CrossRef CAS PubMed.
- W. Zhang, W. Jin, S. Han, Z. Yang and S. Pan, Pb3Ba7B7O20F: a new nonlinear optical material exhibiting large second harmonic generation response induced by its unprecedented Pb-B-O framework, Scr. Mater., 2021, 194, 113700 CrossRef CAS.
- Z. Qi, Y. Chen, H. Gao, F.-Q. Zhang, S.-L. Li and X.-M. Zhang, Two SbX5-based isostructural polar 1D hybrid antimony halides with tunable broadband emission, nonlinear optics, and semiconductor properties, Sci. China: Chem., 2021, 64, 2111–2117 CrossRef CAS.
- K. Liu, C. Deng, C. Li, X. Zhang, J. Cao, J. Yao, J. Zhao, X. Jiang, Z. Lin and Q. Liu, Hybrid Metal-Halide Infrared Nonlinear Optical Crystals of (TMEDA)MI5 (M = Sb, Bi) with High Stability, Adv. Opt. Mater., 2021, 9, 2101333 CrossRef CAS.
- J.-H. Wu, B. Zhang, T.-K. Jiang, F. Kong and J.-G. Mao, From Cs8Sb4Nb5O5F35 to Cs6Sb4Mo3O5F26: the first noncentrosymmetric fluoroantimonite with d0 transition metal, Chin. J. Struct. Chem., 2023, 42, 100016 CrossRef.
- J. Chen, C.-L. Hu, Y.-L. Lin, Y. Chen, Q.-Q. Chen and J.-G. Mao, K3V2O3F4(IO3)3: a high-performance SHG crystal containing both five and six-coordinated V5+ cations, Chem. Sci., 2022, 13, 454–460 RSC.
- J. Chen and K.-Z. Du, ZrF2(IO3)2 and RbGaF3(IO3): Two Promising Birefringent Crystals Featuring 1D Metal-Fluoride Cationic Chains and Wide Bandgaps, Inorg. Chem., 2022, 61, 17893–17901 CrossRef CAS PubMed.
- J. Chen, C.-L. Hu, X.-H. Zhang, B.-X. Li, B.-P. Yang and J.-G. Mao, CsVO2F(IO3): An Excellent SHG Material Featuring an Unprecedented 3D [VO2F(IO3)]− Anionic Framework, Angew. Chem., Int. Ed., 2020, 59, 5381–5384 CrossRef CAS PubMed.
- J. Chen, C.-L. Hu, F.-F. Mao, B.-P. Yang, X.-H. Zhang and J.-G. Mao, REI5O14 (RE = Y and Gd): Promising SHG Materials Featuring the Semicircle-Shaped I5O143ˉ Polyiodate Anion, Angew. Chem., Int. Ed., 2019, 58, 11666–11669 CrossRef CAS PubMed.
- J. Chen, C.-L. Hu, F.-F. Mao, J.-H. Feng and J.-G. Mao, A Facile Route to Nonlinear Optical Materials: Three-Site Aliovalent Substitution Involving One Cation and Two Anions, Angew. Chem., Int. Ed., 2019, 58, 2098–2102 CrossRef CAS PubMed.
- D. Chen, S. Hao, L. Fan, Y. Guo, J. Yao, C. Wolverton, M. G. Kanatzidis, J. Zhao and Q. Liu, Broad Photoluminescence and Second-Harmonic Generation in the Noncentrosymmetric Organic–Inorganic Hybrid Halide (C6H5(CH2)4NH3)4MX7·H2O (M = Bi, In, X = Br or I), Chem. Mater., 2021, 33, 8106–8111 CrossRef CAS.
- D. Sun, D. Wang, Y. Dang, S. Zhang, H. Chen, R. Hou, K. Wu and C. Shen, Organic-Inorganic Hybrid Noncentrosymmetric (Morpholinium)2Cd2Cl6 Single Crystals: Synthesis, Nonlinear Optical Properties, and Stability, Inorg. Chem., 2022, 61, 8076–8082 CrossRef CAS PubMed.
- J. Cheng, Y. Deng, X. Dong, J. Li, L. Huang, H. Zeng, G. Zou and Z. Lin, Homochiral Hybrid Organic-Inorganic Cadmium Chlorides Directed by Enantiopure Amino Acids, Inorg. Chem., 2022, 61, 11032–11035 CrossRef CAS PubMed.
- W. Seo and K. M. Ok, Novel noncentrosymmetric polar coordination compounds derived from chiral histidine ligands, Inorg. Chem. Front., 2021, 8, 4536–4543 RSC.
- H. Cheng, C. Cao, S. Teng, Z. Zhang, Y. Zhang, D. Wang, W. Yang and R. Xie, Sn(II)-doped one-dimensional hybrid metal halide [C5H14NO]CdCl3 single crystals with broadband greenish-yellow light emission, Dalton Trans., 2023, 52, 1021–1029 RSC.
- C. Shen, J. Liu, K. Wu, L. Xu, D. Sun, Y. Dang, J. Wang and D. Wang, High stability and moderate second-order nonlinear optical properties of hybrid lead-free perovskite [(CH3)3NCH2Cl]CdCl3 bulk crystals, CrystEngComm, 2023, 25, 2264–2270 RSC.
- W. Jin, W. Zhang, A. Tudi, L. Wang, X. Zhou, Z. Yang and S. Pan, Fluorine-Driven Enhancement of Birefringence in the Fluorooxosulfate: A Deep Evaluation from a Joint Experimental and Computational Study, Adv. Sci., 2021, 8, 2003594, DOI:10.1002/advs.202003594.
- Y. Liu, Y. Liu, Z. Lin, Y. Li, Q. Ding, X. Chen, L. Li, S. Zhao, M. Hong and J. Luo, Nonpolar Na10Cd(NO3)4(SO3S)4 Exhibits a Large Second-Harmonic Generation, CCS Chem., 2021, 694–699, DOI:10.31635/ccschem.021.202000758.
- X. Hao, M. Luo, C. Lin, G. Peng, F. Xu and N. Ye, M(NH2SO3)2 (M=Sr, Ba): Two Deep-Ultraviolet Transparent Sulfamates Exhibiting Strong Second Harmonic Generation Responses and Moderate Birefringence, Angew. Chem., Int. Ed., 2021, 60, 7621–7625 CrossRef CAS PubMed.
- H. Tian, C. Lin, X. Zhao, F. Xu, C. Wang, N. Ye and M. Luo, Ba(SO3CH3)2: a Deep-Ultraviolet Transparent Crystal with Excellent Optical Nonlinearity Based on a New Polar Non-π-conjugated NLO Building Unit SO3CH3-, CCS Chem., 2023, 1–22, DOI:10.31635/ccschem.022.202202582.
- R. H. Blessing, An empirical correction for absorption anisotropy, Acta Crystallogr., Sect. A: Found. Crystallogr., 1995, 51, 33–38 CrossRef PubMed.
- G. M. Sheldrick, Crystal structure refinement with SHELXL, Acta Crystallogr., Sect. C: Struct. Chem., 2015, 71, 3–8 Search PubMed.
- A. Spek, Single-crystal structure validation with the program PLATON, J. Appl. Crystallogr., 2003, 36, 7–13 CrossRef CAS.
- P. Kubelka and F. Munk, An article on optics of paint layers, Z. Tech. Phys, 1931, 12, 259–274 Search PubMed.
- S. K. Kurtz and T. T. Perry, A Powder Technique for the Evaluation of Nonlinear Optical Materials, J. Appl. Phys., 1968, 39, 3798–3813 CrossRef CAS.
- M. D. Segall, P. J. D. Lindan, M. J. Probert, C. J. Pickard, P. J. Hasnip, S. J. Clark and M. C. Payne, First-principles simulation: ideas, illustrations and the CASTEP code, J. Phys.: Condens.Matter, 2002, 14, 2717 CrossRef CAS.
- V. Milman, B. Winkler, J. A. White, C. J. Pickard, M. C. Payne, E. V. Akhmatskaya and R. H. Nobes, Electronic structure, properties, and phase stability of inorganic crystals: a pseudopotential plane-wave study, J. Quantum Chem., 2000, 77, 895–910 CrossRef CAS.
- J. P. Perdew, K. Burke and M. Ernzerhof, Generalized gradient approximation made simple, Phys. Rev. Lett., 1996, 77, 3865 CrossRef CAS PubMed.
- J. S. Lin, A. Qteish, M. C. Payne and V. Heine, Optimized and transferable nonlocal separable ab initio pseudopotentials, Phys. Rev. B: Condens. Matter Mater. Phys., 1993, 47, 4174 CrossRef PubMed.
- J. Lin, M.-H. Lee, Z.-P. Liu, C. Chen and C. J. Pickard, Mechanism for linear and nonlinear optical effects in β-BaB2O4 crystals, Phys. Rev. B: Condens. Matter Mater. Phys., 1999, 60, 13380 CrossRef CAS.
- S. N. Rashkeev, W. R. Lambrecht and B. Segall, Efficient ab initio method for the calculation of frequency-dependent second-order optical response in semiconductors, Phys. Rev. B: Condens. Matter Mater. Phys., 1998, 57, 3905 CrossRef CAS.
- H. Tllili, S. Walha, S. Elleuch, B. Fares Ali and H. Naïli, Structural, vibrational, DFT and optical studies of a new non-centrosymmetric hybrid material (C4H12N2)[CoBr4], J. Mol. Struct., 2018, 1152, 303–310 CrossRef CAS.
-
J.-H. Yu, Q. Hou, T.-G. Wang, X. Zhang and J.-Q. Xu, Structure characterization of 1:1 adducts of metal(II) halides and piperazine, Elsevier Inc., 2007, vol. 180, pp. 518–522 Search PubMed.
- X.-Y. Zhang, Z.-Q. Zhou, W.-X. Bao, H.-X. Tang, R.-B. Fu, Z.-J. Ma and X.-T. Wu, New lead-iodide formates with a strong second-harmonic generation response and suitable birefringence obtained by the substitution strategy, Chem. Sci., 2023, 14, 136–142 RSC.
- C. Shen, D. Sun, Y. Dang, K. Wu, T. Xu, R. Hou, H. Chen, J. Wang and D. Wang, (C4H10NO)PbX3 (X = Cl, Br): Design of Two Lead Halide Perovskite Crystals with Moderate Nonlinear Optical Properties, Inorg. Chem., 2022, 61, 16936–16943 CrossRef CAS PubMed.
- J.-M. Gong, T. Shao, P.-Z. Huang, C.-Y. Su, M. Chen, D.-W. Fu and H.-F. Lu, Reversible Phase Transition and Second-Harmonic Response Based on a Zero-Dimensional Organic–Inorganic Hybrid Compound, J. Phys. Chem. C, 2022, 126, 15274–15279 CrossRef CAS.
- X. Han, P. Cheng, R. Shi, Y. Zheng, S. Qi, J. Xu and X. H. Bu, Linear optical afterglow and nonlinear optical harmonic generation from chiral tin(IV) halides: the role of lattice distortions, Mater. Horiz., 2023, 10, 1005–1011 RSC.
- C. Yang, X. Liu, C. Teng, Q. Wu and F. Liang, Syntheses, structure and properties of a new series of organic-inorganic Hg-based halides: adjusting halogens resulted in huge performance mutations, Dalton Trans., 2021, 50, 7563–7570 RSC.
- J.-L. Qi, J. Wu, Y. Guo, Z.-P. Xu, W. Liu and S.-P. Guo, Quasi-linear CuX2 (X = Cl, Br) motif-built hybrid copper halides realizing encouraging nonlinear optical activities, Inorg. Chem. Front., 2023, 10, 3319–3325 RSC.
- F.-F. Mao, C.-L. Hu, X. Xu, D. Yan, B.-P. Yang and J.-G. Mao, Bi(IO3)F2: The First Metal Iodate Fluoride with a Very Strong Second Harmonic Generation Effect, Angew. Chem., Int. Ed., 2017, 56, 2151–2155 CrossRef CAS PubMed.
|
This journal is © The Royal Society of Chemistry 2023 |
Click here to see how this site uses Cookies. View our privacy policy here.